Carbon-vacancy engineering approach to g-C3N4 for selective 5-hydroxymethylfurfural oxidation coupled with H2O2 production†
Received
7th July 2023
, Accepted 24th September 2023
First published on 5th October 2023
Abstract
Photocatalytic H2O2 production and selective oxidation of 5-hydroxymethylfurfural (HMF) occur in synergy, which improves the utilization of photogenerated carriers. In this paper, g-C3N4 (CN) under defect engineering was used in the photocatalytic production of H2O2 and HMF oxidation. It was found that H2O2 productivity has the order of Cv-CN (283.9 μmol g−1 h−1) > Nv-CN (241.4 μmol g−1 h−1) > CN (154.0 μmol g−1 h−1), the DFF yield correlates positively with the above order, and that of Cv-CN reached as high as 50.7 μmol L−1. The yield of H2O2 and 2,5-dicarbonylfuran (DFF) was increased by 1.7 times and 6.3 times compared with the original CN, respectively. The lowest PL intensity and longest fluorescence lifetime indicate higher charge separation efficiency of Cv-CN. DFT calculations show that the electron–hole separation on Cv-CN is better compared to CN and the formation of H2O2 on Cv-CN has a lower energy barrier, which will be beneficial for the adsorption/activation of O2. Selective oxidation of HMF to DFF using noble metal-free catalysts under mild reaction conditions combined with H2O2 production can both reduce the electron/hole complexation rate and increase the added value of the reaction. This designed bifunctional photocatalytic system may be more feasible and practical from a sustainable and green development perspective. This work also thus provides a new method/material for photocatalytic production of H2O2 and selective oxidation of HMF.
1. Introduction
Hydrogen peroxide (H2O2) is considered as a potential clean and high-energy oxidant, which is widely applied in biological processes, environmental remediation and the chemical industry. At present, the main method for large-scale production of H2O2 is an anthraquinone oxidation method.1 However, this method has serious drawbacks in terms of environmental pollutants. Therefore, the semiconductor photocatalytic process to generate H2O2 has attracted a lot of attention due to the use of abundant and renewable sunlight as a driving force.2
Recently, graphitic carbon nitride (CN) has been widely used for H2O2 production due to its visible light response and stability.3 As a typical organic semiconductor photocatalyst, CN has a moderate bandgap size (∼2.7 eV) to utilize visible light up to 460 nm,4 while the energy band position can satisfy the thermodynamic requirements for the generation of H2O2, and its unique tri-s-triazine structure can exhibit excellent catalytic activity for organic transformations.5 CN provides a large number of catalytic reaction sites owing to its high surface area and sheet-like morphology.6 But the pristine CN exhibits easy recombination of electron–hole pairs. Therefore, many modification approaches have been proposed to overcome these disadvantages, including structure design,7 and defect engineering.1,8,9 Generally, the π-conjugated structure of CN is symmetrical, and the photogenerated charge migration is anisotropic and easy to quench.10 Introducing vacancies and/or heteroatoms on the CN structure can act as trapping or adsorption sites to boost e−/h+ separation. Meanwhile, the band structure and light absorption of CN can be tuned. Hence, C or N vacancies (Cv or Nv) have been introduced into CN to improve its photocatalytic activity. The enhanced activity of defected CN nanotubes was ascribed to the improved light absorption, high surface area, enhanced photogenerated charge separation and transfer.11 Wang et al.12 demonstrated that Cv and Nv contribute to extending the absorption band of visible light and suppressing carrier recombination, respectively. This may be because vacancies can act as donors or acceptors of electrons and capture specific carriers, thus reducing the recombination rate.13,14
As shown for H2O2 photo-production, Li et al.1 found that H2O2 could be effectively produced by CN in a water/alcohol mixture under visible light irradiation. However, in the absence of alcohol (acting as an organic scavenger), the efficacy of pure CN was very low. According to previous reports, vacancy defects have been found to be able to trap electrons, meanwhile, they can also enhance the adsorption/activation of O2, but the underlying reason for this, such as the localized electrons charge distribution, is unclear. As mentioned above, the use of scavengers can lead to increased production costs and loss of hole oxidation capacity while increasing the yield of H2O2.15 Thus, the replacement of traditional scavengers (tert-butanol, ethanol), in photocatalytic H2O2 evolution by renewable biomass has recently received wide attention.
As we know, 5-hydroxymethylfurfural (HMF) is a value-added biomass-derived chemical, which can be converted into 2,5-furandicarboxylic acid, furfuryl alcohol, and other important chemicals.16–19 Palmisano et al. used CN as a photocatalyst to selectively oxidize HMF to generate DFF. However, the efficacy of pure CN was low in the absence of defect-engineered modifications.20,21 As an excellent organic monomer, DFF has an excellent rigid structure and can be used to prepare various organic porous materials including benzene organics.22 Vacancies engineering may be an effective strategy to optimize catalytic performance and selective conversion of biomass-relevant substrates.23 g-C3N4 has also shown enormous potential in the field of photocatalytic selective oxidation of HMF.24 Thus, coupling the selective oxidation of HMF with the photocatalytic generation of H2O2, high yields of DFF and H2O2 can be obtained simultaneously without loss of hole oxidation capacity.
Thus, in this work, a carbon-vacancy engineering approach to CN was prepared by a calcination method. The catalytic performance of CN, Cv-CN and Nv-CN was compared, and their physical and chemical properties were investigated by a series of characterizations. Combined with DFT calculations, the structure–catalytic effect relationship was analyzed at the molecular level. This study proposes a strategy for vacancy modulation of the photo-absorption and charge separation processes, and this work is expected to provide a reference for the design of photocatalysts with a wide spectral response range and fast charge separation capability.
2. Experiments and DFT calculations
2.1 Synthesis of bulk carbon nitride
Bulk carbon nitride was fabricated by a heat treatment with melamine. Melamine was sintered under an air atmosphere for 3 h at 550 °C in a muffle furnace at a heating rate of 5 °C min−1. The light yellow solid obtained after roasting was fully ground in an agate mortar, and the powder obtained is CN.25
2.2 Synthesis of CN with vacancies
As shown in Scheme 1, for Cv-CN the bulk carbon nitride was placed in a tube furnace and calcined under argon atmosphere at a heating rate of 5 °C min−1 to 520 °C for 4 h. The product was then cooled to room temperature to obtain Cv-CN powder; the resulting agglomerates were fully ground into powder in an agate mortar for further use.1 For Nv-CN, melamine (MA) and NH4Cl were dissolved in deionized water and stirred vigorously at room temperature. Then the MA–NH4Cl mixture was rapidly frozen in liquid nitrogen to form white crystals. The mixture was calcined at 550 °C under nitrogen atmosphere for 4 hours. The product was then cooled to room temperature to obtain Nv-CN powder. The powder was well ground in an agate mortar for further use.26
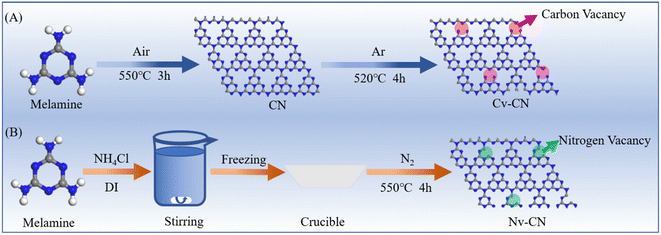 |
| Scheme 1 The synthetic procedure schematic of (A) CV-CN; (B) NV-CN. | |
2.3 Photocatalytic tests
The photocatalytic activity was characterized by photocatalytic selective oxidation of HMF coupled with a H2O2 production experiment. During each photocatalytic experiment, the photocatalyst sample was dispersed in 120 ml of 0.1 mM HMF solution in a vessel with an external cooling water jacket. Afterwards, the photocatalyst dispersion system was stirred in the dark for 0.5 h in an O2 bubble to ensure adsorption–desorption equilibrium between the photocatalyst, dissolved oxygen and water prior to visible light irradiation. Magnetic stirring was performed at ambient pressure and temperature, and approximately 2 mL of suspension was removed from the reaction cell at a given time interval during irradiation, and then filtered to remove the photocatalyst particles for analysis. The concentration of H2O2 solution was analyzed using UV-vis spectra. To determine the yield of the oxidized product (DFF), the catalyst was separated by filtration, and the organic phase was analyzed using HPLC techniques, and the yield of DFF is reported on the basis of the HPLC analysis.27 Before analysis, all liquid products were filtered through 0.45 μm filter membrane, and the products were determined using a high-performance liquid chromatograph (HPLC, Agilent Technologies, CA, USA) equipped with a Kromasil 100-5-C18 column (250 mm × 4.6 mm) and DAD (diode array detector). CH3COOH was selected as the mobile phase with a flow rate of 0.95 mL min−1, the temperature of the column was 30 °C, the injection volume was 20 μL.
The AQYs (apparent quantum yields) were calculated using the following equation:28
where
Ne represents the reaction electrons,
NP represents the incident photons,
M refers to the amount of H
2O
2 molecules,
P is the light intensity determined by photo-radiometer,
S is the light irradiation area,
NA represents the Avogadro constant,
c is the speed of light,
λ is the wavelength of the monochromatic light,
h is the Planck constant, and
t represents the photoreaction time.
For other details see the ESI.†
3. Results and discussion
3.1 Characterizations
As shown in Fig. 1A, XRD tests were performed on the CN, Nv-CN and Cv-CN prepared by the described method. The sample CN shows two diffraction peaks at 2θ = 13.3° and 2θ = 27.4°, which are attributed to the spacing between the corresponding 3-s-triazine ring repeating unit structures of (100) crystalline planes and the interlayer stacking of aromatic groups of (002) crystalline planes, respectively.29 It can be observed that the two characteristic peaks of Nv-CN and Cv-CN were weakened and broadened, indicating the loss of ordered structures within the framework to some extent,30 which may be attributed to the destruction of in-plane long-range order and the larger spacing between layers caused by vacancies.28 A slight shift in the prominent peak was also observed due to the changed stacking distance between the nanosheets.11 At the same time, the weakening of the signal intensity of the diffraction peaks and the shifting of the peaks likewise indicate that the heat treatment destroys the crystallinity of CN and may produce defects.31
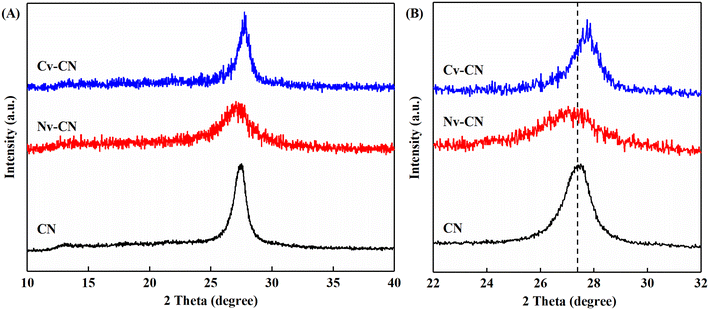 |
| Fig. 1 (A) XRD patterns, and (B) enlarged XRD patterns. | |
We also inspected the FTIR spectra of CN, Nv-CN and Cv-CN. As shown in the Fig. S1,† the bands at 810 cm−1, 1200–1600 cm−1 and 3000–3300 cm−1 can be attributed to the out-of-plane bending vibration of the triazine structure, the typical tensile vibration of the C–N heterocycle and the N–H tensile vibration, which was consistent with the typical infrared spectrum of graphite phase carbon nitride. The FTIR spectra of the defect-modified carbon nitride samples showed no significant changes compared with the carbon nitride, indicating that the basic structure of graphite-like carbon nitride can be well maintained after the vacancy modification. Compared to the CN, the IR signals between 1200 and 1600 cm−1 of the Nv-CN and Cv-CN decreased, which was largely due to part of the C–N heterocycles being broken by introducing the vacancies.26
The morphology and microstructure of the synthesized catalysts are characterized by scanning electron microscopy (SEM) and transmission electron microscopy (TEM). In Fig. 2A, the milled CN shows a typical blocky morphology with apparent agglomeration, which may lead to low specific surface area and light adsorption.32 Compared with bulk CN, the Nv-CN (Fig. S2A†) and Cv-CN exhibit a thin and flat morphology (Fig. 2B), which indicates the successful exfoliation of bulk CN by an argon atmosphere. The defective sites of CN after heat treatment in different atmospheres have a more pronounced and complete nanostructure with some pores, as shown in Fig. 2D, indicating that CN exfoliation occurs in the hot gas flow. The TEM images of Cv-CN in Fig. 2D show a more obvious porous morphology. Further observation of the TEM image of Cv-CN shows that Cv-CN has a sheet-like structure with overlapping layers and contains a large number of in-plane holes.28 The relatively lamellar and transparent morphology of Fig. 2D reveals the formation of nanosheets and a porous structure with a size range of 40–70 nm.33
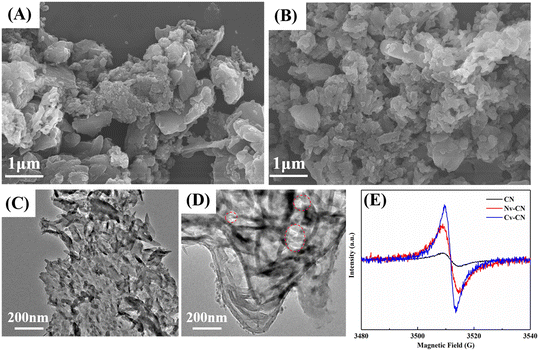 |
| Fig. 2 SEM images of (A) CN and (B) Cv-CN, TEM images of (C) CN and (D) Cv-CN, and (E) ESR spectra. | |
The loss of carbon and nitrogen atoms induces the formation of unpaired electrons in the aromatic rings, which can be demonstrated by ESR spectra to assess the degree of defects.15,34 As shown in Fig. 2E, compared with CN, the successively increasing ESR signal intensity of Nv-CN and Cv-CN proves that the as-prepared CN structure contains defects. Although the ESR signal is not able to distinguish the species of vacant atoms, the stronger ESR intensity of Cv-CN indicates that it possesses the most defects. In order to identify the type of defects formed in the Cv-CN framework, elemental analysis (EA) was used to determine the molar ratio of carbon to nitrogen (C/N) in the products. As shown in Table S1,† the C/N molar ratio of Cv-CN is 0.66, lower than that of CN (0.73). The decrease of C/N ratio further confirms the formation of carbon vacancies.
XPS spectra were measured to acquire the surface element valence state and chemical compositions of the products. As observed in the XPS survey spectra (Fig. S3†), the three samples are mainly composed of C and N elements. The appearance of O 1s peak is probably due to surface adsorbed oxygen species. Specifically, as shown in Fig. 3A, the C 1s spectrum can be deconvoluted into two peaks located at 284.5 eV and 293.3 eV, which are attributed to carbon in adventitious carbon (C–C) and sp2-hybridized carbon (N–C
N) in the conjugated aromatic systems, respectively.35 However, the intensity of the C–C peak of the Cv-CN sample is decreased, which probably implies the absence of some C atoms. In Fig. 3B, the N 1s high-resolution spectrum contains three peaks situated at 398.3 eV, 400.1 eV, and 401.0 eV, which can be ascribed to sp2-hybridized C–N
C(N2C), sp3-hybridized N–(C)3 groups (N3C), and functional groups of C–N–Hx, respectively.36 To characterize the chemical state of the as-prepared photocatalysts, the elemental content of C and N was detected and recorded, as shown in Table S1.† The C/N atomic ratio is commonly used to investigate defect states of samples.37 Compared with CN (3
:
4), Cv-CN shows a decreased C/N atomic ratio (2.65
:
4), indicating the deletion of C atoms in its structure. In contrast, Nv-CN shows an increased C/N atomic ratio (3.54
:
4), indicating the formation of N vacancies in the skeleton, which is in good agreement with ESR results.
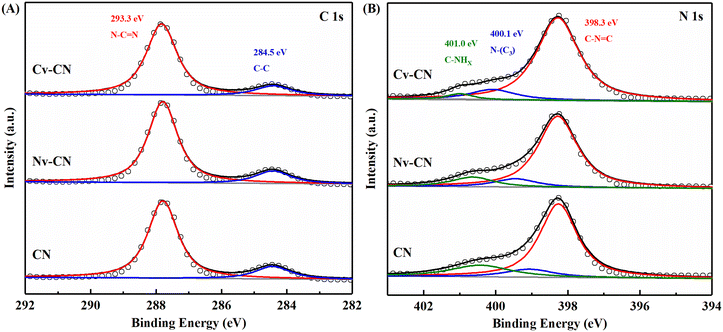 |
| Fig. 3 (A) C 1s and (B) N 1s XPS spectra of the three samples. | |
The influence of the introduction of C vacancies on the electronic band structure and optical properties of CN was analyzed using UV-visible diffuse reflectance absorption spectra. As shown in Fig. 4A, CN exhibits typical semiconductor absorption in the UV and part of the visible region.28 Compared with CN, the presence of vacancies enhanced the optical absorption from 400 to 700 nm compared to the CN samples, with absorption edges of 453, 457 and 475 nm for the three samples CN, Nv-CN and Cv-CN, respectively. The increase in the intensity of adsorption to light can tentatively indicate that carbon vacancies were successfully introduced onto the carbon nitride.
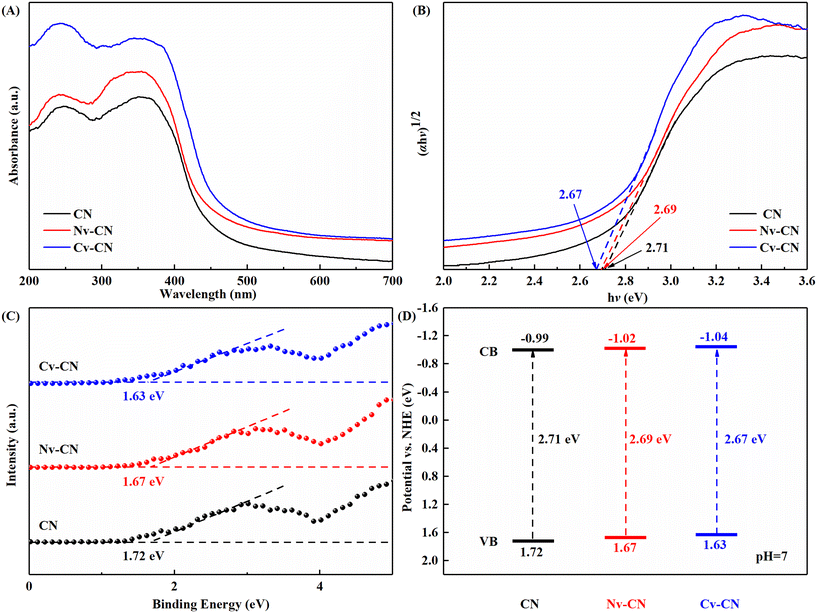 |
| Fig. 4 (A) UV-vis spectra, and (B) Kubelka Munk curves, and (C) XPS valence band spectra, and (D) band structures. | |
Band gap energies of CN and Cv-CN as well as Nv-CN were obtained from the Kubelka Munk plots derived from UV-vis spectra. As shown in Fig. 4B, the band gap energy of CN is 2.71 eV, which is consistent with that reported in the literature.38 Due to the introduction of vacancies in the structure, the band gaps of Nv-CN and Cv-CN are reduced to 2.69 eV and 2.67 eV, respectively. These results clearly indicate that the vacancies can narrow the CN band gap and obtain the best light absorption.39 The excitation of valence band electrons in Cv-CN will be much easier than that in CN.
The valence band (VB) potentials as determined using XPS VB spectra (Fig. 4C) of CN, Nv-CN and Cv-CN are 1.72, 1.67 and 1.63 eV.40 The CB potentials are calculated by using the formula Eg = EVB − ECB (Eg: band gap, EVB:VB potential, ECB: CB potential).1 Hence, the CB positions are located at −0.99, −1.02 and −1.04 eV, respectively (vs. NHE). The determined band structure arrangement is shown in Fig. 4D. The redox potential of HMF/DFF is 1.61 eV, and DFF/oxidized DFF is 2.03 eV.41 Meanwhile, the redox potential of O2/H2O2 is 0.695 eV.1 Therefore, photocatalytic generation of H2O2 and selective oxidation of HMF on Cv-CN are thermodynamically feasible. Particularly, Cv-CN has a more negative CB potential and a narrower band gap, the negative shift of CB potential indicates that electrons have a better reducing ability to trigger the photocatalytic reduction reaction, and the narrowing of the band gap will enhance the light capture ability, which will improve the photocatalytic activity in thermodynamics.
In addition, the optical property of the catalysts can also be characterized by photoluminescence spectra (PL). Fig. 5A exhibits the PL spectra of CN, Nv-CN and Cv-CN at an excitation wavelength of 335 nm, together with UV-340 and UV-39 filters. Compared with the CN, the PL intensity of Nv-CN and Cv-CN are significantly decreased. As is well known, the lower the PL intensity is, the lower the recombination rate of photogenerated charges is. Therefore, the catalysts containing C vacancies and N vacancies can suppress the complexation of photogenerated electron–hole pairs and increase the utilization of light energy, thus further enhancing the reaction efficiency of photocatalysis. Cv-CN exhibits the lowest PL intensity among three samples, implying that introducing C vacancies can effectively suppress the recombination of photo-generated electrons and holes.33
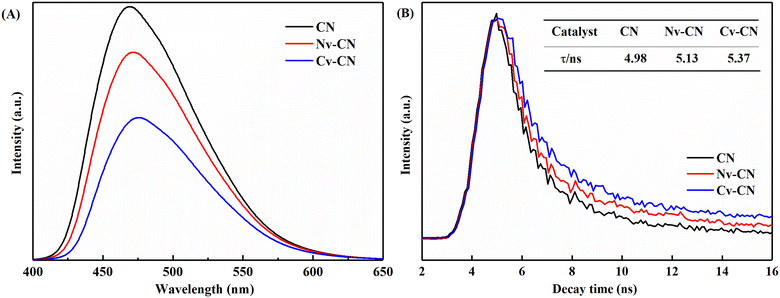 |
| Fig. 5 (A) PL emission spectra, and (B) time-resolved fluorescence spectra. | |
To further investigate the charge carrier lifetimes of CN, Nv-CN and Cv-CN, TRPL (time-resolved photoluminscence) analysis was performed and the results are shown in Fig. 5B. The time-resolved decay curves of visible emission were fitted to the tri-exponential model using eqn (1), and the average decay time was calculated by eqn (2):
| I(t) = A1e−t/τ1 + A2e−t/τ2 + A3e−t/τ3 | (1) |
| τ = (A1τ12 + A2τ22 + A3τ32)/(A1τ1 + A2τ2 + A3τ3) | (2) |
where
Ai (
i = 1, 2 and 3) is the prefactor;
τ1 (
i = 1, 2 and 3) is the excited state luminescence decay time (ns) associated with the sample components. According to the calculations, Nv-CN and Cv-CN exhibit slower decay indices with average lifetimes of 5.13 and 5.37 ns, respectively, with higher fluorescence lifetimes than CN. As shown in
Fig. 5B, the fluorescence lifetimes were in accordance with the sequence of Cv-CN (5.37 ns) > Nv-CN (5.13 ns) > CN (4.98 ns), indicating that Cv-CN has a higher charge separation efficiency, which is consistent with the results of the PL spectra. The above results indicate that C vacancies can effectively inhibit the recombination of photogenerated carriers and enhance the transport and utilization of photogenerated carriers.
In the photocatalytic process, the photocurrent originates from the excitation of electrons from the valence band to the conduction band after the semiconductor is irradiated by light. To further reveal the enhanced activity of Cv-CN, the photocurrent response of CN, Nv-CN and Cv-CN was measured. In Fig. 6A, the saturation photocurrent densities of Cv-CN and Nv-CN are greater than that of CN, while Cv-CN has the strongest photocurrent response. Similarly, in Fig. 6B, the smallest semicircle diameter of the Nyquist plot indicates the smallest migration resistance of carriers in Cv-CN.42 These results indicate that CN obtained by CN calcination under argon flow has the best photogenerated carrier separation efficiency, which facilitates the migration of more carriers to the photocatalyst surface for subsequent redox reactions. This may be due to the fact that both C and N vacancies can act as electron traps and inhibit the radiative recombination of photogenerated charges.43,44
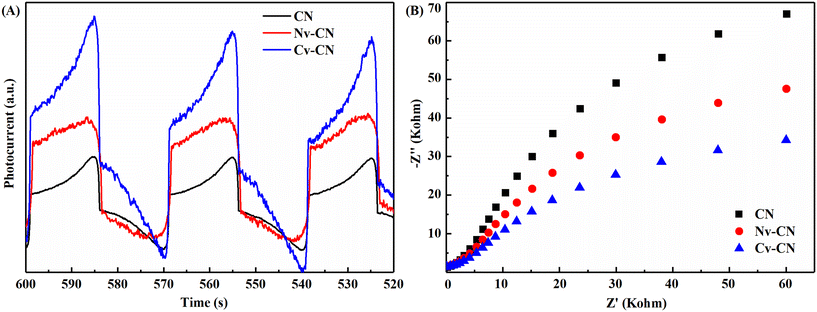 |
| Fig. 6 (A) I–t curves, and (B) EIS plots. | |
3.2 Photocatalytic performance
We conducted experiments on the photocatalytic production of H2O2 coupled with selective oxidation of HMF under visible light. The amount of H2O2 catalyzed on the three catalysts increased with time until equilibrium was reached. As shown in Fig. 7A, the lowest H2O2 yield was 111.1 μmol for CN and the highest H2O2 yield reached 187.9 μmol for Cv-CN, and the order of H2O2 yield for the three materials was Cv-CN (187.9 μmol) > Nv-CN (159.0 μmol) > CN (111.1 μmol), indicating that the construction of C vacancies could provide more chemisorption sites to improve the catalytic performance of CN. Compared with CN, the photocatalytic performances of Nv-CN and Cv-CN were significantly improved, the highest yield of H2O2 was 1.43 and 1.69 times of the original CN, respectively. In addition, we tested the AQY of the photocatalysts under monochromatic light (λ = 420 nm) to more intuitively characterize the photocatalytic efficiency. Fig. S4† shows the comparison of AQY values (H2O2) of CN, Nv-CN and Cv-CN: 6.94% (Cv-CN) > 5.87% (Nv-CN) > 4.11% (CN). Therefore, the CN photocatalyst can improve its overall photocatalytic efficiency through controllable defect engineering. As shown in Fig. S5,† the decomposition of H2O2 on Nv-CN was almost 30% while that on Cv-CN was 27% indicating that Cv-CN could inhibit the decomposition of H2O2 to some extent.
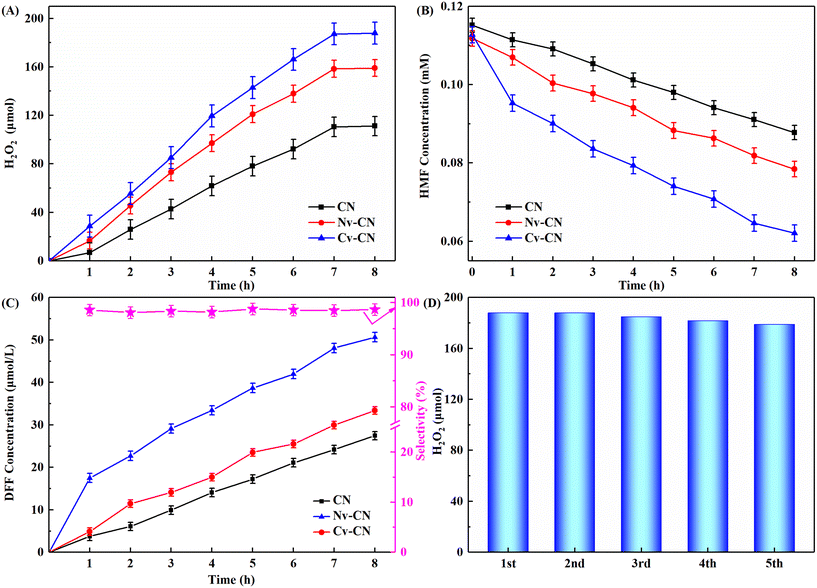 |
| Fig. 7 (A) Photocatalytic H2O2 production, (B) HMF degradation, (C) DFF formation and selectivity, and (D) reusability of Cv-CN. | |
The concentration change of HMF solution under different catalysts is shown in Fig. 7B. The conversion of HMF increased with the increase of reaction time, and the oxidation rate of HMF follows the order of Cv-CN > Nv-CN > CN. As the concentration of HMF in the reaction medium continued to decrease, the concentration of DFF increased with the reaction time (Fig. 7C). The yield of DFF has the order of Cv-CN (50.7 μmol L−1) > Nv-CN (33.4 μmol L−1) > CN (27.4 μmol L−1) when O2 was passed through the reaction. The selectivity of DFF is about 98% due to formation of trace byproducts such as 2-formyl-5-furancarboxylic acid (FFCA). On the other hand, the control experiments show that a certain amount of H2O2 was detected when HMF solution was replaced by ultrapure water, indicating that it is not only the HMF in the substrate that provides H+ in this reaction system (Fig. S6†). These results indicate that: (1) production of DFF and H2O2 are indeed triggered by visible-light-driven photocatalysis; (2) the photocatalytic activities of CN for DFF and H2O2 production can be enhanced simultaneously by defect modification of CN. Therefore, it can be concluded that an efficient bifunctional photocatalytic reaction system for the selective oxidation of biomass-associated alcohols into high value-added fine chemicals while producing clean energy (H2O2) can be realized under mild conditions over the synthesized noble metal-free Cv-CN photocatalysts.45
One of the important criteria to judge the catalytic performance of the catalyst is the stability of the catalyst and its reusability. The catalyst recovered by centrifugation after the reaction was completed was subjected to reproducibility experiments to study the stability of the photocatalyst; as shown in Fig. 7D, the amount of H2O2 produced by Cv-CN in five reproducibility experiments was similar, indicating that the Cv-CN photocatalyst can be reused. As shown in Fig. S7,† the XRD characterization tests before and after the use of Cv-CN photocatalyst showed that the diffraction peak positions did not change before and after the use of the catalyst. This test result indicates that the structure and material composition of the catalyst did not change before and after the catalytic reaction, which indicates that the Cv-CN photocatalyst is stable.
3.3 The electronic properties and reaction mechanism
3.3.1 The electronic properties.
In order to investigate the reason for the significant enhancement of the photocatalytic performance of Cv-CN, and also to investigate the possible electron transfer effect and the transfer path of photogenerated electrons in the defect sites, a theoretical calculation of the differential charge density of O2 adsorbed by the three catalysts was carried out using DFT simulations based on the construction of the optimal structural models of the three catalysts optimized. Fig. S8A, B and C† shows the differential charge images of O2 adsorption by CN, Nv-CN and Cv-CN, where blue represents electron consumption and yellow represents electron accumulation. A large number of electrons generated on the intact CN surface are quickly consumed, which is related to the rapid recombination of electrons and holes, and only a few electrons are involved in the subsequent reaction of O2 molecules, while the largest spacing between O2 and CN monolayers can be seen in Fig. S8A,† indicating the relatively weak adsorption of CN to O2. After the appearance of defect sites, the charge density difference on the CN surface is redistributed. Compared with CN, the charge density distribution of Cv-CN has changed significantly, and a large number of electrons are accumulated at the position where the C defect site appears, which can effectively prevent the complexation of electrons and holes, as shown in Fig. S8C.† A large amount of accumulated electrons is consumed by O2 adsorbed at the C-deficient site and converted to H2O2. The construction of the theoretical model shows that the C-deficient site can be used as an electron aggregation site, which effectively enhances the catalytic performance of CN. The electrons on the tri-s-triazine ring of CN are uniformly delocalized, whereas the charges on Cv-CN are redistributed owing to the asymmetrical structure. The C vacancies lead to a more localized electron density distribution, which may be the primary reason for the efficient photo-induced carrier separation of the Cv-CN.
To illustrate the role of vacancies in the charge separation, the LUMO and HOMO of CN and Cv-CN were determined. As shown in Fig. 8A, based on previous work, the electrons and holes of CN are located in the same tri-s-triazine rings, making them easily recombine.46 Green and yellow isosurfaces represent electron and hole distributions, respectively. Vacancies are introduced into CN to change the molecular orbitals and the distribution of orbital energy. Compared with CN, Cv-CN exhibits different electrons and holes distributions. As shown in Fig. 8A, the highest occupied molecular orbital (HOMO) of CN is chiefly located on two tri-s-triazine rings, while the lowest unoccupied molecular orbital (LUMO) is chiefly distributed in other tri-s-triazine ring. For Cv-CN sample, the HOMO is all localized at one tri-s-triazine ring (Fig. 8B), while the LUMO is located on N3C sites and two other tri-s-triazine rings of which one has a Cv. Thus, the electron–hole distributions are completely separated, leading to suppressed electron–hole recombination. Thus the different positions of LUMO and HOMO due to the presence of carbon vacancies facilitates the separation of space charges.47
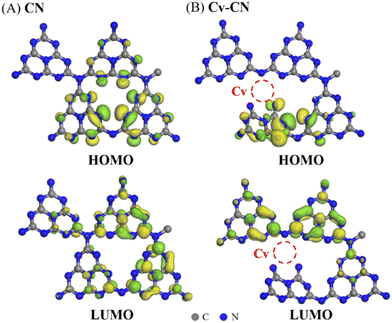 |
| Fig. 8 Distributions of HOMO and LUMO for (A) CN and (B) Cv-CN. | |
DFT calculations were also performed to further understand the reaction mechanism of the high activity H2O2 production over CN with vacancies. As shown in Fig. 9A, the corresponding free energy changes of each step in the photocatalytic H2O2 production process of CN and Cv-CN are given. The optimized surface intermediates were shown in Fig. 9B. The reaction process of photocatalytic H2O2 production mainly includes four steps. First, an O2 molecule is adsorbed on the active sites of the catalysts to form adsorbed *O2, and electrons are transferred from the CB of the catalysts to the adsorbed *O2 to reduce it to *OO. Subsequently, H+ combines with *OO to form *OOH, and *OOH is hydrogenated to form H2O2.28 Notably, the free energy change of CN for O2 adsorption is +1.27 eV, while Cv-CN is −1.89 eV, which indicates that the introduction of C vacancies can effectively enhance the adsorption of O2. Due to the existence of C vacancies, the reaction path of H2O2 formation on Cv-CN is energetically smoother than that of CN, which greatly promotes the formation of key intermediates and H2O2. Therefore, the DFT calculation results show that Cv-CN has stronger O2 adsorption capacity and photocatalytic H2O2 production activity.
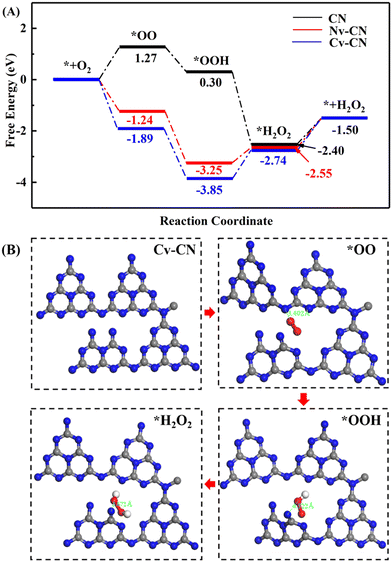 |
| Fig. 9 (A) Gibbs free energy diagrams for photocatalytic H2O2 production, and (B) corresponding optimized geometries. | |
3.3.2 The photo-reaction mechanism.
Based on the comprehensive analysis of the above experimental results and theoretical calculations, a possible mechanism for the photocatalytic selective conversion of HMF to DFF and simultaneous production of H2O2 by Cv-CN under visible light irradiation was proposed. As shown in Scheme 2, the catalyst Cv-CN was photoexcited by visible light, and photoexcited electrons (e−) were generated on the CB of Cv-CN, and an equal amount of photoexcited holes (h+) were generated on the VB of Cv-CN. The photoexcited electrons and holes are spatially separated, and h+ selectively oxidizes HMF to produce DFF and protons (H+), while e− is captured by C vacancies and immediately transferred to the adsorbed O2 molecules, and undergoes a reduction reaction with H+ in solution to generate H2O2. The excellent photocatalytic behavior of Cv-CN is the result of the participation of C vacancies. Firstly, the introduction of C vacancies can induce additional electronic states below the CB, thereby narrowing the band gap and optimizing light absorption. It is worth noting that the introduction of carbon vacancies can effectively adjust the band position of CN. Compared with CN (2.71 eV), Cv-CN has a narrower band gap (2.67 eV) and lower CB potential, indicating that Cv-CN has enhanced light trapping ability and better reduction ability to trigger photocatalytic reduction reactions. Secondly, C vacancies can act as electron traps to capture photogenerated electrons and facilitate the separation and transfer of photogenerated e− and h+ pairs. Thirdly, the C vacancies provide more surface active centers to enhance the adsorption of O2, and reduce the activation energy or overpotential of the photoreduction reaction. The presence of C vacancies promotes the generation–separation–transport of photogenerated carriers on CN and consumes photoexcited charges to produce high-value-added fine chemicals (DFF) and clean energy (H2O2). Thus, a dual-function photocatalytic reaction system with efficient and green recycling could be established under mild reaction conditions.
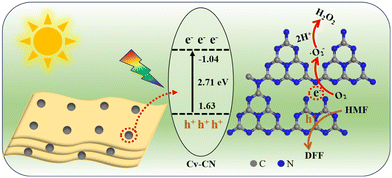 |
| Scheme 2 The photocatalytic mechanism. | |
4. Conclusion
In summary, work was carried out to obtain CN modified with carbon and nitrogen vacancies by simple calcination of CN under different atmospheres; the results can be used as an efficient, dual-functional and visible-light-driven photocatalysts toward selective conversion of biomass-relevant alcohols into high-value-added fine chemicals (aldehydes) and coupled to produce clean energy (H2O2) in one reaction system under mild reaction conditions. The Cv-CN could efficiently reduce O2 to H2O2 under visible light, coupling with selective oxidation of HMF to produce DFF. In terms of reducing O2 and oxidizing HMF, the visible light photocatalytic activity of Cv-CN is 1.7 times and 6.3 times that of CN. The results of UV-vis and XPS spectra show that the introduction of carbon vacancies reduces the band gaps from 2.71 eV to 2.67 eV, and Cv-CN has a more negative CB potential and a narrower band gap compared to CN, thus exhibiting enhanced electron reduction and light trapping capabilities. The free energy for O2 adsorption was +1.27 eV for CN and −1.89 eV for Cv-CN, indicating that the introduction of C vacancies gives Cv-CN a strong O2 adsorption capacity and photocatalytic activity for H2O2 production. The TRPL and EIS tests together with theoretical calculations demonstrate that Cv-CN can effectively promote the separation and transportation of photoexcited charges. This study not only provides a promising approach to design noble metal-free and efficient visible-light-driven composite photocatalysts, but also supplies a dual-functional, cooperative and sustainable way for utilization of photoexcited electrons and holes to synthesize fine chemicals (DFF) and H2O2 under mild reaction conditions.
Author contributions
Jingru Han: investigation, data curation, writing-original draft; Mengzhen Song: investigation, data curation; Yingjie Li: revisions; Yue Yao: investigation; Shuxiang Lu: project administration; Xiaoyuan Liao: investigation, revisions, project administration, funding acquisition.
Conflicts of interest
There are no conflicts to declare.
Acknowledgements
X. Liao would like to thank Dr. Yan-Yan Chen (Work in State Key Laboratory of Coal Conversion, Institute of Coal Chemistry, Chinese Academy of Sciences) for providing the DFT calculation software, and technical support.
References
- S. Li, G. Dong, R. Hailili, L. Yang, Y. Li, F. Wang, Y. Zeng and C. Wang, Effective photocatalytic H2O2 production under visible light irradiation at g-C3N4 modulated by carbon vacancies, Appl. Catal., B, 2016, 190, 26–35 CrossRef CAS.
- T.-P. Fellinger, F. Hasché, P. Strasser and M. Antonietti, Mesoporous Nitrogen-Doped Carbon for the Electrocatalytic Synthesis of Hydrogen Peroxide, J. Am. Chem. Soc., 2012, 134, 4072–4075 CrossRef CAS.
- L. Chen, Y. Wang, S. Cheng, X. Zhao, J. Zhang, Z. Ao, C. Zhao, B. Li, S. Wang, S. Wang and H. Sun, Nitrogen defects/boron dopants engineered tubular carbon nitride for efficient tetracycline hydrochloride photodegradation and hydrogen evolution, Appl. Catal., B, 2022, 303, 120932 CrossRef CAS.
- A. Kumar, P. Choudhary, T. Chhabra, H. Kaur, A. Kumar, M. Qamar and V. Krishnan, Frontier nanoarchitectonics of graphitic carbon nitride based plasmonic photocatalysts and photoelectrocatalysts for energy, environment and organic reactions, Mater. Chem. Front., 2023, 7, 1197–1247 RSC.
- P. Choudhary, A. Kumar and V. Krishnan, Nanoarchitectonics of phosphorylated graphitic carbon nitride for sustainable, selective and metal-free synthesis of primary amides, Chem. Eng. J., 2022, 431, 133695 CrossRef CAS.
- P. Choudhary, A. Bahuguna, A. Kumar, S. S. Dhankhar, C. M. Nagaraja and V. Krishnan, Oxidized graphitic carbon nitride as a sustainable metal-free catalyst for hydrogen transfer reactions under mild conditions, Green Chem., 2020, 22, 5084–5095 RSC.
- Y. Ding, Z. Lin, J. Deng, Y. Liu, L. Zhang, K. Wang, S. Xu and S. Cao, Construction of carbon dots modified hollow g-C3N4 spheres via in situ calcination of cyanamide and glucose for highly enhanced visible light photocatalytic hydrogen evolution, Int. J. Hydrogen Energy, 2022, 47, 1568–1578 CrossRef CAS.
- H. Dong, Y. Zuo, N. Song, S. Hong, M. Xiao, D. Zhu, J. Sun, G. Chen and C. Li, Bimetallic synergetic regulating effect on electronic structure in cobalt/vanadium co-doped carbon nitride for boosting photocatalytic performance, Appl. Catal., B, 2021, 287, 119954 CrossRef CAS.
- Y. Liao, G. Wang, J. Wang, K. Wang, S. Yan and Y. Su, Nitrogen vacancy induced in situ g-C3N4 p-n homojunction for boosting visible light-driven hydrogen evolution, J. Colloid Interface Sci., 2021, 587, 110–120 CrossRef CAS.
- Y. Wang, X. Wang and M. Antonietti, Polymeric Graphitic Carbon Nitride as a Heterogeneous Organocatalyst: From Photochemistry to Multipurpose Catalysis to Sustainable Chemistry, Angew. Chem., Int. Ed., 2012, 51, 68–89 CrossRef CAS PubMed.
- A. Kumar and V. Krishnan, Vacancy Engineering in Semiconductor Photocatalysts: Implications in Hydrogen Evolution and Nitrogen Fixation Applications, Adv. Funct. Mater., 2021, 31, 2009807 CrossRef CAS.
- X. Wang, Q. Li, L. Gan, X. Ji, F. Chen, X. Peng and R. Zhang, 3D macropore carbon-vacancy g-C3N4 constructed using polymethylmethacrylate spheres for enhanced photocatalytic H2 evolution and CO2 reduction, J. Energy Chem., 2021, 53, 139–146 CrossRef CAS.
- R. Wang, X. Wang, X. Li, L. Pei, X. Gu and Z. Zheng, Facile one-step synthesis of porous graphene-like g-C3N4 rich in nitrogen vacancies for enhanced H2 production from photocatalytic aqueous-phase reforming of methanol, Int. J. Hydrogen Energy, 2021, 46, 197–208 CrossRef CAS.
- Z. Zhao, Y. Long, Y. Chen, F. Zhang and J. Ma, Phosphorus doped carbon nitride with rich nitrogen vacancy to enhance the electrocatalytic activity for nitrogen reduction reaction, Chem. Eng. J., 2022, 430, 132682 CrossRef CAS.
- S. Cao, B. Fan, Y. Feng, H. Chen, F. Jiang and X. Wang, Sulfur-doped g-C3N4 nanosheets with carbon vacancies: General synthesis and improved activity for simulated solar-light photocatalytic nitrogen fixation, Chem. Eng. J., 2018, 353, 147–156 CrossRef CAS.
- H. Han, M. Zhang, Y. Zhu, K. Wu, Y. Liu, B. Wang, H. Lu and B. Liang, A green process for separation and recovery of 5-hydroxymethylfurfural from carboxylic acid–choline chloride deep eutectic solvents, New J. Chem., 2023, 47, 10593–10603 RSC.
- Z. Wei, E. Yao, Y. Cheng, J. Hu and Y. Liu, Insight into the dehydration of high-concentration fructose to 5-hydroxymethylfurfural in oxygen-containing polar aprotic solvents, New J. Chem., 2022, 46, 10470–10476 RSC.
- T. Chhabra, S. Dhingra, C. M. Nagaraja and V. Krishnan, Influence of Lewis and Brønsted acidic sites on graphitic carbon nitride catalyst for aqueous phase conversion of biomass derived monosaccharides to 5-hydroxymethylfurfural, Carbon, 2021, 183, 984–998 CrossRef CAS.
- T. Chhabra, A. Bahuguna, S. S. Dhankhar, C. M. Nagaraja and V. Krishnan, Sulfonated graphitic carbon nitride as a highly selective and efficient heterogeneous catalyst for the conversion of biomass-derived saccharides to 5-hydroxymethylfurfural in green solvents, Green Chem., 2019, 21, 6012–6026 RSC.
- I. Krivtsov, E. I. García-López, G. Marcì, L. Palmisano, Z. Amghouz, J. R. García, S. Ordóñez and E. Díaz, Selective photocatalytic oxidation of 5-hydroxymethyl-2-furfural to 2,5-furandicarboxyaldehyde in aqueous suspension of g-C3N4, Appl. Catal., B, 2017, 204, 430–439 CrossRef CAS.
- M. Ilkaeva, I. Krivtsov, E. I. García-López, G. Marcì, O. Khainakova, J. R. García, L. Palmisano, E. Díaz and S. Ordóñez, Selective photocatalytic oxidation of 5-hydroxymethylfurfural to 2,5-furandicarboxaldehyde by polymeric carbon nitride-hydrogen peroxide adduct, J. Catal., 2018, 359, 212–222 CrossRef CAS.
- W. Qi, J. Fang, Z. Yang, B. Li, R. Su and Z. He, A Low-Cost and Easily Prepared Manganese Carbonate as an Efficient Catalyst for Aerobic Oxidation of 5-Hydroxymethylfurfural to 2,5-Diformylfuran, Trans. Tianjin Univ., 2018, 24, 301–307 CrossRef CAS.
- S. Meng, C. Chen, X. Gu, H. Wu, Q. Meng, J. Zhang, S. Chen, X. Fu, D. Liu and W. Lei, Efficient photocatalytic H2 evolution, CO2 reduction and N2 fixation coupled with organic synthesis by cocatalyst and vacancies engineering, Appl. Catal., B, 2021, 285, 119789 CrossRef CAS.
- X. Bao, M. Liu, Z. Wang, D. Dai, P. Wang, H. Cheng, Y. Liu, Z. Zheng, Y. Dai and B. Huang, Photocatalytic Selective Oxidation of HMF Coupled with H2 Evolution on Flexible Ultrathin g-C3N4 Nanosheets with Enhanced N–H Interaction, ACS Catal., 2022, 12, 1919–1929 CrossRef CAS.
- W. Wei, Y. Wang, Y. Huang, Z. Xiao, Y. Cai, Q. Hao, H. Liu and X. Liao, Constructing isotype CN/s-CN heterojunction with enhanced photocatalytic performance, Diamond Relat. Mater., 2020, 101, 107616 CrossRef CAS.
- X. Shi, P. An, Q. Zhang, Q. Song, D. Jiang, D. Tian and D. Li, Synergy of nitrogen vacancies and Fe2P cocatalyst on graphitic carbon nitride for boosting photocatalytic CO2 conversion, Chem. Eng. J., 2022, 446, 137096 CrossRef CAS.
- S. Dhingra, T. Chhabra, V. Krishnan and C. M. Nagaraja, Visible-Light-Driven Selective Oxidation of Biomass-Derived HMF to DFF Coupled with H2 Generation by Noble Metal-Free Zn0.5Cd0.5S/MnO2 Heterostructures, ACS Appl. Energy Mater., 2020, 3, 7138–7148 CrossRef CAS.
- Y. Xue, C. Ma, Q. Yang, X. Wang, S. An, X. Zhang and J. Tian, Construction of g-C3N4 with three coordinated nitrogen (N3C) vacancies for excellent photocatalytic activities of N2 fixation and H2O2 production, Chem. Eng. J., 2023, 457, 141146 CrossRef CAS.
- X. Li, S. Li, J. Xu, L. Wang, K. Liang, H. Zhang, X. Yu and Z. Liu, Synergy of nitrogen vacancies and nanodiamond decoration in g-C3N4 for boosting CO2 photoreduction, Appl. Surf. Sci., 2022, 600, 154199 CrossRef CAS.
- J. Luo, Y. Liu, C. Fan, L. Tang, S. Yang, M. Liu, M. Wang, C. Feng, X. Ouyang, L. Wang, L. Xu, J. Wang and M. Yan, Direct Attack and Indirect Transfer Mechanisms Dominated by Reactive Oxygen Species for Photocatalytic H2O2 Production on g-C3N4 Possessing Nitrogen Vacancies, ACS Catal., 2021, 11, 11440–11450 CrossRef CAS.
- L. Acharya, B. P. Mishra, S. P. Pattnaik, R. Acharya and K. Parida, Incorporating nitrogen vacancies in exfoliated B-doped g-C3N4 towards improved photocatalytic ciprofloxacin degradation and hydrogen evolution, New J. Chem., 2022, 46, 3493–3503 RSC.
- Z. Yang, Y. Zhang, H. Zhang, J. Zhao, H. Shi, M. Zhang, H. Yang, Z. Zheng and P. Yang, Nitrogen vacancies in polymeric carbon nitrides promote CO2 photoreduction, J. Catal., 2022, 409, 12–23 CrossRef CAS.
- R. Li, M. Zheng, X. Zhou, D. Zhang, Y. Shi, C. Li and M. Yang, Carbon vacancies in porous g-C3N4 nanosheets induced robust H2O2 production for highly efficient photocatalysis-self-Fenton system for metronidazole degradation, Chem. Eng. J., 2023, 464, 142584 CrossRef CAS.
- H. Che, L. Liu, G. Che, H. Dong, C. Liu and C. Li, Control of energy band, layer structure and vacancy defect of graphitic carbon nitride by intercalated hydrogen bond effect of NO3− toward improving photocatalytic performance, Chem. Eng. J., 2019, 357, 209–219 CrossRef CAS.
- M. Guo, M. Chen, J. Xu, C. Wang and L. Wang, C, N-vacancies and Br dopant co-enhanced photocatalytic H2 evolution of g-C3N4 from water and simulated seawater splitting, Chem. Eng. J., 2023, 461, 142046 CrossRef CAS.
- P. Yang, L. Wang, H. Zhuzhang, R. Wang, M.-M. Titirici and X. Wang, Photocarving nitrogen vacancies in a polymeric carbon nitride for metal-free oxygen synthesis, Appl. Catal., B, 2019, 256, 117794 CrossRef CAS.
- Q. Yang, W. Yang, F. He, K. Liu, H. Cao and H. Yan, One-step synthesis of nitrogen-defective graphitic carbon nitride for improving photocatalytic hydrogen evolution, J. Hazard. Mater., 2021, 410, 124594 CrossRef CAS.
- Y. Li, J. Zhong and J. Li, Rich carbon vacancies facilitated solar light-driven photocatalytic hydrogen generation over g-C3N4 treated in H2 atmosphere, Int. J. Hydrogen Energy, 2022, 47, 39886–39897 CrossRef CAS.
- B. Yang, J. Han, Q. Zhang, G. Liao, W. Cheng, G. Ge, J. Liu, X. Yang, R. Wang and X. Jia, Carbon defective g-C3N4 thin-wall tubes for drastic improvement of photocatalytic H2 production, Carbon, 2023, 202, 348–357 CrossRef CAS.
- Q. Han, B. Wang, J. Gao, Z. Cheng, Y. Zhao, Z. Zhang and L. Qu, Atomically Thin Mesoporous Nanomesh of Graphitic C3N4 for High-Efficiency Photocatalytic Hydrogen Evolution, ACS Nano, 2016, 10, 2745–2751 CrossRef CAS.
- P. Qiu, Y. Yao, S. Lu, L. Chen, Y. Chen and X. Liao, Decorating Ti-MOF with noble metal as highly efficient photocatalysts: Fine-tuning the valence band to co-production of 2,5-diformylfuran and H2, Fuel, 2023, 351, 129043 CrossRef CAS.
- Y. Yang, G. Zeng, D. Huang, C. Zhang, D. He, C. Zhou, W. Wang, W. Xiong, X. Li, B. Li, W. Dong and Y. Zhou, Molecular engineering of polymeric carbon nitride for highly efficient photocatalytic oxytetracycline degradation and H2O2 production, Appl. Catal., B, 2020, 272, 118970 CrossRef CAS.
- G. Dong, D. L. Jacobs, L. Zang and C. Wang, Carbon vacancy regulated photoreduction of NO to N2 over ultrathin g-C3N4 nanosheets, Appl. Catal., B, 2017, 218, 515–524 CrossRef CAS.
- H. Zeng, L. Liu, D. Zhang, Y. Wang, Z. Li, C. Liu, L. Zhang and X. Cui, Fe(III)-C3N4 hybrids photocatalyst for efficient visible-light driven nitrogen fixation, Mater. Chem. Phys., 2021, 258, 123830 CrossRef CAS.
- S. Meng, H. Wu, Y. Cui, X. Zheng, H. Wang, S. Chen, Y. Wang and X. Fu, One-step synthesis of 2D/2D-3D NiS/Zn3In2S6 hierarchical structure toward solar-to-chemical energy transformation of biomass-relevant alcohols, Appl. Catal., B, 2020, 266, 118617 CrossRef CAS.
- M. Guo, M. Chen, J. Xu, J. Wang and L. Wang, Molecular engineering of g-C3N4 with spatial charge separation for enhancing photocatalytic performances, Mater. Chem. Front., 2022, 6, 1964–1972 RSC.
- B. Zhu, L. Zhang, B. Cheng and J. Yu, First-principle calculation study of tri-s-triazine-based g-C3N4: A review, Appl. Catal., B, 2018, 224, 983–999 CrossRef CAS.
|
This journal is © The Royal Society of Chemistry 2024 |
Click here to see how this site uses Cookies. View our privacy policy here.