DOI:
10.1039/D4RA05446F
(Paper)
RSC Adv., 2024,
14, 28234-28243
Transparent, mechanically robust, conductive, self-healable, and recyclable ionogels for flexible strain sensors and electroluminescent devices†
Received
27th July 2024
, Accepted 30th August 2024
First published on 4th September 2024
Abstract
A mechanically robust, self-healable, and recyclable PVP-based ionogel was achieved through a simple one-pot photoinitiated polymerization process. This ionogel exhibits a combination of excellent properties, including transparency, high mechanical strength, good ionic conductivity, self healability, and recyclability. A wearable resistive strain sensor based on the ionogel is successfully assembled and demonstrated accurate response to human motion. Moreover, a flexible electroluminescent device has been fabricated based on our ionogel, which can maintain optimal luminescence functionality even when subjected to bending. Considering the simple preparation method and excellent applications, we believe that our PVP-based ionogel has promising applications in many fields such as in wearable devices, electronic skin, implantable materials, robotics and human–machine interfaces.
1. Introduction
Ionogels have recently garnered significant attention due to their exceptional properties, including high stretchability, chemical stability, good conductivity, and optical transparency.1,2 These materials are widely utilized in strain sensors, flexible light-emitting devices, human–machine interfaces, and various other applications.3–5 However, due to relatively weak mechanical properties, the ionogel is susceptible to damage during its service life.3 Besides, the irreversible cross-linking structures within certain ionogels prevent network reconstruction once damaged, leading to environmental pollution and resource waste. Consequently, the development of self-healable and recyclable ionogels with high-strength and stretchability is crucial for advancing the field of flexible ionotronics.
To date, various self-healing ionogels have been developed by incorporating dynamic interactions into the polymer network, including hydrogen bonding, 6,7 π–π stacking,8 ion–dipole interactions, 9,10 and supramolecular interaction.11,12 For instance, our research group has previously published findings on the gelation of ionic liquids by means of locking them in a polymer network through ion–dipole interactions between fluorinated polymer networks and ionic liquids.10,13 Furthermore, acrylamide can achieve physical cross-linking of the polymer network through hydrogen bonding interactions in ionic liquids without chemical cross-linkers.13,14 Yan et al. and Wu et al. also demonstrated the potential of reversible dynamic interactions to impart self-healing capabilities to ionogels.11,15,16 However, the harsh environment of practical application scenarios places higher demands on the mechanical properties of these ionogels. Therefore, developing high-performance recyclable conductive ionogels with superior mechanical properties and excellent self-healing performance remains a significant challenge.
The incorporation of fillers,17,18 fibers,19,20 or additives21,22 has been demonstrated to effectively enhance the mechanical properties of gels or elastomers. For instance, Suo et al. achieved a synergistic improvement of rubber materials in terms of modulus and fatigue threshold by introducing dispersed silica nanoparticles into elastomers.22 Yu et al. reported an electrically conductive hydrogel composite with silver nanowires, in which the silver nanowires together with polyacrylamide form a composite network structure that exhibits the properties of both steel and concrete. This composite network structure imparts excellent toughness and fatigue resistance to the hydrogel.18 Xu et al. reported a tendon-mimicking hydrogel made of aramid nanofibers composited with poly vinyl alcohol, in which the hard nanofibers endowed the gel with excellent mechanical properties.23 Wu et al. obtained a fatigue-resistant self-repairing composite ionic skin by introducing nanofibers obtained by electrostatic spinning into an ionogel.19 However, these methods are often perceived as cumbersome and complex. In addition, the incorporation of some fillers can make it difficult or impossible to recycle the ionogel.
Studies have shown that the entanglement of ultra-high molecular weight polymers can effectively enhance the mechanical properties of gels or elastomers, thus offering unique advantages.24 For example, Suo et al. synthesized hydrogel polymer networks that were dominated by dense entanglements.25 The dense entanglements of the polymers confer high toughness, strength, and fatigue resistance upon the hydrogel. Polyvinylpyrrolidone (PVP) is a polymer compound formed by the polymerization of N-vinyl-2-pyrrolidone.26 It typically has a high molecular weight and good solubility, being soluble in water, ethanol, chloroform, and most organic solvents. Due to the presence of abundant lactam groups in the molecular chain, it can form hydrogen bonding interactions with other functional groups.27,28 Moreover, PVP is commercially available, which significantly reduces the difficulty and cost of preparing the materials.26 Therefore, adding ultra-high molecular weight PVP to the polymer matrix is expected to further enhance the mechanical properties of ionogels. Combining reversible hydrogen bonding interactions with the chain entanglement of ultra-high molecular weight polymers to construct a physically cross-linked polymer network holds great potential for designing polymer materials with high mechanical strength, recyclability, and excellent self-healing capabilities at room temperature.
Herein, we present a simple and controllable method for preparing conductive ionogels with excellent mechanical properties through reversible interactions and the entanglement of polymer chains, enabling self-healing of both mechanical and electrical properties. Introducing high molecular weight PVP into the polymer matrix enhances the mechanical strength of the ionogel. Due to the reversible recovery of interactions and molecular chain entanglement, the ionogel can achieve self-healing under room temperature and recyclability with the assistant of solvent. The conductivity and elasticity of the ionogel allow for its assembly as a flexible strain sensor, which has been demonstrated to exhibit accurate sensing performance. Besides, the transparency and ionic conductivity of the ionogel make it a promising candidate for use in flexible electroluminescent devices.
2. Results and discussion
2.1. Synthesis and characterization of the PVP based ionogel
The design principle is to combine a flexible, self-healing, and recyclable polymeric matrix with a hydrophobic and conductive IL. In view that polyacrylates are typically soft polymeric materials with good stretchability and transparency, we chose fluorine-containing 2,2,2-trifluoroethyl acrylate (TFEA), as well as acrylamide (AAm), which provides abundant hydrogen-bonding interactions, as the monomers to form the polymer matrix by copolymerization. A hydrophobic imidazolium containing IL, 1-ethyl-3-methylimidazolium bis(trifluoromethanesulfonyl)imide ([EMIM][TFSI]), was selected to be the conductive and transparent electrolyte salt.13,14 Moreover, for the further improvement of the mechanical properties of the ionogel, a commercially available polymer, PVP, was selected and blended into the ionogel network.26 This polymer has good solvent compatibility, ultra-high molecular weight, and abundant hydrogen bond interactions. The ionogels were prepared via a facile one-pot photo-initiated copolymerization of TFEA and AAm in the PVP solution in [EMIM][TFSI], without any chemical cross-linkers in custom-made silicone molds. The chemical structure and the reversible interactions of the ionogel is illustrated in Fig. 1a. The previous study of our group indicated that when the monomer ratio of AAm to TFEA is 50% and the ratio between polymer and ionic liquid is 40%, the resulting ionogel exhibits optimal properties. Consequently, in this work, the amount of TFEA, AAm, and ionic liquid is fixed to the optimal proportion, and the mechanical properties of the prepared ionogels are modified by adjusting the mass ratio of the added PVP. The ionogels were termed as ionogel-x, where x% is the PVP mass fraction to the ionogel. To investigate the effect of PVP polymer content on the mechanical and ionic conductivity properties of the ionogels, the PVP mass fraction was systematically changed from 2.5 to 15 wt% (Table S1†). The precipitation of the precursor solution at room temperature was observed to occur at an even higher PVP content. In this way, two dynamic interactions-ion–dipole interactions from the –CF3 groups on the polymeric matrix and hydrogen bond interactions from AAm and PVP-were introduced into the polymer networks, resulting in a dynamic physical crosslinked recyclable self-healing ionogel.29
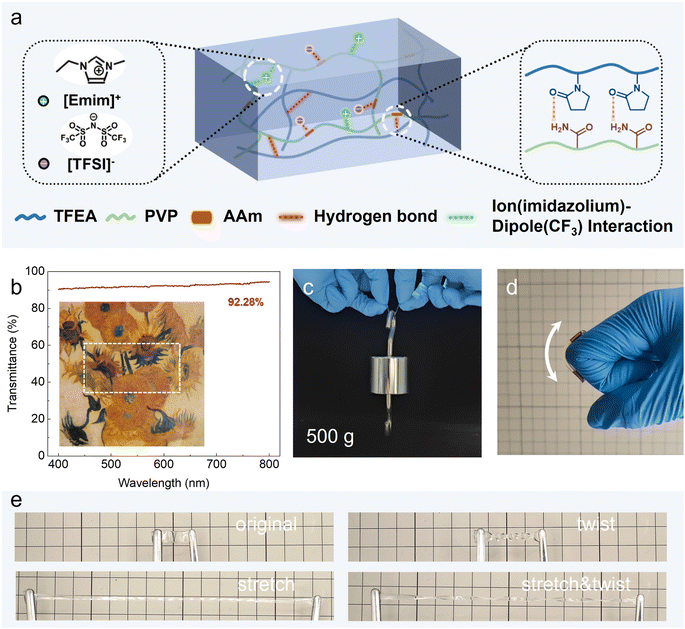 |
| Fig. 1 Schematic illustration for the design of the PVP based ionogel. (a) A flexible P(TFEA-co-AAm)-PVP copolymer network with polar –CF3, amide, and lactam groups interacted with a mobile IL [EMIM][TFSI] to form physically crosslinked ionogels. The ionogels can self-heal through highly reversible ion–dipole and hydrogen bonding interactions. (b) Transmittance spectrum of the ionogel with a film thickness of 1.8 mm. An average transmittance over 92% was recorded in the visible range (400–800 nm). (Inset) Photograph of the film over an image of flowers. (c) Photograph of an ionogel (20 mm × 5 mm × 1.8 mm) holding up a weight of 500 g. (d) Photographs of an ionogel under bending. (e) Photographs of an ionogel (20 mm × 8 mm × 1.8 mm) before and being stretched to 7 times its original length with and without twisting. The side length of the background grid is 10 mm. | |
Importantly, the high miscibility among PVP, P(TFEA-co-AAm) matrix, and [EMIM][TFSI] ensured good homogeneity of the ionogels and thus remarkable optical transparency, which was a critical parameter for optical ionotronic, such as electroluminescent devices.30 As shown in Fig. 1b, the as-prepared 1.8 mm-thick ionogel-7.5 displayed excellent transparency with an average transmittance of 92.28% in the visible light region (400–800 nm). The as prepared ionogel samples exhibited robust mechanical strength, demonstrating the ability to support a weight of 0.5 kg (Fig. 1c) and undergo deformation in response to finger bending (Fig. 1d). Furthermore, the excellent stretchability of the ionogel is evidenced by the stretching and twisting experiments (Fig. 1e).
The ionic liquid [EMIM][TFSI] could interact with the –CF3 groups on the polymeric matrix through the well-documented ion–dipole interactions,9,31 which help enhance the compatibility of the IL with the polymer matrix and improve the stability of the ionogels. Meanwhile, the hydrogen bond interactions between PVP and P(TFEA-co-AAm) polymer matrix endow the ionogel with robust mechanical properties and self-healing properties.13,29 The successful synthesis of the physical cross-linked ionogel was confirmed using Fourier-transform infrared spectroscopy (FT-IR). As shown in Fig. 2a and S1,† the FT-IR spectrum of the PVP based cross-linked ionogel exhibits the emergence of distinctive absorption bands at 1754 cm−1 and 1151 cm−1, which can be attributed to the C
O and CF3 groups of the polymer.13,31 The synergistic effect of ion–dipole interactions and hydrogen bond interactions endowed the ionogel with good mechanical properties, recyclability, and autonomous self-healing ability.
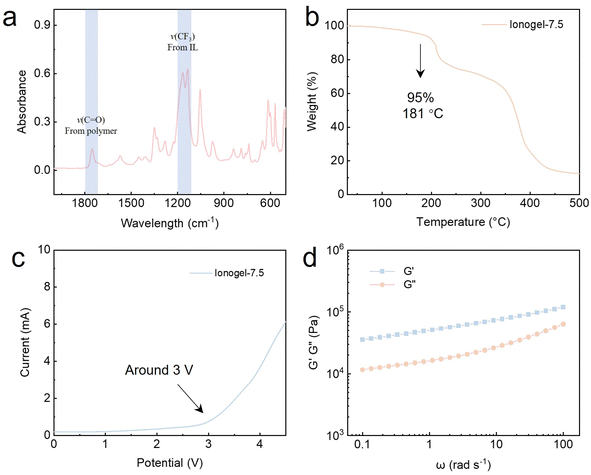 |
| Fig. 2 Physical properties of the PVP based ionogels. (a) ATR-FTIR spectra of the PVP based ionogel-7.5. (b) TGA curves of the ionogel showing high thermal stability with a decomposition temperature (at 5% weight loss) of 181 °C. (c) Linear sweep voltammetry curve of ionogel-7.5 showing the decomposition voltage of around 3 V. (d) Storage modulus G′ and loss modulus G′′ of ionogel-7.5. | |
Thermogravimetric analysis (TGA) was carried out to evaluate the thermostability of the ionogels. As shown in Fig. 2b, the ionogel-7.5 exhibited two steps of weight loss in the temperature range of 250 °C to 500 °C, which corresponds to the decomposition of the polymer matrix and ionic liquid under high temperatures. Taking a weight loss of 5% as the thermal decomposition temperature, through calculations, the thermal decomposition temperature of ionogel-7.5 is determined to be 181 °C. This can be attributed to the high thermostability of the [EMIM][TFSI] ionic liquid. Such a high thermal decomposition temperature indicates that the PVP based ionogel have good heat resistance and thermal stability. The decomposition voltage of ionogel-7.5 obtained from the linear sweep voltammetry curve exceeded 3 V (Fig. 2c), demonstrating a wide electrochemical window and high electric stability of the PVP based ionogels.
Rheology tests were performed to evaluate the viscoelastic properties of the ionogel-7.5. It can be seen from Fig. 2d that within the frequency range of 0.1–100 rad s−1, as the frequency increases, both the storage modulus (G′) and loss modulus (G′′) show an increase. Furthermore, the value of the storage modulus of all samples is always larger than the loss modulus, suggesting that the elastic property of the ionogel is predominant over the whole frequency range and that the material primarily undergoes elastic deformation during stretching.
2.2. Mechanical properties
By tuning the mass fraction of the PVP (2.5–15 wt%), a series of ionogels with different mechanical properties were obtained. As shown in Fig. 3a and Table S2,† all the tested ionogels exhibited good mechanical strength (tensile stress up to 2.04 MPa) and stretchability (breaking strain up to 1484%). With a PVP content increase from 2.5 wt% to 15 wt%, the tensile stress and elongation varied from 0.92 MPa and 1484% to 2.04 MPa and 891%, respectively. As expected, an increase in the PVP content would result in higher maximum tensile stress, higher Young's modulus, and lower stretchability, owing to the increased density of the polymer matrix and hydrogen bonding interactions. The toughness of ionogels in different proportions is determined by integrating the stress–strain curve of the corresponding ionogel. Among the samples, ionogel-7.5 showed the highest toughness of 6.80 MJ m−3 with a breaking stress of 1.74 MPa and a breaking strain of 1194%. The hydrogen bonding and ion–dipole interactions might contribute to the stability of the ionogels to avoid leaking during large strain. Interestingly, judging from the true stress–elongation curves (Fig. 3b) and the corresponding differential modulus–elongation curves (Fig. 3c), the ionogel-7.5 exhibited clear strain-stiffening behavior.32,33 It is noteworthy that the differential modulus–elongation curves of the ionogel show a unique sigmoid shape, which coincides exactly with the deformation response of natural.34,35 Specifically, the ionogel-7.5 sample showed a 32-fold modulus enhancement in the tensile test.
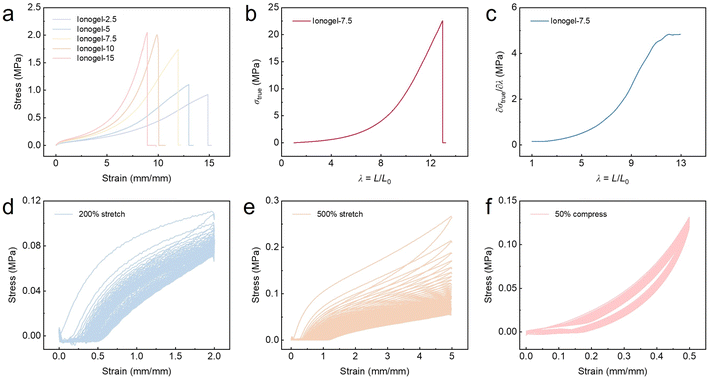 |
| Fig. 3 Mechanical properties of the PVP based ionogels. (a) Stress–strain curves of the ionogels prepared from varied mass ratios of PVP with 1250 kg mol−1. Corresponding true stress (b) and corresponding differential modulus curves (c) of the ionogel as a function of elongation. Cyclic stretch–release curves of the ionogels were conducted under (d) 200% and (e) 500% strain. (f) Cyclic compressive–recovery test of the ionogel with 50% strain. | |
To further investigate the viscoelastic property of the PVP based ionogel, cyclic tensile tests were carried out, from which the residual strain and the hysteresis ratio could be obtained. Upon subjecting the material to successive cyclic tensile tests under a large strain of 500% or a small strain of 200%, it was observed that the tensile strength exhibited a gradual decline with the increasing number of cycles (Fig. 3d and e). This indicated that the noncovalent bonds in the network, which were only partially broken, were unable to recover immediately. As shown in Fig. 3d, hysteresis loops and residual strains were recorded in the loading–unloading curves under the predetermined strains. With an increase of cycle number, the hysteresis ratio and maximum strength of the loading–unloading cycles both decreased. The tensile stress of the second cycle was found to be lower than that of the first cycle, and a residual strain of 7% was observed following the initial stretch–release cycle. Following 50 cycles of the stretch–release method, the residual strain remained at approximately 20%, and the hysteresis of tensile strength was no longer discernible (Fig. S2†). The appearance of hysteresis loops in the loading–unloading cycle of the tensile tests was related to the partial breaking of the reversible noncovalent interactions in the ionogel, including the hydrogen bonding and the ion–dipole interactions in this case. This phenomenon of energy dissipation is a common occurrence in a multitude of gel systems that are characterized by non-covalent interactions.36–38
As the cyclic tensile strain is increased to 500%, the rate of decrease in the strength of the ionogel with the number of stretches accelerated significantly, reaching a point where only 21% of the original strength was maintained after 100 stretches (Fig. 3e and S3†). The application of minor strains results in the disruption of partial interactions, which subsequently leads to the dissipation of energy. As the strain increases, more interactions and chain entanglements are further disrupted, leading to an increase in energy dissipation. Consequently, the higher the strain, the greater the energy dissipation, which ultimately leads to the rupture of the crosslinked network.13 As a result, the ionogel is unable to immediately fully restore its initial shapes after large tensile strains. Nevertheless, ionogel-7.5 samples are capable of fully restoring their original length within a 3 minute period following a 5-fold extension of their original length (Fig. S4†). The hydrogen bonding motifs functioned as the “temporary crosslinking sites” when the ionogels were subjected to a tensile strain at a relatively high strain rate. While some hydrogen bonds were disrupted, the majority remained intact, thereby preventing the polymer chains from sliding freely. Consequently, the ionogels are capable of withstanding tensile strain to a certain extent and subsequently returning to their original dimensions.
Furthermore, ionogel-7.5 demonstrated noteworthy resilience and fatigue resistance in the consecutive compressive test. At a compressive strain of 50%, the stress–strain curves recorded in 100 cycles exhibited minimal variation (Fig. 3f and S5†). The good resilience and cycling stability of the ionogels can be attributed to the synergistic effect of multiple reversible interactions formed between the polymer matrix and ionic liquid.39–41
2.3. Self-healing and recycle properties
The reversibility of hydrogen bonding interactions and ion–dipole interactions endow the physically crosslinked ionogel with both mechanical and electrical self-healing capabilities. To assess the self-healing capacity, the ionogel-7.5 was bisected with a razor blade. The resulting two pieces were then gently pressed together and incubated in a thermostatic oven under a predetermined time (Fig. 4a and b). The ionogels self-healed for 48 hours at room temperature, demonstrating resilience to tensile and curving deformations and maintaining comprehensive wound healing (Fig. 4c and d). It is noteworthy that the ionogel demonstrated the capacity to lift a 0.1 kg weight after 48 hours of self-healing, as shown in the Fig. 4e. Following a designated period of self-healing, the efficiency of the ionogel was quantified through tensile testing, with the stress–strain curve of the healed sample compared to that of the original. As shown in Fig. 4f, the sample healed for 2 h could be stretched up to 500% before breaking. Upon extending the self-healing time to 48 hours, the ionogel can be stretched to 1040% with a breaking strength of 1.3 MPa and a self-healing efficiency of 70%. In this case, the self-healing capability of the ionogels was achieved through the re-entanglement of polymer chains (both PVP and P(TFEA-co-AAm)) and the reversible nature of reversible dynamic interactions, as illustrated in Fig. 1c. An increase in time enhances the entanglement of polymer chains and the recovery of dynamic interactions, thus facilitating the healing process.42 To investigate the effect of different cutting directions on the self-healing properties of the ionogels, self-healing experiments of PVP-based ionogels under different cutting directions were conducted. The results indicate that there is no discernible difference in the strength and elongation at break exhibited by the ionogels under different cutting directions after self-healing at the same room temperature for 24 hours (Fig. S7†).
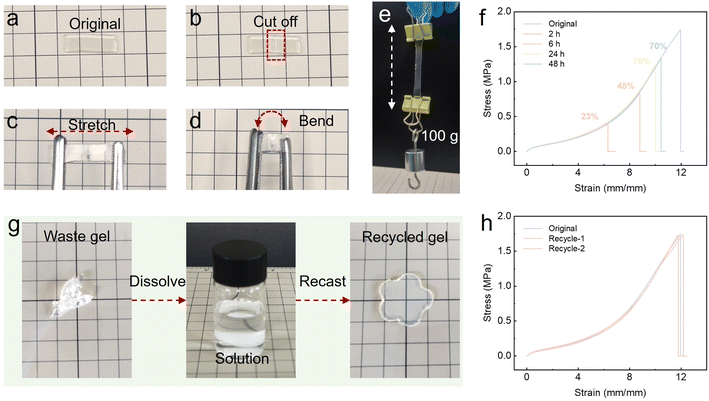 |
| Fig. 4 The self-healing properties and recyclability of the ionogels: photographs of (a) the original and (b) the healed samples. The self-healed samples can withstand (c) bending, (d) stretching, and (e) 100 g loading; (f) stress–strain curves of the ionogel-7.5 healed at room temperature for different times. (g) The ionogel is dissolved and recast in a good solvent to achieve recycling. (h) Representative stress–strain curves of original ionogel-7.5 (blue line) sample and samples after recycling once (red line) and twice (yellow line). | |
The incorporation of self-healing materials offers a range of benefits in electronic devices, conductors, and sensors. In this work, a self-healing ionogel circuit was fabricated using self-healing ionogel-7.5. To demonstrate the electrical healing performance of the ionogel, a rectangular ionogel sample was connected to a circuit comprising a light-emitting diode (LED) light and a battery. As shown in Fig. S6,† initially, the PVP based ionogel exhibited good conductivity, and the LED was turned on when a current was applied. Once the sample was cut off with a blade, the circuit was broken, and the LED was turned off. But, when the severed ionogels were brought into contact and allowed to heal for several minutes, the conductive pathway would be restored, and the LED was turned on again, demonstrating the electrical self-healing property of the ionogel.
The recycling of covalently cross-linked ionogel has generally been a challenging endeavor due to the necessity of destroying at least one rigid, irreversible covalent bond during the recycling process, which inevitably results in a loss of mechanical properties. The field of current research is confronted with a significant challenge in providing reliable mechanical properties through fully reversible non-covalent bond interactions, particularly in comparison to covalent bonds. However, through the use of reversible entanglement, hydrogen bonds, and ionic bonds between the linear segments, ionogels can be recycled and reused.15 To demonstrate the recyclability of the ionogel, a rectangular sample of the ionogel was cut into pieces and then dissolved in ethanol (Fig. 4g). After stirring, the solution becomes uniform and transparent, and is slowly injected into the mold to prevent the formation of bubbles. As the solvent slowly evaporates, the solution in the mold gradually transforms into an ionogel and exhibits mechanical strength similar to that of a pristine ionogel (Fig. 4h). The recyclability of this ionogel makes the process of using the material more environmentally friendly, minimizing waste and pollution.15,43 We believe that the application of this ionogel will contribute to the sustainable development of the world.
2.4. Electrical performance and strain sensor application
The ionic liquid infiltrates the ionogels and migrates within the polymer matrix, thereby endowing the ionogels with high ionic conductivity. Through EIS tests, the Nyquist plot of 4 ionogel-7.5 samples were shown in Fig. 5a, revealing good conductivity (∼1.02 mS cm−1 at 25 °C) reproducibility across batches of samples. The ionic conductivity was calculated by the equation of σ = L/RS, where L was the thickness of the sample, R was the bulk resistance obtained by the Nyquist plot of impedance spectra, and S was the cross-sectional area of the ionogel sample. For comparison, we provide a summary of the five key parameters of our PVP-based ionogel versus 6 recent representative recyclable ionogel materials (Table S3†).11,13,15,43–45 It is clearly evident that our PVP-based ionogel exhibits a combination of comprehensive superiorities. Benefiting from its high ionic conductivity, robust mechanical strength and stretchability, ionogel-7.5 has been found to be an ideal material for the manufacture of high performance resistive flexible strain sensors. As shown in Fig. 5b and Movie S1,† the relative real-time resistance change (ΔR/R0) of the sensor based on the ionogel was monitored at varying strains, spanning a range of 0 to 300%. The gauge factors (GF) within the strain ranges of 0–300% were calculated to be 0.58, indicating good sensitivity. Cyclic stretching and releasing of the sensor under low strains (0.1–5%) and high strains (10–100%) showed accurate and reversible resistance responses (Fig. 5c and d).
 |
| Fig. 5 Electrical and sensing properties of the PVP based ionogel: (a) Nyquist plots of the impedance spectra of the ionogels over different batches. (b) Relative resistance changes of the ionogel-7.5-based strain sensor as a function of tensile strain. Real-time monitoring of relative resistance under different strains ranging from (c) 0.1% to 5% and (d) 10% to 100%. Signals of relative electrical resistance during (e) finger bending and (f) elbow bending. | |
To demonstrate the reliability and durability of the flexible strain sensors under prolonged and multiple strain responses, long cycling experiments were conducted. The experimental results demonstrate that the flexible sensor assembled based on our ionogel is capable of undergoing at least 600 stretch–release cycles under a 20% strain, during which no significant drift was observed (Fig. S8†). Moreover, a comparison of the response curves for the initial and last 8 cycles demonstrated that the sensor exhibited consistent signal accuracy over time (Fig. S9†). This outcome substantiates the durability of the stretchable strain sensor, indicating its potential for applications in wearable flexible electronics.
The ionogel-based sensor was able to detect different kinds of human movement information simply by being applied to the joint with a VHB. As shown in Fig. 5e, the relative resistance change signal exhibited a discernible periodic variation during the process of bending the finger. As the finger bending angle increase from 45° to 90°, the resistive-strain response signal exhibited a notable enhancement. Moreover, the monitoring capacity of the ionogel sensor for elbow activity was investigated. As shown in Fig. 5f, the sensor exhibited consistent and distinctive electrical signals when the elbow underwent fast and slow repeated bending. These results indicated the potential applications of our PVP-based ionogels in the fabrication of wearable sensors.
2.5. Demonstration of flexible electroluminescent device
Transparent and highly flexible electrodes play a key role in developing flexible light-emitting devices.8,46 In this configuration, the light-emitting layer (dielectric layer) is sandwiched between two transparent conductive electrodes.46,47 When the outer conductive electrodes are subjected to an alternating current (AC) electric field, the insulating electroluminescent layer situated in the center emits bright fluorescence, which is driven by the aforementioned AC electric field. To illustrate the potential applications, we prepared a highly flexible electroluminescent device composed of two wires, an ionogel-7.5 transparent flexible electrode, an ITO flexible electrode, and a ZnS emission layer. The wire from the power supply was connected to the ionogel electrode and the ITO electrode, respectively (Fig. 6a). The physical optical photograph of the light-emitting device consisting of ionogel, light-emitting layer and ITO flexible electrode is shown in Fig. 6b. The conductive ionogel is connected to the power supply through a thin copper wire, while the ITO electrode is also linked to the power supply via a wire.
 |
| Fig. 6 Demonstration of flexible electroluminescent device based on the PVP based ionogel: (a) schematic illustration of the flexible electroluminescent device composed of two wires, a ZnS emission layer, a flexible ITO electrode, and a PVP-based ionogel as electrode. Photographs of the flexible electroluminescent device in different scenarios, such as (b) power off, (c) power on and (d) bending. | |
When the ionogel electrodes and ITO electrodes were subjected to a voltage of 110 volts at a frequency of 1400 Hz through the wires, the luminescent layer in the center emitted a bright blue light (Fig. 6c). According to the principle of series capacitor operation, most of the applied voltage in the alternating-current electroluminescent device is distributed to the dielectric emission layer.8 This phenomenon can be attributed to the conductive nature of the PVP-based ionogel electrode within the circuit, which exhibits a relatively low resistance, in contrast to the insulating properties of the dielectric emission layer situated at the center, which displays a resistance value approaching infinity (Fig. S10 and S11†). As a result, the typical voltage distributed to the PVP-based ionogel layer is at the 10−2 V scale, which is considerably smaller than its electrochemical window of approximately 3 V (Fig. 2c). It is therefore surmised that the PVP-based ionogel electrode is stable at the voltage employed in this experiment. The exceptional flexibility of our ionogel, coupled with the flexible ITO electrodes and light-emitting layer, enables the assembled electroluminescent device to retain its light-emitting functionality even when subjected to bending deformation (Fig. 6d). Moreover, it is reasonable to hypothesize that replacing the light-emitting layer and ITO electrodes with stretchable materials will result in an electroluminescent device with stretchable properties. Therefore, the stretchability and transparent feature offer great opportunities for our ionogels as the electrodes in developing flexible or even stretchable luminescent devices.
3. Conclusions
In conclusion, a self-healable and recyclable PVP-based ionogel was successfully developed through a simple one-pot photoinitiated polymerization process. The resulting ionogels exhibited a series of intriguing properties, including transparency, robust mechanical strength, and excellent ionic conductivity, which were integrated into a single material. Wearable resistive sensor based on the ionogels was successfully assembled and demonstrated an accurate response to human body motion. Moreover, a flexible light-emitting device was successfully fabricated and demonstrated the ability to maintain electroluminescence even when subjected to bending deformation. Due to their easy preparation, self-healing and recyclability, it is anticipated that PVP-based ionogels, with their remarkable and multifunctional properties, will find applications in wearable devices, electronic skin, implantable materials, robotics and human–machine interfacing, while being environmentally friendly.
4. Experimental section
A detailed Experimental section can be found in the ESI.† All human skin-attachment experiments were conducted by Z. H., and the authors did not seek or receive identifiable private information. The hands and arms shown in the figures are those of Z. H., who gave consent for these images.
Author contributions
Conceptualization, Z. H. and J. P.; writing–original draft preparation, Z. H.; writing–review and editing, Z. H. and J. P.; supervision, J. P.; acquisition of raw data, T. L., J. X. and P. L.; formal analysis, L. X.; funding acquisition, Z. H. and J. P. All authors have read and agreed to the published version of the manuscript.
Data availability
The data supporting this work are available from the corresponding author upon reasonable request.
Conflicts of interest
The authors declare no conflict of interest.
Acknowledgements
This work was financially supported by the National Natural Science Foundation of China (22301037), Natural Science Foundation of Guangdong Province (No. 2022A1515110867), and Featured Innovation Projects of General Colleges and Universities in Guangdong Province (2022KTSCX361).
References
- M. Wang, P. Zhang, M. Shamsi, J. L. Thelen, W. Qian, V. K. Truong, J. Ma, J. Hu and M. D. Dickey, Tough and stretchable ionogels by in situ phase separation, Nat. Mater., 2022, 21(3), 359–365 CrossRef CAS PubMed.
- W. Zhao, Y. Zheng, M. Jiang, T. Sun, A. Huang, L. Wang, W. Jiang and Q. Zhang, Exceptional n-type thermoelectric ionogels enabled by metal coordination and ion-selective association, Sci. Adv., 2023, 9(43), eadk2098 CrossRef CAS PubMed.
- C.-C. Yan, W. Li, Z. Liu, S. Zheng, Y. Hu, Y. Zhou, J. Guo, X. Ou, Q. Li, J. Yu, L. Li, M. Yang, Q. Liu and F. Yan, Ionogels: Preparation, Properties and Applications, Adv. Funct. Mater., 2024, 34(17), 2314408 CrossRef CAS.
- X. Fan, S. Liu, Z. Jia, J. J. Koh, J. C. C. Yeo, C.-G. Wang, N. E. Surat'man, X. J. Loh, J. Le Bideau, C. He, Z. Li and T.-P. Loh, Ionogels: recent advances in design, material properties and emerging biomedical applications, Chem. Soc. Rev., 2023, 52(7), 2497–2527 RSC.
- Q. Li, F. Yan and J. Texter, Polymerized and Colloidal Ionic Liquids–Syntheses and Applications, Chem. Rev., 2024, 124(7), 3813–3931 CrossRef CAS PubMed.
- W. Li, X. Wang, Z. Liu, X. Zou, Z. Shen, D. Liu, L. Li, Y. Guo and F. Yan, Nanoconfined polymerization limits crack propagation in hysteresis-free gels, Nat. Mater., 2024, 23(1), 131–138 CrossRef CAS PubMed.
- M. Zhu, S. He, Y. Dai, J. Han, L. Gan, J. Liu and M. Long, Long-Lasting Sustainable Self-Healing Ion Gel with Triple-Network by Trigger-Free Dynamic Hydrogen Bonds and Ion Bonds, ACS Sustainable Chem. Eng., 2018, 6(12), 17087–17098 CrossRef CAS.
- C. Luo, Y. Chen, Z. Huang, M. Fu, W. Ou, T. Huang and K. Yue, A Fully Self-Healing and Highly Stretchable Liquid-Free Ionic Conductive Elastomer for Soft Ionotronics, Adv. Funct. Mater., 2023, 33(49), 2304486 CrossRef CAS.
- Y. Cao, Y. J. Tan, S. Li, W. W. Lee, H. Guo, Y. Cai, C. Wang and B. C. K. Tee, Self-healing electronic skins for aquatic environments, Nat. Electron., 2019, 2(2), 75–82 CrossRef.
- F. Hu, Z. Huang, C. Luo and K. Yue, High-sensitivity and ultralow-hysteresis fluorine-rich ionogel strain sensors for multi-environment contact and contactless sensing, Mater. Horiz., 2023, 10(12), 5907–5919 RSC.
- L. Li, W. Li, X. Wang, X. Zou, S. Zheng, Z. Liu, Q. Li, Q. Xia and F. Yan, Ultra-Tough and Recyclable Ionogels Constructed by Coordinated Supramolecular Solvents, Angew. Chem., Int. Ed., 2022, 61(50), e202212512 CrossRef CAS PubMed.
- W. Li, L. Li, Z. Liu, S. Zheng, Q. Li and F. Yan, Supramolecular Ionogels Tougher than Metals, Adv. Mater., 2023, 35(30), 2301383 CrossRef CAS PubMed.
- L. Xu, Z. Huang, Z. Deng, Z. Du, T. L. Sun, Z.-H. Guo and K. Yue, A Transparent, Highly Stretchable, Solvent-Resistant, Recyclable Multifunctional Ionogel with Underwater Self-Healing and Adhesion for Reliable Strain Sensors, Adv. Mater., 2021, 33(51), 2105306 CrossRef CAS PubMed.
- Z. Huang, Y. Chen, J. Peng, T. Huang, F. Hu, X. Liu, L. Xu and K. Yue, Highly stretchable ionotronic pressure sensors with broad response range enabled by microstructured ionogel electrodes, J. Mater. Chem. A, 2023, 11(13), 7201–7212 RSC.
- W. Li, L. Li, S. Zheng, Z. Liu, X. Zou, Z. Sun, J. Guo and F. Yan, Recyclable, Healable, and Tough Ionogels Insensitive to Crack Propagation, Adv. Mater., 2022, 34(28), 2203049 CrossRef CAS.
- Y. Shi, B. Wu, S. Sun and P. Wu, Peeling–Stiffening Self-Adhesive Ionogel with Superhigh Interfacial Toughness, Adv. Mater., 2024, 36(11), 2310576 CrossRef CAS PubMed.
- T. Aziz, F. Haq, A. Farid, M. Kiran, S. Faisal, A. Ullah, N. Ullah, A. Bokhari, M. Mubashir, L. F. Chuah and P. L. Show, Challenges associated with cellulose composite material: Facet engineering and prospective, Environ. Res., 2023, 223, 115429 CrossRef CAS PubMed.
- Y. Wang, H. Qin, Z. Li, J. Dai, H.-P. Cong and S.-H. Yu, Highly compressible and environmentally adaptive conductors with high-tortuosity interconnected cellular architecture, Nat. Synth., 2022, 1(12), 975–986 CrossRef.
- J. Wang, B. Wu, P. Wei, S. Sun and P. Wu, Fatigue-free artificial ionic skin toughened by self-healable elastic nanomesh, Nat. Commun., 2022, 13(1), 4411 CrossRef CAS PubMed.
- B. Xue, Z. Bashir, Y. Guo, W. Yu, W. Sun, Y. Li, Y. Zhang, M. Qin, W. Wang and Y. Cao, Strong, tough, rapid-recovery, and fatigue-resistant hydrogels made of picot peptide fibres, Nat. Commun., 2023, 14(1), 2583 CrossRef CAS.
- J. Wei, Y. Zheng and T. Chen, A fully hydrophobic ionogel enables highly efficient wearable underwater sensors and communicators, Mater. Horiz., 2021, 8(10), 2761–2770 RSC.
- J. Steck, J. Kim, Y. Kutsovsky and Z. Suo, Multiscale stress deconcentration amplifies fatigue resistance of rubber, Nature, 2023, 624(7991), 303–308 Search PubMed.
- M. Sun, H. Li, Y. Hou, N. Huang, X. Xia, H. Zhu, Q. Xu, Y. Lin and L. Xu, Multifunctional tendon-mimetic hydrogels, Sci. Adv., 2023, 9(7), eade6973 CrossRef CAS.
- G. Nian, J. Kim, X. Bao and Z. Suo, Making Highly Elastic and Tough Hydrogels from Doughs, Adv. Mater., 2022, 34(50), 2206577 CrossRef CAS.
- J. Kim, G. Zhang, M. Shi and Z. Suo, Fracture, fatigue, and friction of polymers in which entanglements greatly outnumber cross-links, Science, 2021, 374(6564), 212–216 CrossRef CAS.
- P. Franco and I. De Marco, The Use of Poly(N-vinyl pyrrolidone) in the Delivery of Drugs: A Review, Polymers, 2020, 12(5), 1114 CrossRef CAS PubMed.
- Z. Ajji, M. Maarouf, A. Khattab and H. Ghazal, Synthesis of pH-responsive hydrogel based on PVP grafted with crotonic acid for controlled drug delivery, Radiat. Phys. Chem., 2020, 170, 108612 CrossRef CAS.
- G. G. de Lima, D. W. F. de Lima, M. J. A. de Oliveira, A. B. Lugão, M. T. S. Alcântara, D. M. Devine and M. J. C. de Sá, Synthesis and in Vivo Behavior of PVP/CMC/Agar Hydrogel Membranes Impregnated with Silver Nanoparticles for Wound Healing Applications, ACS Appl. Bio Mater., 2018, 1(6), 1842–1852 CrossRef CAS PubMed.
- H. G. Nam, M. G. Nam, P. J. Yoo and J.-H. Kim, Hydrogen bonding-based strongly adhesive coacervate hydrogels synthesized using poly(N-vinylpyrrolidone) and tannic acid, Soft Matter, 2019, 15(4), 785–791 RSC.
- J. Wang, C. Yan, G. Cai, M. Cui, A. Lee-Sie Eh and P. See Lee, Extremely Stretchable Electroluminescent Devices with Ionic Conductors, Adv. Mater., 2016, 28(22), 4490–4496 CrossRef CAS.
- Y. Cao, T. G. Morrissey, E. Acome, S. I. Allec, B. M. Wong, C. Keplinger and C. Wang, A Transparent, Self-Healing, Highly Stretchable Ionic Conductor, Adv. Mater., 2017, 29(10), 1605099 CrossRef PubMed.
- Y. Ren, J. Guo, Z. Liu, Z. Sun, Y. Wu, L. Liu and F. Yan, Ionic liquid–based click-ionogels, Sci. Adv., 2019, 5(8), eaax0648 CrossRef CAS PubMed.
- B. Yiming, X. Guo, N. Ali, N. Zhang, X. Zhang, Z. Han, Y. Lu, Z. Wu, X. Fan, Z. Jia and S. Qu, Ambiently and Mechanically Stable Ionogels for Soft Ionotronics, Adv. Funct. Mater., 2021, 31(33), 2102773 CrossRef CAS.
- W. Zhang, B. Wu, S. Sun and P. Wu, Skin-like mechanoresponsive self-healing ionic elastomer from supramolecular zwitterionic network, Nat. Commun., 2021, 12(1), 4082 CrossRef CAS PubMed.
- Z. Huang, Z. Deng, X. Liu, T. Huang, Y. Hu, Y. Chen, Y. Liu, Z.-H. Guo and K. Yue, Highly stretchable, strain-stiffening, self-healing ionic conductors for wearable sensors, Chem. Eng. J., 2022, 449, 137633 CrossRef CAS.
- T. Li, Y. Wang, S. Li, X. Liu and J. Sun, Mechanically Robust, Elastic, and Healable Ionogels for Highly Sensitive Ultra-Durable Ionic Skins, Adv. Mater., 2020, 32(32), 2002706 CrossRef PubMed.
- Y. Wang, S. Sun and P. Wu, Adaptive Ionogel Paint from Room-Temperature Autonomous Polymerization of α-Thioctic Acid for Stretchable and Healable Electronics, Adv. Funct. Mater., 2021, 31(24), 2101494 Search PubMed.
- F. Luo, T. L. Sun, T. Nakajima, T. Kurokawa, Y. Zhao, K. Sato, A. B. Ihsan, X. Li, H. Guo and J. P. Gong, Oppositely Charged Polyelectrolytes Form Tough, Self-Healing, and Rebuildable Hydrogels, Adv. Mater., 2015, 27(17), 2722–2727 CrossRef CAS PubMed.
- C. He, Z. Zheng, D. Zhao, J. Liu, J. Ouyang and H. Wang, Tough and super-resilient hydrogels synthesized by using peroxidized polymer chains as polyfunctional initiating and cross-linking centers, Soft Matter, 2013, 9(10), 2837–2844 RSC.
- L. Si, X. Zheng, J. Nie, R. Yin, Y. Hua and X. Zhu, Silicone-based tough hydrogels with high resilience, fast self-recovery, and self-healing properties, Chem. Commun., 2016, 52(54), 8365–8368 RSC.
- M. Wu, J. Chen, Y. Ma, B. Yan, M. Pan, Q. Peng, W. Wang, L. Han, J. Liu and H. Zeng, Ultra elastic, stretchable, self-healing conductive hydrogels with tunable optical properties for highly sensitive soft electronic sensors, J. Mater. Chem. A, 2020, 8(46), 24718–24733 RSC.
- Y. Wang, X. Liu, S. Li, T. Li, Y. Song, Z. Li, W. Zhang and J. Sun, Transparent, Healable Elastomers with High Mechanical Strength and Elasticity Derived from Hydrogen-Bonded Polymer Complexes, ACS Appl. Mater. Interfaces, 2017, 9(34), 29120–29129 CrossRef CAS PubMed.
- Y. Zhao, F. Wang, J. Liu, D. Gan, B. Lei, J. Shao, W. Wang, Q. Wang and X. Dong, Underwater Self-Healing and Recyclable Ionogel Sensor for Physiological Signal Monitoring, ACS Appl. Mater. Interfaces, 2023, 15(23), 28664–28674 CrossRef CAS.
- Y. Cheng, H. Zhu, S. Li, M. Xu, T. Li, X. Yang and H. Song, Stretchable, Low-Hysteresis, and Recyclable Ionogel by Ionic Liquid Catalyst and Mixed Ionic Liquid-Induced Phase Separation, ACS Sustainable Chem. Eng., 2023, 11(41), 15031–15042 CrossRef CAS.
- S. Xiang, F. Zheng, S. Chen and Q. Lu, Self-Healable, Recyclable, and Ultrastrong Adhesive Ionogel for Multifunctional Strain Sensor, ACS Appl. Mater. Interfaces, 2021, 13(17), 20653–20661 CrossRef CAS PubMed.
- S. Chang, J. H. Koo, J. Yoo, M. S. Kim, M. K. Choi, D.-H. Kim and Y. M. Song, Flexible and Stretchable Light-Emitting Diodes and Photodetectors for Human-Centric Optoelectronics, Chem. Rev., 2024, 124(3), 768–859 CrossRef CAS PubMed.
- Z. Zhang, Y. Wang, S. Jia and C. Fan, Body-conformable light-emitting materials and devices, Nat. Photon., 2024, 18(2), 114–126 CrossRef.
|
This journal is © The Royal Society of Chemistry 2024 |
Click here to see how this site uses Cookies. View our privacy policy here.