DOI:
10.1039/D4RA04918G
(Paper)
RSC Adv., 2024,
14, 25844-25851
Synthesis of nicotinimidamides via a tandem CuAAC/ring-cleavage /cyclization/oxidation four-component reaction and their cytotoxicity†
Received
8th July 2024
, Accepted 12th August 2024
First published on 16th August 2024
Abstract
Nicotinamide and its derivatives, recognized as crucial drug intermediates, have been a focal point of extensive chemical modifications and rigorous pharmacological studies. Herein, a series of novel nicotinamide derivatives, nicotinimidamides, were synthesized via a tandem CuAAC/ring-cleavage/cyclization/oxidation four-component reaction procedure from O-acetyl oximes, terminal ynones, sulfonyl azides, and NH4OAc. This strategy is significantly more efficient than previously reported, and the cytotoxicity of the nicotinimidamides is also tested. This project not only exhibits a sustainable and eco-friendly domino methodology for the creation of nicotinimidamides but also presents a promising candidate for liver cancer treatment.
Introduction
Pyridines represent privileged motifs in both organometallic1 and medicinal chemistry2 with series of them having considerable therapeutic potential in particular. One subset of such compounds is the nicotinamides and these have been explored in clinical practice for anti-influenza virus and anti-tubercular activities (Fig. 1, I),3 as effective agents against bacterial wilt (Fig. 1, II),4 potential gyrase B inhibitors (Fig. 1, III),5 novel inhibitors of bacterial DNA gyrase (Fig. 1, IV),6 or treat pellagra and some neurodegenerative diseases.7 Therefore, the synthesis of novel pyridine derivatives has received intense attention and encouraged the development of more valuable synthetic strategies.
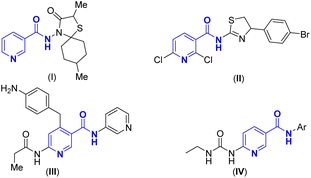 |
| Fig. 1 Examples of nicotinamides, I–IV, that have been explored as drug candidates. | |
Among the various synthetic approaches, multicomponent reactions (MCRs) stand out for their efficiency and simplicity,8 making them highly appealing to pharmaceutical companies that seek to expedite the discovery of new medicinal agents.9 To date, the predominant method for the synthesis of nicotinamide has been through the Hantzsch-type reaction. As illustrated in Scheme 1a, this process typically involves the three-component [3 + 3] aza-annulation reaction of chalcones, β-ketoamides, and ammonium acetate, facilitated by CAN as a Lewis acid,10 or alternatively, the reaction of phenylglyoxal, β-ketoamide, and 5-aminopyrazole through a Hantzsch-type 3-MCR.11 However, these methods often yield suboptimal results, especially when less reactive β-ketoamides are utilized as substrates, requiring high temperatures and pressures for the reaction to proceed. Consequently, there is a pressing need for the development of innovative and modular synthetic methods that can effectively construct novel nicotinamide derivatives, indicating an important frontier in the field of medicinal chemistry.
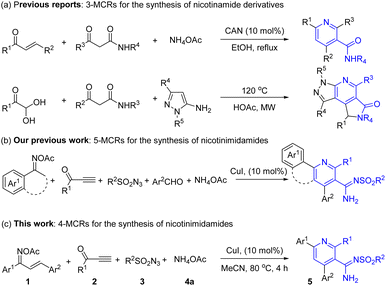 |
| Scheme 1 Synthesis of nicotinamide derivatives. | |
Previous studies have documented the efficacy of Cu-catalyzed multicomponent reactions (MCRs) involving sulfonyl azides and terminal alkynes, which integrate with other components to form N-heterocycles and analogous compounds through a CuAAC/ring-cleavage process. Celebrated for their gentle reaction conditions and the rich tapestry of chemical transformations they enable, these MCRs have become a staple in the synthesis of N-containing compounds, featuring prominently in 3-MCRs12 and, to a lesser extent, in 4-MCRs.13 Although our group has previously reported a 5-MCRs for novel nicotinamide derivative nicotinimidamides (Scheme 1b),14 it is characterized by low yields and the formation of numerous by-products. Moreover, no applications for these new products have been documented. Consequently, there is a clear necessity for the refinement and improvement of this method. Building on this foundation, we present a tandem CuAAC/ring-cleavage/cyclization/oxidation four-component reaction for the synthesis of nicotinimidamides as depicted in Scheme 1c. Our refined synthesis protocol entails the vigorous stirring of a mixture comprising O-acetyl oximes, terminal ynones, sulfonyl azides, and ammonium acetate (NH4OAc) in conjunction with a copper(I) catalyst. Moreover, we preliminarily assessed the antitumor cell activity and cytotoxicity of these nicotinimidamides, considering the impact of various functional groups.
Results and discussion
Our investigation commenced with an examination of the synthesis of nicotinimidamide 5a from O-acetyl oxime 1a, but-3-yn-2-one 2a, and tosyl azide 3a (Table 1). The initial reactions were conducted at 80 °C, catalyzed by CuI, utilizing NH4OAc as the amine source and MeCN as the solvent medium. After 4 h, and following chromatgraphic purification, target 5a was obtained in 88% yield (Table 1, entry 1). In efforts to improve this outcome, various other amine sources were screened and so revealing that only NH4OAc and NH4O2CH were effective (Table 1, entries 1–2), while (NH4)2SO4, NH4Cl, NH4PF6, (NH4)2CO3, PhNH2, and Et2NH ones were not effective (Table 1, entries 3–8). The ammonium salt (Table 1, entries 3–8) with acidic property seemed to stabilize the triazole by protonating its copper complex, preventing ring-cleavage in the CuAAC reaction, which can inhibit the desired reaction or even stop it.15 The organic base (Table 1, entries 7–8) with strong alkaline produce other side reactions that it cannot obtain the target product 5a. Then, informed by earlier studies,16 copper catalyst commonly used for CuAAC/ring-cleavage reactions, was also deployed in the present study. Among the copper catalysts used, CuI catalysts (Table 1, entries 9–10) exhibited higher catalytic reactivity than CuII catalysts (Table 1, entries 11–15). CuI catalyst generated product 5a in the highest yield of 88%, while Cu(OTf)2 (Table 1, entry 17) was the least efficient for this reaction. At last, comparable yields were obtained using DCE, DCM, toluene, THF, DMSO, DMF, dioxane or EtOH, as solvents but employing MeCN as the reaction medium gave target 5a in the highest yield (Table 1, entries 16–23).
Table 1 Optimization of catalytic conditionsa

|
Entry |
Amine (2 equiv.) |
Cat. (10 mol%) |
Solvent (3 mL) |
Yieldb (%) |
Reaction conditions: 1a (0.5 mmol), 3a (0.75 mmol), 4a (1.0 mmol), and the catalyst (10 mol%) in the solvent (3 mL) were added with 2a (0.75 mmol) and stirred at 80 °C for 4 h. Isolated yields. |
1 |
NH4OAc |
CuI |
MeCN |
88 |
2 |
NH4O2CH |
CuI |
MeCN |
42 |
3 |
(NH4)2SO4 |
CuI |
MeCN |
0 |
4 |
NH4Cl |
CuI |
MeCN |
0 |
5 |
NH4PF6 |
CuI |
MeCN |
0 |
6 |
(NH4)2CO3 |
CuI |
MeCN |
0 |
7 |
PhNH2 |
CuI |
MeCN |
0 |
8 |
Et2NH |
CuI |
MeCN |
0 |
9 |
NH4OAc |
CuCl |
MeCN |
84 |
10 |
NH4OAc |
CuBr |
MeCN |
82 |
11 |
NH4OAc |
CuBr2 |
MeCN |
74 |
12 |
NH4OAc |
CuCl2·2H2O |
MeCN |
60 |
13 |
NH4OAc |
Cu(OAc)2 |
MeCN |
72 |
14 |
NH4OAc |
Cu(acac)2 |
MeCN |
51 |
15 |
NH4OAc |
Cu(OTf)2 |
MeCN |
10 |
16 |
NH4OAc |
CuI |
DCE |
52 |
17 |
NH4OAc |
CuI |
DCM |
34 |
18 |
NH4OAc |
CuI |
Toluene |
67 |
19 |
NH4OAc |
CuI |
THF |
76 |
20 |
NH4OAc |
CuI |
DMSO |
56 |
21 |
NH4OAc |
CuI |
DMF |
12 |
22 |
NH4OAc |
CuI |
Dioxane |
40 |
23 |
NH4OAc |
CuI |
EtOH |
53 |
Having defined optimized conditions for the “parent” reaction (Table 1, entry 1), these were then applied to a range of different substrates. Gratifyingly, and as shown in Table 2, the anticipated products were formed when various aryl groups including 4-MeC6H4, 4-ClC6H4, 4-MeOC6H4, 4-BrC6H4, 4-CNC6H4, 4-NO2C6H4, or 2-furanyl were attached to the O-acetyl oximes 1 and so delivering compounds 5a–5i in yields ranging from 40% to 90%, and with 4-NO2C6H4 group resulting in poorer outcomes (see products 5h) presumably as a result of strong electron-withdrawing effect of nitro. When O-acetyl oximes 1 carried either two aromatic substituents including 4-MeC6H4 & 4-MeC6H4, 4-FC6H4 & 4-MeC6H4, 4-FC6H4 & 1-naphthyl, 4-ClC6H4 & 4-MeC6H4, 4-ClC6H4 & 1-naphthyl or 4-MeC6H4 & 4-BrC6H4, then the anticipated products (5j–5p) were all obtained in acceptable yields from 53% to 82%. However, when the alkyl groups instead of aryl groups were attached to the O-acetyl oximes 1 then the prospective products were not obtained.
Table 2 Substrate scope of O-acetyl oximes 1a
Reaction conditions: 1 (0.5 mmol), 3a (0.75 mmol), 4a (1.0 mmol), and the CuI (10 mol%) in the MeCN (3 mL) were added with 2a (0.75 mmol) and stirred at 80 °C for 4 h. |
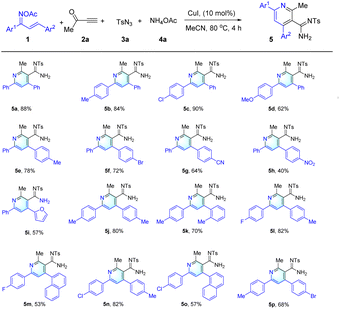 |
The versatility of the reaction was further explored by evaluating the impact of varying the nature of terminal ynones 2 and sulfonyl azides 3, as depicted in Table 3.
Table 3 Substrate scope of terminal ynones 2 and sulfonyl azides 3a
Reaction conditions: 1a (0.5 mmol), 3 (0.75 mmol), 4a (1.0 mmol), and the CuI (10 mol%) in the MeCN (3 mL) were added with 2 (0.75 mmol) and stirred at 80 °C for 4 h. |
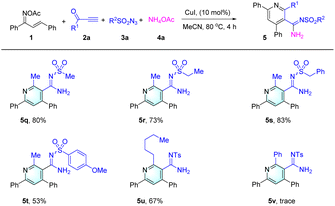 |
It was observed that alterations in the substituents on the sulfonyl azide moiety exerted minimal influence on the reaction's success, accommodating a diverse array of aryl and aliphatic groups. This led to the successful synthesis of products 5q to 5t, which bear –Me, –Et, benzyl, and –OMe groups, with yields ranging from 53% to 83%. In stark contrast, an examination of various terminal ynones 2 indicated that the functional groups attached to these substrates significantly dictate the reaction's outcome. For instance, alkyl groups such as –Me and –n−C5H11 were efficiently transformed into the desired pyridine derivatives 5a and 5u. However, terminal ynone substrates adorned with both aryl and ester groups, exemplified by 2c and 2d (as detailed in ESI Scheme S2†), did not yield the targeted products (e.g. 5v). The chemical structures of the synthesized compounds were meticulously elucidated using a suite of analytical techniques, including 1H NMR, 13C NMR, IR spectroscopy, and high-resolution mass spectrometry (HRMS).
To elucidate the potential mechanism behind the observed transformations, a comprehensive series of experiments was meticulously conducted. Through the synthesis of the CuAAC/ring-cleavage reaction intermediate compound 6, which was achieved by reacting 2a, 3a, and 4a (as depicted in Scheme 2a). As expected, the compound 6 can be transformed to the final product 5a under otherwise standard conditions (Scheme 2b). These experiments collectively suggest that compound 6 is a likely intermediate in the described chemical process.
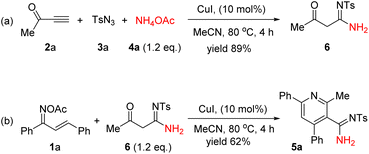 |
| Scheme 2 Investigation of the reaction mechanism. (a) Synthesis of intermediate 6. (b) Synthesis of product 5a from intermediate 6. | |
Consistent with prior hypotheses and the established reaction mechanism for oxime esters as detailed in references,17 the likely cascade annulation reaction mechanism is illustrated in Scheme 3. As per the literature,12 the interaction of substrates 2a and 3a in the presence of a copper(I) catalyst initiates the formation of the metallated triazole 7 via the CuAAC pathway. Subsequently, complex 7 experiences a ring-cleavage rearrangement, culminating in the generation of a highly reactive intermediate, the N-sulfonyl α-acylketenimine 8. This intermediate is swiftly captured by NH4OAc (4a) through a nucleophilic addition event, resulting in the formation of intermediate 6. Intermediate 6 then participates in a Michael addition with precursor 9 to form intermediate 10. An intramolecular nucleophilic attack subsequently forms the dihydropyridine intermediate 11. The synthesis is concluded with the oxidation of intermediate 11 by Cu(II) species, accompanied by the regeneration of the Cu(I) catalyst, thereby producing the desired product 5a. This domino sequence encompasses a CuAAC/ring-cleavage reaction, Michael addition, cyclization, and an oxidation step, showcasing the efficiency of this synthetic strategy.
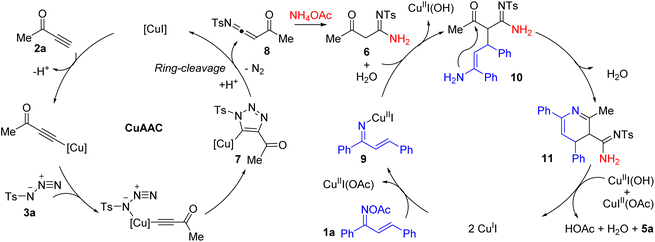 |
| Scheme 3 Plausible reaction mechanism. | |
An in-depth evaluation of the synthesized nicotinimidamide derivatives 5a–5u was conducted to determine their in vitro inhibitory effects on HepG2 cells, utilizing the MTT assay as a measure of cell viability. The outcomes, depicted as half-maximal inhibitory concentration (IC50) values in Table 4, represent the mean of no fewer than three separate experiments, ensuring the reliability of the results.
Table 4 The in vitro anti-proliferative activities (IC50, μM)a of compounds 5a–5u
Compounds |
HepG2 IC50(μM) |
Compounds |
HepG2 IC50(μM) |
MTT method. |
5a |
>100 |
5l |
56 |
5b |
>100 |
5m |
>100 |
5c |
73 |
5n |
46 |
5d |
>100 |
5o |
>100 |
5e |
>100 |
5p |
8.6 |
5f |
23 |
5q |
64 |
5g |
55 |
5r |
78 |
5h |
>100 |
5s |
>100 |
5i |
32 |
5t |
>100 |
5j |
>100 |
5u |
26 |
5k |
>100 |
Nicotinamide |
>100 |
As illustrated in Table 4, the majority of the nicotinimidamides demonstrated a notable anticancer potency, irrespective of their electron-donating or electron-withdrawing characteristics. Specifically, those bearing a –Me group (e.g., 5a, 5b, 5e, 5j, 5k), a –OMe group (e.g., 5d, 5t), or identified as insoluble products (e.g., 5h, 5m, 5o), displayed moderate anticancer activity, with IC50 values surpassing 100 μM. In contrast, the introduction of halogen groups (e.g., 5c, 5f, 5l, 5n, 5p), alkyl substituents (e.g., 5q, 5r, 5u), a –CN group (5g), or a furyl group (5i), engendered a pronounced apoptotic effect, with IC50 values within a range from 8.6 to 78.0 μM. Notably, the derivatives featuring a –Br group (e.g., 5p and 5f), showcased the most remarkable apoptotic activity, with IC50 values of 8.6 μM and 23 μM, respectively. This performance is significantly superior to that of nicotinamide, which showed an IC50 greater than 100 μM against HepG2 cells. This result is consistent with the literature reports that most halogens against antiproliferative activities.18 Furthermore, an assessment of the most active compounds—5p and 5f—on a healthy cell line (LO2 cells) revealed IC50 values above 100 μM, indicating their low toxicity towards non-cancerous cells.
Conclusions
A novel copper-catalyzed four-component domino reaction has been developed for the synthesis of nicotinimidamides. This innovative method utilizes a mixture of O-acetyl oximes, terminal ynones, sulfonyl azides, and NH4OAc, proceeding via a CuAAC/ring-cleavage mechanism. The process not only streamlines the synthesis of nicotinimidamides but also enhances the production efficiency. In addition to the synthetic advancements, an in-depth evaluation of all synthesized nicotinimidamides was conducted to assess their in vitro cytotoxic activity against a panel of human hepatoma (HepG2) cell lines. Among the derivatives, the one featuring a bromine substituent (5p) demonstrated exceptional potency, with an IC50 value of 8.6 μM. The findings of this study underscore the potential of nicotinimidamides as antineoplastic agents, highlighting their significance and warranting further investigation.
Experimental
General
All melting points were determined on a Yanaco melting point apparatus (Kyoto, Japan) and were uncorrected. IR spectra were recorded as KBr pellets on a Nicolet FT-IR 5DX spectrometer (Waltham, MA, USA). All spectra of 1H NMR (400 MHz) and 13C NMR (100 MHz) were recorded on a Bruker AVANCE NEO 400 MHz spectrometer (Berne, Switzerland) in DMSO-d6 or CDCl3 (unless otherwise indicated), with TMS used as an internal reference and the J values given in Hz. HRMS were obtained on a Thermo Scientific Q Exactive Focus Orbitrap LC-MS/MS spectrometer (Waltham, MA, USA). Optical rotations are measured on a P–2000, serial number: B209161232, JASCO corporation (Tokyo, Japan). O-acetyl oximes (1a–1p, see ESI Scheme S2†) were prepared by using reported methods.19 All terminal ynones (2a–2d, see ESI Scheme S2†) were prepared by the manufacturer and sulfonyl azides (3a–3e, see ESI Scheme S3†) were prepared using methods in the literature.20
General procedure for the synthesis of nicotinimidamides 5a–5u
We added O-acetyl oximes (1) (0.5 mmol), terminal ynones (2) (0.75 mmol), sulfonyl azides (3) (0.12 mmol), CuI (0.05 mmol), NH4OAc (4a, 77.1 mg, 1.0 mmol) and MeCN (3 mL) to an oven-dried Schlenk tube, equipped with a magnetic stirring bar. After the reaction was stirred at 80 °C for 4 h, and cooled to room temperature, the solvent was removed by evaporation in vacuum. The residue was directly purified by flash column chromatography (silica gel, using hexanes/EtOAc as eluent) to afford the corresponding products nicotinimidamides 5. Details of the compound characterizations are provided in the following subsections.
2-Methyl-4,6-diphenyl-N′-tosylnicotinimidamide (5a). Yield 88%, white solid, mp 226–228 °C (lit.14 230–231 °C). 1H NMR (400 MHz, DMSO-d6) δ 8.94 (s, 1H), 8.39 (s, 1H), 8.15 (d, J = 6.7 Hz, 2H), 7.74 (s, 1H), 7.55 (d, J = 8.0 Hz, 2H), 7.52–7.44 (m, 5H), 7.39 (t, J = 7.4 Hz, 1H), 7.31–7.27 (m, 4H), 2.47 (s, 3H), 2.40 (s, 3H); 13C NMR (100 MHz, DMSO-d6) δ 163.6, 155.6, 154.4, 147.7, 142.2, 137.9, 137.6, 129.4, 129.2 (3C), 128.7 (2C), 128.3 (2C), 128.2 (4C), 126.8 (2C), 126.2 (2C), 118.2, 22.2, 21.0.
2-Methyl-4-phenyl-6-(p-tolyl)-N′-tosylnicotinimidamide (5b). Yield 84%, white solid, mp 192–194 °C (lit.14 188–190 °C). 1H NMR (400 MHz, DMSO-d6) δ 8.98 (s, 1H), 8.44 (s, 1H), 8.06–8.04 (m, 2H), 7.68 (s, 1H), 7.54 (s, 2H), 7.43 (s, 2H), 7.30–7.29 (m, 5H), 7.06 (s, 2H), 2.48 (s, 3H), 2.40 (s, 3H), 2.36 (s, 3H); 13C NMR (100 MHz, DMSO-d6) δ 163.7, 163.5, 161.0, 155.7, 154.3, 146.8, 142.4, 139.2, 135.2, 134.0, 130.3, 130.2, 129.4 (2C), 129.3 (2C), 128.2, 126.8 (2C), 126.3 (2C), 117.9, 115.2, 115.0, 22.3, 21.0, 20.9.
6-(4-Chlorophenyl)-2-methyl-4-phenyl-N′-tosylnicotinimidamide (5c). Yield 90%, white solid, mp 233–234 °C (lit.14 229–231 °C). 1H NMR (400 MHz, DMSO-d6) δ 8.93 (s, 1H), 8.40 (s, 1H), 8.20 (d, J = 8.4 Hz, 2H), 7.78 (s, 1H), 7.56–7.54 (m, 4H), 7.47 (d, J = 7.4 Hz, 2H), 7.40–7.34 (m, 1H), 7.31–7.26 (m, 4H), 2.47 (s, 3H), 2.40 (s, 3H); 13C NMR (100 MHz, DMSO-d6) δ 163.6, 154.6, 154.4, 147.9, 142.4, 137.6, 136.8, 134.4, 129.4, 129.3 (2C), 128.9 (2C), 128.7 (3C), 128.5, 128 (3C), 126.3 (2C), 125.7, 118.4, 22.3, 21.1.
6-(4-Methoxyphenyl)-2-methyl-4-phenyl-N′-tosylnicotinimidamide (5d). Yield 62%, white solid, mp 224–226 °C (lit.14 226–228 °C). 1H NMR (400 MHz, DMSO-d6) δ 8.92 (s, 1H), 8.36 (s, 1H), 8.11 (d, J = 8.6 Hz, 2H), 7.66 (s, 1H), 7.54 (d, J = 7.8 Hz, 2H), 7.45 (d, J = 7.2 Hz, 2H), 7.38 (t, J = 7.2 Hz, 1H), 7.30 (d, J = 7.7 Hz, 4H), 7.03 (d, J = 8.6 Hz, 2H), 3.81 (s, 3H), 2.44 (s, 3H), 2.40 (s, 3H); 13C NMR (100 MHz, DMSO-d6) δ 164.0, 160.6, 155.5, 154.3, 147.7, 142.4, 137.9, 130.5, 129.3 (3C), 128.4 (3C), 128.3 (4C), 127.7, 126.3 (2C), 117.4, 114.2 (2C), 55.4, 22.4, 21.1.
2-Methyl-6-phenyl-4-(p-tolyl)-N′-tosylnicotinimidamide (5e). Yield 85%, white solid, mp 221–223 °C (lit.14 223–224 °C). 1H NMR (400 MHz, DMSO-d6) δ 8.94 (s, 1H), 8.38 (s, 1H), 8.13 (d, J = 6.7 Hz, 2H), 7.70 (s, 1H), 7.56 (d, J = 8.0 Hz, 2H), 7.51–7.43 (m, 3H), 7.34 (t, J = 8.4 Hz, 4H), 7.05 (t, J = 7.8 Hz, 2H), 2.46 (s, 3H), 2.41 (s, 3H), 2.33 (s, 3H); 13C NMR (100 MHz, DMSO-d6) δ 163.9, 155.7, 154.5, 147.8, 142.3, 138.1, 137.9, 134.8, 129.5, 129.3 (2C), 128.9 (3C), 128.8 (2C), 128.4, 128.1 (2C), 126.9 (2C), 126.4 (2C), 118.2, 22.3, 21.1, 21.0.
4-(4-Bromophenyl)-2-methyl-6-phenyl-N′-tosylnicotinimidamide (5f). Yield 72%, white solid, mp 237–239 °C (lit.14 241–243 °C). 1H NMR (400 MHz, DMSO-d6) δ 8.96 (s, 1H), 8.30 (s, 1H), 8.10 (d, J = 6.6 Hz, 2H), 7.66 (s, 1H), 7.55 (d, J = 8.5 Hz, 1H), 7.50–7.43 (m, 5H), 7.29–7.19 (m, 5H), 2.53 (s, 3H), 2.40 (s, 3H); 13C NMR (100 MHz, DMSO-d6) δ 163.0, 155.5, 155.0, 147.4, 142.6, 139.2, 138.2, 138.0, 133.0, 130.8, 130.5, 130.0, 129.6 (2C), 129.3 (3C), 127.3, 127.2 (2C), 126.4 (2C), 122.3, 119.3, 22.7, 21.5.
4-(4-Cyanophenyl)-2-methyl-6-phenyl-N′-tosylnicotinimidamide (5g). Yield 64%, white solid, mp 228–230 °C (lit.14 226–227 °C). 1H NMR (400 MHz, DMSO-d6) δ 9.12 (s, 1H), 8.52 (s, 1H), 8.14, (d, J = 7.0 Hz, 2H), 7.76 (s, 1H), 7.62 (s, 2H), 7.53–7.46 (m, 7H), 7.29, (d, J = 7.7 Hz, 2H), 2.55 (s, 3H), 2.44 (s, 3H). 13C NMR (100 MHz, DMSO-d6) δ 163.2, 156.1, 154.8, 146.5, 142.7, 142.3, 137.9, 132.1 (2C), 129.8, 129.4 (3C), 129.1, 129.0 (3C), 128.3, 127.1 (2C), 126.5 (2C), 118.8, 118.1, 111.2, 22.5, 21.2.
2-Methyl-4-(4-nitrophenyl)-6-phenyl-N′-tosylnicotinimidamide (5h). Yield 40%, white solid, mp 236–238 °C (lit.14 233–234 °C). 1H NMR (400 MHz, DMSO-d6) δ 9.14 (s, 1H), 8.54 (s, 1H), 8.16 (d, J = 6.9 Hz, 2H), 7.98 (d, J = 6.2 Hz, 2H), 7.80 (s, 1H), 7.58 (d, J = 6.6 Hz, 2H), 7.53–7.46 (m, 5H), 7.23 (d, J = 7.6 Hz, 2H), 2.58 (s, 3H), 2.36 (s, 3H); 13C NMR (100 MHz, DMSO-d6) δ 163.0, 156.0, 154.7, 147.1, 146.0, 144.2, 142.5, 137.7, 129.7, 129.5 (2C), 129.2 (3C), 128.9 (2C), 128.2, 127.0 (2C), 126.4 (2C), 123.2 (2C), 118.0, 22.4, 20.9.
4-(Furan-2-yl)-2-methyl-6-phenyl-N′-tosylnicotinimidamide (5i). Yield 57%, white solid, mp 138–140 °C (lit.14 135–137 °C). 1H NMR (400 MHz, DMSO-d6) δ 8.99 (s, 1H), 8.62 (s, 1H), 8.14 (d, J = 7.2 Hz, 2H), 8.04 (s, 1H), 7.71–7.64 (m, 3H), 7.54–7.46 (m, 3H), 7.32 (d, J = 7.0 Hz, 2H), 7.03 (s, 1H), 6.59–6.58 (m, 1H), 2.40 (s, 3H), 2.38 (s, 3H); 13C NMR (100 MHz, DMSO-d6) δ 155.9, 155.1, 148.9, 144.8, 142.4, 138.0, 135.2, 129.6, 129.3 (2C), 128.9 (3C), 126.8 (3C), 126.5 (2C), 112.8, 112.5, 112.0, 22.2, 21.1.
2-Methyl-4,6-di-p-tolyl-N′-tosylnicotinimidamide (5j). Yield 80%, white solid, mp 140–142 °C (lit.14 144–146 °C). 1H NMR (DMSO-d6, 400 MHz) δ 8.92 (s, 1H), 8.37 (s, 1H), 8.04 (d, J = 8.1 Hz, 2H), 7.66 (s, 1H), 7.56 (d, J = 7.8 Hz, 2H), 7.34–7.28 (m, 6H), 7.05 (d, J = 7.7 Hz, 2H), 2.45 (s, 3H), 2.41 (s, 3H), 2.36 (s, 3H), 2.33 (s, 3H); 13C NMR (DMSO-d6, 400 MHz) δ 164.0, 155.7, 154.3, 147.6, 142.3, 139.1, 137.8, 135.3, 134.8, 129.4 (2C), 129.2 (3C), 128.8 (3C), 128.1 (2C), 126.8 (2C), 126.4 (2C), 117.8, 22.3, 21.1, 20.9, 20.8.
2-Methyl-4-(o-tolyl)-6-(p-tolyl)-N′-tosylnicotinimidamide (5k). Yield 70%, white solid, mp 140–142 °C. IR (KBr) ν 3371.6, 3051.9, 1631.8, 1546.9, 1446.6, 1149.6, 1083.9 cm−1. 1H NMR (DMSO-d6, 400 MHz) δ 8.90 (s, 1H), 8.36 (s, 1H), 8.05 (d, J = 8.0 Hz, 2H), 7.68 (s, 1H), 7.53 (d, J = 8.0 Hz, 2H), 7.32–7.28 (m, 5H), 7.25 (d, J = 6.9 Hz, 1H), 7.20–7.14 (m, 2H), 2.45 (s, 3H), 2.39 (s, 3H),2.36 (s, 3H), 2.28 (s, 3H); 13C NMR (DMSO-d6, 400 MHz) δ 163.8, 155.6, 154.3, 147.8, 142.3, 139.1, 137.8, 137.5, 135.3, 129.4 (2C), 129.2 (3C), 129.0 (2C), 128.1, 126.8 (2C), 126.1 (3C), 125.3, 117.8, 22.3, 21.1. 21.0, 20.9. Calcd for C28H27N3O2S, [M + H]+ 470.1897; found 470.1899.
6-(4-Fluorophenyl)-2-methyl-4-(p-tolyl)-N′-tosylnicotinimidamide (5l). Yield 82%, white solid, mp 210–212 °C. IR (KBr) ν 3440.2, 3263.5, 1624.1, 1550.2, 1161.2, 1087.9 cm−1. 1H NMR (DMSO-d6, 400 MHz) δ 8.94 (s, 1H), 8.39 (s, 1H), 8.22–8.19 (m,2H), 7.72 (s, 1H), 7.57 (d, J = 7.7 Hz, 2H), 7.35–7.29 (m, 6H), 7.06 (t, J = 7.5 Hz, 2H), 2.46 (s, 3H), 2.41 (s, 3H),2.32 (s, 3H); 13C NMR (DMSO-d6, 400 MHz) δ 163.1 (d, J = 245.4 Hz), 163.8, 154.7, 154.5, 147.8, 142.3, 137.9, 134.7, 134.6 (d, J = 2.8 Hz), 129.3 (4C), 129.2 (d, J = 8.5 Hz), 128.9 (3C), 128.4, 128.2 (2C), 126.4 (2C), 118.1, 115.7 (d, J = 21.2 Hz), 25.4, 22.3, 20.8. Calcd for C27H24FN3O2S, [M + H]+ 474.1646; found 474.1650.
6-(4-Fluorophenyl)-2-methyl-4-(naphthalen-1-yl)-N′-tosylnicotinimidamide (5m). Yield 53%, white solid, mp 122–124 °C. IR (KBr) ν 3450.7, 3221.2, 1631.6, 1549.1, 1442.8, 1273.0, 1083.9 cm−1. 1H NMR (DMSO-d6, 400 MHz) δ 9.03 (s, 1H), 8.42 (s, 1H), 8.27–8.23 (m, 2H), 8.04 (s, 1H), 7.95 (d, J = 7.4 Hz, 1H), 7.88 (s, 1H), 7.79 (d, J = 7.9 Hz, 2H), 7.62–7.56 (m, 3H), 7.43 (d, J = 8.1 Hz, 2H), 7.33 (t, J = 8.8 Hz, 2H), 7.05 (d, J = 7.9 Hz, 2H), 2.52 (s, 3H), 2.30 (s, 3H); 13C NMR (DMSO-d6, 400 MHz) δ 164.2, 163.8, 162.0, 154.7, 154.6, 147.9, 142.2, 135.2, 134.5 (d, J = 2.9 Hz), 132.7, 132.6, 129.3, 129.2 (d, J = 8.4 Hz), 129.1 (2C), 128.6, 128.3 (2C), 127.7 (d, J = 31.2 Hz), 127.6, 126.8, 126.6, 126.1 (3C), 118.5, 115.7 (d, J = 214.7 Hz), 22.4, 21.1 (2C). Calcd for C30H24FN3O2S, [M + H]+ 510.1646; found 510.1641.
6-(4-Chlorophenyl)-2-methyl-4-(p-tolyl)-N′-tosylnicotinimidamide (5n). Yield 82%, white solid, mp 201–204 °C. IR (KBr) ν 3371.6, 3251.6, 1627.9, 1546.9, 1303.9, 1157.3, 1083.9 cm−1. 1H NMR (DMSO-d6, 400 MHz) δ 8.94 (s, 1H), 8.39 (s, 1H), 8.18 (d, J = 6.3 Hz, 2H), 7.74 (s, 1H), 7.55–7.53 (m, 4H) 7.32 (d, J = 8.9 Hz, 4H), 7.05 (d, J = 6.6 Hz, 2H), 2.46 (s, 3H), 2.41 (s, 3H), 2.32 (s, 3H); 13C NMR (DMSO-d6, 400 MHz) δ 164.2, 155.0, 154.8, 148.3, 142.7, 138.4, 137.3, 135.1, 134.7, 129.6 (3C), 129.2 (4C), 129.1 (3C), 128.5 (2C), 125.7 (2C), 118.7, 26.8, 22.7, 21.5. Calcd for C27H24ClN3O2S, [M + H]+ 490.1354; found 490.1358.
6-(4-Chlorophenyl)-2-methyl-4-(naphthalen-1-yl)-N′-tosylnicotinimidamide (5o). Yield 57%, white solid, mp 126–128 °C (lit.14 128–130 °C). 1H NMR (DMSO-d6, 400 MHz) δ 9.03 (s, 1H), 8.42 (s, 1H), 8.23 (d, J = 7.5 Hz, 2H), 8.04 (s, 1H), 7.94 (d, J = 7.6 Hz, 1H), 7.91 (s, 1H), 7.79 (d, J = 7.5 Hz, 2H), 7.62–7.55 (m, 5H), 7.43 (t, J = 7.2 Hz, 2H), 7.04 (d, J = 7.6 Hz, 2H), 2.52 (s, 3H), 2.30 (s, 3H); 13C NMR (DMSO-d6, 400 MHz) δ 163.7, 154.7, 154.4, 148.0, 124.2, 136.9, 135.2, 134.4, 132.7, 132.6, 129.1 (3C), 129.0 (2C), 128.8 (2C), 128.3, 127.8 (2C), 127.6, 126.8, 126.6, 126.1 (3C), 126.0, 118.6, 22.4, 22.1.
4-(4-Bromophenyl)-2-methyl-6-(p-tolyl)-N′-tosylnicotinimidamide (5p). Yield 68%, white solid, mp 164–166 °C (lit.14 162.3–165.2 °C). 1H NMR (DMSO-d6, 400 MHz) δ 8.98 (s, 1H), 8.42 (s, 1H), 8.05 (d, J = 8.2 Hz 2H), 7.69 (s, 1H), 7.55 (d, J = 8.0 Hz, 2H), 7.39 (d, J = 7.7 Hz, 2H), 7.31 (t, J = 7.3 Hz, 6H), 2.50 (s, 3H), 2.43 (s, 3H), 2.36 (s, 3H); 13C NMR (400 MHz, DMSO-d6) δ 163.7, 155.8, 154.5, 146.6, 142.4, 139.2, 136.9, 135.2, 131.1 (3C), 130.2, 129.5 (2C), 129.3 (3C), 127.9, 126.9 (2C), 126.4 (2C), 122.1, 117.7, 22.4, 21.2, 21.0.
2-Methyl-N′-(methylsulfonyl)-4,6-diphenylnicotinimidamide (5q). Yield 80%, white solid, mp 102–104 °C (lit.14 92 °C). 1H NMR (400 MHz, DMSO-d6) δ 8.88 (s, 1H), 8.24 (s, 1H), 8.15 (d, J = 7.7 Hz, 2H), 7.76 (s, 1H), 7.59 (d, J = 6.8 Hz, 2H), 7.52–7.44 (m, 6H), 2.62 (s, 6H); 13C NMR (100 MHz, DMSO-d6) δ 163.9, 155.9, 154.6, 148.1, 138.2, 138.1, 129.6, 129.0 (2C), 128.7, 128.6 (4C), 127.1 (3C), 118.3, 22.6 (2C).
N′-(Bthylsulfonyl)-2-methyl-4,6-diphenylnicotinimidamide (5r). Yield 73%, white solid, mp 105–107 °C (lit.14 98–100 °C). 1H NMR (400 MHz, DMSO-d6) δ 8.87 (s, 1H), 8.25 (s, 1H), 8.16 (d, J = 7.0 Hz, 2H), 7.76 (s, 1H), 7.61 (d, J = 7.7 Hz, 2H), 7.53–7.44 (m, 6H), 2.74–2.64 (m, 2H), 2.69 (s, 3H), 0.96 (t, J = 7.2 Hz, 3H); 13C NMR (100 MHz, DMSO-d6) δ 164.0, 155.7, 154.5, 147.9, 138.1, 129.5, 128.9 (2C), 128.7, 128.6, 128.5 (2C), 128.4 (3C), 127.0 (2C), 118.3, 47.4, 22.6, 7.8. This structure has been identified by X-ray structure (CCDC deposition number 2043697).14
N'-(Benzylsulfonyl)-2-methyl-4,6-diphenylnicotinimidamide (5s). Yield 83%, white solid, mp 150–152 °C (lit.14 148–150 °C). 1H NMR (400 MHz, DMSO-d6) δ 8.83 (s, 1H), 8.27 (s, 1H), 8.16 (d, J = 7.0 Hz, 2H), 7.77 (s, 1H), 7.58 (d, J = 8.8 Hz, 2H), 7.52–7.44 (m, 6H), 7.33–7.31 (m, 5H), 4.00 (s, 2H), 2.51 (s, 3H); 13C NMR (100 MHz, DMSO-d6) δ 164.2, 155.7, 154.6, 147.9, 138.1, 138.0, 131.1 (2C), 130.2, 129.5, 128.9 (2C), 128.6 (3C), 128.5 (3C), 128.2 (2C), 127.9, 127.0 (2C), 118.2, 58.6, 22.5.
N′-((4-Methoxyphenyl)sulfonyl)-2-methyl-4,6-diphenylnicotinimid amide (5t). Yield 53%, white solid, mp 164–166 °C (lit.14 160–162 °C). 1H NMR (400 MHz, DMSO-d6) δ 8.92 (s, 1H), 8.36 (s, 1H), 8.14 (d, J = 7.0 Hz, 2H), 7.73 (s, 1H), 7.61 (d, J = 8.0 Hz, 2H), 7.49 (d, J = 7.3 Hz, 5H), 7.38 (t, J = 7.0 Hz, 1H), 7.31 (d, J = 7.0 Hz, 2H), 7.00 (d, J = 8.5 Hz, 2H), 3.85 (3H), 2.46 (s, 3H); 13C NMR (100 MHz, DMSO-d6) δ 163.5, 162.1, 155.8, 154.6, 147.8, 138.1, 137.7, 129.5, 128.9 (2C), 128.5 (2C), 128.4 (2C), 128.3 (4C), 127.8, 127.0 (3C), 118.3, 114.1, 55.8, 22.4.
2-Pentyl-4,6-diphenyl-N′-tosylnicotinimidamide (5u). Yield 67%, yellow oil (lit.14 yellow oil). 1H NMR (400 MHz, DMSO-d6) δ 8.93 (s, 1H), 8.35 (s, 1H), 8.15 (d, J = 8.3 Hz, 2H), 7.72 (s, 1H), 7.62 (d, J = 7.8 Hz, 2H), 7.51–7.45 (m, 5H), 7.39 (d, J = 7.3 Hz, 1H), 7.34–7.32 (m, 4H), 2.61 (t, J = 8.0 Hz, 2H), 2.40 (s, 3H), 1.68 (t, J = 6.8 Hz, 2H), 1.30–1.23 (m, 3H), 0.87 (t, J = 7.0 Hz, 4H); 13C NMR (100 MHz, DMSO-d6) δ 163.6, 158.1, 155.7, 147.8, 142.4, 138.2, 137.8, 129.5, 129.3 (3C), 128.9 (2C), 128.5, 128.4 (2C), 128.3 (2C), 128.1, 126.9 (2C), 126.4 (2C), 118.2, 34.9, 31.4, 28.5, 22.1, 21.1, 14.0.
3-Oxo-N′-tosylbutanimidamide (6). To a solution of NH4OAc (0.77 g, 10 mmol), CuI (0.19 g, 1.0 mmol), TsN3 (3a, 2.37 g, 12 mmol) in MeCN (15 mL) was added. Then added the but-3-yn-2-one (2a, 0.82 g, 12 mmol) slowly within 30 min at 0 °C. After the reaction was stirred at 0 °C for 1 h, room temperature for 12 h, the mixture was evaporated in vacuum. The residue was purified by a flash chromatography [silica gel, 50% EtOAc in petroleum ether (60–90 °C)] to give 1.57 g (62%) of product 5 as a white solid, mp 130–134 °C (lit.14 128–129 °C). 1H NMR (400 MHz, DMSO-d6) δ 8.52 (s, 1H), 8.18 (s, 1H), 7.69 (d, J = 7.3 Hz, 2H), 7.33 (d, J = 7.3 Hz, 2H), 3.54 (s, 2H), 2.35 (s, 3H), 2.10 (s, 3H); 13C NMR (400 MHz, DMSO-d6) δ 201.4, 163.7, 142.1, 140.0, 129.2 (2C), 126.0 (2C), 49.8, 29.8, 21.0.All NMR spectra please see ESI Section 3.†
Biological assay
The HepG2 cells and LO2 cells were obtained from the American Type Culture Collection and cultured in an environment of 5% CO2 at 37 °C in RPMI-1640 medium supplemented with 10% fetal bovine serum (FBS). Human liver (HepG2) cancer cell lines were seeded in 96-well plates at a density of 3000 cells/well in normoxia for 12 h. Then, measures of 100 μL drug-containing medium, with a series of concentrations, were dispensed into the wells to attain the final concentration as 100, 80, 20, 10, 5, and 2 μM. After 48 h incubated under normoxia or hypoxia, 20 μL MTT solution (Beyotime Biotechnology, Nantong, China, 5 mg mL−1 MTT dissolved in PBS) was added. Then, following incubation for another 4 h, the medium was discarded, followed by the addition of 200 μL DMSO. The absorbance was measured at 570 nm with a microplate reader. Experiments were conducted in triplicate. The IC50 values are the average of at least three independent experiments.
Data availability
The data supporting this article have been included as part of the ESI.†
Author contributions
Xi Chen and Guanrong Li: main contributor in this manuscript who did experiment, data curation, formal analysis, investigation, and methodology. Zixin Huang: experiment, spectroscopic characterization. Qiaoli Luo: spectroscopic characterization. Tao Chen and Weiguang Yang: main contributor in this manuscript who did conceptualization, funding acquisition, project administration, resources, supervision, writing original draft, and review. All authors have read and agreed to the published version of the manuscript.
Conflicts of interest
There are no conflicts to declare.
Acknowledgements
This research was funded by the Scientific Research Project of General Universities in Guang-dong Province (2023KTSCX036).
Notes and references
-
(a) M. Schlosser and F. Mongin, Chem. Soc. Rev., 2007, 36, 1161–1172 RSC;
(b) M. R. Stentzel and D. A. Klumpp, J. Org. Chem., 2020, 85, 12740–12746 CrossRef CAS PubMed;
(c) D. C. Leary, Y. Zhang, J. G. Rodriguez, N. G. Akhmedov, J. L. Petersen, B. S. Dilinar and C. Milsmann, Organometallics, 2023, 42, 1220–1231 CrossRef CAS PubMed;
(d) G. R. Peddiahgari Vasu, K. R. Motakatla Venkata, R. R. Kakarla, K. V. S. Ranganath and T. M. Aminabhavi, Environ. Res., 2023, 225, 115515 CrossRef CAS PubMed.
-
(a) R. Roman, L. Pintilie, D. C. Nuţă, M. T. Căproiu, F. Dumitrascu, I. Zarafu, P. Ionită, I. C. Marinas, L. Mărutescu, E. Kapronczai, S. Ardelean and C. Limban, Pharmaceutics, 2023, 15, 2501 CrossRef CAS PubMed;
(b) J. A. Tali, G. Kumar, B. K. Sharma, Y. Rasool, Y. Sharma and R. Shankar, Org. Biomol. Chem., 2023, 21, 7267–7289 RSC;
(c) M. B. Islam, M. I. Islam, N. Nath, T. B. Emran, M. R. Rahman, R. Sharma and M. M. Matin, Biomed Res. Int., 2023, 2023, 9967591 CrossRef PubMed;
(d) Y. Wu, T. Wu and Y. Huang, Arch. Pharm., 2023, 356, e2300067 CrossRef PubMed.
- G. Cihan-Üstündağ, Ç. Acar, L. Naesens, G. Erköse-Genç and D. Şatana, Arch. Pharm., 2022, 355, e2200224 CrossRef PubMed.
- Y. H. I. Mohammed, I. M. Shamkh, A. H. Shntaif, M. Sufyan, M. T. Rehman, M. F. AlAjmi, M. Shahwan, S. Alghamdi, A. E. Abd El-Lateef, E. B. Khidir, A. S. Abouzied, N. E. Khalifa, W. M. A Khojali, B. Huwaimel, D. A. Al Farraj and S. M. Almutairi, Sci. Rep., 2024, 14, 11118 CrossRef CAS PubMed.
- M. A. Azam and J. Thathan, SAR QSAR Environ. Res., 2017, 28, 275–296 CrossRef CAS PubMed.
- I. A. Yule, L. G. Czaplewski, S. Pommier, D. T. Davies, S. K. Narramore and C. W. Fishwick, Eur. J. Med. Chem., 2014, 86, 31–38 CrossRef CAS PubMed.
-
(a) L. Fania, C. Mazzanti, E. Campione, E. Candi, D. Abeni and E. Dellambra, Int. J. Mol. Sci., 2019, 3, 5946 CrossRef PubMed;
(b) M. A. Chiacchio, D. Iannazzo, S. Romeo, S. V. Giofre and L. Legnani, Curr. Med. Chem., 2019, 26, 7166–7195 CrossRef CAS PubMed;
(c) P. W. Ondachi, A. H. Castro, J. M. Bartkowiak, C. W. Luetje, M. I. Damaj, S. W. Mascarella, H. A. Navarro and F. I. Carroll, J. Med. Chem., 2014, 57, 836–848 CrossRef CAS PubMed.
-
(a) C. Allais, J. M. Grassot, J. Rodriguez and T. Constantieux, Chem. Rev., 2014, 114, 10829–10868 CrossRef CAS PubMed;
(b) N. Deibl, K. Ament and R. Kempe, J. Am. Chem. Soc., 2015, 137, 12804–12807 CrossRef CAS PubMed;
(c) J. Zhu and H. Bienaymé, The Discovery of New Isocyanide-Based Multicomponent Reactions, Wiley-VCH, Weinheim, Germany, 2005 Search PubMed.
-
(a) D. Insuasty, J. Castillo, D. Becerra, H. Rojas and R. Abonia, Molecules, 2020, 25, 505 CrossRef PubMed;
(b) C. S. Graebin, F. V. Ribeiro, K. R. Rogerio and A. E. Kummerle, Curr. Org. Synth., 2019, 16, 855–899 CrossRef CAS PubMed;
(c) A. E. van der Westhuyzen, L. V. Frolova, A. Kornienko and W. A. L. van Otterlo, Alkaloids, 2018, 79, 191–220 CAS;
(d) J.-P. Wan, L. Gan and Y. Liu, Org. Biomol. Chem., 2017, 15, 9031–9043 RSC;
(e) S. Brauch, S. S. Berkel and B. van Westermann, Chem. Soc. Rev., 2013, 42, 4948–4962 RSC.
- G. Tenti, M. T. Ramos and J. C. Menéndez, ACS Comb. Sci., 2012, 14, 551–557 CrossRef CAS PubMed.
-
(a) X. Feng, J. Wang, D. Liu, H. Shi, W. Lu and D. Shi, J. Org. Chem., 2023, 88, 6682–6690 CrossRef CAS PubMed;
(b) H. Tan and Y. Wang, ACS Comb. Sci., 2020, 22, 468–474 CrossRef CAS PubMed.
-
(a) S. H. Kim, S. H. Park, J. H. Choi and S. Chang, Chem.–Asian J., 2011, 6, 2618–2634 CrossRef CAS PubMed;
(b) I. Bae, H. Han and S. Chang, J. Am. Chem. Soc., 2005, 127, 2038–2039 CrossRef CAS PubMed;
(c) S. H. Cho, E. J. Yoo, I. Bae and S. Chang, J. Am. Chem. Soc., 2005, 127, 16046–16047 CrossRef CAS PubMed;
(d) W. Yang, G. Li, D. Luo, Z. Huang, M. G. Banwell and X. Luo, Adv. Synth. Catal., 2024 DOI:10.1002/adsc.202400339;
(e) C.-G. Wang, R. Wu, T.-P. Li, T. Jia, Y. Li, D. Fang, X. Chen, Y. Gao, H.-L. Ni, P. Hu, B.-Q. Wang and P. Cao, Org. Lett., 2020, 22, 3234–3238 CrossRef CAS PubMed;
(f) G. Li, D. Luo, Q. Luo, Z. Huang, W. Zhuang, H. Luo and W. Yang, J. Org. Chem., 2024, 89, 2190–2199 CrossRef CAS PubMed;
(g) L. Xu, T. Zhou, M. Liao, R. Hu and B. Z. Tang, ACS Macro Lett., 2019, 8, 101–106 CrossRef CAS PubMed;
(h) S. Guo, P. Dong, Y. Chen, X. Feng and X. Liu, Chiral Guanidine/Copper Catalyzed Asymmetric Azide-Alkyne Cycloaddition/[2+2] Cascade Reaction, Angew. Chem., Int. Ed., 2018, 57, 16852–16856 CrossRef CAS PubMed.
-
(a) M. Nematpour, H. F. Dastjerdi, S. M. I. M. Rabbani and S. A. Tabatabai, J. Heterocycl. Chem., 2019, 56, 2604–2611 CrossRef CAS;
(b) D. Cheng, F. Ling, C. Zheng and C. Ma, Org. Lett., 2016, 18, 2435–2438 CrossRef CAS PubMed;
(c) G. Murugavel and T. Punniyamurthy, J. Org. Chem., 2015, 80, 6291–6299 CrossRef CAS PubMed;
(d) R. Husmann, Y. S. Na, C. Bolm and S. Chang, Chem. Commun., 2010, 46, 5494–5496 RSC;
(e) W. Song, W. Lu, J. Wang, P. Lu and Y. Wang, J. Org. Chem., 2010, 75, 3481–3483 CrossRef CAS PubMed;
(f) W. Song, M. Lei, Y. Shen, S. Cai, W. Lu, P. Lu and Y. Wang, Adv. Synth. Catal., 2010, 352, 2432–2436 CrossRef CAS;
(g) E. J. Yoo, S. H. Park, S. H. Lee and S. Chang, Org. Lett., 2009, 11, 1155–1158 CrossRef CAS PubMed.
- Y. Zhao, L. Li, Z. Zhou, M. Chen, W. Yang and H. Luo, Org. Biomol. Chem., 2021, 19, 3868–3872 RSC.
- E. J. Yoo, M. Ahlquist, I. Bae, K. B. Sharpless and V. V. Fokin, J. Org. Chem., 2008, 73, 5520–5528 CrossRef CAS PubMed.
-
(a) X. Luo, Z. Yang, J. Zheng, G. Liang, H. Luo and W. Yang, Org. Lett., 2022, 24, 7300–7304 CrossRef CAS PubMed;
(b) W. Yang, Y. Zhao, Q. Bu, L. Li, B. Zhou and Z. Huang, Org. Lett., 2022, 24, 457–461 CrossRef CAS PubMed.
-
(a) H. Jiang, J. Yang, X. Tang, J. Li and W. Wu, J. Org. Chem., 2015, 80, 8763–8771 CrossRef CAS PubMed;
(b) H. Jiang, J. Yang, X. Tang, J. Li and W. Wu, J. Org. Chem., 2016, 81, 2053–2061 CrossRef CAS PubMed.
-
(a) B. Muthu Ramalingam, N. Dhatchana Moorthy, S. R. Chowdhury, T. Mageshwaran, E. Vellaichamy, S. Saha, K. Ganesan, B. N. Rajesh, S. Iqbal, H. K. Majumder, K. Gunasekaran, R. Siva and A. K. Mohanakrishnan, J. Med. Chem., 2018, 61, 1285–1315 CrossRef CAS PubMed;
(b) D. E. Beck, M. Abdelmalak, W. Lv, P. V. Reddy, G. S. Tender, E. O'Neill, K. Agama, C. Marchand, Y. Pommier and M. Cushman, J. Med. Chem., 2015, 58, 3997–4015 CrossRef CAS PubMed.
- P. C. Too, Y. F. Wang and S. Chiba, Org. Lett., 2010, 12, 5688–5691 CrossRef CAS PubMed.
- D. Das and R. Samanta, Adv. Synth. Catal., 2018, 360, 379–384 CrossRef CAS.
|
This journal is © The Royal Society of Chemistry 2024 |
Click here to see how this site uses Cookies. View our privacy policy here.