DOI:
10.1039/D4RA04781H
(Paper)
RSC Adv., 2024,
14, 25273-25288
Ni–Sr/TiZr for H2 from methane via POM: Sr loading & optimization†
Received
1st July 2024
, Accepted 6th August 2024
First published on 13th August 2024
Abstract
Achieving remarkable H2 yield with significantly high H2/CO over Ni-based catalysts through partial oxidation of methane (POM) is a realistic approach to depleting the concentration of CH4 and using H2 and CO as synthetic feedstock. This study examined Ni catalysts on titania–zirconia for methane conversion via POM at 600 °C and atmospheric pressure. The addition of strontium to the catalyst was explored to improve its performance. Catalysts were characterized by X-ray diffraction, Raman-infrared-UV-vis spectroscopy, and Temperature-programmed reduction-desorption techniques (TPR, TPD). 2.5 wt% Sr addition induced the formation of the highest concentration of extreme basic sites. Interestingly, over the unpromoted catalyst, active sites are majorly generated by hardly reducible NiO species whereas upon 2.5 wt% promoted Sr promotional addition, most of active sites are derived by easily reducible NiO species. 45% CH4 conversion and 47% H2 yield with H2/CO = 3.5 were achieved over 2.5 wt% Sr promoted 5Ni/30TiO2 + ZrO2 catalyst. These results provide insight into the role of basic sites in enhancing activity through switching indirect pathways over direct pathways for POM. Further process optimization was carried out in the range of 10
000–22
000 SV, 0.35–0.75 O2/CH4, and 600–800 °C reaction temperature over 5Ni2.5Sr/30TiO2 + ZrO2 by using central composite design under response surface methodology. The optimum activity as high as ∼88% CH4 conversion, 86–87% yield of H2, and 2.92H2/CO were predicted and experimentally validated at 800 °C reaction temperature, 0.35O2/CH4 ratio, and 10
000 space velocity.
1. Introduction
In the current global warming scenario, mitigation of greenhouse gases is urgently needed to save the ecosystem on Earth. Among the greenhouse gases, methane is 25 times more potent than CO2.1 Catalyst communities across the globe are trying to develop a proper catalytic route for the conversion of CH4. The catalytic direct decomposition of CH4 into C and H2 is struggling due to massive carbon decomposition and quick deactivation. Oxidant-assisted oxidation of CH4 also gives way to continuous oxidation of carbon deposits into the syngas (H2 and CO). In dry reforming of methane (DRM), CO2 is the oxidant; in the steam reforming of methane (SRM), H2O is the oxidant; and in partial oxidation of methane, O2 is the oxidant for CH4 oxidation (eqn (1)–(3)). The typical stoichiometric H2/CO ratio in DRM, POM, and SRM is 1, 2, and 3, respectively. |
CH4 + CO2 ↔ 2H2 + 2CO
| (1) |
|
CH4 + 0.5O2 ↔ 2H2 + CO
| (2) |
According to the stoichiometry of the POM reaction, the H2/CO ratio over the POM reaction should be equal to 2. But recently, >3H2/CO ratio during POM reaction has drawn marked attention.2,3 It indicates the presence of indirect reaction pathways along with the direct POM pathway (Fig. 1) The indirect reaction pathway of POM can be summarized as the total oxidation of CH4 into “CO2 and H2O” (Fig. 1, step 1) followed by the involvement of “CO2 and H2O” into the oxidation of CH4 through DRM (Fig. 1, step 2a) and SRM reaction (Fig. 1, step 2b) respectively. The total oxidation of CH4 is catalysed by NiO, whereas POM, DRM, and SRM are catalysed by metallic Ni.4 Overall, the direct and indirect pathways of POM decide the final production distribution.
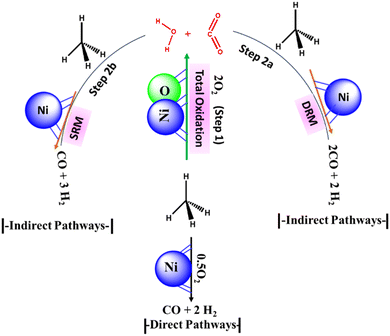 |
| Fig. 1 The reaction scheme for POM (A) direct pathways (B) indirect pathways. | |
CH4 and O2 undergo control oxidation over B2O3-based catalysts and form formate species which is not dissociated further.5 Ru dispersed over Al2O3 (Ru/Al2O3) carried out the complete oxidation of CH4 and O2 into syngas. However, adding Re to Ru/Al2O3 catalyses CH4 and O2 to control oxidation into formate species, which dissociates into syngas.6 Combining noble metals like Rh, Ru, or Pd with a less expensive metal like nickel creates highly effective catalysts for (POM) reactions. Still, the usual high cost of catalysts is a significant limitation in practical applications.6–8 Selecting proper supports and promoters for Ni-based catalysts may be as efficient as noble metal-based catalysts. Ni supported over lantana and Ni supported over titania have little life span due to the oxidation of active sites Ni by support lantana or titania.9–11 The partial coverage of active sites by diffusion of TiO2 and partial phase transformation of titania phase were also reported.12 The alumina support may be a suitable carrier for Ni catalysts, but the acidic nature of alumina may be less interactive with acidic CO2 gas. So, one route of POM's indirect pathway (step 2a) may be affected. If we select essential support like MgO for the dispersion of Ni, The Ni/MgO catalyst was found to be more prompt to total oxidation of methane than partial oxidation.9 Ni has less dispersion over silica than zirconia.13 Even ZrO2 and yttria-stabilized-ZrO2 efficiently carry out POM reactions.14 Over yttria-stabilized-ZrO2, both CH4 and O2 undergo control oxidation and form format species, which further break down into syngas. That means it favours the direct methanation route. Again, Ni-supported over ZrO2 has a higher H2 uptake than Ni-supported over CeO2.10 The support ZrO2 has phase transition issues at high temperatures, and Ni-supported over ZrO2 may be seriously affected by metal sintering. Overall, ZrO2 and TiO2 support have excellent redox properties. Their mobile lattice oxygen is easily accessible in an oxidation reaction, which can minimize the time delay in oxygen transport in POM reaction. However, individually, both have a severe phase transition, and titania has excessive diffusion and active sites' oxidation issues. We have recently developed titania-modified ZrO2 support, and the new hybrid support has eliminated all the challenges of phase transition, excessive diffusion, and over-oxidation.15 When different types of promoters (Ce, Cs, and Sr) were added, Ni distributed over titania-modified zirconia (Ni/TiZr) continually maintained an H2/CO ratio of >3 for 300 minutes on stream.3 Sr addition over Ni/TiZr caused the highest activity due to the maximum concentration of active sites and the higher edge of reducibility. Cs addition over Ni/TiZr resulted in inferior catalytic activity due to the lowest density of basic sites. The enhanced reducibility was also observed upon the promotional addition of Sr over Ni/ZrO2–Al2O3 catalyst.16 The addition of Sr increases the basicity of the surface, which interacts with more acidic gases like CO2 in the indirect POM pathway and helps in the activation of CO2. Further, a large size of Sr can stabilize CO2 over the catalyst surface as a bidentate carbonate intermediate, as well as enhance the dissociation of C–H by surface oxygen species.17 Sr+2-mediated CO2 was found to be a better oxidizing agent, inhibiting the deposition of carbyne-type carbon.15 So, it is necessary to extend the research on the different loadings of Sr promoters over Ni/titania–zirconia catalysts toward POM. After proper catalyst selection, the next step is to set reaction conditions/experimental factors (like temperature, O2/CH4 ratio, and space velocity) to get maximum H2 yield with a high H2/CO ratio. Performing fresh reactions after varying reaction conditions in order to optimize activity needs much time, workforce, and expenditure. With the help of statistical tools like response surface methodology (RSM), the optimum activity can be predicted with a very high level of accuracy by few experimental data. The activity is collected as the response of a mathematical equation that considers all the experimental factors and their interactions.18 In dry reforming reaction, central composite design and Box–Behnken design under RSM are investigated where predicted values and experiment values are found very close to each other.19–23 However, for POM reactions, such statistical investigations are less considered and need to be explored by the catalytic community.
Herein, titania modified-ZrO2 is investigated for supporting catalytic active site “5 wt% Ni” and promoter “1–3 wt% Sr” in the target of limiting phase transition of ZrO2 and restricting the diffusion for “TiO2” and stabilizing the CO2 intermediate in favor of POM reaction. The catalysts are characterized by surface area-porosity measurement, X-ray diffraction, Raman, infrared, ultraviolet-visible spectroscopy, H2-temperature-programmed reduction, and O2-temperature-programmed oxide experiments, and CO2-temperature programmed desorption. The thorough characterization and catalytic activity reveal the role of Sr promoter for inducing phase stability and edge and strength of reducibility-basicity of 5Ni/30TiO2 + ZrO2 catalyst in the favour of POM reaction. The best catalyst is further investigated in process optimization by using a central composite design under response surface methodology in the range of 10
000–22
000 SV, 0.35–0.75 O2/CH4, and 600–800 °C reaction temperature.
2. Experimental
2.1 Materials
Ni(NO3)2·6H2O (purity 98%, Alfa Aesar), Sr(NO3)2 (Aldrich), 30 wt% TiO2–70 wt% ZrO2 (Daiichi Kigenso Kagaku Kogyo Co). As per the specification of 30 wt% TiO2–70 wt% ZrO2 (from Daiichi Kigenso Kagaku Kogyo Co., Ltd), it has a tetragonal-ZrO2 phase and diffuse anatase-TiO2 phase. The surface area of the 30 wt% TiO2–70 wt% ZrO2 is 124 m2 g−1, and 50% of the particles in the catalyst are smaller than 8.6 nm (D50 = 8.6 nm).
2.2 Catalyst preparation
5 wt% equivalent of Ni(NO3)2·6H2O aqueous solution and 1–3 wt% equivalent of Sr(NO3)2 solution nitrate aqueous solution is added over 30 wt.% TiO2–70 wt% ZrO2 under stirring at 80 °C temperature. Stirring continues until the solution evaporates and the mixture turns into a paste. The solution was dried at 110 °C for 24 hours and then calcined at 600 °C for three hours. The catalyst is abbreviated as Ni/30TiO2 + ZrO2 and NixSr/30TiO2 + ZrO2 (x = 1, 2, 2.5, 3 wt%).
2.3 Catalyst characterization
The BET surface area Powder X-ray diffraction (XRD) analysis of fresh catalyst was conducted by Rigaku (Miniflex) diffractometer using Cu Kα1 radiation (λ = 0.15406 nm) operated at 40 mA and 40 kV. The N2 adsorption–desorption and porosity results were obtained using a Micromeritics Tristar II 3020 surface area analyzer. 0.2–0.3 g of catalyst was degassed, and all samples were degassed before analysis using the Barrett, Joyner & Halenda (BJH) method. Temperature-programmed hydrogen reduction (H2-TPR) and temperature-programmed carbon dioxide desorption (CO2-TPD) measurements were performed on a chemisorption device (Micromeritics AutoChem II) by using a thermal conductivity detector over 70 mg catalyst sample, respectively. In H2-TPR, H2 absorption is monitored up to 1000 °C under 10% H2/He gas, whereas in CO2-TPD, CO2 desorption is monitored upon raising the temperature to 800 °C under 10% CO2/He gas. The transmission electron microscopy was conducted at 200 kV using an aberration-corrected JEM-ARM200F (JEOL) with a CEOS corrector. The spent catalyst sample underwent Raman analysis within the 1250–3000 cm−1 range using a Laser Raman Spectrometer (JASCO, Japan) with a 532 nm beam excitation and 1.6 mW laser intensity. The exposure time was set to 10 seconds with 3 accumulations.
2.4 Catalyst activity test
The partial oxidation of methane was carried out over a 0.1 g catalyst in a tubular stainless-steel fixed bed reactor (PID Eng. & Tech, 9 mm I.D.). The temperature for the catalytic reaction was provided by the cylindrical furnace circumference of the catalyst bed. The temperature at the catalyst bed was monitored by an axially fixed K-type thermocouple in the catalyst bed. In the target of creating active sites before partial oxidation of methane, a reductive pretreatment of the catalyst was carried out under hydrogen (flow rate 30 mL min−1) for 1 h at 800 °C. Further, to remove the hydrogen gas from the catalyst bed, the reactor was purged with N2. Then, the temperature of the reactor was stabilized at 600 °C for the POM reaction. The reaction gas mixture which consisted of 50% CH4, 25% O2, and 25% N2 gases was allowed to pass through the fixed catalyst bed with the total flow maintained at 24 mL min−1 and 14
400 mLg−1 h−1 space velocity. The products were analysed by a gas chromatograph equipped with a propak Q column, molecular sieve columns, and a thermal conductivity detector. The composition of effluent gases was calculated by the normalization method, and the equations for the determination of CH4 conversion, CO2 conversion, H2 yield, CO yield, and H2/CO ratio are used as follows: |
 | (4) |
|
 | (5) |
|
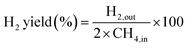 | (6) |
|
 | (7) |
|
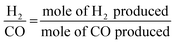 | (8) |
3. Result and discussion
3.1 N2 physisorption
The N2 adsorption isotherm and surface parameters (surface area, pore volume, and pore diameter) of 5Ni/30TiO2 + ZrO2 and 5NixSr/30TiO2 + ZrO2 (x = 1, 2, 2.5, 3) catalysts are shown in Fig. 2 and Table 1. The adsorption–desorption profile of 5Ni/30TiO2 + ZrO2 and 5NixSr/30TiO2 + ZrO2 (x = 1, 2, 2.5, 3) catalysts are characterized by type IV adsorption with H1 hysteresis loop which indicates the presence of mesoporous domains of the cylindrical architect.24,25 For such isotherm, a desorption branch is recommended for pore size analysis,26 and the “dV/dlog(w)” vs. “w” (“w” is pore width) plot shows pore size distribution. The 5Ni/30TiO2 + ZrO2 has a maximum surface area (124 m2 g−1), pore volume (0.36 cm3 g−1), and an average 8.6 nm pore diameter. The catalyst has a bimodal distribution of pore size in the range of 7.0 nm and 10.2 nm. Interestingly, upon promotional addition of Sr over 5Ni/30TiO2 + ZrO2, the pore size distribution becomes narrower and monomodal in the 6.3–6.8 nm range. The pore volume remains almost intact upon the addition of Sr promoters over the 5Ni/30TiO2 + ZrO2 catalyst. Upon adding 1–2 wt% Sr loading over 5Ni/30TiO2 + ZrO2, the surface area of the catalyst is decreased marginally. The 5Ni2Sr/30TiO2 + ZrO2 catalyst has a minimum surface area (119 m2 g−1) but a maximum pore diameter of 8.6 nm among the promoted samples.
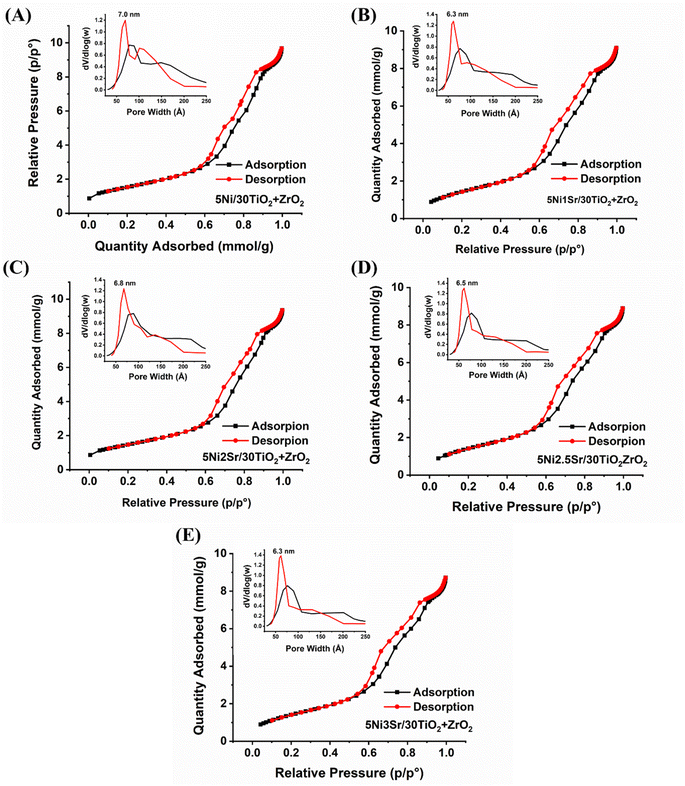 |
| Fig. 2 N2 adsorption isotherm and porosity distribution (inset figure) of 5Ni/30TiO2 + ZrO2 and 5NixSr/30TiO2 + ZrO2 (x = 1–3 wt%) catalysts. | |
Table 1 Surface area, pore volume, and average pore size of 5Ni/30TiO2 + ZrO2 and 5NixSr/30TiO2 + ZrO2 (x = 1–3 wt%) catalysts
Catalyst |
Surface area (m2 g−1) |
Pore volume (cm3 g−1) |
Average pore diameter (nm) |
5Ni/30TiO2 + ZrO2 |
124 |
0.36 |
8.6 |
5Ni1Sr/30TiO2 + ZrO2 |
123 |
0.36 |
7.8 |
5Ni2Sr/30TiO2 + ZrO2 |
119 |
0.37 |
8.6 |
5Ni2.5Sr/30TiO2 + ZrO2 |
122 |
0.36 |
7.8 |
5Ni3Sr/30TiO2 + ZrO2 |
121 |
0.35 |
7.6 |
3.2 X-ray diffraction
The X-ray diffraction study of fresh and spent 5Ni/30TiO2 + ZrO2 and 5NixSr/30TiO2 + ZrO2 (x = 1–3 wt%) catalysts are shown in Fig. 3. Fresh 5Ni/30TiO2 + ZrO2 catalyst has intense tetragonal ZrO2 phase (at Bragg's angle 2θ = 30.28°, 35.15°, 43.05°, 50.50°, 53.60°, 60.32°, 62.76°; JCPDS reference number 01-079-1771) and diffuse peaks for Rutile TiO2 phase (at Bragg's angle 2θ = 27.51°, 35.15°, 53.60°; JCPDS reference number 00-034-0180), anatase TiO2 phase (at Bragg's angle 2θ = 25.28°, 47.76°, 53.60°, 63.77°; JCPDS reference number 01-071-1168) and cubic NiO phase (at Bragg's angle 2θ = 43.20°; JCPDS reference number 00-047-1049). The crystalline phases for promoter oxides are not observed over Sr-promoted catalysts, which indicates the fine dispersion of promoter oxide. The X-ray diffraction intensity for ZrO2 and TiO2 phases is found to be a maximum of over 2.5 wt% Sr promoted 5Ni/30TiO2 + ZrO2 catalyst compared to other catalysts. The loading above 2.5 wt% Sr over 5Ni/30TiO2 + ZrO2 results in a fall of crystallinity of ZrO2 and TiO2 phases. Overspent catalysts, the diffraction pattern for metallic cubic Ni (44.68°; JCPDS reference number 00-004-0850) appears, and the peak intensity of tetragonal ZrO2, Rutile TiO2 and Anatase TiO2 phases are intensified. During the POM reaction, the crystalline peak intensity of tetragonal ZrO2 is grown slowest over 2 wt% Sr promoted 5Ni/30TiO2 + ZrO2 than the rest of the catalyst (Fig. 3E and F). It indicates the role of the promoter on support's crystallinity during the POM reaction.
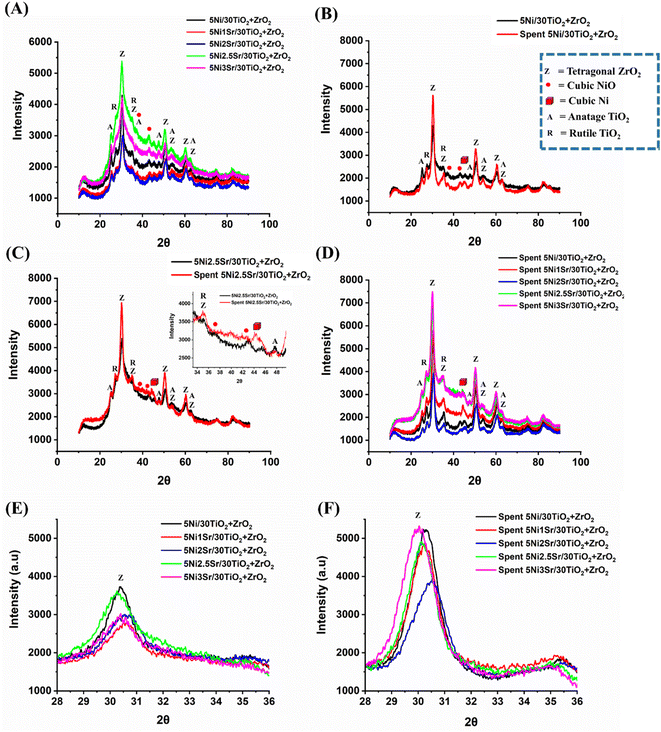 |
| Fig. 3 (A) Fresh XRD of all catalysts, (B) fresh-spent XRD of 5Ni/30TiO2 + ZrO2, (C) fresh-spent XRD of 5Ni2.5Sr/30TiO2 + ZrO2, (D) spent XRD of all catalysts, (E) fresh XRD peak of ZrO2 about 30.5° Bragg's angle (F), spent XRD peak of ZrO2 about 30.5° Bragg's angle. | |
3.3 Raman, infrared and ultra-violet spectra
The Raman spectra of 5Ni/30TiO2 + ZrO2 and 5NixSr/30TiO2 + ZrO2 (x = 1, 2, 2.5, 3) catalyst are shown in Fig. 4A. The Raman band at 395 cm−1 (B1g), 517 cm−1 (A1g, B1g), 642 cm−1 (Eg) are observed for anatase TiO2 phases whereas the Raman band at 834 cm−1 (B2g) signifies for rutile phase.27,28 The intensity of the anatase phase is higher than the rutile phase over titania–zirconia-supported Ni catalyst. Previously, it was reported that incorporating zirconia atom into titania lattice stabilized the anatase phase.27,29 The Raman band at 283 cm−1 and 642 cm−1 may be associated with partially tetragonal zirconia and partially by segregated TiO2 phases, or it may be related to (ZrTi) Ox material. Sr addition over 5Ni/30TiO2 + ZrO2 catalyst is found to induce the Raman vibration pattern incredibly. Upon addition of just 1 wt% Sr over 5Ni/30TiO2 + ZrO2, the Eg vibration band for anatase TiO2 (at 642 cm−1) and the B2g vibration band for rutile phase (834 cm−1) is suppressed, and the new Raman vibration band about 550 cm−1 for amorphous ZrO2 phase is appeared.27 Upon further loading of Sr up to 3% over 5Ni/30TiO2 + ZrO2, the intensity of most of the Raman bands declined sharply. Sr addition over 5Ni/30TiO2 + ZrO2 diminishes the degree of polarizability greatly. Infrared spectra reveal that upon Sr loading, the intensity of the O–H vibration peak (stretching vibration at 2435 cm−1 and bending vibration at 1638 cm−1) is decreased sharply (Fig. 4B). The depletion of surface hydroxyl's intensity upon Sr loading may be due to the formation of Sr–O–M (M = Ti, Zr, Ni) by condensation of SrOH and MOH (M = Ti, Zr, Ni). Even at simple atmospheric conditions, the unpromoted catalyst (5Ni/30TiO2 + ZrO2) shows the vibration band bidentate formate at 1355 cm−1 whereas Sr promoted 5Ni/30TiO2 + ZrO2 catalyst has a vibration band for both bidentate formate at 1355 cm−1 and ionic carbonate at 1460 cm−1 (Fig. 4B).30,31 Sr incorporation does not alter the material's UV absorption properties or the energy gap between its valence and conduction bands (Fig. 4C and D). The bandgap in all catalysts remains at about 3.1 eV. That means Sr addition doesn't affect the electronic transition pattern over the catalyst surface.
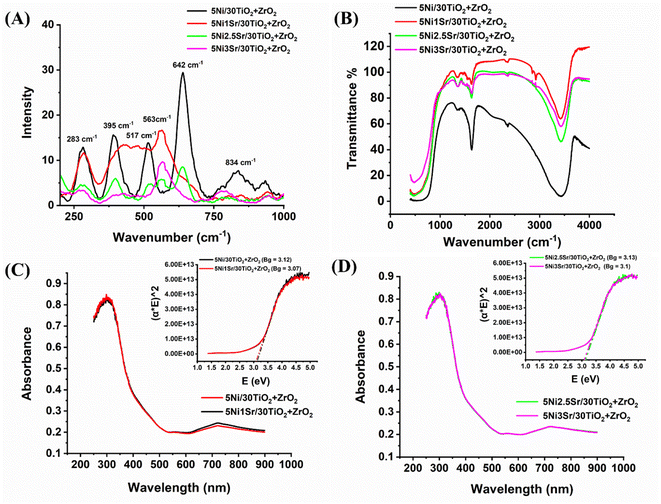 |
| Fig. 4 (A) Raman spectra, (B) infrared spectra (full range), (C) ultra-violet spectra of 5Ni/30TiO2 + ZrO2 and 5Ni1Sr/30TiO2 + ZrO2 catalysts, (D) ultra-violet spectra of 5Ni2.5Sr/30TiO2 + ZrO2 and 5Ni3Sr/30TiO2 + ZrO2 catalysts. | |
3.4 H2-temperature and CO2-temperature programmed profiles
The H2-temperature programmed reduction study of 5Ni/30TiO2 + ZrO2 and 5NixSr/30TiO2 + ZrO2 (x = 1, 2, 2.5, 3) catalysts are shown in Fig. 5A. The reduction profile of the catalyst at different temperatures indicates the extent of the interaction of reducible species with support. A peak in the reduction process is observed around 600 °C. This suggests the reduction of nickel oxide (NiO) species that moderately interacted with the ESI.†
32 The CO2-temperature programmed desorption profile of 5Ni/30TiO2 + ZrO2 and 5NixSr/30TiO2 + ZrO2 (x = 1, 2, 2.5, 3) catalysts is shown in Fig. 5B and Table S1.† The CO2 desorption profile of the 5Ni/30TiO2 + ZrO2 catalyst system is populated with weak basic sites cantered at about 100 °C and moderate strength basic sites presented by a broad peak in the range of 150 to 400 °C. The first peak (about 100 °C) is due to the desorption of CO2 from surface hydroxyl (constituting weak basic sites), whereas the second peak is due to the desorption of CO2 from surface oxide ions (constituting moderate strength basic sites).33–36 Upon addition of 1 wt% Sr over 5Ni/30TiO2 + ZrO2 catalyst, the intensity of moderate strength basic sites increases, and a new desorption peak cantered about 475 °C also appears. It can be termed as a strong basic site constituted by bonded carbonate species (by Sr+2) over the catalyst surface.37 Overall, it can be said that CO2 interaction over the catalyst surface is increased at various basic sites upon the addition of basic Sr oxide. Upon 2 wt% Sr loading over 30TiO2 + ZrO2, the CO2 desorption peak at 420 °C disappeared, and a diffuse peak at about 730 °C appeared, which can be termed as extreme basic sites. 730 °C is reported for the decomposition temperature of SrCO3.38 Over 5Ni2Sr/30TiO2 + ZrO2 catalyst, it can also be noticeable that when a new peak of about 730 °C is surged, the intensity of weak basic sites and moderate strength basic sites are not grown. This can be attributed to the engagement of SrO with CO2 and forms SrCO3, constituting an extreme basic site over the catalyst surface33 Further loading of Sr (2.5 wt%), weak basic sites, moderate strength basic sites, and extreme basic sites are grown. With increasing the Sr loading further to 3 wt%, both weak basic sites and moderate strength basic sites are grown, but the intensity of extreme basic sites declined. Overall, the basic site concentration is grown upon Sr loading over and 5Ni/30TiO2 + ZrO2 catalyst and 5Ni3Sr/30TiO2 + ZrO2 have the highest amount of basic sites (Table S1†).
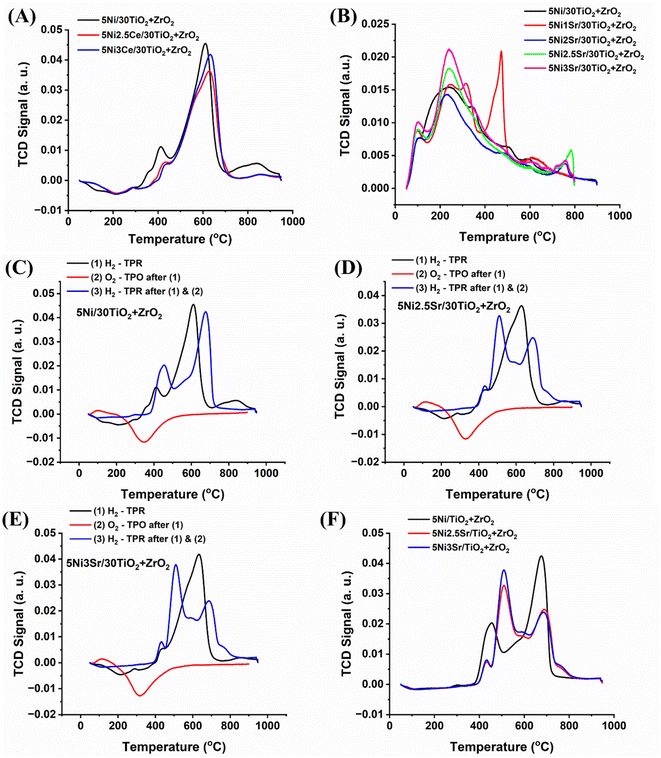 |
| Fig. 5 (A) H2-temperature programmed reduction profile of 5Ni/30TiO2 + ZrO2 and 5NixSr/30TiO2 + ZrO2 (x = 1, 2, 2.5, 3) catalysts, (B) CO2-temperature programmed desorption profile of 5Ni/30TiO2 + ZrO2 and 5NixSr/30TiO2 + ZrO2 (x = 0, 1, 2, 2.5, 3) catalysts. (C) Cyclic H2TPR-O2TPO-H2TPR profile of 5Ni/30TiO2 + ZrO2, (D) cyclic H2TPR-O2TPO-H2TPR profile of 5Ni2.5Sr/30TiO2 + ZrO2, (E) cyclic H2TPR-O2TPO-H2TPR profile of 5Ni3Sr/30TiO2 + ZrO2, (F) the final H2TPR (from cyclic H2TPR-O2TPO-H2TPR profile) of 5Ni/30TiO2 + ZrO2 and 5NixSr/30TiO2 + ZrO2 (x = 2.5, 3) catalysts. | |
POM is carried out over a reduced catalyst system where metallic Ni is the active site. However, it should be noticed that in POM, O2 is the oxidizing gas that can oxidize metallic Ni into NiO which turns the active sites inactive. In POM, H2 gas is coming out as a reaction product. It is reducing gas also and it can further reduce NiO into Ni. The presence of O2 and H2 may bring an oxidation–reduction cycle during the POM reaction which may change the reduction profile of the catalyst. To understand the reduction profile and rearrangement of the reduction profile under oxidizing gas (O2) and reducing gas (H2) during POM, an H2TPR-O2TPO-H2TPR cyclic experiment is carried out.
After sequential reduction–oxidation–reduction treatment (by H2TPR-O2TPO-H2TPR cyclic experiment) of 5Ni/30TiO2 + ZrO2 catalyst, the reduction peak is shifted to the higher temperature (from 640 °C). More interestingly, a new reduction peak of relatively less intensity appeared at about 400 °C which is attributed to NiO species having weak interaction with the support (Fig. 5C). That means a major part of NiO species undergoes stronger metal–support interaction and some parts of NiO interact with weak interaction. The high-temperature peak (640 °C) is reduced hardly whereas the low-temperature reduction peak (400 °C) is easily reducible. Interestingly if 2.5–3 wt% Sr promoted 5Ni/30TiO2 + ZrO2 catalysts are sequentially tested for reduction–oxidation–reduction treatment (by H2TPR-O2TPO-H2TPR cyclic experiment), the low-temperature reduction peak is magnified than the high-temperature peak (Fig. 5D–F). It indicates that over 5Ni2.5Sr/10TiO2 + ZrO2 and 5Ni3Sr/10TiO2 + ZrO2 catalysts, the amount of easily reducible NiO is more than hardly reducible NiO species. So, 5Ni2.5Sr/10TiO2 + ZrO2 and 5Ni3Sr/10TiO2 + ZrO2 catalysts have more active sites which is derived from easily reducible NiO and available from early temperature ranges.
3.5 Catalytic activity results
Generally, zirconia has prominent monoclinic phases, which are unstable, and in the same way, titania has both anatase and rutile phases. Interestingly, 30 wt% titania–70 wt% zirconia has only a stable tetragonal zirconia phase and anatase titania phases. That means the presence of titania stabilizes the tetragonal phase of zirconia, whereas the incorporation of zirconia into the titania crystal stabilizes the anatase phase of titania.27,29 The stable phases of titania–zirconia make it a good choice for supporting the active site “Ni” for partial methane oxidation.
The catalytic activity in terms of CH4 conversion, H2 yield, CO yield and H2/CO ratio, and CO2 yield over Ni/30TiO2 + ZrO2 and NixSr/30TiO2 + ZrO2 (x = 0, 1, 2, 2.5, 3) catalysts are shown in Fig. 6. NiO is stabilized over a 30TiO2 + ZrO2 catalyst with moderate strength (as verified by H2-TPR). The 5Ni/30TiO2 + ZrO2 catalyst is reduced before POM in the target of preparing active sites “Ni” for POM reaction. Under the oxidizing gas stream O2 (which is one of the feeds of POM) and reducing gas stream H2 (which is one of the products of POM), active sites are reorganized and major of the active sites (Ni) is generated by the reduction of hardly reducible NiO. 5Ni/30TiO2 + ZrO2 catalyst achieves 43–40% CH4 conversion during 240 minutes time on stream. In the mean of product distribution, ∼30% H2 yield (H2/CO ∼4) and ∼30% CO2 yield was maintained for up to 240 minutes on stream. From here, two points should be discussed in more depth. The first one is “equal H2 yield and CO2 yield” over a 5Ni/30TiO2 + ZrO2 catalyst. It indicates the presence of both partial oxidation and total oxidation of methane over titania–zirconia supported Ni catalyst. Jin et al. observed that CH4 was catalysed over metallic Ni surface and gave only gaseous H2, whereas NiO catalyses CH4 and gives CO, CO2, and H2O.4 That means NiO is an active site for total oxidation of methane and oxidation of metallic Ni into NiO (by O2) can be easily predicted during POM.39–41 Overall, the in situ concentration of both Ni and its oxide (NiO) decides the participation of CH4 in partial oxidation, in total oxidation, or both. The second point is that the mole of H2 is about 4 times the mole of CO over an unpromoted catalyst. If H2 and CO come from a POM reaction over a 5Ni/30TiO2 + ZrO2 catalyst, the stoichiometric ratio of H2/CO may not be more than 2. This indicates that H2 is also generated by other reaction pathways. This means, the total oxidation reaction takes place and the CO2 and H2O produced will react with methane in dry reforming and steam reforming, respectively.42 These pathways are known as indirect pathways of POM.43 Overall, the H2/CO ratio ∼4 indicates the increased participation of indirect pathways of POM compared to direct pathways. The water gas shift reaction (CO + H2O → CO2 + H2) is also feasible in this temperature range (600 °C) and it may also increase H2/CO ratio.2,44,45
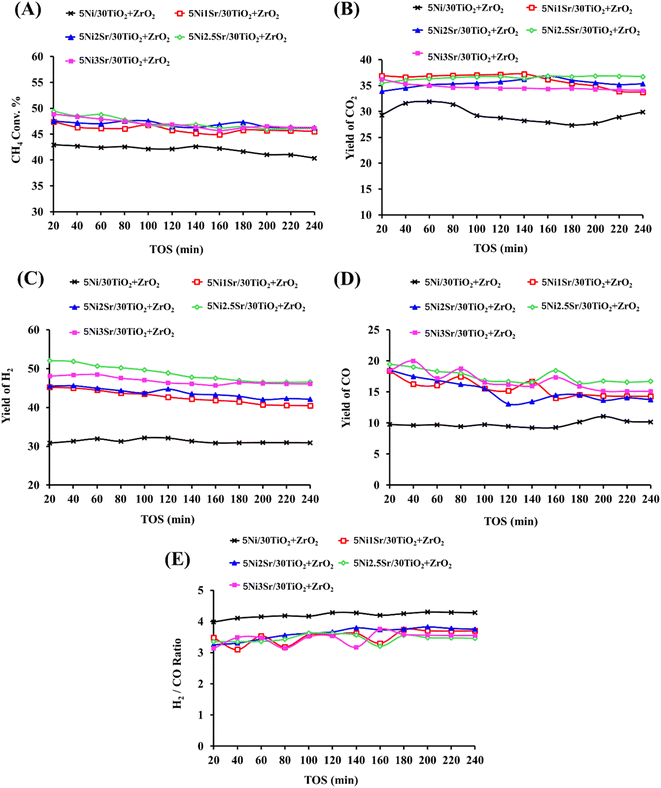 |
| Fig. 6 Time on stream of the catalytic activity results of 5Ni/30TiO2 + ZrO2 and 5NixSr/30TiO2 + ZrO2 (x = 1, 2, 2.5, 3) catalysts (A) CH4 conversion (%), (B) CO2-yield (%), (C) H2-yield (%). (D) CO yield (%), (E) H2/CO ratio. | |
Further, in search of optimum product yield, promotional addition of 1–3 wt% Sr is carried out over 5Ni/30TiO2 + ZrO2 catalyst where Sr is stabilized over catalyst surface by Sr–O–M (M = Ti, Zr, Ni) bond. Adding Sr over 5Ni/30TiO2 + ZrO2 catalyst brings narrower pore size distribution and enhances the formation of stronger basic sites over the catalyst surface. 1 wt% Sr over 5Ni/30TiO2 + ZrO2 catalyst may be specified with the presence of strong basic sites (bonded carbonate species by Sr+2), whereas 2–3 wt% Sr promoted 5Ni/30TiO2 + ZrO2 has the presence of extreme basic sites (due to SrCO3). 5Ni2.5Sr/30TiO2 + ZrO2 catalyst has the highest population of extreme basic sites among others. During the POM reaction, the active sites are distributed with different interactions with support in the presence of oxidizing gas (O2) and reducing gas (H2). H2TPR-O2-TPO-H2TPR cyclic profile shows that upon addition of 2.5 wt% Sr over 5Ni/30TiO2 + ZrO2, the amount of easily reducible NiO is increased than hardly reducible NiO. So, active sites generated by easily reducible NiO are exposed for POM from a quite early temperature. The CH4 conversion is enhanced to 47% and 50% over 5Ni1Sr/30TiO2 + ZrO2 and 5Ni2.5Sr/30TiO2 + ZrO2 catalysts, respectively. However, at the end of 100 minutes, CH4 conversion over both catalysts reached to 45%. However, at the end of 240 minutes; 5Ni2.5Sr/30TiO2 + ZrO2 catalyst achieved optimum catalytic activity in mean of higher H2 yield (47%) and CO2 yield (37%) than 5Ni1Sr/30TiO2 + ZrO2 catalyst (H2 yield = 40%, CO2 yield = 34%). This indicates that extreme basic sites over 5Ni2.5Sr/30TiO2 + ZrO2 catalysts induce POM reactions more towards indirect pathways than 5Ni1Sr/30TiO2 + ZrO2 catalysts. The H2/CO ratio over 5Ni2.5Sr/30TiO2 + ZrO2 catalyst is equal to 3.5 which indicates the precise presence of indirect pathways of POM. Upon further loading of Sr (3 wt%) over 5Ni/30TiO2 + ZrO2, the active sites distribution profile is similar than 5Ni2Sr/30TiO2 + ZrO2. The concentration of extreme basic sites decreases, whereas the concentration of moderate strength basic sites has grown. Overall, the catalytic activity over 5Ni3Sr/30TiO2 + ZrO2 is found to be relatively inferior to 5Ni2.5Sr/30TiO2 + ZrO2. 5Ni3Sr/30TiO2 + ZrO2 showed 46% CH4 conversion, 34% CO2 yield, 46% H2 yield at the end of 240 minutes on stream.
3.6 Process optimization
In the current POM experiment, temperature, O2/CH4 ratio, and space velocity are experimental factors that can be adjusted to get the maximum CH4 conversion, H2 yield, and H2/CO ratio. Now a days, central composite design (CCD) under response surface methodology is utilized frequently in optimization process. It forecasts the optimum response (activity) by using a few experimental data based on variation in experimental factors. Each experimental factor (xi) has a lower limit (ximin) and an upper limit (ximax). (ximax + ximin)/2
and (ximax − ximin)/2 are described as centre point of the experiment (
oi) and the deviation of each limit from center of experiment (Δxi) respectively. By using
oi and Δxi; the original value of experimental factors (xi) are coded into a dimensionless variable (Xi) as Xi = (xi −
oi)/Δxi. Table 2 lists the actual and coded values of the experimental factors.
Table 2 The actual and dimensionless variable of the experimental factors
Experimental factors |
Actual value (lower limit & upper limit) |
Centre point of the experiment ( oi) |
Deviation from the centre of the experiment (Δxi) |
Dimensionless variable (Xi) |
Space velocity (ccg−1 h−1) |
10 000 |
16 000 |
6000 |
−1 |
22 000 |
+1 |
Temperature (°C) |
600 |
700 |
100 |
−1 |
800 |
+1 |
O2 : CH4 |
0.35 |
0.55 |
0.2 |
−1 |
0.75 |
+1 |
RSM predicts the response (Ŷ) as per the function of experimental factors modeled under the quadratic polynomial model by using Taylor series expansion as shown below (eqn (9)). The basic terms of statistics related to error metrics (R2, APE, MAPE, MAE) are briefed in ESI under heading S2.† To refine the model and identify the significant factors & their interaction; analysis of variance (ANOVA) method for various components was carried out and shown in Table S3.† High F-values, high R2 values and low P-values indicate that the model terms are significant at approximately the 95% confidence level or at (p-values below 0.05). After excluding the insignificant function, models for CH4 conversion, yield of H2, and H2/CO ratio have been proposed using Design-Expert software version 13 (eqn (10)–(12)). Based on these models predicted values of response variables are shown in Table 3. The experimental data and the model's predicted data for CH4 conversion, yield of H2, and H2/CO ratio are shown in Table 3. R2 values for the expected models of CH4 conversion, yield H2, and H2/CO are 0.9898, 0.9933, and 0.9870, respectively. Table 3 confirms a strong correlation between predicted values and experimental results, with R2's near 1. Plotting predicted against actual values is crucial for model assessment, with close alignment to the X = Y line indicating a good fit. On average, the predicted values have absolute error of 0.95%, 0.81% and 0.78% compared to the actual values. A lower MAPE value indicates a higher level of accuracy in the models.
|
 | (9) |
where
X1,
X2,
X3 are the inputs in actual or coded values of the experimental factors, β0 is intercept coefficient, β
i, i = 1, 2, 3 are the linear coefficients,
βii are the quadratic coefficients,
βij,
j = 1, 2, 3 are the interaction coefficients and
ε is the error term.
18 |
 | (10) |
|
 | (11) |
|
 | (12) |
where
A,
B,
C represent temperature, O
2/CH
4 ratio and space velocity respectively.

is conversion of CH
4 and

is yield of hydrogen.
Table 3 Experimental and predicted data results for various components of the reaction system (Temp. = temperature, SV = space velocity, Ex. = experimental, Pre. = predicted, Er. = error)
Exp no |
Variables |
Response |
A (Temp.) |
B (O2/CH4) |
C (SV) |
CH4 conversion |
Yield of H2 |
H2/CO |
Ex. |
Pre. |
%|Er.| |
Ex. |
Pre. |
%|Er.| |
Ex. |
Pre. |
%|Er.| |
1 |
532 |
0.55 |
16 000 |
5.74 |
5.98 |
4.18 |
3.18 |
3.08 |
3.14 |
3.22 |
3.28 |
1.86 |
2 |
600 |
0.35 |
10 000 |
39.13 |
39.62 |
1.25 |
35.97 |
37.68 |
4.75 |
2.72 |
2.69 |
1.10 |
3 |
600 |
0.75 |
22 000 |
40.35 |
42.03 |
4.16 |
35.98 |
37.41 |
3.97 |
3.36 |
3.32 |
1.19 |
4 |
600 |
0.35 |
22 000 |
27.11 |
27.82 |
2.62 |
24.69 |
25.7 |
4.09 |
2.75 |
2.76 |
0.36 |
5 |
600 |
0.75 |
10 000 |
55.2 |
53.83 |
2.48 |
51.74 |
49.39 |
4.54 |
3.3 |
3.24 |
1.82 |
6 |
700 |
0.21 |
16 000 |
61.09 |
63.11 |
3.31 |
59.93 |
62.07 |
3.57 |
3.27 |
3.24 |
0.92 |
7 |
700 |
0.55 |
16 000 |
78.01 |
75.19 |
3.61 |
75.01 |
72.02 |
3.99 |
2.32 |
2.29 |
1.29 |
8 |
700 |
0.55 |
16 000 |
77.5 |
75.19 |
2.98 |
75.1 |
72.02 |
4.10 |
2.32 |
2.29 |
1.29 |
9 |
700 |
0.89 |
16 000 |
90.84 |
87.26 |
3.94 |
81.49 |
81.98 |
0.60 |
2.59 |
2.66 |
2.70 |
10 |
700 |
0.55 |
5909 |
83.55 |
85.11 |
1.87 |
81.65 |
82.09 |
0.54 |
2.14 |
2.22 |
3.74 |
11 |
700 |
0.55 |
16 000 |
78.01 |
75.19 |
3.61 |
74.01 |
72.02 |
2.69 |
2.32 |
2.29 |
1.29 |
12 |
700 |
0.55 |
26 091 |
63.41 |
65.26 |
2.92 |
62.18 |
61.95 |
0.37 |
2.32 |
2.36 |
1.72 |
13 |
800 |
0.75 |
10 000 |
97.14 |
99.44 |
2.37 |
96.32 |
99.06 |
2.84 |
1.63 |
1.69 |
3.68 |
14 |
800 |
0.75 |
22 000 |
86.75 |
90.63 |
4.47 |
83.46 |
87.08 |
4.34 |
1.86 |
1.77 |
4.84 |
15 |
800 |
0.35 |
22 000 |
78.22 |
76.43 |
2.29 |
76.84 |
75.37 |
1.91 |
2.89 |
3 |
3.81 |
16 |
800 |
0.35 |
10 000 |
87.67 |
88.23 |
0.64 |
85.96 |
87.35 |
1.62 |
2.98 |
2.92 |
2.01 |
17 |
868 |
0.55 |
16 000 |
91.22 |
87.63 |
3.94 |
89.29 |
86.53 |
3.09 |
2.21 |
2.18 |
1.36 |
|
|
|
|
MAPE |
2.98 |
|
|
2.95 |
|
|
2.06 |
3.6.1 Simulation on design expert program.
3.6.1.1 One factor effect (2D) plot. The effect of each process parameter on the reaction responses is shown in Fig. 7–9. Fig. 7 indicates that increasing temperature, increasing the O2/CH4 ratio, and decreasing the SV value will increase CH4 conversion. Fig. 8 indicates that increasing temperature, increasing the ratio and decreasing the SV value will increase Yield H2. Fig. 9 indicates that increasing temperature, increasing the ratio and increasing the SV value will increase H2/CO ratio.
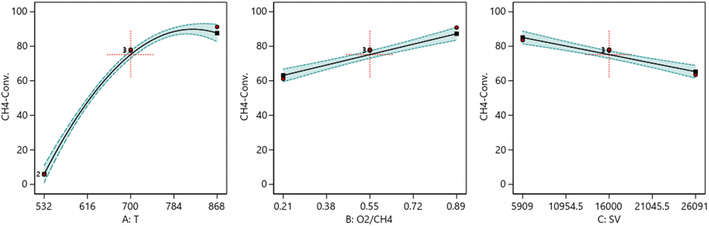 |
| Fig. 7 The relationship between the reaction parameters and CH4 conversion percentage. | |
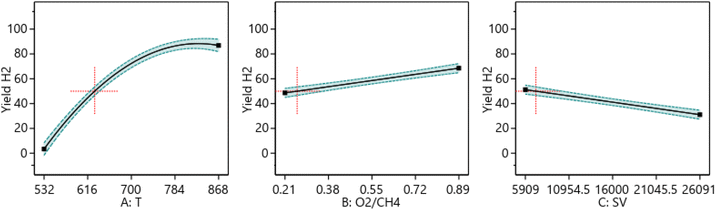 |
| Fig. 8 The relationship between the reaction parameters and yield H2 percentage. | |
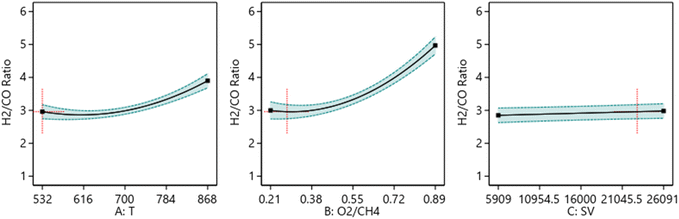 |
| Fig. 9 The relationship between the reaction parameters and H2/CO ratio. | |
3.6.1.2 Two factors effect (3D plot). One factor effect (2D) Plot is not suitable for assessing the relative impact of each factor due to coefficient scaling, and the intercept doesn't align with the center of the design space. Through the aid of the resulting equations, and design expert program the response surface plots were constructed for the predicted conversion or formation of the various components comprising the reaction system versus two process variables while keeping the third at a constant level or value as shown in the 3D models in Fig. 10–15. Fig. 10–11 show the three-dimensional response surface plots, which represents the effects of the factors (Temperature, SV, and ratio O2
:
CH4) on the variation of CH4 conversion. Fig. 10 shows the surface plots which represent the relationship between the response variable (CH4 conversion), and the two factors (Temperature and the ratio O2
:
CH4) at SV = 16
201. It is shown with increasing the temperature and increasing the ratio O2
:
CH4, the CH4 conversion increase. Fig. 11 shows the surface plots that represent the functional relationship between a designated response variable (CH4 conversion), and the two factors variables (Temperature and SV) with O2
:
CH4 fixed at 0.6112. The response surface shows with increasing the temperature and decreasing the SV, the CH4 conversion increases. It was observed to increase from 5.74% at 600 °C to 97.14% at 800 °C. All factors have significant effects but the temperature has the major effect on the variation of CH4 conversion.
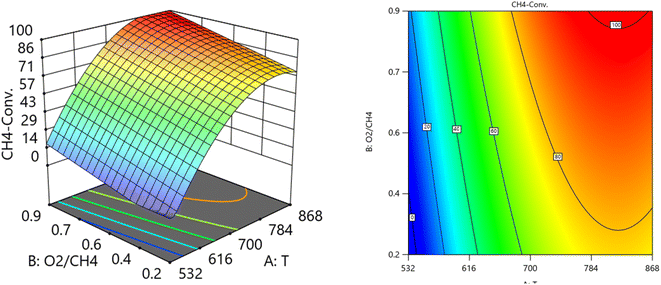 |
| Fig. 10 The relationship between the temperature, ratio, and CH4 conversion % at SV = 16 201. | |
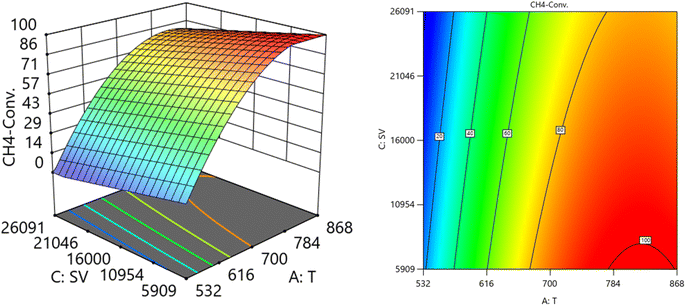 |
| Fig. 11 The relationship between the temperature, SV, and CH4 conversion % at ratio = 0.6112. | |
Fig. 12 and 13 show the three-dimensional response surface plots, which represents the effects of the factors (temperature, SV, and ratio O2
:
CH4) on the variation of yield of H2. Fig. 12 shows the surface plots which represent the relationship between the response variable (yield H2), and the two factors (temperature and the ratio O2
:
CH4) at SV = 7119.92. It is shown that by increasing the temperature and increasing the ratio O2
:
CH4, the Yield H2 increases Fig. 13 shows the surface plots that represent the functional relationship between a designated response variable (yield H2), and the two factors variables (temperature and SV) with O2
:
CH4 fixed at 0.4888. The response surface shows with increasing the temperature and decreasing the SV, the yield H2 increase. It was observed to increase from 3.18% at 600 °C to 96.32% at 800 °C.
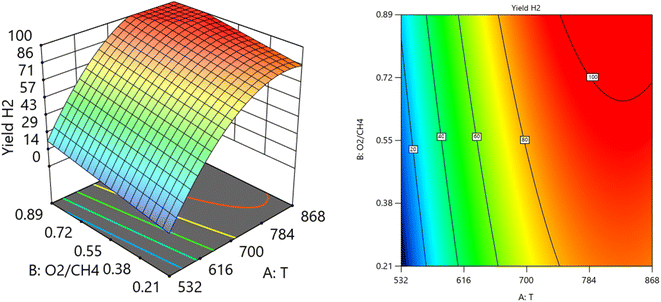 |
| Fig. 12 The relationship between the temperature, ratio, and yield H2 at SV = 7119.92. | |
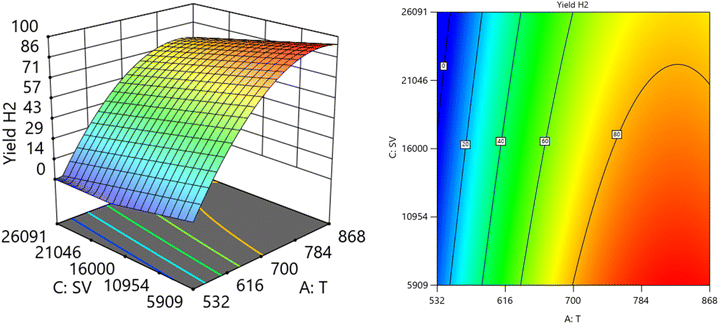 |
| Fig. 13 The relationship between the temperature, SV, and yield H2 at Ratio = 0.4888. | |
Fig. 14 and 15 show the three-dimensional response surface plots, that represents the effects of the factors (temperature, SV, and ratio O2
:
CH4) on the variation of H2/CO. Fig. 14 shows the surface plots which represent the relationship between the response variable (H2/CO), and the two factors (temperature and ratio O2
:
CH4) at SV = 23
000 at. It is shown the lowest H2/CO ratio is found at moderate values of both temperature and O2/CH4 ratio, which means neither too high nor too low values of both temperature and SV are optimal for minimizing the H2/CO ratio. Fig. 15 shows the surface plots that represent the relationship between the response variable H2/CO, and the two factors variables (temperature and SV) with O2
:
CH4 fixed at 0.3732. The plot shows that the H2/CO ratio is firstly influenced by temperature, with higher temperatures leading to lower H2/CO ratios, the space velocity has a lesser effect. It was observed to increase from 1.63% at 600 °C to 3.36% at 800 °C.
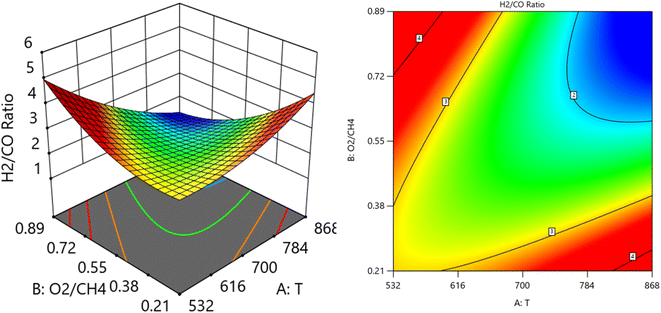 |
| Fig. 14 The relationship between the temperature, ratio, and H2/CO at SV = 23 000. | |
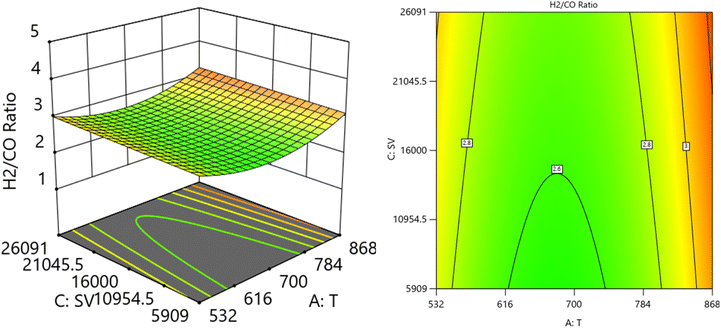 |
| Fig. 15 The relationship between the temperature, SV, and H2/CO at ratio = 0.3732. | |
3.6.1.3 Model prediction and validation. Fig. 16 shows optimum predicted simultaneous values of CH4 conversion, yield H2, and H2/CO ratio. Table 4 represents the comparison between predicted with experimental findings. The optimum catalytic activity (88.23% CH4 conversion, 87.35% yield H2, and 2.92H2/CO) towards POM over 5Ni2.5Sr/30TiO2 + ZrO2 catalyst is predicted at 800 °C reaction temperature, 0.35O2/CH4 ratio and 10
000 space velocity (SV). At these given reaction conditions, ∼88% CH4 conversion, 86% yield of H2, and 2.92H2/CO are achieved experimentally. The closeness of prediction results and experimental results validate the effectiveness of theoretical models.
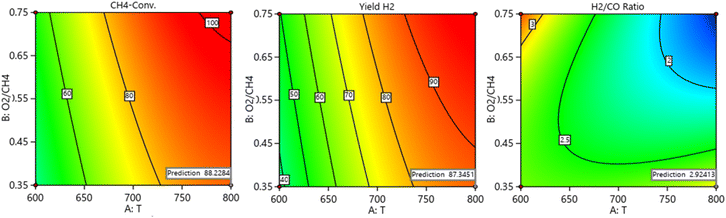 |
| Fig. 16 Optimum predicted simultaneous values of CH4 conversion, yield H2, and H2/CO ratio. | |
Table 4 Comparison of theoretical model predictions and experimental findings
|
Variables |
Objective function: max (CH4-conv.) & max (yield H2) & max (H2/CO) |
Criteria |
T Value |
O2 : CH4 value |
SV value |
H2/Co max. |
Yield H2% max. |
CH4-conv. max. |
Theoretical |
800 |
0.35 |
10 000 |
2.924 |
87.345 |
88.228 |
Experimental |
800 |
0.35 |
10 000 |
2.921 |
86.250 |
87.850 |
Further, the best catalyst (5Ni2.5Sr/30TiO2 + ZrO2) is investigated for long time on stream (28.5 hours) study at 800 °C, 0.35O2/CH4 ratio, and 10
000 space velocity (Fig. S1A and B†) and the spent catalyst is characterized with thermogravimetry analysis (Fig. S1C†). 44% weight loss is observed over spent 5Ni2.5Sr/30TiO2 + ZrO2 catalyst after 28.5 h time on stream reaction. But, the CH4 conversion and H2 yield remains above than 70% during entire time on stream. That means, active sites for POM remains exposed even after high carbon deposit.
4. Conclusion
30 wt% titania–70 wt% zirconia (30TiO2 + ZrO2) support has stable tetragonal ZrO2 and anatase TiO2 phases, which can stabilize the catalytic active Ni towards POM reaction. During the POM, the active sites undergo re-organization in the presence of oxidizing and reducing gases (O2 and H2). The majority of these active sites are formed from NiO species that are scarcely reducible. With equal hydrogen (30%) and CO2 (30%) yields, the 5Ni/30TiO2 + ZrO2 catalyst demonstrated 42% CH4 conversion, suggesting the existence of both partial (producing H2 and CO) and total (producing CO2 and H2O) oxidation. Due to the presence of a sufficient number of basic sites of moderate strength over 5Ni/30TiO2 + ZrO2, the total oxidation products (CO2 and H2O) interact with the surface, subsequently with CH4 under indirect pathways and achieve H2/CO ∼4. The promotional addition of 1 wt% Sr over 5Ni/30TiO2 + ZrO2 catalyst enhances additional strong basic sites, whereas 2.5 wt% Sr addition over 5Ni/30TiO2 + ZrO2 catalyst induces the generation of the highest concentration of extreme basic site. And 5Ni2.5Sr/30TiO2 + ZrO2 catalyst contains easily reducible NiO too. The easy reducibility promotes methane conversion whereas extreme basicity triggers an indirect pathway of POM by involving total oxidation products, CO2 and H2O, in the oxidation of CH4. The high yield of hydrogen (47%) with a ratio of hydrogen to carbon monoxide (H2/CO) of 3.5 suggests that these basic sites promote indirect pathways over direct pathways for methane conversion. Further process optimization is carried out over the best catalyst (5Ni2.5Sr/30TiO2 + ZrO2 catalyst) in the range of 10
00−22000 SV, 0.35–0.75 O2/CH4, and 600–800 °C reaction temperature by using central composite design under response surface methodology. ∼88% CH4 conversion, 86–87% yield of H2, and 2.92H2/CO is predicted and experimentally validated at 800 °C reaction temperature, 0.35O2/CH4 ratio, and 10
000 space velocity (SV). Hence, the validation experiment confirmed the correctness of the model since the experimental findings obtained under the expected optimal operating circumstances matched the predicted values pretty well.
Data availability
All data that support the findings of this study are included within the article.
Conflicts of interest
The authors declare that they have no known competing financial interest or personal relationships that could have appeared to influence the work reported in this paper.
Acknowledgements
The authors express their gratitude to Princess Nourah bint Abdulrahman University Researchers Supporting Project (Grant No. PNURSP2024R11), Princess Nourah bint Abdulrahman University, Riyadh, Saudi Arabia. Also the authors would like to extend their sincere appreciation to Researchers Supporting Project number (RSPD2024R612), King Saud University. RK and DMV acknowledge Indus University for supporting research.
References
- N. El Hassan, M. N. Kaydouh, H. Geagea, H. El Zein, K. Jabbour, S. Casale, H. El Zakhem and P. Massiani, Appl. Catal., A, 2016, 520, 114–121 CrossRef CAS.
- V. R. Choudhary, K. C. Mondal and T. V Choudhary, Appl. Catal., A, 2006, 306, 45–50 CrossRef CAS.
- A. S. Al-Fatesh, D. M. Vadodariya, K. M. Banabdwin, A. A. Ibrahim, A. H. Fakeeha, S. F. Adil, R. Kumar and A. A. M. Abahussain, Catal. Lett., 2024, 12, 1 Search PubMed.
- R. Jin, Y. Chen, W. Li, W. Cui, Y. Ji, C. Yu and Y. Jiang, Appl. Catal., A, 2000, 201, 71–80 CrossRef CAS.
- J. Tian, J. Tan, Z. Zhang, P. Han, M. Yin, S. Wan, J. Lin, S. Wang and Y. Wang, Nat. Commun., 2020, 11, 1–7 CrossRef.
- L. Li, N. H. MD Dostagir, A. Shrotri, A. Fukuoka and H. Kobayashi, ACS Catal., 2021, 11, 3782–3789 CrossRef CAS.
- S. Fazlikeshteli, X. Vendrell and J. Llorca, Fuel, 2023, 334, 126799 CrossRef CAS.
- Y. G. Chen, K. Tomishige, K. Yokoyama and K. Fujimoto, Appl. Catal., A, 1997, 165, 335–347 CrossRef CAS.
- C. Alvarez-Galvan, M. Melian, L. Ruiz-Matas, J. L. Eslava, R. M. Navarro, M. Ahmadi, B. R. Cuenya and J. L. G. Fierro, Front. Chem., 2019, 7, 1–16 CrossRef.
- Y. Qian-Gu, L. Chun-Rong, W. Wei-Zheng, Y. Le-Fu, W. Hui-Lin and W. Ting-Hua, Acta Phys. Chim. Sin., 2001, 17, 733–738 Search PubMed.
- H. Yang, Z. An, Y. Xu, L. Wu, L. Tan and Y. Tang, Mol. Catal., 2023, 547, 113374 CrossRef CAS.
- M. Shah, A. Bordoloi, A. K. Nayak and P. Mondal, Fuel Process. Technol., 2019, 192, 21–35 CrossRef CAS.
- Q. S. Jing and X. M. Zheng, Energy, 2006, 31, 2184–2192 CrossRef CAS.
- J. Zhu, J. G. Van Ommen and L. Lefferts, J. Catal., 2004, 225, 388–397 CrossRef CAS.
- A. Y. Elnour, A. H. Fakeeha, A. A. Ibrahim, A. I. Osman, A. E. Abasaeed, S. F. Adil, R. Kumar and A. S. Al-Fatesh, Res. Chem. Intermed., 2024, 50(3), 1211–1230 CrossRef CAS.
- A. S. Al-Fatesh, A. A. Ibrahim, A. I. Osman, A. E. Abasaeed, M. F. Alotibi, S. A. Alfatesh, D. W. Rooney, A. H. Fakeeha and C. Y. Yin, Energy Sci. Eng., 2023, 11, 3780–3789 CrossRef CAS.
- A. S. Al-Fatesh, M. M. Alrashed, R. A. El-Salamony, M. H. Roushdy, S. M. Alwan, A. I. Osman, M. Bayazed, A. H. Fakeeha, A. A. Ibrahim and R. Kumar, J. CO2 Util., 2023, 75, 102578 CrossRef CAS.
- B. V Ayodele, M. R. Khan, S. S. Nooruddin and C. K. Cheng, Clean Technol. Environ. Policy, 2017, 19, 1181–1193 CrossRef.
- M. S. A. Mohd Jailani, S. N. Miskan, M. B. Bahari and H. D. Setiabudi, Mater. Today Proc., 2023 DOI:10.1016/j.matpr.2023.04.690.
- M. A. Hossain, B. V. Ayodele, C. K. Cheng and M. R. Khan, J. Energy Inst., 2019, 92, 177–194 CrossRef CAS.
- M. Yusuf, A. S. Farooqi, M. A. Alam, L. K. Keong, K. Hellgardt and B. Abdullah, Int. J. Hydrogen Energy, 2022, 47, 31058–31071 CrossRef CAS.
- H. U. Hambali, A. A. Jalil, A. A. Abdulrasheed, T. J. Siang, A. H. K. Owgi and F. F. A. Aziz, Chem. Eng. Sci., 2021, 231, 116320 CrossRef CAS.
- C. C. Chong, Y. W. Cheng, H. D. Setiabudi, N. Ainirazali, D.-V. N. Vo and B. Abdullah, Int. J. Hydrogen Energy, 2020, 45, 8507–8525 CrossRef CAS.
- S. Mandal, A. Sinhamahapatra, B. Rakesh, R. Kumar, A. Panda and B. Chowdhury, Catal. Commun., 2011, 12, 734–738 CrossRef CAS.
- G. Price, Catal. Prep., 2006, 1, 283–295 Search PubMed.
- R. Kumar, Surface Characterization Techniques: from Theory to Research, Walter de Gruyter GmbH & Co KG, 2022 Search PubMed.
- M. Kyotani, H. Goto, K. Suda, T. Nagai, Y. Matsui and K. Akagi, J. Nanosci. Nanotechnol., 2008, 8, 1999–2004 CrossRef CAS.
- W. Liu, W. Zhong and Y. W. Du, J. Nanosci. Nanotechnol., 2008, 8, 2781–2792 CrossRef CAS PubMed.
- A. Naumenko, I. Gnatiuk, N. Smirnova and A. Eremenko, Thin Solid Films, 2012, 520, 4541–4546 CrossRef CAS.
- X. Jia, X. Zhang, N. Rui, X. Hu and C. jun Liu, Appl. Catal., B, 2019, 244, 159–169 CrossRef CAS.
- J. Khatri, A. S. Al-Fatesh, A. H. Fakeeha, A. A. Ibrahim, A. E. Abasaeed, S. O. Kasim, A. I. Osman, R. Patel and R. Kumar, Mol. Catal., 2021, 504, 111498 CrossRef CAS.
- A. E. Abasaeed, M. S. Lanre, S. O. Kasim, A. A. Ibrahim, A. I. Osman, A. H. Fakeeha, A. Alkhalifa, R. Arasheed, F. Albaqi, N. S. Kumar, W. U. Khan, R. Kumar, F. Frusteri, A. S. Al-Fatesh and A. A. Bagabas, Int. J. Hydrogen Energy, 2023, 48, 26492–26505 CrossRef CAS.
- A. A. M. Abahussain, A. S. Al-Fatesh, Y. B. Rajput, A. I. Osman, S. B. Alreshaidan, H. Ahmed, A. H. Fakeeha, A. S. Al-Awadi, R. A. El-Salamony and R. Kumar, ACS Omega, 2024, 9(8), 9309–9320 CrossRef CAS PubMed.
- S. Ogo, A. Onda, Y. Iwasa, K. Hara, A. Fukuoka and K. Yanagisawa, J. Catal., 2012, 296, 24–30 CrossRef CAS.
- A. H. Lahuri, M. A. Yarmo and M. N. A. Tahari, Proceedings of the 3rd International Conference on Separation Technology, ed. M. A. A. Zaini, M. Jusoh and N. Othman, Springer Singapore, Singapore, 2021, pp. 175–195 Search PubMed.
- A. S. Al-Fatesh, R. Kumar, S. O. Kasim, A. A. Ibrahim, A. H. Fakeeha, A. E. Abasaeed, H. Atia, U. Armbruster, C. Kreyenschulte, H. Lund, S. Bartling, Y. Ahmed Mohammed, Y. A. Albaqmaa, M. S. Lanre, M. L. Chaudhary, F. Almubaddel and B. Chowdhury, Ind. Eng. Chem. Res., 2022, 61, 164–174 CrossRef CAS.
- F. Miccio, A. N. Murri and E. Landi, Ind. Eng. Chem. Res., 2016, 55, 6696–6707 CrossRef CAS.
- Y. Zhu, J. Sunarso, W. Zhou and Z. Shao, Appl. Catal., B, 2015, 172–173, 52–57 CrossRef CAS.
- S. Mrowec and Z. Grzesik, J. Phys. Chem. Solids, 2004, 65, 1651–1657 CrossRef CAS.
- R. Haugsrud, Corros. Sci., 2003, 45, 211–235 CrossRef.
- P. A. Hintz and K. M. Ervin, J. Chem. Phys., 1995, 103, 7897–7906 CrossRef CAS.
- S. Yu, Y. Hu, H. Cui, Z. Cheng and Z. Zhou, Chem. Eng. Sci., 2021, 232, 116379 CrossRef CAS.
- W. K. Darkwah, A. B. Appiagyei, J. B. Puplampu and J. Otabil Bonsu, Langmuir, 2023, 39, 8568–8588 CrossRef CAS PubMed.
- N. Patel, A. S. Al-Fatesh, N. A. Bamatraf, A. I. Osman, S. B. Alreshaidan, A. H. Fakeeha, I. Wazeer and R. Kumar, Catal. Lett., 2024, 30, 1–6 Search PubMed.
- M. Serhan, M. Sprowls, D. Jackemeyer, M. Long, I. D. Perez, W. Maret, N. Tao and E. Forzani, AIChE Annuaul Meettin Conference Proceedings, 2022, vol. 349, p. 118485 Search PubMed.
|
This journal is © The Royal Society of Chemistry 2024 |
Click here to see how this site uses Cookies. View our privacy policy here.