DOI:
10.1039/D4RA04280H
(Paper)
RSC Adv., 2024,
14, 28376-28389
Zn–Al and Mg–Al layered double hydroxide nanoparticles improved primary and secondary metabolism of geranium plants†
Received
11th June 2024
, Accepted 24th August 2024
First published on 5th September 2024
Abstract
Layer double hydroxide (LDH) nanoparticles (NPs) have been applied to enhance plant growth and productivity. However, their effects on carbon and nitrogen metabolism of aromatic plants, are not well understood. Therefore, we investigated the impact of foliar application of Zn–Al LDH and Mg–Al LDH NPs (10 ppm) on the growth and metabolism of geranium plants. Zn–Al LDH and Mg–Al LDH NPs significantly increased the dry biomass, photosynthetic pigment, and Zn and Mg uptake by treated plants. These increases were consistent with increased primary metabolism such as soluble sugars and their metabolic enzymes (invertase and amylase). The supply of high sugar levels induced TCA organic accumulation, providing a pathway for amino acid biosynthesis. Among amino acids, proline level and its biosynthetic enzymes such as pyrroline-5-carboxylate reductase (P5CR), ornithine aminotransferase (OAT), and pyrroline-5-carboxylate synthetase (P5CS), glutamine synthetase (GS), and arginase were increased. Increased primary metabolites can then be channeled into secondary metabolic pathways, leading to higher levels of secondary metabolites including tocopherols, phenolics, and flavonoids. These observed increases in primary and secondary metabolites also improve the biological value of geranium plants. Overall, our research highlights the potential of Zn–Al LDH and Mg–Al LDH NPs as elicitors to enhance metabolism in geranium plants, thereby improving their growth bioactivity.
1. Introduction
Nanotechnology is a modern research field dealing with materials having a particle size of less than 100 nm, with diverse applications in various fields.1 Nanotechnology has become a crucial field in modern agriculture, facilitating accelerated plant growth and offering improved plant protection with less environmental impact compared to traditional approaches.2 The use of layered double hydroxide (LDH) nanoparticles (NPs) in agriculture influences plants' physiological and biochemical processes by fertilizing and suppressing pests.3 The physicochemical properties of NPs include a high surface-to-volume ratio.4 These features enable the use of NPs as fertilizers, which aids plant growth and development by allowing for the targeted and regulated release of mineral nutrients.5
LDHs are a type of ion exchanger with alternating positively charged metal hydroxide layers and negatively charged interlayer anions.5 LDH NPs are considered environmentally safe.6 They also possess a unique layered structure that enables ion exchange, resulting in more effective slow-release fertilizers for nutrients.5,7 Furthermore, the advantages of LDH NPs over other NP alternatives are their low toxicity, regulated release, and biocompatibility.8 Zinc–aluminum (Zn–Al) LDH and magnesium–aluminum (Mg–Al) LDH have attracted a lot of attention due to their unique properties compared to other nanomaterials. Zn–Al LDH consists of alternating Zn2+ and Al3+ layers with hydroxide ions (OH−) in between, while Mg–Al LDH consists of alternating Mg2+ and Al3+ layers with hydroxide ions (OH−) in between. In agreement, previous studies showed that Zn2+ and Mg2+ ions incorporated into LDH structures can form Zn(OH)2, ZnO, Mg(OH)2, and MgO. Zn–Al and Mg–Al LDH NPs have excellent biocompatibility and bioavailability, making them suitable for agricultural use. Their controlled release properties minimize toxicity risks and ensure essential nutrients are available to plants. When they are applied at appropriate concentrations, they enhance nutrient uptake and promote plant growth without inducing toxicity.9 LDHs combined with NPK fertilizers effectively promote plant growth and reduce nutrient leaching in soil.10–12 In addition to agriculture applications, their in vivo and in vitro biocompatibility is utilized for gene delivery, drug delivery, bioimaging, and biosensing areas.13 Zn–Al and Mg–Al LDH improve the bioavailability of essential nutrients by providing a steady and controlled release of ions such as Zn2+ and Mg2+.5,7
Many metabolic functions in plants rely on Zn and Mg.14 Zn is an essential mineral element required for several physiological activities, including the formation of carbohydrates, proteins, and chlorophyll.15,16 Mg is a crucial component of chlorophyll because it is the center atom and keeps ribosomes structurally intact, which is necessary for protein synthesis, and also activates several enzymes.17 Fertilizers containing Zn and Mg have a beneficial effect on mineral absorption and cell division, which increases plant growth.18 Numerous NPs have a major impact on the growth and development of plants, as recent studies have shown. For instance, it has been shown that applying ZnO NPs significantly enhanced the growth of wheat, maize, and cotton, increasing crop production.19–21 Similarly, macronutrient levels in cotton plants, such as nitrogen (N), phosphorus (P), potassium (K), and Mg, were significantly and favorably affected by the foliar application of MgO NPs. In this context, a dose of 60 ppm of MgO NPs was found to be the optimal dose for increasing the SPAD chlorophyll value, which resulted in increased cotton growth.22 Tobacco plants treated with MgO NPs exhibit higher Mg absorption and growth stimulation.23
NPs not only affect primary growth parameters but also play a crucial role in modulating metabolic pathways. NPs increase sugar accumulation, indicating the availability of carbon for the biosynthesis of both primary and secondary metabolites.24 The impact of NPs has a significant effect on related metabolic pathways, such as the sugar and proline metabolism.25 Sugars and proline are involved in the storage and transfer of energy.26–28 Thus previous research indicated that increased proline levels improved plant growth.29 NPs are further supported by the upregulation of secondary metabolites, including tocopherol, phenolics, and flavonoids.30 These secondary metabolites are typically present in low concentrations and play a crucial role in all living organisms as antioxidants.31 Overall, there has been an increasing interest in these substances due to their practical applications in nutrition, medicine, and cosmetics, as well as their undeniable role in plant stress physiology.31
Given the potential of LDH NPs to enhance sustainable agriculture by improving crop quality and yield, this study aims to examine the effects of Zn–Al LDH and Mg–Al LDH NPs on both the primary and secondary metabolic parameters in geranium plants. We specifically investigated their effects on growth (shoot fresh weight and shoot dry weight), photosynthesis, primary metabolism, and the production of secondary metabolites such as flavonoids, phenolics, and tocopherols. Our goal is to gain a comprehensive understanding of the potential benefits of LDH NPs in enhancing geranium growth by elucidating these effects.
2. Materials and methods
2.1. LDH NP synthesis and characterization
The co-precipitation techniques were utilized to synthesize Mg–Al and Zn–Al LDH.32–34 Mg–Al LDH and Zn–Al LDH (molar ratio of 2
:
1) were synthesized using the co-precipitation method. MgSO4 (0.2 M) and Al2(SO4)3 (0.1 M) were dissolved in 100 mL H2O, and the pH was adjusted to 10 using 2 N NaOH under vigorous stirring at 60 °C. After 24 hours of stirring, the prepared LDH was collected and washed several times with warm distilled water until the pH reached 7 and then dried at 50 °C overnight.32–34 To prepare 10 ppm of Mg–Al and Zn–Al LDH,10 mg of catalyst was dissolved in 1000 mL of distilled water under continuous stirring for 3 hours. In parallel, Zn–Al LDH was synthesized using zinc sulfate (0.2 M). Stabilization of Zn–Al LDH and Mg–Al LDH NPs was measured through zeta potential analysis. All emulsions exhibited zeta potential values exceeding −30 mV, suggesting the presence of enough repulsive force between particles, ensuring colloidal stability. The samples of ZnAl LDH and MgAl LDH were found to have negative charges of −35.7 mV and −36.3 mV, respectively. The zeta potential experiments were conducted to assess the stability and charge of the NPs, based on their electrophoretic mobility.35
The ZS Malvern Zetasizer, using DLS and an automatic titrator, measured the particle size distribution, showing polydispersity with peaks at 2903 nm and 892 nm and average PdIs of 0.6 and 0.1 for ZnAl LDH and MgAl LDH, respectively (Fig. 2). These sizes are larger than those from SEM and XRD (ESI Fig. 1 and 2†) due to NP aggregation in the aqueous solution during DLS measurement, which doesn't occur in the dry state for SEM or XRD. The hydrodynamic diameters indicate swelling over time, reflecting the layered structure and thermal stability of LDHs in polymer matrices.
The surface properties were assessed using scanning electron microscopy (SEM). The samples were scanned in powder form, and surface modifications were done by mounting fine grains on conductive copper double-face tape, coating by gold using DC sputtering coater (SPI-instruments- Japan), and observation under Tungsten filament scanning electron microscope (ZEISS Sigma 500 VP microscope).
The crystalline structure of the synthesized materials was assessed through X-ray diffraction (XRD) analysis, using an X-ray diffractometer (PANalytical, Empyrean, Netherlands) with Cu-Kα radiation (wavelength 1.54045 Å). The XRD was operated at 40 kV and a current of 30 mA. To identify various functional groups, Fourier transform infrared (FTIR) spectra of the samples were recorded using a Vertex 70 system (400–4000 cm−1). Potassium bromide (KBr) was used as a control spectrum for FTIR because it is inactive in IR spectroscopy.
2.2. Experimental setup, sample collection, and treatments
The experiment was carried out in a greenhouse at Riyadh Village, located in the Beni-Suef Governorate of Egypt. The temperatures were 26 °C/18 °C Day/night, the light/dark cycle was 16/8 h, and humidity levels ranged from 50% to 60%. Clay soil rich with nutrients was used. The soil initially contained 13.7 mg organic carbon (C), 14.8 mg nitrate-nitrogen (N), 1.1 mg ammonium-N, and 10.7 mg phosphorus (P)/g air-dry soil at a humidity of 0.33 g water/g dry soil. The pH was 7.26, EC was 3.74 dS m−1 and K was 2.45 meq L−1. Geranium seedlings were provided from local farmland and treated with Zn-AL LDH and Mg–Al LDH NPs. At the vegetative stage, the plants were sprayed with 10 ppm of Zn-AL LDH and Mg–Al LDH NPs. The control group was sprayed with water. After 30 days of foliar application of LDH NPs, plant samples were collected for analysis. The samples' fresh weight was determined, followed by oven-drying at 70 °C. The outcomes represent the dry weights of the shoots.
2.3. Photosynthetic pigment
Total chlorophyll was measured using 80% acetone to extract 0.5 gm of fresh leaves, followed by centrifugation (10
000 g for 5 min). Results were expressed as (mg gm−1 f.wt.).36
2.4. Estimation of Zn and Mg content
Grounded geranium leaf (0.3 gm) was added to a digestive vessel and then mixed with 10 mL of 6 N HCl. The resulting mixture was heated on a hot plate using a thermostat set to 45 °C for 24 hours, keeping the temperature at 50 °C or below. Following digestion, the materials were put into a 25 millilitre volumetric flask and precisely diluted with deionized water. The technique of atomic absorption spectrophotometry (AAS) was used to quantify the amounts of zinc and magnesium present in the digested tissues after they had been filtered.37
2.5. Measurement of soluble sugars and starch content
Soluble sugars and starch content were measured.38 Sugars were extracted in 80% ethanol at room temperature. After drying, the extracts were again suspended in dH2O. For additional analysis, the supernatants were kept in storage at −20 °C. For starch extraction, was boiled to break down the starch grains and homogenized in water. After adding one milliliter of amyloglucosidase solution (0.5 units per mL in acetate buffer pH 4.5), the mixture was shaken at 55 °C to facilitate the digestion of starch. For ten minutes, the extraction was centrifuged at 2000 g. HPLC equipped with A diode array detector was used to detect soluble sugars, and the Coulter PACE system 5500 was used to determine concentrations.
2.6. Extraction and evaluation of the major enzymes involved in sugar metabolism
Sugar metabolism enzymes were initially extracted,39 and invertase activity was assessed in a TAE buffer (pH 8.5) with 0.1 M sucrose as substrate. Starch synthase activity was evaluated by using a reaction buffer containing citrate and glycogen.40 By tracking the breakdown of starch at 620 nm, amylase enzyme activity was determined.41
2.7. Organic acids in the tricarboxylic acid cycle (TCA)
The geranium shoots were treated with a solution containing 0.1% phosphoric acid and 0.3% butylated hydroxyanisole to extract organic acids using a MagNALyser (Roche, Vilvoorde, Belgium). The quantities of TCA organic acids were measured using an HPLC equipped with a SUPELCOGEL C-610H column and an ultraviolet (UV) detection system that operated at 210 nm (LaChrom L-7455 diode array, LaChrom, Tokyo, Japan). The mobile phase, 0.1% phosphoric acid, was eluted at a rate of 0.45 mL min−1.38
2.8. Metabolites of amino acids
Amino acids were extracted from geranium shoots using 80% (v/v) from aqueous ethanol. During the extraction process, norvaline—the internal standard—was introduced. The next step was centrifugation (16
000 g, 20 min). The supernatant was evaporated, and the residue was resuspended in chloroform. After centrifugation, the plant residue was extracted once more in HPLC-quality water, and the resulting supernatant was mixed with suspended chloroform pellets. The extracts were centrifuged and then run through 0.2 lm-pore size Millipore microfilters. Amino acids were separated on a BEH amide 2.1 9 50 column, and the outcomes were measured using Waters Acquity UPLC-tqd mass spectroscopy.42,43
2.9. Key enzymes involved in the metabolism of amino acids: extraction and estimate
Glutamine synthetase (GS), glutamine oxoglutarate aminotransferase (GOGAT), pyrroline-5-carboxylate dehydrogenase (P5CDH), pyrroline-5-carboxylate reductase (P5CR), and pyrroline-5-carboxylate synthetase (P5CS) enzymes activities were measured.44 The samples were extracted using Tris–HCl buffer 0.05 M (pH 7.4) containing 0.004 M DTT, 0.001 M EDTA, 10% glycerol, 2% (w/v) polyvinylpyrrolidone, 0.002 M PMSF, and 0.01 M MgCl2. Arginase (ARG) and ornithine aminotransferase (OAT) have been extracted using 0.05 M potassium phosphate buffer (pH 7.0) containing 0.002 M PMSF, 0.01 M 2-mercaptoethanol, 0.001 M EDTA, 15% glycerol, and 2% (w/v) polyvinylpyrrolidone. Protein content in the extracts was calculated using the Lowry method.45 Following the oxidation of NADH at A340 allowed for the measurement of P5CR activity.46 P5CS was evaluated using the measurement of the γ-glutamyl hydroxamate synthesis at A535. After developing the reduced form of NADH at A340 nm researchers measured P5CDH activity.47 The GOGAT activity was assessed by measuring the glutamine-dependent NADH oxidation at A340.48 A340 produced γ-glutamyl hydroxamate, indicating the presence of GS.49 OAT activity was measured by detecting the decrease of NADH at A340,50 whereas ARG activity was detected by following urea accumulation with the diacetyl monoxime method.51
2.10. Tocopherol content
HPLC analysis was used to determine the tocopherols, according to the following method.44 Using the MagNALyser (Roche, Vilvoorde, Belgium; 1 min, 7000 g), tocopherols were extracted with hexane. The tocopherols were separated and measured by HPLC (Shimadzu,'s Hertogenbosch, The Netherlands) (normal phase conditions, Particil Pac 5 μm column material, length 250 mm, i.d. 4.6 mm) after the dried extract (CentriVap concentrator, Labconco, KS, United States) was resuspended in hexane. The internal standard utilized was dimethyl tocol (DMT) at 5 ppm. The software Shimadzu Class VP 6.14 was used to analyze the data.
2.11. Phenolics
Using an orbital shaker, acetone water (250 mL, 4
:
1, v/v) was used to extract geranium samples, which were left at room temperature for 24 hours. The DPF hydroxyacetone crude extract was then obtained by filtering the extracts and centrifuging them for 10 minutes at 4000 g (Hettich Zentrifugen, Tuttlingen, Germany). Using a rotary evaporator (IKA-WERKE-RV06ML, Stanfer, Germany), the supernatant was concentrated under decreased pressure for three hours at 40 °C. The residues were measured as previously stated by52 and dissolved in HPLC grade MeOH. A sample (20 μL) that had been dissolved in methanol was briefly injected into a Shimadzu HPLC system (SCL-10 A vp, Shimadzu Corporation, Kyoto, Japan). The HPLC system consisted of a diode-array detector and a Lichrosorb Si-60 column measuring 7 μm by 3 × 150 mm. Water/formic acid (90
:
10, v/v) and acetonitrile/water/formic acid (85
:
10
:
5, v/v/v) made up the mobile phase. The quantification of flavonoids and phenolic acids that were tentatively identified was done using a calibration curve that was obtained using the relevant standards. The findings were given as μmol gm−1 DW.
2.12. Statistical analysis
All data were analyzed using one-way analysis of variance (ANOVA) followed by Duncan's multiple range test at a significance level of P < 0.05. Each experiment was performed at least three times (n = 3). SPSS version 20 was used for statistical analysis, and GraphPad Prism was used for creating the graphs. Data normality was checked by using Levene's test. PCA analysis Euclidean distance was performed using the R stat software package (version 4.5.0, the R).
3. Results
3.1. Characterization of LDH NPs
3.1.1 Scanning electron microscopy (SEM). SEM analysis revealed that Zn–Al LDH NPs appear as stacked arranged layers with non-uniform nonporous features and the average particle size is 22 nm. Mg–Al LDH NPs exhibited layer-shaped surface morphology with consistent nonporous properties and the average particle size is 41 nm Fig. S1.†
3.1.2 Fourier-transform infrared spectroscopy (FTIR). The Zn–Al LDH and Mg–Al LDH NPs' FTIR spectra showed distinctive absorption peaks, indicating the existence of crucial functional groups within the LDH structure. All LDH samples exhibited shared absorption bands, including a broad absorption band spanning the 3300–3500 cm−1 range attributed to the OH stretching mode of the basal layer and interlayer water. Additionally, a band within the 1620–1650 cm−1 range was credited to the bending mode of interlayer water and anion groups. A distinct band in the region of 400 to 800 cm−1 was observed, related to MO and MOH vibrations. These functional groups could have significant implications for interaction with plant tissues Fig. S2.†
3.1.3 X-ray diffraction (XRD). The Zn–Al LDH and Mg–Al LDH NPs' XRD patterns confirmed the formation of crystalline phases. Zn–Al LDH NPs showed diffraction peaks at various angles, indicating a layered structure with an average crystalline size near 15–20 nm. Mg–Al LDH NPs also displayed diffraction peaks at different angles, with an average crystalline size near 30–35 nm Fig. S2.†
3.2. Growth and biomass buildup
In an experiment, we exposed geranium seedlings to Zn–Al LDH and Mg–Al LDH NPs and then measured the fresh and dry weights of the seedlings to assess their growth. Compared to the control group, the application of Zn–Al LDH and Mg–Al LDH NPs led to a significant increase in fresh weight by 34% and 39%, respectively, and dry weight by 48% and 54%, respectively Table 1.
Table 1 The effects of Zn–Al LDH and Mg–Al LDH NPs on geranium shoot fresh weight, dry weight, total chlorophyll, and mineral content. The values show the three independent replicates' mean ± standard error. Statistically significant treatment differences (P < 0.05) are indicated by different letters after the means
Parameters |
Control |
Zn–Al LDH NPs |
Mg–Al LDH NPs |
Shoot fresh weight (gm) |
16.9 ± 0.52b |
24.9 ± 1.5a |
26 ± 0.57a |
Shoot dry weight (gm) |
3.63 ± 0.29b |
5.66 ± 0.53a |
6.46 ± 0.53a |
Total chlorophyll (mg g−1 FW) |
8.88 ± 2.11b |
10.92 ± 0.5 ab |
12.59 ± 0.89a |
Zn content (mg kg−1 FW) |
2.6 ± 1.33c |
16.9 ± 3.42a |
4.1 ± 1.05b |
Mg content (mg kg−1 FW) |
1.33 ± 0.44c |
2.4 ± 1.91b |
20.2 ± 7.75a |
3.3. Pigments analysis
We assessed the levels of total chlorophyll in geranium seedlings after exposing them to Zn–Al LDH and Mg–Al LDH NPs. The results in Table 1 reveal that Mg–Al LDH NPs had a favorable effect on geranium total chlorophyll. Geranium treated with Mg–Al LDH NPs had a greater total chlorophyll content (41%). Zn–Al LDH NPs demonstrated a non-significant increase in total chlorophyll.
3.4. Carbohydrates and sugar metabolism
Under Zn–Al LDH and Mg–Al LDH NPs, the amounts of soluble sugars (glucose, fructose, and sucrose) and insoluble sugars (starch) and the associated enzymes were measured in geranium Fig. 1. Obtained data showed that Mg–Al LDH NP application caused a significant increase in invertase by 216% compared to the control. These increases were parallel with the non-significant inhibition of sucrose (4%) and accumulation of glucose and fructose by 62% and 105% respectively. This was also matched with non-significant increases in starch compared to the control.
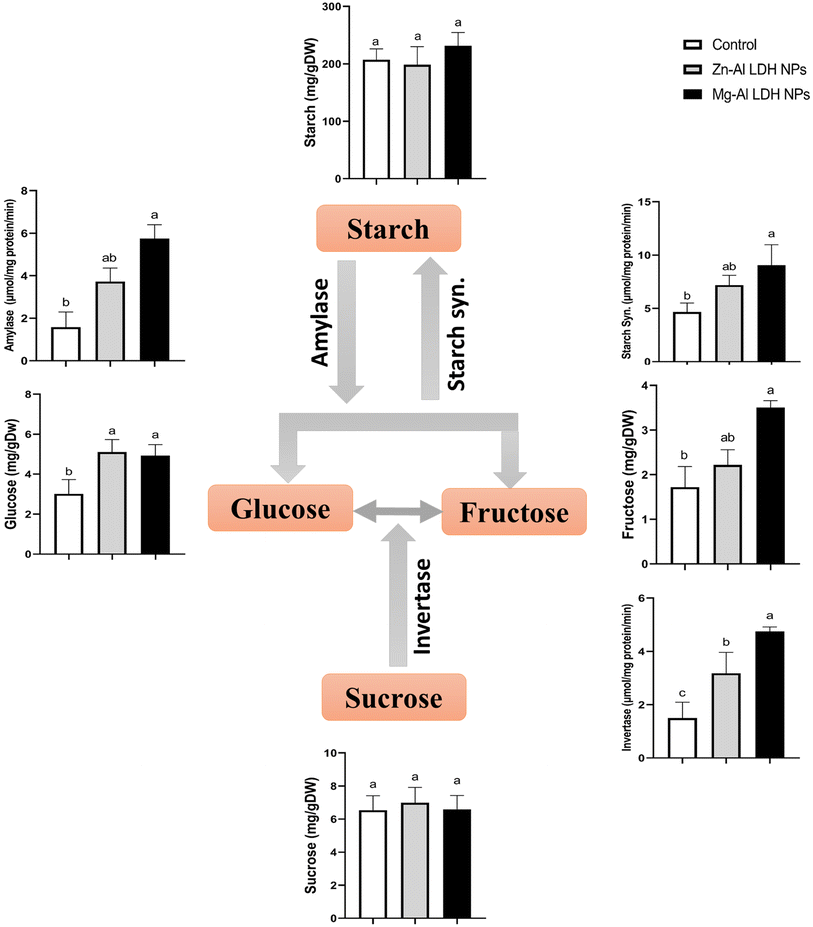 |
| Fig. 1 The changes in the contents of soluble and non-soluble sugars in geranium shoots treated with Zn–Al LDH and Mg–Al LDH NPs. Vertical bars represented standard error (±SE). Different letters denoting significant changes between treatments are displayed above the bars (P ≤ 0.05). | |
3.5. Tri carboxylic acid contents (TCA)
The study measured the concentrations of six TCA – oxalate, malate, succinate, citrate, lactate, and trans-aconitic acids in geranium shoots treated with Zn–Al LDH and Mg–Al LDH NPs. The findings demonstrated that in contrast to the control group, the application of Zn–Al LDH and Mg–Al LDH NPs increased citrate levels by 133% and 100%, respectively, and oxalate by 89% and 82%, respectively. The levels of malate, succinate, lactate, and trans-aconitic were not significantly affected by the treatment with either Zn–Al LDH or Mg–Al LDH NPs Fig. 2.
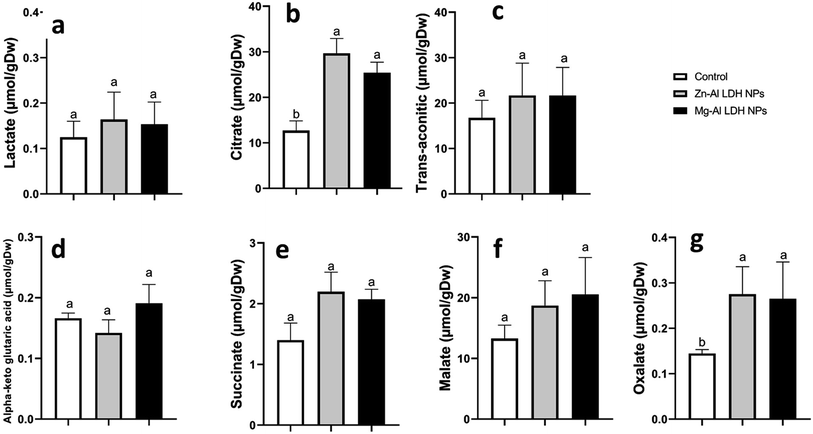 |
| Fig. 2 The changes in the organic acids contents in geranium plant shoots treated with Zn–Al LDH and Mg–Al LDH NPs. Vertical bars represented standard error (±SE). Different letters denoting significant changes between treatments are displayed above the bars (P ≤ 0.05). | |
3.6. Total protein and amino acids content
The amount of total protein and amino acids, including glycine, valine, phenylalanine, tyrosine, leucine, lysine, histidine, alanine, isoleucine, methionine, threonine, serine, aspartate, cystine, and asparagine, were measured in geranium shoots treated with Zn–Al LDH and Mg–Al LDH NPs (Fig. 3). Compared to the control, the application of Zn–Al LDH and Mg–Al LDH NPs had non-significant effects on the levels of both protein and amino acids, except for glycine, valine, phenylalanine, and tyrosine, which increased significantly in the case of Zn–Al LDH NPs by 66%, 127%, 101%, and 66%, respectively. On the other hand, treatment with Mg–Al LDH NPs resulted in significant increases in the amount of aspartate and tyrosine by 151% and 60%, respectively.
 |
| Fig. 3 The changes in amino acid contents in geranium shoots treated with Zn–Al LDH and Mg–Al LDH NPs. Vertical bars represented standard error (±SE). Different letters denoting significant changes between treatments are displayed above the bars (P ≤ 0.05). | |
3.7. Proline metabolism
In this study, we examined the metabolites and important enzymes involved in the production of proline in geranium shoots via the glutamate and ornithine pathways (Fig. 4 and 5). The data showed that Mg–Al LDH NP increased the proline level by 170%, which correlated with the upregulation of both the ornithine (Fig. 5) and glutamine (Fig. 4) pathways. When considering the ornithine pathway, comparing Mg–Al LDH NP to the control, there was not a significant difference in the levels of arginine and ornithine (Fig. 5). This finding was consistent with the upregulation of arginase by 276% and OAT by 99%. As for the glutamate pathway, Mg–Al LDH NP had a non-significant effect on α-ketoglutarate, glutamine, and glutamate. These results were associated with the activation of GOGAT (89%), GS (89%), P5CS (94%), and P5CR (121%) in contrast to the control group (Fig. 4).
 |
| Fig. 4 The changes in glutamate pathway, a part of proline metabolism, in geranium shoots treated with Zn–Al LDH and Mg–Al LDH NPs. Vertical bars represented standard error (±SE). Different letters denoting significant changes between treatments are displayed above the bars (P ≤ 0.05). | |
 |
| Fig. 5 The changes in amino acids and enzyme activities involved in the ornithine pathway, a part of proline metabolism, in geranium plant shoots treated with Zn–Al LDH and Mg–Al LDH NPs. OAT is ornithine aminotransferase. Vertical bars represented standard error (±SE). Different letters denoting significant changes between treatments are displayed above the bars (P ≤ 0.05). | |
3.8. Secondary metabolites
3.8.1 Tocopherols. In this study, we analyzed the secondary metabolites such as alpha-tocopherol, beta-tocopherol, gamma-tocopherol, and total tocopherol in geranium shoots (Fig. 6). The data indicated that Zn–Al LDH NP increased alpha-tocopherol by 7%, beta-tocopherol by 33%, gamma-tocopherol by 140%, and total tocopherol by 25%. However, there is no significant increase in alpha-tocopherol. Mg–Al LDH NPs increased alpha-tocopherol by 32%, beta-tocopherol by 53%, gamma-tocopherol by 112%, and total tocopherol by 45% compared to the control.
 |
| Fig. 6 The changes in tocopherol levels (alpha-tocopherol, beta-tocopherol, gamma-tocopherol, and total tocopherol) in geranium plant shoots treated with Zn–Al LDH and Mg–Al LDH NPs. Vertical bars represented standard error (±SE). Different letters denoting significant changes between treatments are displayed above the bars (P ≤ 0.05). | |
3.8.2 Phenolic content. In this investigation, the phenolic content of geranium shoots was analyzed Fig. 7. The data indicated that Zn–Al LDH NP increased rosmarinic acid by 990% compared to the control. Additionally, Mg–Al LDH NPs increased gallic acid by 103%, p-coumaric acid by 427%, and chicoric acid by 69% in contrast to the control group.
 |
| Fig. 7 The changes in phenolics content in geranium plant shoots treated with Zn–Al LDH and Mg–Al LDH NPs. Vertical bars represented standard error (±SE). Different letters denoting significant changes between treatments are displayed above the bars (P ≤ 0.05). | |
3.8.3 Flavonoid content. In this investigation, the flavonoid content of geranium shoots was examined Fig. 8. The data indicated that the content of flavonoids is not significantly affected by Zn–Al LDH or Mg–Al LDH NPs, except in the case of Mg–Al LDH NP causing a 134% increase in kaempferol content compared to the control.
 |
| Fig. 8 The changes in flavonoid content in geranium plant shoots treated with Zn–Al LDH and Mg–Al LDH NPs. Vertical bars represented standard error (±SE). Different letters denoting significant changes between treatments are displayed above the bars (P ≤ 0.05). | |
3.9. Principal component analysis (PCA)
To test the specific responses of shoots of geranium plant shoots plants to Zn–Al LDH and Mg–Al LDH NPs, we performed PCA with growth, minerals, total chlorophyll, primary and secondary metabolites, and their related metabolic enzymes activate data set. The PCA embodied uniform growth, metabolites, and enzyme parameters along with PC1 and PC2 which declared 54% and 21% of the data variability, respectively (Fig. 9). PC1 separated the measured parameters based on LDH NP treatment (control vs. Zn–Al LDH and Mg–Al LDH NPs), whereas the Zn–Al LDH vs. Mg–Al LDH NPs specific responses were separated along PC2. For LDH NP treatment, PC1 showed that these LDH NPs induced upregulation in all measured parameters. PC2 showed that Mg–Al LDH NPs increased amino acid accumulation, however, major fatty acids and organic acids were more responsive to Zn–Al LDH NP treatment.
 |
| Fig. 9 Principal component analysis (PCA) of parameters involved in growth, minerals, total chlorophyll, primary and secondary metabolites, and their related metabolic enzymes activates of geranium plant shoots treated with Zn–Al LDH and Mg–Al LDH NPs. | |
4. Discussion
4.1. Zn and Mg LDH NPs improved the biomass accumulation in geranium plants
Fertilizers are chemical chemicals used in plant cultivation to increase yield and productivity. Nano fertilizers are fertilizers made from nano-sized materials that supply one or more types of nutrients needed for the cultivation of plants.53 Nano fertilizers have several advantages, such as improving nutrient uptake efficiency, increasing plant assimilation, and decreasing the negative environmental effects of conventional chemical fertilizers.54,55 Mg and Zn fertilizers positively induce cell division and mineral absorption, resulting in increased plant growth.18,56 The current study was done to evaluate the role of Zn–Al LDH and Mg–Al LDH NPs in growth and metabolic profiles in geranium plants. The growth enhancement of plants due to MgO-NP treatment can be attributed to various mechanisms, including improved nutrient availability, enhanced nutrient uptake, better water absorption, and increased antioxidant activity.57 The impact of both LDH NPs on Zn and Mg accumulation was investigated by measuring the Zn and Mg content in the shoots of geranium seedlings (Table 1). This increase in Zn and Mg accumulation was linked to a significant rise in the biomass of treated geranium plants. Similarly, it was observed that Mg and Zn nutrients enhance growth parameters in mung bean plants.56 Thus, our findings highlight the vital role LDH NPs play in enhancing photosynthesis.
4.2. Zn and Mg LDH NPs induced bioactive primary metabolites accumulation in geranium plants
NPs can improve the metabolism of carbon and nitrogen, which is connected to stimulated photosynthesis and enhances the growth and development of plants.58 The metabolism of nitrogen and carbon are closely related activities.25 Our study shows that utilizing Zn–Al LDH and Mg–Al LDH NPs increased photosynthetic pigments (Table 1). Numerous studies found similar improvements in photosynthetic pigments. For example, spraying foliar ZnO NPs on coffee plants improved photosynthesis and biomass growth.59 NPs boosted the activity of major photosynthetic enzymes such as Rubisco, PEP carboxylase, and carbonic anhydrase.60 This increase in enzyme activity, along with increased chlorophyll content, resulted in higher photosynthetic rates.61 Furthermore, NPs had a dual effect, lowering ROS generation while speeding up the electron transport chain in chloroplasts; these pathways lead to increased CO2 fixation and photosynthetic performance.62
A C-skeleton and an energy supply are provided by carbohydrate biosynthesis during photosynthesis for several plant functions, such as N absorption and inorganic N fixing.63 It's important to note that C and N assimilate as sugars and amino acids serve as osmotic regulators and energy sources.64 Because LDH NPs enhanced photosynthesis, more sugar was metabolized by enzymes such as amylase, starch synthase, and invertase activity (Fig. 1). The observed rise in starch level and non-significant increase in soluble sugar content could be the result of altered carbohydrate metabolism65 and elevated enzyme activity that breaks down sucrose,66 which was very consistent with our data (Fig. 1). Soluble sugars like glucose and sucrose are crucial organic compounds in higher plants, providing energy for various physiological functions.67 As a result, sugar consumption increased due to the dark respiration processes, which accelerated the accumulation of organic acids. This also provided a route for the creation of amino and fatty acids.68 Organic acids are crucial for plant growth and health as they enhance nutrient uptake and availability.69 They play a key role in metabolic pathways, such as the tricarboxylic acid (TCA) cycle, which is essential for energy production and carbon metabolism.70 Organic acids serve as the building blocks of plant metabolism.71 Plants have a vast array of metabolites, comprising organic acids like citric, malic, succinic, fumaric, and oxalic acids, that serve vital roles in plant metabolism and are engaged in critical processes such as the Krebs cycle.72 Malic acid is also involved in respiration and photosynthesis.73 TCA organic acids are regarded as the connection between carbon and nitrogen metabolism. They are formed by the respiratory breakdown of carbohydrates and serve as the carbon skeleton needed for nitrogen assimilation and amino acid synthesis.74 In line with our results, LDH NPs had non-significant effects on the levels of TCA acids levels (Fig. 2). This is related to the consumption of organic acids to synthesize amino acids. In the instance of Zn–Al, LDH NP had considerable effects on amino acid levels like tyrosine, phenylalanine, valine, and glycine (Fig. 3).
Essential amino acids have critical roles in increasing plant bioactivity by participating in many metabolic pathways that promote growth, stress tolerance, and overall health.75 Amino acids are essential not only as protein building blocks but also as precursors for bioactive compounds that regulate physiological and biochemical processes. Glycine and serine play crucial roles in maintaining photorespiratory fluxes and providing carbon and nitrogen for metabolism in response to environmental changes in nature.76 Glutamate, glutamine, aspartate, and asparagine are the principal products of nitrogen absorption, therefore pools are enormous, particularly in light.77,78 Amino acids like phenylalanine and tyrosine are precursors for flavonoid molecules, which play an important role in plant defense mechanisms.79 Tocopherols, also known as vitamin E, are plant-derived natural products derived from tyrosine.80 In addition to the accumulation of the bioactive and nitrative essential amino acids, proline is an amino acid known for its conformational rigidity and is vital for primary metabolism.27 In many plants, proline accumulation supports the plant's defense mechanisms.81 Another proposed function of proline is the storage and transfer of energy.26–28 We observed an increase in proline in the instance of Mg–Al LDH NP-treated plants. This matched with the activation of GOGAT, GS, P5CS, and P5CR compared to the control.44 Proline is synthesized using the glutamate and ornithine routes.82 Our data demonstrate that in geranium, both the glutamate and ornithine pathways (P5CS, P5CR, and OAT) are equally active under LDH NPs (Fig. 4 and 5). Previous studies have shown that ZnO-NPs lead to increased proline accumulation in tomato plants83 and SiO2-NPs have a similar effect on squash.84
4.3. Zn and Mg LDH NPs improved the redox status of geranium plants
Increased amounts of primary metabolites, such as sugars and amino acids can serve as precursors for secondary metabolite synthesis. In this context, induced primary metabolism can influence regulatory mechanisms that control secondary metabolic pathways, further enhancing their production.85 In line with our results, there was a rise in antioxidants, like tocopherols, phenolic, and flavonoid levels (Fig. 6–8). These metabolites are typically present in low concentrations and play a crucial role in all living organisms.31 Recently, there has been an increasing interest in these compounds due to their practical applications in nutrition, medicine, and cosmetics, as well as their undeniable role in plant stress physiology.31 These compounds, such as phenolic compounds, flavonoids, ascorbic acid, and vitamin E, help in scavenging harmful free radicals.86 This suggests that the treated plants could reduce membrane lipid peroxidation by scavenging reactive oxygen species (ROS) and thus maintaining cellular structure.87
Plants contain α-tocopherol, a key vitamin E component required for medical uses and human nourishment.88 Tocopherols are powerful lipid-soluble antioxidants that protect cellular membranes from oxidative damage by scavenging lipid peroxyl radicals.89 Tocopherols protect the thylakoid membranes in chloroplasts from oxidative damage, thus preserving the photosynthetic machinery. For instance, alpha-tocopherol is found in high concentrations in chloroplasts, where it helps protect photosystem II (PSII) from oxidative stress caused by excess light.90 Phenolic compounds and flavonoids act as antioxidants and have nutritional and medicinal properties. Bioactive compounds like phenolic acids are nutritional elements that are beneficial to human health and are found in small quantities in foods.91 Mg–Al LDH NPs increase the level of gallic acid, p-coumaric, and chicoric acid. Gallic acid has medicinal properties such as anti-fungal activity.92 P-coumaric is a good antioxidant and also exhibits strong antimicrobial properties.93 Chicoric acid has been shown to have potential antioxidant, anti-inflammatory, antiviral, and immune-stimulating properties.94 Zn–Al LDH NPs increase the levels of rosmarinic acid (Fig. 7). Similarly, lower concentrations of silver nanoparticles (SNPs) increased phenolic compounds, such as rosmarinic acid, in sage plants.95 Flavonoids, an important class of natural antioxidants, play a significant role in removing free radicals96 and have medicinal properties as antiviral, anti-inflammatory, anti-cancer, and anti-diabetic.97 Our results indicate a positive effect of Mg–Al LDH NPs on kaempferol (Fig. 8). Similarly, other findings suggest that the application of ZnO or Fe3O4 NPs causes the highest concentration of flavonoids, specifically kaempferol, in potatoes.98 TiO2 and SiO2 NPs led to increased production of α-tocopherol in argan.88 Stevia callus tissue treated with 100 mg L−1 of ZnO NPs showed the highest levels of total flavonoid content, total phenolic content, and total antioxidant capacity.99 NPs of CuO and ZnO increased the content of phenolic compounds in licorice seedlings.100 MgO and Fe2O3 NPs had a significant effect on flavonoid levels in roselle.101 Nigella arvensis treated with TiO2, Al2O3, and NiO NPs led to increased production of total flavonoids.102
5. Conclusion
Based on the findings of this study, we can conclude that both LDH NPs significantly enhanced the growth and photosynthetic pigment and metabolic profiles in geranium plants.
Data availability
All data are presented in this article, and raw data is available upon request from the corresponding author.
Conflicts of interest
All authors declare that they have no competing interests.
Acknowledgements
The authors extend their appreciation to the researchers supporting project number (RSPD2024R931) at King Saud University, Riyadh, Saud Arabia.
References
- Y. Khan, H. Sadia, S. Zeeshan, A. Shah, M. N. Khan, A. A. Shah, N. Ullah, M. F. Ullah, H. Bibi, O. T. Bafakeeh, N. Ben Khedher, S. M. Eldin, B. M. Fadhl and M. I. Khan, Catalysts, 2022, 12, 1386 CrossRef CAS
. - L. R. Khot, S. Sankaran, J. M. Maja, R. Ehsani and E. W. Schuster, Crop Prot., 2012, 35, 64–70 CrossRef CAS
. - A. Singha Roy, S. Kesavan Pillai and S. S. Ray, ACS Omega, 2022, 7, 20428–20440 CrossRef CAS PubMed
. - A. Geremew, L. Carson, S. Woldesenbet, H. Wang, S. Reeves, N. Brooks, P. Saganti, A. Weerasooriya and E. Peace, Front. Plant Sci., 2023, 14, 1–16 Search PubMed
. - C.-H. Lin, H.-L. Chu, W.-S. Hwang, M.-C. Wang and H.-H. Ko, AIP Adv., 2017, 7(12), 125005 CrossRef
. - X. Li, L. Wang, B. Chen, Y. Xu, H. Wang, F. Jin, Z. Shen and D. Hou, Appl. Clay Sci., 2024, 249, 107262 CrossRef CAS
. - A. Singha Roy, S. Kesavan Pillai and S. S. Ray, ACS Omega, 2023, 8, 8427–8440 CrossRef CAS PubMed
. - V. K. Ameena Shirin, R. Sankar, A. P. Johnson, H. V. Gangadharappa and K. Pramod, J. Controlled Release, 2021, 330, 398–426 CrossRef CAS PubMed
. - R. Salinas-Jiménez, G. Vera, M. Tobar, J. Moscote, G. Acha, A. Herrera-Vásquez, D. Rojas-Rivera, E. A. Vidal, A. M. Almeida and M. Ahumada, Environ. Sci.: Nano, 2024, 11, 2249–2261 RSC
. - M. Hamzah Saleem, K. Usman, M. Rizwan, H. Al Jabri and M. Alsafran, Front. Plant Sci., 2022, 13, 1033092 CrossRef PubMed
. - M. U. Hassan, M. Aamer, M. U. Chattha, T. Haiying, B. Shahzad, L. Barbanti, M. Nawaz, A. Rasheed, A. Afzal and Y. Liu, Agriculture, 2020, 10(9), 396 CrossRef CAS
. - A. Singha Roy, S. Kesavan Pillai and S. S. Ray, ACS Omega, 2022, 7, 20428–20440 CrossRef CAS PubMed
. - J. Kameliya, A. Verma, P. Dutta, C. Arora, S. Vyas and R. S. Varma, Inorganics, 2023, 11(3), 121 CrossRef CAS
. - J. Hong, C. Wang, D. C. Wagner, J. L. Gardea-Torresdey, F. He and C. M. Rico, Environ. Sci.: Nano, 2021, 8, 1196–1210 RSC
. - A. Babajani, A. Iranbakhsh, Z. Oraghi Ardebili and B. Eslami, Environ. Sci. Pollut. Res., 2019, 26, 24430–24444 CrossRef CAS PubMed
. - M. Faizan, J. A. Bhat, C. Chen, M. N. Alyemeni, L. Wijaya, P. Ahmad and F. Yu, Plant Physiol. Biochem., 2021, 161, 122–130 CrossRef CAS PubMed
. - N. Pandey, Plant Nutrients and Abiotic Stress Tolerance, 2018, pp. 51–93 Search PubMed
. - A. Abbasifar, F. Shahrabadi and B. ValizadehKaji, J. Plant Nutr., 2020, 43, 1104–1118 CrossRef CAS
. - P. Venkatachalam, N. Priyanka, K. Manikandan, I. Ganeshbabu, P. Indiraarulselvi, N. Geetha, K. Muralikrishna, R. C. Bhattacharya, M. Tiwari and N. Sharma, Plant Physiol. Biochem., 2017, 110, 118–127 CrossRef CAS PubMed
. - A. Hussain, S. Ali, M. Rizwan, M. Z. ur Rehman, M. R. Javed, M. Imran, S. A. S. Chatha and R. Nazir, Environ. Pollut., 2018, 242, 1518–1526 CrossRef CAS PubMed
. - A. Srivastav, D. Ganjewala, R. K. Singhal, V. D. Rajput, T. Minkina, M. Voloshina, S. Srivastava and M. Shrivastava, Plants, 2021, 10, 2556 CrossRef CAS PubMed
. - D. Kanjana, J. Plant Nutr., 2020, 43, 3035–3049 CrossRef CAS
. - L. Cai, M. Liu, Z. Liu, H. Yang, X. Sun, J. Chen, S. Xiang and W. Ding, Molecules, 2018, 23, 3375 CrossRef PubMed
. - H. AbdElgawad, V. Avramova, G. Baggerman, G. Van Raemdonck, D. Valkenborg, X. Van Ostade, Y. Guisez, E. Prinsen, H. Asard and W. Van den Ende, Plant, Cell Environ., 2020, 43, 2254–2271 CrossRef CAS PubMed
. - H. AbdElgawad, Y. M. Hassan, M. O. Alotaibi, A. E. Mohammed and A. M. Saleh, Sci. Total Environ., 2020, 749, 142356 CrossRef CAS PubMed
. - E. Ábrahám, G. Rigó, G. Székely, R. Nagy, C. Koncz and L. Szabados, Plant Mol. Biol., 2003, 51, 363–372 CrossRef PubMed
. - L. Szabados and A. Savouré, Trends Plant Sci., 2010, 15, 89–97 CrossRef CAS PubMed
. - P. E. Verslues and S. Sharma, Arabidopsis Book/American Society of Plant Biologists, 2010 Search PubMed
. - A. El Moukhtari, C. Cabassa-Hourton, M. Farissi and A. Savouré, Front. Plant Sci., 2020, 11, 553924 Search PubMed
. - S. de J. Rivero-Montejo, M. Vargas-Hernandez and I. Torres-Pacheco, Agriculture, 2021, 11, 134 CrossRef CAS
. - A. Bartwal, R. Mall, P. Lohani, S. K. Guru and S. Arora, J. Plant Growth Regul., 2013, 32, 216–232 CrossRef CAS
. - K. M. Bester, W. W. Focke and F. J. W. J. Labuschagné, AIP Conf. Proc., 2019, 2055, 050009 CrossRef
. - F. Mohamed, N. Bhnsawy and M. Shaban, Sci. Rep., 2021, 11, 1–14 CrossRef PubMed
. - F. Mohamed, M. R. Abukhadra and M. Shaban, Sci. Total Environ., 2018, 640–641, 352–363 CrossRef CAS PubMed
. - J. Xie, K. Lee, H. Park, H. Jung and J.-M. Oh, Nanomaterials, 2023, 13, 567 CrossRef CAS PubMed
. - H. K. Lichtenthaler, C. Buschmann and M. Knapp, Photosynthetica, 2005, 43, 379–393 CrossRef CAS
. - A. R. M. Albakaa, D. S. M. Ameen, N. K. Abed, Z. A. Jabbar and L. A. Musaa, J. Phys.: Conf. Ser., 2021, 1853(1), 012018 CrossRef CAS
. - S. Al Jaouni, A. M. Saleh, M. A. M. Wadaan, W. N. Hozzein, S. Selim and H. AbdElgawad, J. Plant Physiol., 2018, 224, 121–131 CrossRef PubMed
. - P. S. Kerr, T. W. Rufty Jr. and S. C. Huber, Plant Physiol., 1985, 77, 275–280 CrossRef CAS PubMed
. - A. Nishi, Y. Nakamura, N. Tanaka and H. Satoh, Plant Physiol., 2001, 127, 459–472 CrossRef CAS PubMed
. - M. Madany and R. Khalil, Egypt. J. Exp. Biol., 2017, 13, 119–133 Search PubMed
. - M. S. M. Mohamed, H. M. Mostafa, S. H. Mohamed, S. I. Abd El-Moez and Z. Kamel, Microb. Drug Resist., 2020, 26, 1410–1420 CrossRef CAS PubMed
. - A. K. Sinha, T. Giblen, H. AbdElgawad, M. De Rop, H. Asard, R. Blust and G. De Boeck, Aquat. Toxicol., 2013, 130, 86–96 CrossRef PubMed
. - H. Abdelgawad, D. De Vos, G. Zinta, M. A. Domagalska, G. T. S. Beemster and H. Asard, New Phytol., 2015, 208, 354–369 CrossRef CAS PubMed
. - O. H. Lowry, N. J. Rosebrough, A. L. Farr and R. J. Randall, J. Biol. Chem., 1951, 193, 265–275 CrossRef CAS PubMed
. - S. Lutts, V. Majerus and J. Kinet, Physiol. Plant., 1999, 105, 450–458 CrossRef CAS
. - G. Forlani, M. Bertazzini, M. Zarattini and D. Funck, Front. Plant Sci., 2015, 6, 150038 Search PubMed
. - S. A. Robinson, A. P. Slade, G. G. Fox, R. Phillips, R. G. Ratcliffe and G. R. Stewart, Plant Physiol., 1991, 95, 509–516 CrossRef CAS PubMed
. - S. J. Temple, S. Kunjibettu, D. Roche and C. Sengupta-Gopalan, Plant Physiol., 1996, 112, 1723–1733 CrossRef CAS PubMed
. - C. Charest and C. Ton Phan, Physiol. Plant., 1990, 80, 159–168 CrossRef CAS
. - H. Chen, B. C. McCaig, M. Melotto, S. Y. He and G. A. Howe, J. Biol. Chem., 2004, 279, 45998–46007 CrossRef CAS PubMed
. - N. H. Gomaa and H. R. AbdElgawad, Span. J. Agric. Res., 2012, 10, 492–501 CrossRef
. - C. Dissanayake and R. Chandrajith, J. Natl. Sci. Found. Sri Lanka, 2009, 37, 153–165 CrossRef CAS
. - J. S. Duhan, R. Kumar, N. Kumar, P. Kaur, K. Nehra and S. Duhan, Biotechnol. Rep., 2017, 15, 11–23 CrossRef PubMed
. - R. Liu and R. Lal, Sci. Total Environ., 2015, 514, 131–139 CrossRef CAS PubMed
. - A. T. Thalooth, M. M. Tawfik and H. M. Mohamed, World J. Agric. Sci., 2006, 2, 37–46 Search PubMed
. - M. A.-A. Amin, A. M. Abu-Elsaoud, A. I. Nowwar, A. T. Abdelwahab, M. A. Awad, S. E.-D. Hassan, F. Boufahja, A. Fouda and A. Elkelish, Green Process. Synth., 2024, 13, 20230215 CrossRef
. - S. S. Mukhopadhyay and N. Kaur, Plant Nanotechnology: Principles and Practices, 2016, pp. 329–348 Search PubMed
. - L. Rossi, L. N. Fedenia, H. Sharifan, X. Ma and L. Lombardini, Plant Physiol. Biochem., 2019, 135, 160–166 CrossRef CAS PubMed
. - A. Jahan, M. M. A. Khan, B. Ahmad, K. B. M. Ahmed, Y. Sadiq and M. Gulfishan, Braz. J. Bot., 2023, 46, 337–349 CrossRef
. - S. Kataria, M. Jain, A. Rastogi, M. Živčák, M. Brestic, S. Liu and D. K. Tripathi,
in Nanomaterials in Plants, Algae and Microorganisms, Elsevier, 2019, pp. 103–127 Search PubMed
. - T. C. Thounaojam, T. T. Meetei, Y. B. Devi, S. K. Panda and H. Upadhyaya, Acta Physiol. Plant., 2021, 43, 1–21 CrossRef
. - M. D. Bolton, Mol. Plant-Microbe Interact., 2009, 22, 487–497 CrossRef CAS PubMed
. - I. I. Shabbaj, H. AbdElgawad, A. Tammar, W. A. Alsiary and M. M. Y. Madany, Plant Physiol. Biochem., 2021, 166, 1131–1141 CrossRef CAS PubMed
. - M. H. Rabie, M. E. Eleiwa, M. A. Aboseoud and K. M. Khalil, J. King Abdulaziz Univ., Sci., 1992, 4(1), 37–43 CrossRef
. - R. Manivasagaperumal, S. Balamurugan, G. Thiyagarajan and J. Sekar, Curr. Bot., 2011, 2, 11–15 CAS
. 2011•core.ac.uk, https://core.ac.uk/. - R. N. Tharanathan, G. Muralikrishna, P. V. Salimath and M. R. R. Rao, Proc. Plant Sci., 1987, 97, 81–155 CrossRef CAS
. - H. AbdElgawad, A. E. Mohammed, J. R. van Dijk, G. T. S. Beemster, M. O. Alotaibi and A. M. Saleh, Front. Plant Sci., 2023, 14, 1–14 Search PubMed
. - D. L. Jones, Plant Soil, 1998, 205, 25–44 CrossRef CAS
. - W. C. Plaxton and F. E. Podestá, CRC Crit. Rev. Plant Sci., 2006, 25, 159–198 CrossRef CAS
. - E. Hadavi and N. Ghazijahani, in Biostimulants: Exploring Sources and Applications, Springer, 2022, pp. 71–105 Search PubMed
. - T. A. Bennet-Clark, New Phytol., 1933, 37–71 CrossRef CAS
. - C. Pereira, L. Barros, A. M. Carvalho and I. C. F. R. Ferreira, Food Anal. Methods, 2013, 6, 1337–1344 CrossRef
. - M. Lancien, P. Gadal and M. Hodges, Plant Physiol., 2000, 123, 817–824 CrossRef CAS PubMed
. - T. M. Hildebrandt, A. N. Nesi, W. L. Araújo and H.-P. Braun, Mol. Plant, 2015, 8, 1563–1579 CrossRef CAS PubMed
. - X. Fu, L. M. Gregory, S. E. Weise and B. J. Walker, Nat. Plants, 2023, 9, 169–178 CrossRef CAS PubMed
. - G. M. Coruzzi, Arabidopsis Book/American Society of Plant Biologists, 2003 Search PubMed
. - H.-M. Lam, K. Coschigano, C. Schultz, R. Melo-Oliveira, G. Tjaden, I. Oliveira, N. Ngai, M.-H. Hsieh and G. Coruzzi, Plant Cell, 1995, 7, 887 CAS
. - B. Heinemann and T. M. Hildebrandt, J. Exp. Bot., 2021, 72, 4634–4645 CrossRef CAS PubMed
. - C. A. Schenck and H. A. Maeda, Phytochemistry, 2018, 149, 82–102 CrossRef CAS PubMed
. - M. Senthil-kumar and K. S. Mysore, Plant, Cell Environ., 2012, 35, 1329–1343 CrossRef CAS PubMed
. - M. M. F. Mansour and K. H. A. Salama, Plant Ecophysiology and Adaptation under Climate Change: Mechanisms and Perspectives II: Mechanisms of Adaptation and Stress Amelioration, 2020, pp. 357–397 Search PubMed
. - M. Faizan, A. Faraz, M. Yusuf, S. T. Khan and S. Hayat, Photosynthetica, 2018, 56, 678–686 CrossRef CAS
. - M. H. Siddiqui, M. H. Al-Whaibi, M. Faisal and A. A. Al Sahli, Environ. Toxicol. Chem., 2014, 33, 2429–2437 CrossRef CAS PubMed
. - S. W. Drew and A. L. Demain, Annu. Rev. Microbiol., 1977, 31, 343–356 CrossRef CAS PubMed
. - D. M. Kasote, S. S. Katyare, M. V. Hegde and H. Bae, Int. J. Biol. Sci., 2015, 11, 982–991 CrossRef CAS PubMed
. - I. I. Shabbaj, M. M. Y. Madany, A. Tammar, M. A. Balkhyour and H. AbdElgawad, Environ. Sci.: Nano, 2021, 8, 1960–1977 RSC
. - G. A. Hegazi, W. M. Ibrahim, M. H. Hendawy, H. M. Salem and H. E. Ghareb, Plant Arch., 2020, 20, 2431–2437 Search PubMed
. - E. Niki, Free Radical Biol. Med., 2014, 66, 3–12 CrossRef CAS PubMed
. - S. Munné-Bosch and L. Alegre, CRC Crit. Rev. Plant Sci., 2002, 21, 31–57 CrossRef
. - H. Boz, Int. J. Food Sci. Technol., 2015, 50, 2323–2328 CrossRef CAS
. - A. El-Nagar, A. A. Elzaawely, N. A. Taha and Y. Nehela, Agronomy, 2020, 10, 1402 CrossRef CAS
. - P. S. Ferreira, F. D. Victorelli, B. Fonseca-Santos and M. Chorilli, Crit. Rev. Anal. Chem., 2019, 49, 21–31 CrossRef CAS PubMed
. - J. Lee and C. F. Scagel, Food Chem., 2009, 115, 650–656 CrossRef CAS
. - S. H. Moazzami Farida, R. Karamian and B. R. Albrectsen, Physiol. Plant., 2020, 170, 415–432 CrossRef CAS PubMed
. - M. Modarresi, A. Chahardoli, N. Karimi and S. Chahardoli, Heliyon, 2020, 6(6), e04265 CrossRef PubMed
. - T. Wang, Q. Li and K. Bi, Asian J. Pharm. Sci., 2018, 13, 12–23 CrossRef PubMed
. - A. R. Sallam, A. A. Mahdi and K. Y. Farroh, Plant Cell Biotechnol. Mol. Biol., 2022, 23, 1–16 Search PubMed
. - R. Javed, B. Yucesan, M. Zia and E. Gurel, Sugar Technol., 2018, 20, 194–201 CrossRef CAS
. - H. Oloumi, R. Soltaninejad and A. Baghizadeh, Indian J. Plant Physiol., 2015, 20, 157–161 CrossRef
. - H. Kiapour, P. Moaveni, B. Sani, F. Rajabzadeh and H. Mozafari, J. Med. Plants By-Prod., 2020, 9, 19–31 Search PubMed
. - M. Modarresi, A. Chahardoli, N. Karimi and S. Chahardoli, Heliyon, 2020, 6, e04265 CrossRef PubMed
.
|
This journal is © The Royal Society of Chemistry 2024 |
Click here to see how this site uses Cookies. View our privacy policy here.