DOI:
10.1039/D4RA03606A
(Paper)
RSC Adv., 2024,
14, 23505-23510
Polyfluorene–poly(ethylene oxide) diblock copolymers: synthesis and electron transport behavior†
Received
17th May 2024
, Accepted 8th July 2024
First published on 26th July 2024
Abstract
Under mild reaction conditions, we synthesized diblock copolymers of poly(9,9-dioctylfluorene)-block-poly(ethylene oxide) (PFO-b-PEO) via end-capping poly(9,9-dioctylfluorene) (PFO) with poly(ethylene oxide) (PEO) on one end. We investigated the thermal, optical, electrochemical and crystalline properties as well as electron transport performance of these polymers. Our results demonstrate that PFO-b-PEO diblock copolymers with short PEO chains (Mn = 1000 and 2000 g mol−1) exhibit higher electron mobilities compared to the PFO homopolymer and longer PEO chain (Mn = 4000 g mol−1) attached copolymers. This enhanced electron mobility is attributed to the higher crystallinity induced by the shorter PEO chain end-capping.
Introduction
As one of the promising polymers for light-emitting diode materials, polyfluorene exhibits superior processability, high photoluminescence quantum efficiency, and satisfactory stabilities in both thermal and electrochemical detection.1,2 However, it expresses insufficient color stability, attributed to aggregates/excimers, interchain inter-actions, and/or emissive keto defects generated by troublesome thermo-, photo-, or electro-oxidative degradation during device operation.3–8 Moreover, another severe problem for polyfluorene is poor electro-luminescence (EL) efficiency associated with electron traps, which induces hole–electron transporting imbalance and Shockley–Read–Hall (SRH) trap-assisted recombination between trapped electrons and free holes.9,10
To suppress these adverse effects, decoration of the polyfluorene backbone, such as an alkyl/phenyl structure introduced at methylene bridged hydrogen,11,12 functional groups attached as endcaps,13–17 and elimination of adverse impact from electron traps via dilution of electron trap or the formation of more regular morphology or phase.18–21
Poly(9,9-dioctylfluorene) (PFO), as a polyfluorene derivative, with octyl groups in 9 active positions as side chains, was reported to induce good solubility and β-phase formation.20,22
As either an additive or an attached building block,23–26 poly(ethylene oxide) (PEO) was widely chosen for its superior viscoelastic properties, which are well suited for improving the morphology of conjugated polymers. Previously, we synthesised a diblock copolymer of P3HT by incorporating PEO, which induced micro-phase separation and enhanced charge mobility.27 This technique inspired us to assemble PEO into block copolymer architectures through chain elongation from terminals of the other polymer.
We synthesized PFO and PEO diblock copolymers for a luminescent material in electron-only (EO) devices. PEO segments were introduced at the terminals of PFO via the Steglich coupling reaction. Gel permeation chromatography (GPC) and 1H-NMR were used to characterize the structures of the PFO homo-polymer and block copolymers. The thermal, optical, electrochemical, and crystalline properties of the polymers and the performances of the corresponding EO devices were examined.
Experimental
Materials
We prepared 2-(7-bromo-9,9-dioctyl-fluoren-2-yl)-4,4,5,5-tetramethyl-1,3,2-dioxaborolane (1) and 2-(4-iodophenoxy)-1-[tert-butyldimethylsiloxy]ethane (2) as reported in the literature.28,29 Dry tetrahydrofuran (THF) and other reagents were obtained as commercial products and used without further purification. α-Monocarboxylic-ω-monomethoxy poly(ethylene oxide)s (PEO–COOHs), with number-average molecular weights (Mn) of 1000, 2000, and 4000, were synthesized according to an established procedure.15
Synthesis of hydroxyl end-functionalized polyfluorene (PFO-2)
Compound 1 (1.77 g) and deoxidized toluene (17 mL) were added to a 100 mL flask, and oxygen was removed via three freeze-and-thaw cycles with liquid nitrogen bath. Subsequently, to the mixture, 2 N K2CO3 (30 mL) (after 0.5 h nitrogen bubbling), compound 2 (35.4 mg), and Pd(PPh3)4(0) (37 mg) were added and deoxygenated via another three freeze-and-thaw cycles. The mixture was stirred at 90 °C under nitrogen for 24 h. After the reaction, we added chloroform (100 mL) at room temperature and then washed the mixture with brine thrice. After drying with MgSO4, filtration, concentration, and reprecipitation in methanol, we obtained 0.99 g yellow solid product tert-butyldimethylsiloxyethoxy end-capped PFO (PFO-1).
The prepared PFO-1 (0.99 g) was dissolved in dry THF (50 mL), and then HF/pyridine (0.36 mL) was dropwise added under nitrogen. The mixture was stirred at room temperature for 24 h. Following concentration and reprecipitation in methanol, we obtained 0.80 g of the yellow solid product PFO-2 (yield: 80%).
Synthesis of PFO-b-PEO
PFO-2 (0.5 g, 0.05 mmol), PEO–COOH (0.5 mmol), dicyclohexylcarbodiimide (DCC) (1.78 g, 8.6 mmol), and 4-dimethylaminopyridine (DMAP) (1.06 g, 8.6 mmol) and dry dichloromethane (60 mL) were placed under nitrogen in a 100 mL flask. The mixture was then stirred at room temperature for 24 h. The resulting solution was evaporated to remove the solvent, and the residue was crushed with acetone (60 mL) by stirring overnight. Then, via Soxhlet extraction of the filtrated solid with acetone for 48 h, we produced the final lime solid PFO-b-PEO samples. Characteristics of PFO-2 and PFO-b-PEOs are listed in Table 1.
Table 1 Characteristics of PFO-2 and PFO-b-PEOs
Polymer |
Mn,PEO-sectionb (g mol−1) |
Mn,PFO-b-PEO (g mol−1) |
PDIa |
PEOb (wt%) |
Yields |
Determined via GPC. Determined via 1H-NMR. |
PFO-2 |
— |
9231a |
8697b |
2.22 |
— |
80% |
PFO-b-PEO1 |
975 |
10 552a |
9568b |
2.15 |
3.7 |
73% |
PFO-b-PEO2 |
1914 |
10 966a |
10 009b |
3.10 |
6.4 |
63% |
PFO-b-PEO4 |
3135 |
11 543a |
12 059b |
4.31 |
15.3 |
55% |
Characterizations
1H-NMR spectra were obtained on a JEOL ALPHA300 instrument at 300 MHz and 25 °C. Deuterated chloroform was used as a solvent with tetramethyl silane as an internal standard.
Mn and polydispersity index (PDI) was determined by gel permeation chromatography (GPC) analysis with a JASCO RI-2031 detector and eluted with chloroform at a flow rate of 0.5 mL min−1 at room temperature and calibrated by standard polystyrene samples.
The polymers' glass transition temperature (Tg) was measured by differential scanning calorimetry (DSC) analysis performed on a Rigaku DSC-8230 under a nitrogen atmosphere at heating and cooling rates of 10 °C min−1.
Ultraviolet-visible (UV-vis) absorption spectra were obtained on the JASCO V-570 spectrophotometer, and photoluminescence (PL) spectra were detected on the JASCO FP-6500 spectrophotometer with an excitation at 380 nm. The films were formed in 2.5 cm × 2.5 cm glass plates. The polymer layer was laminated by spin-coating at 1000 rpm for 60 s from chlorobenzene solution (40 mg mL−1) filtered using a 0.45 mm membrane filter. The procedure of film fabrication was almost the same as for EO devices on ITO substrates.
Cyclic voltammetry (CV) was conducted on a Niko Keisoku Model NPGFZ-2501-A Potentiostat/Galvanostat. All measurements were carried out at room temperature in a typical three-electrode cell with a working electrode (glassy carbon electrode), a reference electrode (Ag/AgCl), and a counter electrode (Pt wire) at a scanning rate of 0.1 V s−1. All measurements were performed using acetonitrile and tetrabutylammonium tetra-fluoroborate (Bu4NBF4) (0.1 M) as solvents and supporting electrolytes, respectively.
The crystal structure of the films on the glass was measured by grazing incidence wide-angle X-ray diffraction (GIWAXD) (RIGAKU X-ray Diffractometer SmartLab) (Cu Kα, λ = 1.5418 Å, 45 kV, and 200 mA) from 3° to 30° with a step of 0.02° at the scan speed of 1° min−1 in the out-of-plane measurements. The incident angle was fixed at 0.14°. The thickness of the films and metals was measured using a Stylus-type surface profiler (BRUKER, Dektak XT-S).
Results and discussion
Synthesis and characterization
As shown in Scheme 1, we synthesized tert-butyldimethylsiloxyethoxy end-capped PFO-1 via the Suzuki–Miyaura reaction, and by following deprotection of tert-butyldimethylsiloxyethoxy with HF/pyridine, PFO-2 was produced with low steric hindrance PhOCH2CH2OH terminating group. PFO-2 and PEO–COOH were then linked to form diblock copolymer PFO-b-PEO via a mild Steglich coupling reaction (room temperature, 24 h). The key factor that influences the Steglich coupling reaction for the formation of PFO-b-PEOs is the low steric hindrance of the hydroxyl group on PFO-2 and the carboxyl group on PEO. This low steric hindrance is crucial as it allows the functional groups to react more readily, minimizing spatial barriers and facilitating efficient coupling.
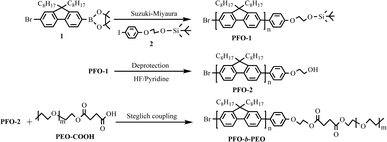 |
| Scheme 1 Synthesis route. | |
By employing different Mn PEO–COOHs, we successfully obtained a series of diblock copolymers with different PEO weight content (shown in Table 1). The PDI of the PFO-b-PEO4 was significantly larger compared to other polymers. This can be attributed to the increased crystallinity of longer PEO chains. As the Mn of the PEO segments increases (e.g., Mn = 4000 g mol−1), their crystallinity is enhanced, leading to stronger interchain interactions and a broader PDI.30
Thermal properties
As depicted in Fig. 1, homopolymer (PFO-2) showed an endothermic peak around 63 °C, attributed mainly to the melting of the conjugated PFO backbone. The curves of diblock copolymers (PFO-b-PEO1 and PFO-b-PEO2) revealed double endothermic peaks near 58 C° and 63 °C, and the newly emerging rise might be related to the melting of the PEO chains. In the curve of PFO-b-PFE4, the peak around 63 °C became not obvious; we attributed it to the large-scale crystallization of longer PEO chains, which might inhibit the crystallization of the conjugated PFO backbone. This fact also echoes the previous discussion on the impact of long-chain PEO on PDI.
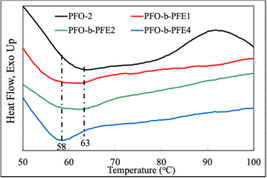 |
| Fig. 1 DSC thermograms for PFO-2 and PFO-b-PEOs. | |
Optical properties
As shown in Fig. 2 and Table 2, the UV-vis spectra of all homo- and diblock copolymers demonstrated similar λmax around 384 nm in both states. In addition, all polymers expressed similar redshift at about 433 nm in Fig. 2b, owing to the β-phase formation in the film state. It is reported that the β-phase formation is related to a series of attractive features in PLED, such as higher carrier mobility, higher current efficiency, and excellent spectral stability.31
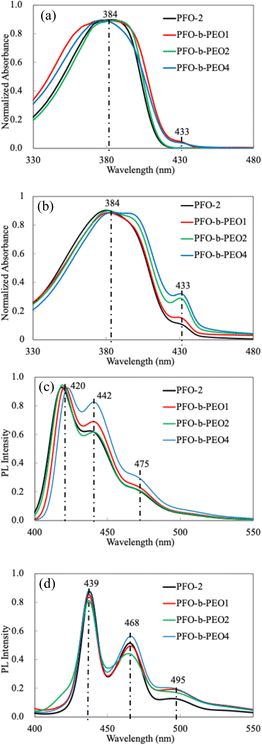 |
| Fig. 2 UV-vis and PL spectra of PFO-2 and PFO-b-PEOs: (a) UV-vis, solution, (b) UV-vis, film, (c) PL, solution, and (d) PL, film. | |
Table 2 Optical and electrochemical properties of PFO-2 and PFO-b-PEOs
Polymer |
λmax (nm), film |
Ered (V) |
ELUMOa (−eV) |
ELUMO = −(Ered + 4.4) (eV). |
PFO-2 |
383, 433 |
−0.74 |
3.66 |
PFO-b-PEO1 |
383, 433 |
−0.74 |
3.66 |
PFO-b-PEO2 |
384, 433 |
−0.73 |
3.67 |
PFO-b-PEO4 |
384, 433 |
−0.74 |
3.66 |
From Fig. 2a and b, we observed an increase in the β-phase composition with higher Mn PEO segments. This phenomenon can be attributed to reduced chain mobility. Longer PEO chains decrease the mobility of polymer chains through entanglements and enhance the intermolecular interactions, thereby promoting the formation of the stable β-phase conformation in PFO chains.32
On the other hand, all polymers exhibited similar PL spectra in both solution and film states. This indicates that incorporating PEO as a block segment exerts minimal impact on the PL properties of PFO. The PL spectra of all the polymers showed a primary emission peak at around 420 nm in the solution and 439 nm in the film, a shoulder peak approximately at 442 nm in the solution and 468 nm in the film, and a long-wavelength emission peak around 475 nm in the solution and 495 nm in film; these peaks correspond to the π–π* transitions within the polyfluorene molecules, the microstructural features of the polyfluorene chains, and additional microstructural characteristics of the polyfluorene chains, respectively.33,34
Electrochemical properties
The electrochemical properties of the polymers were investigated by CV, as shown in Table 2; the reduction process of PFO-2 and PFO-b-PEOs started at about −0.74 V, and LUMO levels of all polymers were calculated to be about −3.66 eV, indicating that the PEO block section has little effect on the LUMO levels of PFO polymer.
Crystalline structure analysis
As shown in Fig. 3, stronger peaks appeared in the diblock copolymers curves at ca. 2θ ≈ 20°, corresponding to π–π stacking distances of 4.2–4.4 Å, exhibiting crystalline patterns with distinct diffraction peaks.35,36 In the case of PFO-2, the rise was relatively weaker, implying a nearly non-crystalline (amorphous) profile. The same result was observed at 2θ ≈ 7.5°, corresponding to lamellar patterned d-spacings.37
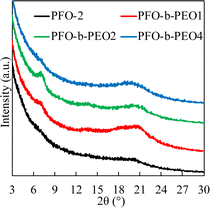 |
| Fig. 3 GIWAXD profiles obtained with out-of-plane geometry for thin films of PFO and PFO-b-PEOs fabricated with chlorobenzene. | |
Furthermore, we found that both peaks at ca. 2θ ≈ 20° and 2θ ≈ 7.5° were stronger in PFO-b-PEO1 and PFO-b-PEO2 than in PFO-b-PFO4, indicating the inhibition of the crystallite formation in the presence of overlong PEO chains, echoing the results of the thermal properties we discussed.
Electron transporting properties
The performance of the EO devices based on PFO-2 homopolymer and PFO-b-PEO block copolymers with the configuration of ITO/Al/polymer/LiF/Al was evaluated. Electron mobility in the space-charge-limited current, the SCLC region was determined from the I–V characteristic curves utilizing the following eqn (1), taking the series resistance (12 Ω) into consideration and assuming that the built-in voltage was close to zero due to the slight difference of work functions for both electrodes.38 |
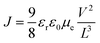 | (1) |
where J is the electron current density, µe is the electron mobility, εr is the relative permittivity of the material (3.5), ε0 is the permittivity of vacuum, L is the thickness of the active layer, and V is the voltage drop across the device.
The electron mobilities of devices fabricated from PFO-2 and PFO-b-PEOs are shown in Fig. 4 and Table 3. PFO-b-PEO1 and PFO-b-PEO2 exhibited higher electron mobility than PFO-2 and PFO-b-PEO4. Since they had similar performances in optical and electrochemical properties, we owed this enhancement to the fact that a short PEO polymer chain block section can improve the crystalline properties of PFO.
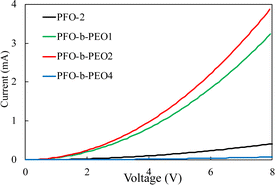 |
| Fig. 4 Current–voltage characteristics of EO devices based on PFO-2 and PFO-b-PEOs. | |
Table 3 Electron mobility(cm2 V−1 s−1) of PFO-2 and PFO-b-PEOs
Polymer |
Electron mobilitya (cm2 V−1 s−1) |
Average values determined for four devices. |
PFO-2 |
4.5 × 10−7 |
PFO-b-PEO1 |
3.3 × 10−6 |
PFO-b-PEO2 |
3.9 × 10−6 |
PFO-b-PEO4 |
1.1 × 10−7 |
Conclusions
In summary, we successfully synthesized PFO-b-PEOs using a mild Steglich coupling reaction. The investigation of these copolymers revealed their potential to enhance the electron transport properties, with particular improvements noted in the materials with shorter PEO chain segments. This work highlights the significant impact of chain length and structural modifications on the properties of PFO-b-PEO copolymers.
Future studies should focus on further exploring the relationship between the molecular architecture of PFO-b-PEO copolymers and their electronic properties. Additionally, there is considerable potential in investigating the application of these materials in various optoelectronic devices, such as organic photovoltaics (OPVs) and field-effect transistors (FETs). Understanding the detailed mechanisms of electron transport and phase behavior in these copolymers could lead to the development of more efficient and stable electronic materials.
Data availability
1H-NMR spectra of PFO-1, PFO-2, PFO-b-PEO1, PFO-b-PEO2, and PFO-b-PEO4 are available in the ESI (https://doi.org/10.1039/d4ra03606a). Fig. S1: 1H-NMR spectra of PFO-1 in CDCl3 at 300 MHz; Fig. S2: 1H-NMR spectra of PFO-2 in CDCl3 at 300 MHz; Fig. S3: 1H-NMR spectra of PFO-b-PEO1 in CDCl3 at 300 MHz; Fig. S4: 1H-NMR spectra of PFO-b-PEO2 in CDCl3 at 300 MHz; Fig. S5: 1H NMR spectra of PFO-b-PEO4 in CDCl3 at 300 MHz.†
Author contributions
Jin Cheng and Kenji Ogino conceived and designed the experiments; Jin Cheng performed the experiments; Jin Cheng, Ruoyu Jiang, and Kenji Ogino analyzed the data; Jin Cheng wrote the main bulk of the article; Yuhua Shan, Hong Sun, and Kenji Ogino improved and finalized the article; Yuhua Shan, Shinji Kanehashi and Kenji Ogino contributed reagents/materials/analysis tools.
Conflicts of interest
The authors declare no conflict of interest.
Acknowledgements
This research was funded by the “Jiangsu Province Key Laboratory of Fine Petrochemical Engineering, grant number KF2023.” and the “Grant-in-Aid for Scientific Research from the Japan Society for the Promotion of Science (JSPS), KAKENHI, grant number (15K05661)”.
Notes and references
- C. A. Young, A. Hammack, H. J. Lee, H. Jia, T. Yu, M. D. Marquez, A. C. Jamison, B. E. Gnade and T. R. Lee, ACS Omega, 2019, 27, 22332 CrossRef PubMed.
- G. Sardar, A. Shukla, E. G. Moore, G. Banappanavar, S. Lo, E. B. Namdas and D. Kabra, J. Phys. Chem. C, 2022, 126, 9069 CrossRef CAS.
- E. J. W. List, R. Guentner, P. S. de Freitas and U. Scherf, Adv. Mater., 2002, 911, 734 Search PubMed.
- L. Romaner, A. Pogantsch, P. S. de Freitas, U. Scherf, M. Gaal, E. Zojer and E. J. W. List, Adv. Funct. Mater., 2003, 13, 597 CrossRef CAS.
- J. K. Jin, S. K. Kwon and Y. H. Kim, Macromolecules, 2009, 42, 6339 CrossRef CAS.
- A. Bolognesi, P. Betti, C. Botta and S. Destri, Macromolecules, 2009, 42, 1107 CrossRef CAS.
- M. Kreyenschmidt, G. Klarner, T. Fuhrer, J. Ashenhurst, S. Karg, W. D. Chen, V. Y. Lee, J. C. Scott and R. D. Miller, Macromolecules, 1998, 31, 1099 CrossRef CAS.
- J. I. Lee, G. Klarner and R. D. Miller, Chem. Mater., 1999, 11, 1083 CrossRef CAS.
- X. Gong, P. K. Iyer, D. Moses, G. C. Bazan, A. J. Heeger and S. S. Xiao, Adv. Funct. Mater., 2003, 13, 325 CrossRef CAS.
- G. Klarner, J. I. Lee, V. Y. Lee, E. Chan, J. P. Chen, A. Nelson, D. Markiewicz, R. Siemens, J. C. Scott and R. D. Miller, Chem. Mater., 1999, 11, 1800 CrossRef.
- Q. S. Zhang, Chin. J. Chem., 2010, 28, 1482 CrossRef CAS.
- S. K. Lee, T. Ahn and N. S. Cho, J. Polym. Sci., Part A: Polym. Chem., 2007, 45, 1199 CrossRef CAS.
- H. Hsieh, C. Hung, K. Watanabe, J. Chen, Y. Chiu, T. Isono, Y. Chiang, R. R. Reghu, T. Satoh and W. Chen, Polym. Chem., 2018, 9, 3820 RSC.
- L. Verheyen, K. Janssens, M. Marinelli, E. Salatelli and G. Koeckelberghs, Macromolecules, 2019, 52, 6578 CrossRef CAS.
- H. H. Ahn, S. H. Jang, B. Hwang, J. Yoon, S. Y. Kim, S. J. Noh, J. Y. Han and Y. K. Kwon, Polymer, 2013, 54, 4864 CrossRef CAS.
- Y. Tan, Z. Gu, K. Tsuchiya and K. Ogino, Polymer, 2012, 53, 1444 CrossRef CAS.
- K. Saito, T. Isono, H. Sun, T. Kakuchi, W. Chen and T. Satoh, Polym. Chem., 2015, 6, 6959 RSC.
- A. Kunz, P. W. M. Bloma and J. J. Michels, J. Mater. Chem. C, 2017, 5, 3042 RSC.
- E. Khodabakhshi, P. W. M. Blom and J. J. Michels, Appl. Phys. Lett., 2019, 114, 093301 CrossRef.
- D. W. Bright, F. B. Dias, F. Galbrecht, U. Scherf and A. P. Monkman, Adv. Funct. Mater., 2009, 19, 67 CrossRef CAS.
- W. Zhu, C. Wei, X. An, J. Weng, B. Liu, N. Wang, N. Sun, L. Sun, M. Yu, J. Lin, L. Bai, C. Sun, D. Lu, L. Xie and W. Huang, ACS Appl. Polym. Mater., 2019, 1, 2352 CrossRef CAS.
- P. Wilhelm, D. Blank, J. M. Lupton and J. Vogelsang, ChemPhysChem, 2020, 21, 1 CrossRef PubMed.
- C. H. Lyons, E. D. Abbas, J.-K. Lee and M. F. Rubner, J. Am. Chem. Soc., 1998, 120, 12100 CrossRef CAS.
- S. Chien, F. Chen, M. Chung and C. Hsu, J. Phys. Chem. C, 2012, 116, 1354 CrossRef CAS.
- Y. Honmou, S. Hirata, H. Komiyama, J. Hiyoshi, S. Kawauchi, T. Iyoda and M. Vacha, Nat. Commun., 2014, 5, 4666 CrossRef CAS PubMed.
- A. B. Schantz, P. O. Saboe, I. T. Sines, H.-Y. Lee, K. J. M. Bishop, J. K. Maranas, P. D. Butler and M. Kumar, Macromolecules, 2017, 50, 2484 CrossRef CAS.
- K. Hayashi, K. Kanehashi and K. Ogino, Structural Analysis of Poly(3‐Hexylthiophene)‐b‐Poly(ethylene Oxide) and Evaluation of Hole Mobility, Autumn Research Conference of the Society of Fiber Science and Technology, Nagano, Japan, 2019 Search PubMed.
- X. Zhang, H. Tian, Q. Liu, L. Wang, Y. Geng and F. Wang, J. Org. Chem., 2006, 71, 4332 CrossRef CAS PubMed.
- B. Malo-Forest, G. Landelle, J. Roy, J. Lacroix, R. C. Gaudreault and J. Paquin, Bioorg. Med. Chem. Lett., 2013, 23, 1712 CrossRef CAS PubMed.
- H. Feng, X. Lu, W. Wang, N.-G. Kang and J. W. Mays, Polymers, 2017, 9, 494 CrossRef PubMed.
- X. Zhang, Q. Hu, J. Lin, Z. Lei, X. Guo, L. Xie, W. Lai and W. Huang, Appl. Phys. Lett., 2013, 103, 153301 CrossRef.
- N. Golzari, J. Adams and S. Beuermann, Polymers, 2017, 9, 306 CrossRef PubMed.
- Q. Zhang, P. Wang, G. L. Ong, S. H. Tan, Z. W. Tan, Y. H. Hii, Y. L. Wong, K. S. Cheah, S. L. Yap, T. S. Ong, T. Y. Tou, C. H. Nee, D. J. Liaw and S. S. Yap, Polymers, 2019, 11, 840 CrossRef CAS PubMed.
- H. T. Kidanu, J. H. Lee and C. Chen, Mater. Adv., 2021, 2, 3589 RSC.
- E. Lim, B. J. Jung, J. Lee, H. K. Shim, J. I. Lee, Y. S. Yang and L. M. Do, Macromolecules, 2005, 38, 4531 CrossRef CAS.
- Y. Wu, Y. Li, S. Gardner and B. S. J. Ong, J. Am. Chem. Soc., 2005, 127, 614 CrossRef CAS PubMed.
- S. Hayashi, S. Inagi and T. Fuchigami, Polym. J., 2010, 42, 772 CrossRef CAS.
- C. R. Singh, G. Gupta, R. Lohwasser, S. Engmann, J. Balko, M. Thelakkat, T. Thurn-Albrecht and H. Hoppe, J. Polym. Sci., Part B: Polym. Phys., 2013, 51, 943 CrossRef CAS.
|
This journal is © The Royal Society of Chemistry 2024 |
Click here to see how this site uses Cookies. View our privacy policy here.