DOI:
10.1039/D4RA03342F
(Paper)
RSC Adv., 2024,
14, 26340-26353
Improving the optical properties of magnesium spinel chromites through Ni and Cu substitutions for optoelectronic applications
Received
6th May 2024
, Accepted 17th July 2024
First published on 22nd August 2024
Abstract
In this study, we investigated the optoelectronic performance of magnesium spinel chromites with nickel (Ni) and copper (Cu) substitutions. Using the sol–gel method, we synthesized two spinel chromites: Mg0.6Ni0.4Cr2O4 (MNCO) and Mg0.6Cu0.4Cr2O4 (MCCO). We extensively characterized these samples to analyze their thermal, structural, elastic, and optical properties. Structural analysis reveals good agreement between the calculated and refined structural parameters, which supports the proposed cation distributions for the samples. By calculating stiffness constants from force constants, we derived elastic moduli such as bulk modulus, longitudinal modulus, and rigidity modulus. MCCO exhibited lower values for these moduli, as well as the Debye temperature, compared to MNCO. Both samples displayed a brittle mechanical nature according to the Pugh ratio, while the Poisson ratio remained constant at 0.25, indicating isotropic elasticity. UV-vis-NIR spectroscopy revealed that MNCO has higher band-gap (Eg) and Urbach (Eu) energies than MCCO. Further analysis of refractive index, penetration depth, extinction coefficients, nonlinear optical parameters, optical conductivity, and optical dielectric constants highlighted the promising optoelectronic applications of the synthesized materials. Our study found that the band-gap energy values of the as-synthesized samples were smaller than reported values for MgCr2O4 spinel chromites, indicating that Ni and Cu substitutions offer an opportunity to extend the sunlight absorption range of magnesium chromites.
1. Introduction
Spinel ACr2O4 chromites (where A represents Fe, Mn, Co, Ni, Cu, and Zn) have garnered considerable attention in research due to their captivating physicochemical properties. These materials have been extensively explored for industrial and technological applications, as evidenced by numerous studies (see ref. 1–3 and references cited therein). Spinel chromites have found utility in a wide range of areas, including solid oxide fuel cells, supercapacitor electrodes, pigments, gas sensors, photocatalysts, non-volatile memory, and magnetic switching. They exhibit great potential as candidates for nano-device fabrication, sensor components, strengthening agents, combustion catalysts, and protective coatings. Moreover, chromite nanoparticles have exhibited promise in areas such as photocatalysis, organic transformation, and superconductivity. Given their diverse applications, spinel ACr2O4 chromites play a pivotal role in advancing various scientific and technological fields.
The sol–gel method is a significant approach employed for the production of pure spinel chromites with precise control over their chemical composition and grain size.1,4 This technique offers numerous advantages, including reduced calcination times, lower processing temperatures, improved material homogeneity, and high purity of the synthesized materials. By adjusting various parameters such as the pH of the initial solution, concentration, calcination time, and calcination temperature, the sol–gel synthesis can further enhance the properties of spinel chromites.4,5
As a member of the spinel chromites, magnesium chromium oxides (MgCr2O4) hold significant importance and offer a wide range of applications due to their exceptional properties (see ref. 6–8 and references therein). MgCr2O4 chromites exhibit outstanding resistance to thermal shock and demonstrate superior catalytic activity. With a high melting point, they ensure thermal stability even under harsh conditions. Additionally, MgCr2O4 spinels showcase remarkable mechanical strength at elevated temperatures and possess humidity-sensing properties. These characteristics make MgCr2O4 highly desirable in the field of refractory materials, where its stability at high temperatures and resistance to slag attack have been extensively explored.
In terms of optoelectronic properties, MgCr2O4 spinel chromites have been identified to possess relatively high bandgap energies,8–10 making them particularly suitable for photodetector applications. The bandgap energies of MgCr2O4 are close to those observed in some semiconductors such as TiO2, ZnO, and CuO, which are known for their ability to absorb ultraviolet photons.11–13 Consequently, due to the higher band gap energies of MgCr2O4 chromites, they mainly absorb UV photons. While solar light predominantly consists of a minimal proportion of UV photons, accounting for approximately 5% of the total light energy, room-light lamps primarily emit visible photons.4 In order to expand the spectral response of MgCr2O4 chromites to include visible light, it is necessary to decrease their band gap energies. In fact, the smaller band gap indicates the higher absorption of visible light in photocatalytic reactions.4 The purpose of this study is to investigate the optical properties of MgCr2O4 chromites through the substitution of Ni and Cu. We have employed the sol–gel method to synthesize both Mg0.6Ni0.4Cr2O4 (MNCO) and Mg0.6Cu0.4Cr2O4 (MCCO) spinel chromites and investigated their thermal, structural, elastic, and optical properties. The substitution of Ni2+ and Cu2+ at the tetrahedral (A) site in MgCr2O4 chromites leads to modifications in their average A-site radius and crystal geometry, which in turn leads to a change in their optical properties. This study reveals significant results for the as-prepared samples. Significantly, the MNCO and MCCO samples demonstrated lower bandgap energies compared to MgCr2O4 chromites. This suggests the potential for improved visible light absorption of MgCr2O4 chromites through the substitution of Ni2+ and Cu2+. This could be useful in optoelectronic applications, particularly in light-emitting diodes (LEDs). Moreover, the as-synthetized samples possess additional advantages such as their excellent transparency, efficient light absorption, suitability for nonlinear applications, and, above all, their ease and cost-effectiveness of synthesis.
2. Experimental details
The spinel chromites, Mg0.6Ni0.4Cr2O4 and Mg0.6Cu0.4Cr2O4, were synthesized via the sol–gel method using high-purity nitrates ([Mg(NO3)2·6H2O], [Cu(NO3)2·6H2O], [Ni(NO3)2·6H2O], and [Cr(NO3)3·9H2O]). The weighed precursors of each compound were dissolved in distilled water under magnetic stirring on two hot plates at 90 °C, resulting in aqueous solutions. Once the nitrates were completely dissolved, precise amounts of citric acid (C6H8O7) were added to each solution as a complexing agent and dissolved under magnetic stirring. Ammonia (NH4OH) was then added to adjust the pH to approximately 7. Controlled amounts of ethylene glycol (C2H6O2) were subsequently added as a polymerization agent. The mixture was continuously heated and stirred until it formed a gel. The obtained gel was dried at 250 °C for 12 hours to achieve a dry foam, which was ground using an agate mortar. To decompose the organic matter, the resulting powders were annealed at 500 °C for 12 h in an electrical muffle furnace, followed by slow cooling to room temperature. The prepared powders underwent repeated cycles of grinding, pelletizing, and calcination within the temperature range of 600 °C to 1000 °C to promote grain crystallization of the samples, ensuring the formation of single-phase products. In this study, all characterizations were performed on the MNCO and MCCO chromites that were annealed at 1000 °C for 24 h.
The thermal characteristics of the samples were assessed through thermogravimetric analysis (TGA), which was conducted at temperatures ranging from 25 °C to 1000 °C with a temperature step of 0.33 °C. X-ray diffraction (XRD) patterns were obtained using a Panalytical X'Pert Pro System employing Cu-Kα radiation (λ = 1.5406 Å). The XRD data was collected within an angular range of 10° to 90° with an angular step of 0.02°. Fourier transform infrared (FTIR) spectra were acquired using an FTIR-8400S spectrometer, covering the wavenumber range of 400 cm−1 to 1000 cm−1 with a step of 1 cm−1. Optical properties were determined at room temperature using a UV-3101PC scanning spectrophotometer. This instrument was equipped with a dual-beam monochromator capable of covering a wavelength spectra from 200 nm to 2400 nm with a wavelength step of 2 nm. Two light sources were utilized for the optical measurements: a xenon lamp for the UV-visible range and a halogen lamp for the infrared range. This technique allows for the determination of absorbance (A) and reflectance (R) as functions of the wavelength. From these two measured optical parameters, the variations of other derived parameters can be studied, such as the absorption coefficient, penetration depth, extinction coefficient, refractive index, and non-linear optical parameters.
3. Results and discussions
3.1. Thermal analysis
The weight losses (W%) for Mg0.6Ni0.4Cr2O4 and Mg0.6Cu0.4Cr2O4 samples are shown in Fig. 1. The DTA curves versus temperature, also known as differential thermal analysis or the first derivative of weight loss, dW/dT, are also shown in the inset of Fig. 1. It is evident that losing weight primarily involves three steps. A peak in the dW/dT curves centered at 60 °C represents a weight loss that occurs in the first stage, which is ∼1% in the temperature range below 150 °C. The release of water molecules that are lost from beginning hydrated precursors may be indicated by this range of mass loss.8 The DTA curves for MNCO and MCCO show exothermic peaks at approximately 295 °C and 305 °C, respectively, which occur in the temperature range of 150 °C to 400 °C, which correspond to the second step weight loss and have a maximum weight loss of approximately 1.5%. Within this temperature range, there appears to be a mass decrease that indicates moisture, and leftover gases are escaping.4 A weight loss of up to 6% occurs during the third stage, which occurs in the 400–1000 °C temperature range. The formation of the spinel phases is indicated by the detection of exothermic peaks in the DTA curves at 890 °C for MNCO and 900 °C for MCCO. As such, it is possible to optimize the calcination temperature to any temperature above 900 °C.
 |
| Fig. 1 Thermogravimetric curve (TGA) for MNCO and MCCO spinel chromites. The inset shows the differential thermal curves (DTA). | |
3.2. Structural properties
3.2.1. Theoretical structural analysis. According to earlier research, Mg2+, Ni2+, and Cu2+ ions are dispersed throughout the A sites of spinel chromites containing Mg, Ni, Cu, and Cr, whereas Cr3+ ions are located at the B sites.14,15 Thus, (Mg0.62+Ni0.42+)A[Cr23+]BO42− and (Mg0.62+Cu0.42+)A[Cr23+]BO42− can be proposed as the cation distributions for Mg0.6Ni0.4Cr2O4 and Mg0.6Cu0.4Cr2O4, respectively. Based on these distributions, we can deduce that the degree of inversion for both samples is equal to zero. Therefore, MNCO and MCCO possess normal spinel structures. The theoretical lattice constant (ath) is calculated using the proposed cation distributions of the MNCO and MCCO samples as follows:16 |
 | (1) |
where
and
are the average ionic radii per molecule for the corresponding A and B-sites; in this case, Ci is the concentration of the element i with ionic radius ri on a specific site. The ionic radius of oxygen is represented by rO = 1.38 Å. Table 1 displays the calculated values of ath for MCCO and MNCO. The larger ath value of MCCO compared to MNCO is attributed to the higher ionic radius of Cu2+ (0.57 Å) compared to Ni2+ (0.55 Å).17 Using the theoretical lattice constant, the value of the oxygen positional parameter “u” can be calculated as follows:18 |
 | (2) |
Table 1 Calculated and refined structural parameters for MNCO and MCCO spinel chromites
Sample |
|
MNCO |
MCCO |
Structural parameters |
Calculated values |
Refined values |
Calculated values |
Refined values |
a (Å) |
|
8.310 |
8.326 (3) |
8.322 |
8.327 (7) |
V (Å3) |
|
573.856 |
577.323 |
576.345 |
577.468 |
u |
|
0.3849 |
0.3846 |
0.3853 |
0.3852 |
δ |
|
0.0099 |
0.0099 |
0.0103 |
0.0103 |
LA (Å) |
|
3.5983 |
3.603 |
3.6035 |
3.617 |
LB (Å) |
|
2.9380 |
2.933 |
2.9423 |
2.952 |
dAL (Å) |
|
1.9420 |
1.941 (4) |
1.9500 |
1.946 (4) |
dBL (Å) |
|
1.9884 |
1.989 (4) |
1.9986 |
1.992 (4) |
dAE (Å) |
|
3.1713 |
3.176 (6) |
3.1843 |
3.179 (6) |
dBE (Å) |
|
2.7048 |
2.704 (6) |
2.7002 |
2.701 (6) |
dBEU (Å) |
|
2.9427 |
2.946 (6) |
2.9473 |
2.948 (6) |
Me–Me distances (Å) |
b |
2.9380 |
2.935 |
2.9423 |
2.942 |
|
c |
3.4451 |
3.454 |
3.4501 |
3.461 |
|
d |
3.5983 |
3.602 |
3.6035 |
3.608 |
|
e |
5.3975 |
5.401 |
5.4053 |
5.432 |
|
f |
5.0888 |
5.087 |
5.0962 |
5.094 |
Me–O distances (Å) |
p |
1.9950 |
1.997 (4) |
1.9950 |
1.999 (4) |
|
q |
1.9420 |
1.946 (4) |
1.9500 |
1.949 (4) |
|
r |
3.7187 |
3.689 (4) |
3.7340 |
3.695 (4) |
|
s |
3.6495 |
3.653 (4) |
3.6529 |
3.661 (4) |
Bond angles (°) |
θ1 |
122.098 |
121.30 (2) |
121.990 |
120.91 (2) |
|
θ2 |
139.750 |
140.65 (1) |
139.300 |
140.36 (1) |
|
θ3 |
94.840 |
94.88 (2) |
95.028 |
95.18 (2) |
|
θ4 |
126.35 |
126.17 (1) |
126.39 |
126.27 (1) |
|
θ5 |
71.212 |
72.58 (1) |
71.923 |
72.73 (1) |
Agreement factors |
Rp (%) |
|
5.98 |
|
6.66 |
|
Rwp (%) |
|
8.12 |
|
8.98 |
|
Rexp (%) |
|
7.42 |
|
7.54 |
|
RBragg (%) |
|
2.38 |
|
3.63 |
|
χ2 (%) |
|
1.10 |
|
1.19 |
Table 1 displays the u values of MCCO and MNCO. As observed in this table, the u value remains nearly constant for both samples. In a cubic spinel structure, the ideal u value is 0.375. However, the calculated u values are slightly larger than the ideal value, indicating a possible displacement of the oxygen anion from its ideal position. By analyzing the deviation from the ideal oxygen parameter (δ = u – 0.375), it is confirmed that there is a slight distortion in the lattice for both samples. On the other hand, the inter-ionic distances between magnetic ions, known as the hopping lengths (LA and LB), provide insight into the strength of spin interaction between ions in the tetrahedral and octahedral sites. To determine these hopping lengths, Stanley's equations were employed:18
|
 | (3) |
Table 1 presents the calculated values of LA and LB for both samples. The hopping lengths are higher for MCCO than MNCO. The following equations are provided for the calculation of A and B bond lengths (dAL and dBL), the tetrahedral edge length (dAE), and the shared (dBE) and unshared (dBEU) octahedral edge lengths.18 These distances are calculated for MNCO and MCCO using the values of ath and u as follows:
|
 | (4) |
|
 | (5) |
|
 | (6) |
|
 | (7) |
|
 | (8) |
Table 1 lists the values for dAL, dBL, dAE, dBE, and dBEU distances for both samples. It is evident from the table that these parameters are higher for MCCO compared to MNCO. Additionally, the cation–anion bond distances (p, q, r, and s) and cation–cation bond distances (b, c, d, e, and f) in spinels (see Fig. 2a) were used to determine the bond angles (θ1, θ2, θ3, θ4, and θ5).19 In spinel materials, metal cations occupy positions A and B, with neighboring cations being their closest neighbors. Consequently, three types of super-exchange interactions exist: A–A, B–B, and A–B. These interactions can be further categorized into five types due to variations in the chemical bond angles between metal cations and oxygen ions. The bond angles θ1 and θ2 correspond to A–O–B interactions, θ3 and θ4 relate to B–O–B interactions, and θ5 is associated with A–O–A interactions. Fig. 2a illustrates the configuration of ion pairs in spinel materials with favorable distances. By applying the principle of super-exchange and considering the five relative positions in Fig. 2a, it becomes evident that A–B super-exchange is the strongest, followed by B–B super-exchange, while A–A super-exchange is the weakest. The following equations were used to calculate the cation–anion (Me–O) distances, cation–cation (Me–Me) distances, as well as the bond angles (Me–O–Me):18,19
|
 | (9) |
|
 | (10) |
|
 | (11) |
|
 | (12) |
|
 | (13) |
|
 | (14) |
|
 | (15) |
 |
| Fig. 2 (a) Favorable interionic distances and angles in spinel samples for better magnetic interaction. (b) XRD patterns for MNCO and MCCO spinel chromites. All peaks are indexed in the cubic spinel type structure (Fd m space group). The inset shows observed XRD profiles of the most intense peaks (Bragg reflections (3 1 1)). (c and d) Structural refinement of the XRD patterns using Rietveld method for MNCO and MCCO, respectively. | |
The calculated values of all these parameters are displayed in Table 1. The table clearly demonstrates that the Me–Me and Me–O distances are higher for MCCO compared to MNCO. In addition, the analysis of the data presented in Table 1 reveals the dominance of A–B interactions over A–A and B–B interactions, corroborating the findings depicted in Fig. 2a. Moreover, the bond angles exhibit a trend that alternates between decreasing and increasing for both MCCO and MNCO samples.
3.2.2. Rietveld refinement of experimental XRD patterns. The crystalline structure and phase purity of the Mg0.6Ni0.4Cr2O4 and Mg0.6Cu0.4Cr2O4 samples were confirmed by XRD analysis, as illustrated in Fig. 2b. The diffraction peaks show cubic spinel phases for both samples according to the space group Fd
m. Furthermore, the XRD patterns verify that the produced powders are homogeneously crystallized, displaying a single, pure phase devoid of any other phases. The positions and intensities of all distinguishable peaks closely corresponded to those found in the standard JCPDS card no. 77-0007.8 Furthermore, the XRD patterns exhibit a slight shift towards lower diffraction angles for MCCO compared to MNCO, as observed in the inset of Fig. 2b. This shift indicates a higher lattice constant (a) and cell volume (V) for MCCO. The experimental analysis of the synthesized samples involved the utilization of Rietveld refinement,20 employing the proposed cation distributions discussed in Section 3.2.1. The difference between the calculated and observed patterns is illustrated in Fig. 2(c) and (d). The Rietveld refinement assigned B cations, A cations, and oxygen to positions 16d (1/2, 1/2, 1/2), 8a (1/8, 1/8, 1/8), and 32e (x, y, z), respectively. The reliability of the Rietveld fitting was evaluated using the R-factors (expected Rexp, Bragg RBragg, profile Rp, and weighted profile Rwp), all of which were found to be less than 10%. The goodness of fit, represented by χ2 = Rwp/Rexp, was close to one, indicating a good fit. The atomic position of oxygen (0.2618(4) for MNCO and 0.2625(4) for MCCO) adheres to the spinel's typical characteristics. Table 1 summarizes the refined structural parameters and compares them to the theoretical values from Section 3.2.1. The good agreement between the refined and calculated structural parameters supports the proposed cation distribution for the Mg0.6Ni0.4Cr2O4 and Mg0.6Cu0.4Cr2O4 chromite samples. Based on the data presented in Table 1, it is evident that the refined lattice constants of our samples are higher in comparison to the undoped MgCr2O4 chromites.6,9 This increase can be attributed to the difference in the ionic radii between Ni2+, Cu2+, and Mg2+.17 Furthermore, we calculated the average crystallite size (D) using the Debye–Scherrer formula.6 The average crystallite size for MCCO (104 nm) is greater than that of MNCO (98 nm). This discrepancy can be attributed to the larger ionic radius of Cu2+ in comparison to Ni2+. Additionally, we determined the experimental X-ray density (ρx)6 for both samples. The difference in density values between MNCO (4.74 g cm−3) and MCCO (4.78 g cm−3) can be attributed to the fact that nickel has a lower density (8.91 g cm−3) compared to copper (8.96 g cm−3).21
3.3. Infrared and elastic properties
3.3.1. FTIR spectra analysis. The FTIR spectra were analyzed to investigate the functional groups present in the samples and to determine the vibrational frequencies of the network structures. Fig. 3a displays the infrared spectra of MCCO and MNCO chromite spinels. These spectra exhibit two absorption bands related to the vibrational bands of incorporated metal oxides.1,9,10 The presence of these bands confirms the successful synthesis of the chromite samples with metal-oxide bonds involving Ni, Cu–O and Cr–O. The first band (νB), observed near 450 cm−1, is attributed to metal–oxygen (Cr–O) stretching vibrations at the octahedral site. The second band (νA), appearing near 560 cm−1, is associated with the generation of tetrahedral bonds (Ni, Cu–O). Upon examining Fig. 3a, it becomes evident that the bands associated with metal–oxygen stretching vibrations at both the octahedral and tetrahedral sites demonstrate a slight shift towards lower wavenumbers in the case of MCCO when compared to MNCO. As presented in Table 2, the νA value decreases from 575 cm−1 for MNCO to 566 cm−1 for MCCO. However, the νB value decreases from 461 cm−1 for MNCO to 455 cm−1 for MCCO. This decrease in the bands associated with metal–oxygen stretching vibrations at both the A and B sites can indeed be related to the higher Me–O distances for MCCO compared to MNCO, as shown in the structural part. In spinel structures, the vibrational frequencies of metal–oxygen stretching vibrations are influenced by the bond lengths and bond strengths. When the cation–oxygen bond length increases, it indicates a lengthening of the bonds between the metal cations and oxygen atoms. The elongation of the cation–oxygen bond lengths leads to a decrease in bond strength, which in turn results in a decrease in the vibrational energy of the metal–oxygen bonds. This decrease is reflected in the FTIR spectra as a shift towards lower wavenumbers or frequencies for the bands associated with metal–oxygen stretching vibrations. Therefore, as the Me–O distances are higher for MCCO compared to MNCO, the metal–oxygen stretching vibrations at both sites experience a decrease in vibrational energy. This decrease is manifested as a shift towards lower wavenumbers in the FTIR spectra.
 |
| Fig. 3 (a) FTIR spectra for MNCO and MCCO spinel chromites. (b) UV-vis-NIR absorbance spectra versus wavelength. (c) Reflectance spectrum R(λ). | |
Table 2 Elastic and thermal parameters for MNCO and MCCO spinel chromites
Sample |
|
MNCO |
MCCO |
Absorption bands (cm−1) |
νB |
461 |
455 |
νA |
575 |
566 |
Force constant (N m−1) |
Kt |
95.89 |
97.65 |
Ko |
117.35 |
114.32 |
Kav |
106.62 |
105.98 |
Stiffness constant (GPa) |
C11 |
128.05 |
127.27 |
C12 |
42.68 |
42.42 |
C44 |
67.42 |
68.07 |
Wave velocity (m s−1) |
νl |
5197.51 |
5160.01 |
νt |
3000.78 |
2979.13 |
νm |
3331.44 |
3307.40 |
Longitudinal modulus (GPa) |
L |
128.05 |
127.27 |
Rigidity modulus (GPa) |
G |
42.82 |
42.42 |
Bulk modulus (GPa) |
B |
71.14 |
70.71 |
Poisson's ratio |
σ |
0.25 |
0.25 |
Pugh ratio |
B/G |
1.661 |
1.667 |
Debye temperature (K) |
θD |
455.96 |
452.53 |
Thermal conductivity (W m−1 K−1) |
Kmin |
0.97 |
0.96 |
3.3.2. Elastic properties. Spinel materials exhibit significant elastic and thermal properties due to the existence of interatomic and interionic forces. The most popular technique for figuring out elastic constants and Debye temperatures is ultrasonic pulse transmission.22 Even though the elastic properties of these materials are governed by external stresses, several studies have examined the correlations between elastic, thermal, structural, and infrared properties. Modi et al. developed a detailed method for investigating the elastic properties of spinel systems using FTIR spectra.23 The force constants (Kt) for the tetrahedral site and (Ko) for the octahedral site are directly related to the active infrared phonon mode's wavenumbers νA and νB. The following Waldron relations were used to calculate the force constants (Kt) and (Ko) in the cubic spinel structure:16 |
 | (16) |
The molecular mass MA at the A sites was calculated using the molecular masses of Mg, Ni and Cu elements as MA = 0.6 × M(Mg) + 0.4 × M(Ni) = 38.06 g mol−1 for (Mg0.62+Ni0.42+)A[Cr23+]BO42− and MA = 0.6 × M(Mg) + 0.4 × M(Cu) = 40.00 g mol−1 for (Mg0.62+Cu0.42+)A[Cr23+]BO42−. However, the molecular mass (MB) at the B sites remained constant for both samples, with a value of MB = 2 × M(Cr) = 103.99 g mol−1. Table 2 presents the comparison between the force constants (Kt and Ko) and the average force constant
for MNCO and MCCO samples. It is evident that the estimated Ko values are significantly higher than the corresponding Kt values for both samples. Additionally, the average force constant Kav is lower for MCCO in comparison to MNCO. This decrease in Kav is typically associated with the longer Me–O bond distances observed in MCCO compared to MNCO. Hook's law establishes a relationship between stress (σi), strain (εij), and stiffness (Cij) through the stress–strain approach.23 The stiffness values (Cij) play a crucial role in determining the elastic constants. In the case of cubic symmetry, only three dominant stiffness values are considered: C11, C12, and C44. Among these, C11 represents elasticity in length, while C12 and C44 represent elasticity in shape. The stiffness coefficients (C11, C12, and C44) were calculated using the following equations:18
|
 | (17) |
In these equations, a represents the unit cell parameter, ρ represents the X-ray density,
is the transverse wave velocity (where
is the longitudinal wave velocity), and
is the Poisson's ratio (where L = ρνl2 and G = ρνt2 indicate the longitudinal and rigidity moduli, respectively). The values of stiffness constants, transverse and longitudinal wave velocity, rigidity and longitudinal moduli, and Poisson's ratio are all tabulated in Table 2. The lower values of νl and νt for MCCO than MNCO may be ascribed to the decrease of average force constant Kav. For both samples, the Poisson ratio remains constant at σ = 0.25. This value is consistent with the isotropic elasticity theory because it falls between −1 and 0.5.18 Furthermore, it is noted from Table 2 that the stiffness constants (C11 and C12) are lower for MCCO than MNCO. Besides, to ascertain the ductility or brittleness of the prepared materials based on the Pugh criteria,18 the ratio between the bulk modulus (B = 1/3(C11 + 2C12)) and the rigidity modulus (G) was evaluated. A ductile material's B/G ratio should be greater than 1.75, whereas a brittle material should be smaller than 1.75. Table 2 demonstrates that MCCO exhibits lower values for the bulk modulus (B), rigidity modulus (G), and longitudinal modulus (L) compared to MNCO. The change in these elastic moduli for both MNCO and MCCO can be attributed to the interatomic binding between different atoms within their spinel lattices. In addition, the calculated values of the Pugh ratio are below the critical value of 1.75, suggesting the brittle mechanical nature of the prepared samples. On the contrary, the Debye temperature (θD) is an essential parameter that characterizes the upper limit of atomic lattice vibrations and provides valuable insights into the thermodynamic properties of a solid. The following equation can be used to estimate the θD value in accordance with Anderson's formula:23
|
 | (18) |
where
kB is Boltzmann's constant, ℏ is Planck's constant,
M is the molecular mass,
ρ is the X-ray density,
NA is Avogadro's number,
q is the number of atoms per unit formula (equals to 7 in a cubic spinel system), and

represents the mean wave velocity. In addition, the thermal conductivity is a thermodynamic property that quantifies a material's capacity to conduct heat. The minimum value of thermal conductivity, represented as
Kmin, can be determined using this equation:
18 |
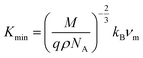 | (19) |
In Table 2, it is observed that the calculated values of θD, νm, and Kmin are consistently lower for MCCO compared to MNCO. The decrease in νm can be attributed to the lower transverse and longitudinal wave velocities (νl and νt) observed in MCCO. Furthermore, the lower Debye temperature for MCCO can be attributed to the reduced bonding strength in this sample.24
3.4. Optoelectronic properties
3.4.1. UV-vis-NIR absorbance and reflectance spectra. Fig. 3(b) and (c) shows the reflectance spectra (R(λ)) and absorption spectra (A(λ)) of Mg0.6Ni0.4Cr2O4 and Mg0.6Cu0.4Cr2O4 chromites. The A(λ) data show UV-vis absorption bands, indicating that the samples are capable of efficiently absorbing UV-vis light. They are therefore attractive options for photo-catalysis materials.25,26 The compounds also show NIR absorption bands, indicating that they could be suitable candidates for NIR optoelectronic devices.4 For both samples, the band gap energies were estimated using Tauc's law as follows:5,10where α = 2.303 × A/d is the optical absorption coefficient (with A is the absorbance and d is the thickness of each sample), Eg is the band-gap energy, hv is the photon energy, and β is a parameter describing the compound's degree of disorder. The value of the exponent n denotes the type of optical transition: direct optical transitions for n = 1/2 and indirect optical transitions for n = 2. Accordingly, the following equation is employed to validate the optical transition in the samples: |
ln(αhν) = ln(β) + n ln(hν − Egd)
| (21) |
Based on the [(αhν)1/2 vs. hν] curves depicted in Fig. 4(a) and (b), it can be observed that neither of the samples demonstrate indirect optical transitions. From the [(αhν)2 vs. hν] curves shown in Fig. 4(a) and (b), the direct (Egd) band gap values were determined to be 2.78 eV for MNCO and 2.65 eV for MCCO. The [ln(αhν) vs. ln(hν − Eg)] curves in Fig. 4(c) and (d) indicate that the n exponent values are approximately 0.5 for both samples. Therefore, both MNCO and MCCO spinel chromites exhibit a direct optical transition, consistent with previous findings on other spinel chromites.4,26 This direct optical transition indicates a direct band gap that allows for the movement of electrons from the valence band to the conduction band without the presence of intermediate energy states. With their remarkable light-absorbing and light-emitting properties, these samples are well-suited for applications in solar cells, lasers, and optoelectronic devices. According to the data in Table 3, the band gap energy (Eg) decreases from 2.78 eV for MNCO to 2.65 eV for MCCO. The band gap energy is typically influenced by factors such as grain size, structural parameters, and impurities.4 In the structural part, our findings indicate that the grain size is higher in MCCO compared to MNCO. With an increase in grain size, the presence of grain boundaries is reduced. This reduction in grain boundaries leads to a decrease in the scattering of carriers at these boundaries, ultimately resulting in a lower band gap energy (Eg) for MCCO compared to MNCO. Furthermore, the enlargement of grain size can also minimize the effects of quantum confinement, thereby contributing to the overall reduction in the band gap energy. The band gap energies (Eg) of the as-prepared samples, presented in Table 3, have been compared to those of some semiconductors11–13 and other spinel chromites.2,3,8–10,25,27–30 It is worth noting that materials with larger Eg values are known to absorb ultraviolet (UV) photons, as evidenced by the values provided for some semiconductors11–13 in Table 3. However, since sunlight primarily consists of visible light (with only around 5% UV photons) and most room-light lamps emit visible photons,4 there is a growing interest in studying materials for their response to visible light.2,3,25,27 Notably, the estimated Eg values for the as-synthesized MNCO and MCCO samples are lower than those reported for certain semiconductors and other spinel chromites.11–13,28–30 A lower band gap energy allows for greater absorption of visible light in photocatalytic reactions,27 making our samples potential candidates for visible light absorption. Additionally, our study revealed that the band gap energy values of the synthesized samples were smaller than the reported values for MgCr2O4 chromites, which are typically employed as photocatalysts under UV illumination.8–10 This suggests that the substitution of Ni and Cu provides an opportunity to extend the sunlight absorption range of MgCr2O4 spinel chromites. It is important to note that the Eg values for the MCCO and MNCO samples also exceed the minimum energy requirement for water splitting (Eg > 1.23 eV). Consequently, our samples can also serve as photocatalytic materials.4
 |
| Fig. 4 (a and b) Plots of (αhν)1/2 and (αhν)2 versus hν for MNCO and MCCO spinel chromites. (c and d) Plots of ln(αhν) versus ln(hν − Eg). | |
Table 3 Band gap energies for MNCO and MCCO spinel chromites compared to those reported in previous works
Sample |
Band gap energy (eV) |
Reference |
TiO2 |
3.20 |
11 |
ZnO |
3.37 |
12 |
CuO |
3.85 |
13 |
CoCr2O4 |
1.19 |
2 |
ZnCr2O4 |
1.80 |
3 |
Ni0.5Mn0.5Cr2O4 |
1.90 |
27 |
Ni0.7Cd0.3Cr2O4 |
2.20 |
25 |
CuCr2O4 |
3.55 |
28 |
Mg0.5Zn0.5Cr2O4 |
3.46 |
29 |
Mg0.5Zn0.3Co0.2Cr2O4 |
3.00 |
29 |
CdCr2O4 |
3.5 |
30 |
Cd0.5Co0.5Cr2O4 |
3.325 |
30 |
CoCr2O4 |
3.00 |
30 |
MgCr2O4 |
3.10 |
8 |
MgCr2O4 |
3.47 |
9 |
MgCr2O4 |
2.98 |
10 |
Mg0.6Ni0.4Cr2O4 |
2.78 |
This work |
Mg0.6Cu0.4Cr2O4 |
2.65 |
This work |
3.4.2. Penetration depth and extinction coefficient. The penetration depth (δ) describes how deeply light or radiation can penetrate a material.26 It depends on the material's ability to reduce the intensity of incident radiation. The variation of δ(λ) can be estimated using the reciprocal of the absorption coefficient (α(λ)). The δ(λ) curves for MCCO and MNCO chromite samples in Fig. 5a exhibit distinct variations in relation to the absorption coefficient. In the UV-vis-NIR regions, the δ(λ) curves show specific absorption features with different intensities. Light can penetrate deeper into the materials within these three wavelength ranges. Fig. 5a also shows lower values of δ(λ) for both samples. Similar orders of magnitude in δ(λ) values have been observed for other spinel chromites.25–27 This indicates improved light absorption and energy conversion efficiency for MCCO and MNCO chromites. They are also suitable for high-performance photodetectors, detecting light across various wavelengths.
 |
| Fig. 5 (a) Penetration depth (δ) versus λ and (b) extinction coefficient (k) versus hν for MNCO and MCCO spinel chromites. (c and d) Plot of ln(α) versus hν. The insets show the curve of (α/λ)2 vs. 1/λ. | |
The extinction coefficient (k) can be calculated using the product of the absorption coefficient (α) and the wavelength (λ), divided by 4π.25 Fig. 5b demonstrates the behavior of the k coefficient in relation to photon energy (hν) for MCCO and MNCO samples. Notably, the k-values exhibit higher values at lower hν values, but as hν increases, they dramatically decrease and approach zero, indicating minimal light loss within the higher energy range. Similar trends in k values decreasing as the photon energy increases have been observed in other materials.4,27 The change in k values falls within the order of 10−4, indicating that the scattering and absorption losses during light propagation through the samples are minimal. This behavior signifies the good transparency of MCCO and MNCO chromites.
3.4.3. Urbach energy. The Urbach energy (Eu) is a parameter used in optical spectroscopy to characterize the degree of disorder, impurities, and defects in a material.25 It provides information about the localized states within the band gap and the broadening of electronic transitions. It represents the characteristic decay length of the exponential tail and is inversely proportional to the spatial extent of localized states. A smaller Eu value indicates a more ordered and less disordered material, while a larger Eu value corresponds to a higher density of defects and disorder. The Urbach energy (Eu) can be determined from the photon energy (hν) as follows:27 |
 | (22) |
In this equation, α represents the absorption coefficient, and α0 is a constant. The logarithmic form of this equation is often used to analyze experimental data and extract the Urbach energy from absorption spectra. In Fig. 5(c) and (d), the Urbach energy (Eu) values (see Table 4) were extracted from the [ln(α) vs. (hv)] curves. Table 4 indicates that the Eu value decreases from 2.14 eV for MNCO to 1.86 eV for MCCO. These values exceed the Urbach energies obtained at room temperature for certain conventional semiconductor materials like ZnO (Eu = 0.067 eV)31 and GaAs (Eu = 0.044 eV).32 This highlights the significance of disorder and defects in the as-prepared samples when comparing them to these semiconductor materials. However, the estimated Eu values for the MNCO and MCCO spinels are consistent with those reported for other spinel systems, such as Ni0.5Mn0.5Cr2O4 (Eu = 2.34 eV)27 and ZnFe1.96Co0.04O4 (Eu = 1.861 eV).33 On the other hand, the lower Eu value for MCCO compared to MNCO can be attributed to the larger grain size of MCCO in comparison to MNCO. The decrease in Urbach energy with an increase in grain size can be explained by the reduction in structural disorder and defects within the material. When grain size increases, the material's crystalline structure becomes more continuous and coherent, resulting in fewer structural imperfections such as grain boundaries, dislocations, and defects. The Urbach energy (Eu) can be related to the steepness parameter S(T) through this equation:34
|
 | (23) |
Table 4 The values of optical parameters for MNCO and MCCO spinel chromites
Sample |
MNCO |
MCCO |
Eg (eV) |
2.78 |
2.65 |
Eu (eV) |
2.14 |
1.86 |
S |
0.012 |
0.014 |
Ee–ph (eV) |
55.55 |
47.62 |
λT (nm) |
580 |
487 |
n0 |
1.92 |
1.64 |
n1 |
0.024 |
0.010 |
n2 |
0.162 |
0.355 |
E0 (eV) |
5.66 |
4.62 |
Ed (eV) |
17.65 |
7.22 |
εop |
4.19 |
2.56 |
 |
2.03 |
1.60 |
λ0 (nm) |
468 |
455 |
S0 (10−6 m−2) |
11.55 |
6.94 |
 |
1.59 |
1.19 |
χ(1) (SI) |
0.224 |
0.139 |
χ(3) (10−13 m2 V−2) |
4.26 |
0.64 |
n2 (10−12 m2 V−1) |
8.25 |
1.45 |
In this equation, kB represents the Boltzmann constant, and T represents the room temperature. The steepness parameter, S(T), describes the broadening of the absorption edge caused by interactions between electrons–phonons or excitons–phonons, as referenced in ref. 35. It quantifies the rate at which the absorption coefficient changes with respect to photon energy near the band edge. A larger S(T) value indicates a steeper absorption edge and a broader energy range over which the absorption coefficient changes significantly. As shown in Table 4, the S parameter was estimated to be 0.012 and 0.014 for MNCO and MCCO, respectively. In this case, the higher S(T) value for MCCO indicates a greater broadening of the absorption edge compared to MNCO. According to eqn (24), the electron–phonon interaction energy (Ee–ph) can be estimated from the S parameter as:35
|
 | (24) |
The Ee–ph value represents the energy associated with the interaction between electrons and phonons in the material. It is an important parameter in understanding the electronic and thermal properties of a material. A higher Ee–ph value indicates a stronger electron–phonon interaction, suggesting that the material has a higher propensity for energy dissipation through lattice vibrations. In our case, by applying eqn (24), we have determined the Ee–ph values for our samples as 55.55 eV for MNCO and 47.62 eV for MCCO (see Table 4). The higher Ee–ph value for the MNCO sample suggests a stronger electron–phonon interaction, indicating a higher propensity for energy dissipation through lattice vibrations compared to the MCCO sample. On the other hand, in optoelectronic devices, the maximum wavelength of incident radiation, denoted as λT, also known as the threshold wavelength or critical wavelength, is a significant factor in determining the suitability of a compound for such applications. The λT value represents the minimum wavelength of light required to initiate specific optoelectronic processes, such as absorption or emission, in a material. To estimate the λT values, we have used this equation:34
|
 | (25) |
where
α represents the absorption coefficient,
λ is the wavelength of incident radiation, and
C is a constant. As mentioned in the insets of
Fig. 5(c) and (d) and
Table 4, the fitting curves yield values of
λT that decrease from 580 nm for MNCO to 487 nm for MCCO. The observed decrease in
λT indicates that the bandgap energy of the MCCO material is lower compared to the MNCO sample, consistent with previous observations. In general, a lower bandgap energy corresponds to a lower threshold wavelength. This implies that the material requires larger-energy photons (lower wavelengths) to initiate optoelectronic processes.
3.4.4. Refractive index. The refractive index (n) is an important parameter in the field of spectral dispersion applications. It characterizes how light propagates through a material and is influenced by factors such as the material's composition, density, and optical properties. The refractive index (n) can be estimated using the R(λ) and the k(λ) data as follows:25 |
 | (26) |
In Fig. 6(a) and (b), the n(λ) curves for the MNCO and MCCO spinel chromites are displayed. The presence of high absorption bands in the UV-vis-NIR zones indicates the high quality and compactness of the samples.26 To model the variations of the refractive index (n) with wavelength, the following Cauchy relation is used:
|
 | (27) |
 |
| Fig. 6 (a and b) Refractive index n versus λ for MNCO and MCCO spinel chromites, respectively. The insets show the fit of n(λ) curves using Cauchy relation. (c and d) Plots of 1/(n2 − 1) versus (hν)2 for MNCO and MCCO, respectively. The insets show the plots of 1/(n2 − 1) versus (1/λ2). | |
In this equation, n0, n1 and n2 represent the Cauchy parameters, which describe the wavelength dependence of the refractive index. The insets of Fig. 6(a) and (b) illustrate the variations of the refractive index (n) versus
, as modeled by eqn (27). The Cauchy parameters were estimated in Table 4 for MNCO and MCCO samples. These Cauchy parameters provide a mathematical representation of how the refractive index (n) varies with wavelength for our samples. They provide valuable information for applications involving spectral dispersion and light propagation in materials.
Dispersion is a crucial factor that plays a significant role in material selection for potential applications and the design of optoelectronic devices with spectral dispersion.36 Thus, in order to comprehend the dispersion parameters of the refractive index and dispersion energies of Mg0.6Ni0.4Cr2O4 and Mg0.6Cu0.4Cr2O4 spinel chromites, a thorough discussion of this topic is required. Wemple–Di-Domenico's Single Effective Oscillator Model37 is the best model to study these parameters. The effective single oscillator energies (E0) and dispersion or strength energies (Ed) have been successfully investigated by this model. The refractive index (n) and the energies (E0 and Ed) are connected by the following expression, per the Wemple and Di-Domenico dispersion theory:37
|
 | (28) |
This equation can be graphically represented by plotting the refractive index factor [1/(n2 − 1)] against the square of photon energy [(hν)2]. Fig. 6(c) and (d) illustrates this graphical representation. By analyzing the resulting straight line, one can determine the slope [1/(E0Ed)] and the y-axis intercept (E0/Ed). Therefore, the energy values of E0 and Ed can be easily obtained for MNCO and MCCO from Fig. 6(c) and (d) and presented in Table 4. According to Tanaka,38 E0 is approximately twice the value of the bandgap energy (Eg). With the estimated values of E0 and Ed, we can calculate the zero-frequency dielectric constant
and the static refractive index
. As presented in Table 4, the calculated values were calculated as εop = 4.19 and
for MNCO and as εop = 2.65 and
for MCCO. It is noteworthy that the calculated values of the static refractive index
agree well with the n0 values estimated from the Cauchy fit. This agreement indicates the consistency and reliability of the results obtained through different methods. The average oscillator strength (S0) and the oscillator wavelength (λ0) for the MNCO and MCCO samples were determined using eqn (29).36 This equation relates the refractive index (n), the average oscillator strength (S0), the oscillator wavelength (λ0), and the wavelength (λ) of the light as follows:
|
 | (29) |
As shown in the insets of Fig. 6(c) and (d), the curves of
were plotted. From these plots, the values of λ0 were determined as 468 nm for MNCO and 455 nm for MCCO (see Table 4). The S0 values were found to be 11.55 × 10−6 m−2 and 6.94 × 10−6 m−2 for MNCO and MCCO, respectively. These values provide information about the average oscillator strength and the characteristic wavelength of the optical transitions in the studied spinel ferrites. They are important parameters in understanding the optical behavior and electronic structure of materials. Comparing the values of S0 and λ0 for the MNCO and MCCO samples, we observe that both parameters are lower for MCCO. These differences indicate variations in the optical properties and behavior of the spinel chromites, potentially due to changes in their electronic structure or composition. On the other hand, the static refractive index
can be calculated as follows:39
|
 | (30) |
The values for
were estimated, respectively, as 1.59 and 1.19 for the MNCO and MCCO, respectively. In particular, the estimated values of
agree well with those obtained from the Cauchy adjustment and the calculation from E0 and Ed.
3.4.5. Non-linear optical parameters. Nonlinear optics delves into investigating the way light engages with matter. Typically, a material's optical reaction varies linearly in accordance with the magnitude of the electric field. Yet, under elevated power conditions, material properties may undergo swifter alterations, resulting in nonlinear phenomena. Linear and nonlinear susceptibilities are interconnected with induced polarization, manifested as a series of powers of the light's electric field intensity E, as governed by the following relation:40 |
P = ε0[χ(1)E + χ(2)E2 + χ(3)E3 +…]
| (31) |
where χ(1) represents the linear susceptibility, and χ(2) and χ(3) denote the second and third-order nonlinear susceptibilities, respectively. However, Lines et al. have indicated that χ(2) is nearly negligible in optically isotropic glasses and in crystals possessing a symmetry centre. The linear optical susceptibility (χ(1)) can be anticipated based on the linear refractive index as follows: |
 | (32) |
The nonlinear optical susceptibility χ(3) and the linear optical susceptibility χ(1) are directly related according to Miller's rule:41
|
 | (33) |
where
A = 1.7 × 10
−10 esu is a frequency-independent constant,
40 with 1 esu being equivalent to 1.4 × 10
−8 m
2 V
−2. In the extended wavelength range, as the product (
hv) approaches zero, the refractive index tends to the static refractive index

, and
eqn (32) and
(33) become as follows:
|
 | (34) |
|
 | (35) |
The nonlinear refractive index n2 can be expected from the nonlinear susceptibility χ(3) via this relation:41
|
 | (36) |
The anticipated values of χ(1), χ(3), and n2 are also summarized in Table 4 for MNCO and MCCO samples. The values of these parameters agree well with those found for other samples considered as promising candidates for nonlinear applications such as lasing, telecommunication, optical switching, modulators, optical information processing, and ultrafast optical communication.40
3.4.6. Optical conductivity and optical dielectric constants. In this section, we have evaluated the optical conductivity (σop(λ)), real component of optical permittivity (ε1(λ)), imaginary component (ε2(λ)), and optical loss factor (tan(δ)) for the MNCO and MCCO spinel chromites. The following equations were used to calculate these parameters based on the absorption coefficient (α), refractive index (n), speed of light (c), and extinction coefficient (k).4,40 |
 | (37) |
|
ε1(λ) = n2(λ) − k2(λ)
| (38) |
|
 | (40) |
Based on the observed behavior of the optical conductivity (σop(λ)) as depicted in Fig. 7a, it is evident that the materials exhibit a strong photoresponse. The values of σop(λ) were found to be higher at shorter wavelengths, indicating increased conductivity and light absorption in this range. Conversely, the values were weaker at longer wavelengths, suggesting reduced conductivity and absorption of light in that region. This behavior implies that the prepared materials are more responsive to light containing higher-energy photons. The higher conductivity at shorter wavelengths indicates a greater ability to conduct and interact with light in this energy range. This phenomenon could be attributed to the excitation of electrons within the material. As shown in Fig. 7b, the real part of the optical dielectric constant, ε1(λ), showed a similar trend to the refractive index, indicating a consistent behavior in the prepared chromites. This similarity suggests that changes in the refractive index can be attributed to variations in the ε1(λ) constant. Furthermore, the imaginary part of the dielectric constant, ε2(λ), shows a slight increase with increasing wavelength (see Fig. 7c). This indicates a minor level of absorption or energy dissipation as the wavelength becomes longer, making the samples suitable for efficient light absorption. This behavior is consistent with similar observations in other spinel compounds and can be attributed to various factors, such as electronic transitions, lattice vibrations, or defect-related processes within the material.4,42 The optical loss factor, tan(δ), quantifies the energy dissipation in the material when subjected to an electric or electromagnetic field. The behavior of tan(δ) was examined in Fig. 7d, and it was found to increase with increasing wavelengths. This indicates an increase in energy dissipation or loss in the material as the wavelength becomes longer.42
 |
| Fig. 7 (a) Optical conductivity (σop) versus λ for MNCO and MCCO spinel chromites. (b) Real part of dielectric permittivity ε1(λ). (c) Imaginary part of the dielectric permittivity ε2(λ). (d) Variation of the optical loss factor tan(δ). | |
4. Conclusion and future perspective
To conclude, the sol–gel-prepared Mg0.6Ni0.4Cr2O4 (MNCO) and Mg0.6Cu0.4Cr2O4 (MCCO) spinel chromites were confirmed to be pure single-phase samples based on X-ray diffraction analysis. The experimental structural parameters closely matched the theoretical values. FTIR spectroscopy was employed to investigate the elastic properties, revealing that MCCO exhibited lower elastic moduli and Debye temperature compared to MNCO. Furthermore, the band-gap (Eg) and Urbach (Eu) energies were also found to be lower for MCCO than MNCO. A comprehensive analysis of various optical parameters, including refractive index, penetration depth, extinction coefficients, nonlinear optical parameters, optical conductivity, and dielectric constants, was also presented. Our findings indicate that the substitution of Ni2+ and Cu2+ ions in magnesium chromites (MgCr2O4) enhances their visible light absorption. The MNCO and MCCO samples demonstrate significant advantages, including their easy and cost-effective synthesis, lower band-gap energies, good transparency, efficient light absorption, and effective energy conversion. These materials hold potential for various optoelectronic fields, including solar cells, photocatalysis, and nonlinear applications.
While our study has shed light on the promising characteristics of MNCO and MCCO spinel chromites, future research should focus on exploring a broader range of compositions to establish a comprehensive understanding of the structure–property relationships. Additionally, investigating the electrical conductivity and exploring doping strategies and surface modifications will be instrumental in enhancing the optoelectronic properties of these spinel chromites, enabling their integration into various technological applications.
Data availability
The data used to support the findings of this study are available from the corresponding author upon reasonable request.
Conflicts of interest
There are no conflicts to declare.
Acknowledgements
The authors extend their appreciation to Prince Sattam bin Abdulaziz University for funding this research work through the project number (PSAU/2024/01/28786).
References
- S. Ghotekar, S. Pansambal, V. H. Nguyen, S. Bangale, K. Y. A. Lin, H. C. A. Murthy and R. Oza, J. Sol-Gel Sci. Technol., 2023, 105, 176 CrossRef CAS PubMed.
- S. Ghotekar, D. Sanap, K. Y. A. Lin, H. Louis, D. Pore and R. Oza, Res. Chem. Intermed., 2024, 50, 49 CrossRef CAS.
- S. Ghotekar, D. Sanap, K. Patel, Y. Abhale, A. Chauhan, L. Li, D. Kumar, K. Y. A. Lin and R. Oza, Res. Chem. Intermed., 2024, 50, 2199 CrossRef CAS.
- M. L. Bouazizi, S. Hcini, K. Khirouni, F. Najar and A. H. Alshehri, J. Inorg. Organomet. Polym., 2023, 33, 2127 CrossRef CAS.
- Y. Benrighi, N. Nasrallah, T. Chaabane, H. Belkacemi, K. W. Bourkeb, H. Kenfoud and O. Baaloudj, J. Sol-Gel Sci. Technol., 2022, 101, 390 CrossRef CAS.
- S. P. Deshmukh, K. R. Sanadi, R. S. Diggikar, V. B. Koli and A. V. Mali, J. Mater. Sci.: Mater. Electron., 2021, 32, 6810 CrossRef CAS.
- L. Mao, H. Cui, C. Miao, H. An, J. Zhai and Q. Li, J. Mater. Cycles Waste Manag., 2016, 18, 573 CrossRef CAS.
- A. Abbasi, S. M. S. Sajadi, O. Amiri, M. Hamadanian, H. Moayedi, M. Salavati-Niasari and M. M. Beigi, Composites, Part B, 2019, 175, 107077 CrossRef CAS.
- M. A. Kassem, A. A. El-Fadl, A. M. Nashaat and H. Nakamura, J. Alloys Compd., 2019, 790, 853 CrossRef CAS.
- V. Mykhailovych, A. Kanak, Ş. Cojocaru, E. D. C. Arsene, M. N. Palamaru, A. R. Iordan, O. Korovyanko, A. Diaconu, V. G. Ciobanu, G. Caruntu, O. Lushchak, P. Fochuk, Y. Khalavka and A. Rotaru, Catalysts, 2021, 11, 1476 CrossRef CAS.
- A. Fujishima, X. Zhang and D. A. Tryk, Surf. Sci. Rep., 2008, 63, 515 CrossRef CAS.
- J. Xie, H. Wang, M. Duan and L. Zhang, Appl. Surf. Sci., 2011, 257, 6358 CrossRef CAS.
- N. R. Dhineshbabu, V. Rajendran, N. Nithyavathy and R. Vetumperumal, Appl. Nanosci., 2016, 6, 933 CrossRef CAS.
- D. P. Shoemaker and R. Seshadri, Phys. Rev. B: Condens. Matter Mater. Phys., 2010, 82, 214107 CrossRef.
- K. Manjunatha, P. Z. Si, G. V. J. Gowda, A. El-Denglawey and V. J. Angadi, Ceram. Int., 2022, 48, 11654 CrossRef CAS.
- T. Tatarchuk, A. Shyichuk, I. Trawczyńska, I. Yaremiy, A. T. Pędziwiatr, P. Kurzydło, B. F. Bogacz and R. Gargula, Ceram. Int., 2020, 46, 27517 CrossRef CAS.
- R. D. Shannon, Acta Crystallogr. Sect. A Cryst. Phys. Diffr. Theor. Gen. Crystallogr., 1976, 32, 751 CrossRef.
- M. A. Islam, A. K. M. A. Hossain, M. Z. Ahsan, M. A. A. Bally, M. S. Ullah, S. M. Hoque and F. A. Khan, RSC Adv., 2022, 12, 8502 RSC.
- W. Zhang, A. Sun, X. Pan, Y. Han, X. Zhao, L. Yu, Z. Zuo and N. Suo, J. Mater. Sci.: Mater. Electron., 2019, 30, 18729 CrossRef CAS.
- T. Roisnel and J. Rodriguez-Carvajal, LLB-LCSIM, 2003 Search PubMed.
- M. A. Ahmed, S. F. Mansour and M. Afifi, J. Magn. Magn. Mater., 2012, 324, 4 CrossRef CAS.
- K. Pubby, K. V. Babu and S. B. Narang, Mater. Sci. Eng. B., 2020, 255, 114513 CrossRef CAS.
- K. B. Modi, J. Mater. Sci., 2004, 39, 2887 CrossRef CAS.
- Y. Gao, Z. Wang, J. Pei and H. Zhang, J. Alloys Compd., 2019, 774, 1233 CrossRef CAS.
- H. Dhibi, O. Rejaiba, J. Khelifi, M. Nasri, O. Amorri, K. Khirouni and M. L. Bouazizi, J. Sol-Gel Sci. Technol., 2023, 108, 159 CrossRef CAS.
- H. Dhibi, O. Rejaiba, J. Khelifi, M. Nasri, K. Khirouni, M. L. Bouazizi, E. K. Hlil and J. Inorg, Organomet. Polym., 2023, 33, 3984 CrossRef CAS.
- F. Hcini, S. Hcini, M. M. Almoneef, M. H. Dhaou, M. S. Alshammari, A. Mallah, S. Zemni, N. Lefi and M. L. Bouazizi, J. Mol. Struct., 2021, 1243, 130769 CrossRef CAS.
- F. Beshkar, S. Z. Ajabshir and M. S. Niasari, J. Mater. Sci. Mater. Electron., 2015, 26, 5043 CrossRef CAS.
- P. Choudhary and D. Varshney, J. Magn. Magn. Mater., 2018, 454, 274 CrossRef CAS.
- A. A. El-Fadl, A. M. Nashaat and M. A. Kassem, Mater. Res. Express, 2019, 6, 1150a7 CrossRef.
- M. Caglar, S. Ilican, Y. Caglar and F. Yakuphanoglu, Appl. Surf. Sci., 2009, 255, 4491 CrossRef CAS.
- S. R. Johnson and T. Tiedje, J. Appl. Phys., 1995, 78, 5609 CrossRef CAS.
- A. A. Ansari, M. Abushad, M. Arshad, S. Naseem, H. Ahmed, S. Husain and W. Khan, J. Mater. Sci.: Mater. Electron., 2021, 32, 21988 CrossRef CAS.
- S. Husain, A. O. A. Keelani and W. Khan, Nano-Struct. Nano-Objects, 2018, 15, 17 CrossRef CAS.
- O. Rejaiba, K. Khirouni, M. H. Dhaou, B. Alzahrani, M. L. Bouazizi and J. Khelifi, Opt. Quantum Electron., 2022, 54, 315 CrossRef CAS.
- A. M. Alsaad, Q. M. Al-Bataineh, A. A. Ahmad, Z. Albataineh and A. Telfah, Optik, 2020, 211, 164641 CrossRef CAS.
- S. H. Wemple and M. Didomenico, Phys. Rev. B: Solid State, 1971, 3, 1338 CrossRef.
- K. Tanaka, Thin Solid Films, 1980, 66(3), 271 CrossRef CAS.
- A. M. Ismail, M. I. Mohammed and E. G. El-Metwally, Indian J. Phys., 2019, 93, 175 CrossRef CAS.
- F. Z. Rachid, L. H. Omari, H. Lassri, H. Lemziouka, S. Derkaoui, M. Haddad, T. Lamhasni and M. Sajieddine, Opt. Mater., 2020, 109, 110332 CrossRef CAS.
- V. Ganesh, L. Haritha, M. Anis, M. Shkir, I. S. Yahia, A. Singh and S. AlFaify, Solid State Sci., 2018, 86, 98 CrossRef CAS.
- W. Jdey, N. Hamdaoui, S. Hcini, S. Mansouri and L. El Mir, Opt. Mater., 2024, 147, 114666 CrossRef CAS.
|
This journal is © The Royal Society of Chemistry 2024 |
Click here to see how this site uses Cookies. View our privacy policy here.