DOI:
10.1039/D4RA02639J
(Review Article)
RSC Adv., 2024,
14, 14017-14040
Nanoscale strides: exploring innovative therapies for breast cancer treatment
Received
8th April 2024
, Accepted 23rd April 2024
First published on 29th April 2024
Abstract
Breast cancer (BC) is a predominant malignancy in women that constitutes approximately 30% of all cancer cases and has a mortality rate of 14% in recent years. The prevailing therapies include surgery, chemotherapy, and radiotherapy, each with its own limitations and challenges. Despite oral or intravenous administration, there are numerous barriers to accessing anti-BC agents before they reach the tumor site, including physical, physiological, and biophysical barriers. The complexity of BC pathogenesis, attributed to a combination of endogenous, chronic, intrinsic, extrinsic and genetic factors, further complicates its management. Due to the limitations of existing cancer treatment approaches, there is a need to explore novel, efficacious solutions. Nanodrug delivery has emerged as a promising avenue in cancer chemotherapy, aiming to enhance drug bioavailability while mitigating adverse effects. In contrast to conventional chemotherapy, cancer nanotechnology leverages improved permeability to achieve comprehensive disruption of cancer cells. This approach also presented superior pharmacokinetic profiles. The application of nanotechnology in cancer therapeutics includes nanotechnological tools, but a comprehensive review cannot cover all facets. Thus, this review concentrates specifically on BC treatment. The focus lies in the successful implementation of systematic nanotherapeutic strategies, demonstrating their superiority over conventional methods in delivering anti-BC agents. Nanotechnology-driven drug delivery holds immense potential in treating BC. By surmounting multiple barriers and capitalizing on improved permeability, nanodrug delivery has demonstrated enhanced efficacy and reduced adverse effects compared to conventional therapies. This review highlights the significance of systematic nanotherapy approaches, emphasizing the evolving landscape of BC management.
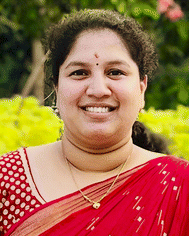 Sruthi Laakshmi Mugundhan | Sruthi Laakshmi Mugundhan is currently a research scholar at SRM Institute of Science and Technology. She obtained her Master's degree in Pharmacy (Pharmaceutics) from SRM College of Pharmacy, SRMIST. Her doctoral research focuses on the formulation development of novel nanocarrier drug delivery system for targeting breast cancer. Under the mentorship of Dr M. Mothilal, her research endeavors to chart new avenues in the treatment of breast cancer. |
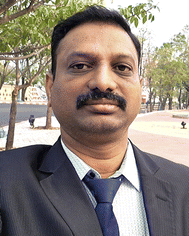 Mothilal Mohan | Dr Mothilal Mohan, B.Pharm, M.Pharm, PhD, is a Professor in the Department of Pharmaceutics at SRM College of Pharmacy, SRMIST, Kattankulathur, Tamil Nadu. His research spans novel formulation development and drug delivery strategies, incorporating nanomedicine for various conditions including targeting cancer, drug delivery to brain, etc. His work aims to optimize nanoparticle properties to enhance drug delivery specificity and therapeutic outcomes while minimizing side effects. Dr Mothilal has published numerous international publications, and serves as a reviewer and committee member for various journals and committees. |
1. Introduction
Cancer can progress through the division of abnormal cells without ending or extending to other body tissues.1 Breast cancer (BC) is one of the predominant types of cancer in women, accounting for nearly 30% of all cancers, and has had a mortality rate of 14% in the last few years in India.2,3 BC is characterized by the expression of estrogen (ER), progesterone (PR) and human epidermal growth factor receptor-2 (HER-2).4 The receptors of BC cells determine the basis of targeted-treatment approaches and play a significant role in the prognosis and treatment of this disease. Like for other types of cancer, the standard therapy for BC includes surgery, chemotherapy and radiotherapy.5 Usually, anti-BC agents are administered via the oral or intravenous (i.v.) route, and the drug must pass through a number of barriers to reach the tumor site. These barriers encompass physical, physiological and biophysical factors.6 The objective of chemotherapy is to use cytotoxic anticancer drugs with or without surgery to compete with the division and development of tumor cells. Increased levels of certain proteins in tumor cells cause resistance to multidrug therapy, decreasing the effectiveness of chemotherapeutic agents. The pathology of BC is highly complicated among all cancers due to endogenous, chronic exposure to diverse intrinsic and extrinsic factors, as well as genetic interconnections.7 Considering these shortcomings in current approaches to cancer treatment, new beneficial solutions must be found. Delivering anticancer drugs to cancer tissues with the help of nondrug delivery in cancer chemotherapy increases bioavailability (in BAs) and greatly minimizes the adverse effects of drugs, which has recently become a research hotspot.8 Unlike standard chemotherapy, cancer nanotechnology disturbs cancer cells via improved permeability and leads to enhanced pharmacokinetic profiles in comparison with conventional therapy.9,10 NPs can provide tumor cells with large doses of therapeutic factors while bypassing normal cells.11 Nano-oncology attempts to remodel the delivery of chemical agents to targeted cancer cells through its merits: (i) it resolves problems with the drug's poor solubility and BA; (ii) it increases the permeability of therapeutic targets to tumor cells and allows for gradual drug release; and (iii) NPs are nontoxic, biodegradable, highly photoluminescent and small (1–100 nm) structures that can carry drugs.12 The application of nanotechnology in cancer treatment requires several nanotechnological tools, and in one review, it is unlikely that all of these tools will be included. This review will therefore focus solely on BC treatment, with emphasis on the successful use of systematic nanotherapy approaches over conventional methods for carrying BC anticancer agents.
2. Prevalence
Breast cancer affects women of all ages after puberty in every country on earth, although incidence rates tend to rise in later years. Despite substantial improvements in treatments for BC, over the span, death rates have remained relatively unchanged for approximately the last 3 decades.13 The incidence of BC increased by 130% between 2008 and 2020, from 1.38 million new cases to 1.67 million in 2012, 2.1 million in 2018, and 2.3 million new cases in 2020.14 In 2022, there were 2.3 million new cases diagnosed and 670
000 deaths worldwide due to breast cancer. Approximately upto 36% of oncological patients are breast cancer survivors.15 In the United States, there are presently over four million women who survived breast cancer. This includes women who have gone through the treatment as well as those who are currently undergoing it.16 An estimated 310
720 new cases of invasive breast cancer will be diagnosed in women in 2024, while there will be 2790 new cases in men. Additionally, there will be 56
500 cases of ductal carcinoma in situ (DCIS) in women in the US.17
Globally, there was a roughly two-fold variation in the overall cumulative risk of breast cancer before 40 years of age. Oceania had the highest risk (0.69%), followed by Europe (0.63%), the Americas (0.53%), Africa (0.49%), and Asia (0.38%). Based on national comparisons across 185 countries, South Korea had the greatest cumulative incidence rate (0.95%), followed by the United States, Canada, and the United Kingdom (0.77%, 0.61%, and 0.61%, respectively), and Guinea (0.13%) had the lowest.18 While the average incidence of breast cancer in Asia before 40 years of age is lowest, there is a six-fold variation across Asian nations.19 These discrepancies may be related to the growing “westernization” of lifestyle practices in some developing nations (e.g., dietary modifications and reduced physical activity) that raise the risk of breast cancer, as well as the absence of public registries with reliable demographic data.20
Based on the latest Globocan (WHO) data, Fig. 1 shows the absolute numbers of breast cancer incidence and mortality in various countries worldwide. In a study conducted by Yuyan et al., in 2023, it was reported that there were 700
660 BC death cases worldwide in 2019 as compared to 380
910 incidents in 1990. Globally in 2050, there will be 1
503
694 death cases of BC (1
481
463 women and 22
231 males).21
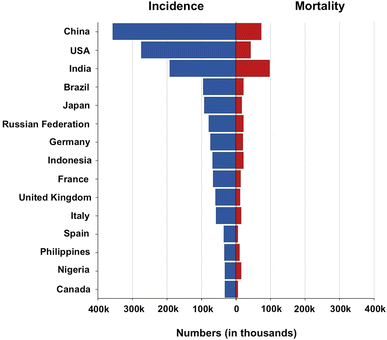 |
| Fig. 1 Absolute numbers of breast cancer incidence and mortality in various countries worldwide in 2022. | |
Additionally, Fig. 2 represents the distribution of BC cases diagnosed by anatomical site in females in the UK during 2016–2018.22
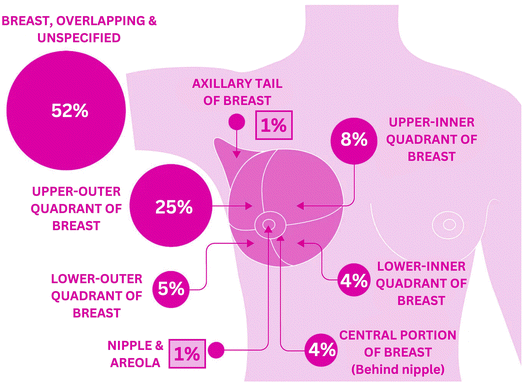 |
| Fig. 2 Percentage distribution of breast cancer incidence based on anatomical site in females (2016–2018, UK). | |
3. Etiology
The potential reasons for a hereditary link to BC include the following: (1) an elevated occurrence of BC in those with a family history of BC, (2) several members of the family affected with BC, and (3) cancer progression consistent with autosomal dominant inheritance. A predisposition gene for autosomal dominant cancer may be inherited and transmitted by both males and females.23 Drug resistance and the potential to metastasize to peripheral organs, such as the lymph nodes, lung, liver and bone, are responsible for the majority of BC deaths.24 A high birth weight is associated with an increased risk of BC, which might be due to changes in leptin, adiponectin, glucose, insulin, and insulin-like growth factor levels, as well as changes in the pregnancy estrogenic environment.25 Certain chemical contaminants, including 2,3,7,8-tetrachlorodibenzo-p-dioxin (TCDD), bisphenol, perfluorooctanesulfonic acid (PFOA), and benzo(a)pyrene, have recently been found to be associated with BC. Styrene, benzene, carbon tetrachloride, and formaldehyde are also substances that have been linked to an increased risk of BC.26
4. Clinical classification
Over the years, the categorization of BC has slowly progressed from merely being figurative based on morphological findings to being comburative, considering clinical characteristics, tissue-based biomarkers and genomes and involving protein expression profiles.27
Fig. 3 shows the histological classification of BC.28 The identification of biomarkers of cancer is among the most effective methods for identifying malignant and even premalignant lesions in the early stages.29 The study of gene expression arrays has contributed to the understanding of many markedly distinct BC subtypes.30 BC can currently be categorized as luminal A, luminal B, HER2-overexpressing, or triple-negative on the basis of genetic testing, histopathology and immunohistochemical analysis, based on the 2011 St. Gallen Consensus.31 As shown in Table 1,32 this classification involves the immunohistochemical analysis of ER and PR expression;, increased expression and/or induction of the HER2 oncogene;, and the use of the cell proliferation marker Ki-67 as a marker index to classify tumor subtypes. Most BCs overexpress the ER, although approximately 25% of BCs overexpress the HER. Nearly 15 percent of breast tumors do not express ER, PR or HER2; this type of tumor is known as triple-negative BC (TNBC) and is deemed the most problematic category of breast tumors.33
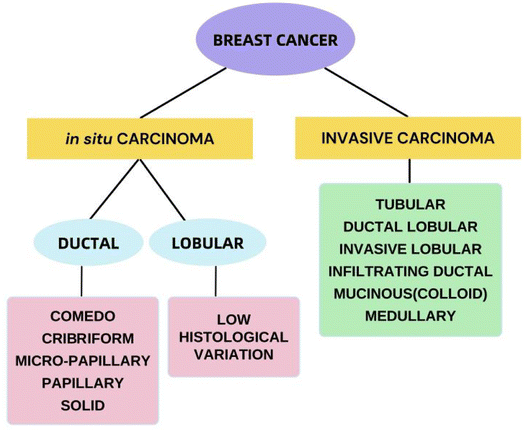 |
| Fig. 3 Histological classification of breast cancer. | |
Table 1 Clinical classification of breast cancer subtypes
Intrinsic subtype |
Clinical definition |
Representation |
Luminal A |
✓ Expresses ER and/or PR |
ER+, PR+ and HER2- |
✓ Does not express HER2 |
Luminal B (2 categories) |
✓ Expresses ER and/or PR |
ER+, PR+ and HER2- |
✓ Does not express HER2- |
✓ High Ki67 expression |
✓ Expresses ER and/or PR |
ER+, PR+ and HER2+ |
✓ Also expresses HER2 |
✓ (Mostly in elderly) |
HER2-overexpression |
✓ Does not express hormone receptors |
ER-, PR- and HER2+ |
✓ Expresses HER2 |
Triple-negative |
✓ Does not express ER, PR and HER2 |
ER-, PR- and HER2- |
5. Nanodrug delivery for breast cancer treatment
All of these nanocarriers have the potential to provide enormous drug delivery potential and are therefore being explored for their potential use.34,35 By nature, nanomedicine can be applied clinically to materials with dimensions less than 100 nm, while devices with dimensions ranging from 100 to 200 nm also function as nanomedicines.36 Nanomedicine was reported to strongly decrease the peak free drug concentration (Cmax) while increasing the area under the curve (AUC) in plasma and tumor tissues. NPs have been shown to have an increased enhanced permeability and retention (EPR) effect.37
Since nanomedicines are favorable for attaining the ‘right objective’ and ‘right exposure,’ they are meant to have an enhanced therapeutic index when compared to standard treatment.38 Highly permeable blood vessels in tumors are assumed to promote the deposition of nanoparticles in tumors.39 There are three possible routes for NPs to enter the interstitial tumor space through tumor blood vessels: through intercellular openings between endothelial cells, through transcellular holes and through endothelial cell fenestrae.40 However, the sizes of these pores differ among several tumor types, tumor microenvironments, and tumor species.41 The potential of altering the various attributes of NPs has transformed them into fruitful therapeutic vehicles for the treatment of cancer. For example, liposomes and polymer micelles enclose drugs inside a center, basically expanding their dissolvability, shielding them from deterioration, and inhibiting their untimely discharge into the circulation system.42 The nanoscopic size of NPs, deregulated vascular structure and upgraded EPR effects aid in preventing RESs, increasing the circulation half-life of drugs in the body and increasing the number of amassed NPs in tumor sites. The superficial presence of polyethylene glycol (PEG) on liposomes and different nanoparticles empowers a prolonged time course of the drug in blood vessels.43 Common types of nanomedicines that are effectively used in BC treatment are listed in Fig. 4. There are ample data on the use of nanotechnology in recent years to resolve the deficiency of chemotherapy in the targeted delivery of BC (Fig. 5). Using nanotechnology, the key features of breast tumors can be exploited to target drugs at the site. This basic strategy allows for increased specificity, boosting the success rate of anticancer chemotherapy. Fig. 6 illustrates the hallmarks of nanotherapy in the treatment of BC. In the last few decades, significant progress has been made in the development of potential nanomedicines for breast cancer treatment. Clinical application of nanomedicine in the treatment of breast cancer is still in the early stages of development, with only a limited number of products having been implemented. However, various approved products available on the market and a few clinical trials in progress are summarized in Tables 2 and 3.
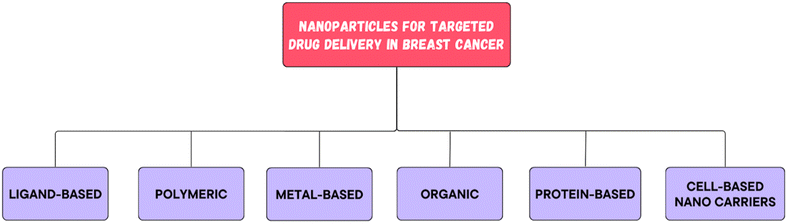 |
| Fig. 4 Types of nanoparticles used in breast cancer therapy. | |
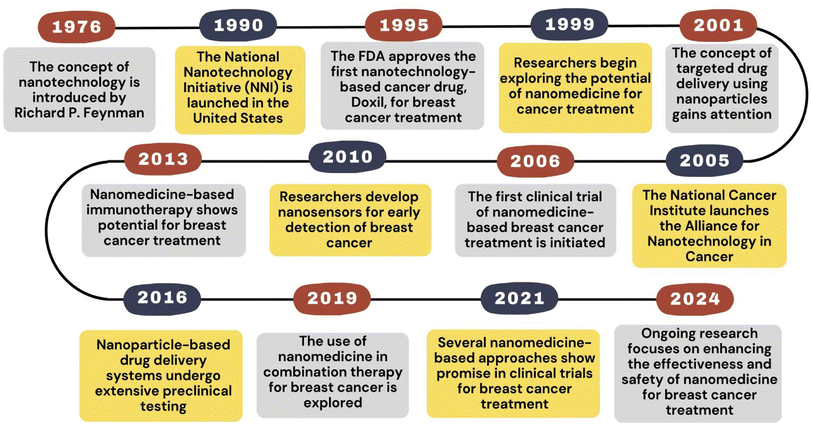 |
| Fig. 5 Historical timeline of nanomedicine for breast cancer treatment. | |
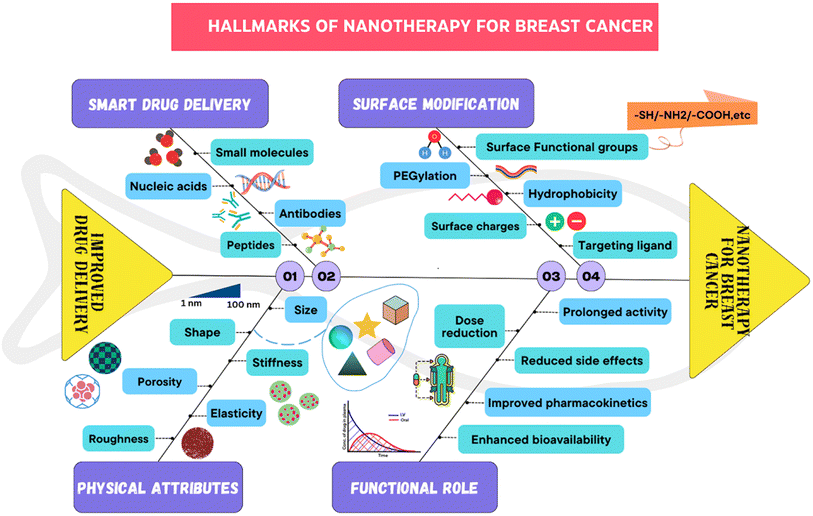 |
| Fig. 6 Hallmarks of nanotherapy in breast cancer. | |
Table 2 Approved nanoparticle-based formulations for breast cancer treatmenta
Product |
Company |
Composition |
Drug |
Approval organization and year |
Reference |
Abbreviations: PEG: polyethylene glycol; MPEG-DSPE: N-(carbonyl-methoxypolyethylene glycol 2000)-1,2-distearoyl-sn-glycero3-phosphoethanolamine sodium salt; HSPC: fully hydrogenated soy phosphatidylcholine; CHO: cholesterol; PC: phosphatidylcholine; NaOH: sodium hydroxide; mPEG: methoxypolyethylene glycol; PDLLA: poly(D,L-lactide); ADC: antibody-drug conjugate; EMA: European medicines agency; FDA: US food and drug administration. |
Doxil® |
Ortho Biotech |
PEGylatyed STEALTH® liposomes composed of MPEG-DSPE, HSPC, CHO |
Doxorubicin |
FDA (1995) |
61 |
EMA (1996) |
Caelyx® |
Schering-Plough |
PEGylated liposomes composed of MPEG-DSPE, HSPC, CHO |
Doxorubicin |
EMA (1996) |
62 |
Myocet® |
Teva Pharmaceutical Industries Ltd |
Liposomes (non-PEGylated) composed of PC, CHO, citric acid, and NaOH |
Doxorubicin |
EMA (2000) |
63 |
Lipusu™ |
Luye Pharmaceutical Co. Ltd |
Liposomes composed of lecithin and cholesterol |
Paclitaxel |
China (2003) |
64 |
Abraxane® |
Abraxis BioScience, Celgene Corporation |
Colloidal suspension without solvent bound to albumin (active substance) in the form of a spherical nanoparticle |
Paclitaxel |
FDA (2005) |
65 |
EMA (2008) |
Genexol®-PM |
Samyang Biopharmaceuticals |
Lyophilized polymeric micellar formulation containing mPEG-PDLLA block copolymers |
Paclitaxel |
South Korea (2007) |
66 |
Lipodox® |
Sun Pharmaceutical Industries Ltd. (SPIL) |
Liposomes with surface-bound mPEG |
Doxorubicin |
FDA (2013) |
67 |
Kadcyla™ |
Genentech, Roche |
Protein-based nanocompound containing ADC |
Ado-trastuzumab emtansine |
FDA and EMA (2013) |
68 |
Pazenir® |
Ratiopharm GmbH |
Albumin-bound nanoparticles as powder for dispersion for infusion |
Paclitaxel |
EMA (2019) |
69 |
Enhertu™ |
AstraZeneca/Daiichi Sankyo |
Humanized monoclonal antibody trastuzumab covalently linked to the topoisomerase I inhibitor deruxtecan (a derivative of exatecan) |
Trastuzumab deruxtecan |
FDA, EMA, China (2019) |
70 |
Trodelvy™ |
Immunomedics |
ADC composed of active metabolite of irinotecan (SN-38) conjugated to a monoclonal antibody targeting trophoblast cell surface antigen 2, an epithelial cell surface antigen |
Sacituzumab govitecan |
FDA (2020) |
71 |
Table 3 Current clinical trials in progress on nanomedicine for breast cancer72–79
Trial ID |
Sponsors |
Trial objective |
Phases |
Interventions |
Type of nano-formulation |
Study country |
Recruitment status |
NCT00629499 |
SCRI Development Innovations, LLC/Celgene Corporation |
To evaluate the efficacy of nanoparticle albumin-bound (nab) paclitaxel/cyclophosphamide in early stage breast cancer(with trastuzumab in HER2-positive patients) |
II |
Nab paclitaxel, cyclophosphamide, transtuzumab |
Nanoparticle albumin-bound |
USA |
Completed |
NCT01644890 |
Nippon Kayaku Co., Ltd |
To compare NK105 vs. paclitaxel in patients with metastatic or recurrent breast cancer |
III |
NK105 |
Micellar nanoparticle |
Japan, Korea, Taiwan |
Completed |
NCT03671044 |
Jina Pharmaceu-ticals Inc |
To evaluate the efficacy and safety of nanosomal docetaxel lipid suspension in triple negative breast cancer patients |
III |
Nanosomal docetaxel lipid suspension, Taxotere® |
Nanosomal lipid suspension |
USA, India |
Recruiting |
NCT04137653 |
Shengjing Hospital |
To compare the therapeutic effect of nab-P with solvent-based paclitaxel in TNBC patients |
III |
Nab-paclitaxel, carboplatin |
Nanoparticle albumin-bound |
China |
Recruiting |
NCT04917900 |
West China Hospital |
To evaluate the Pyrotinib in combination with albumin-bound paclitaxel and trastuzumab to neoadjuvant therapy efficacy and safety of Her2-positive early or locally advanced breast cancer |
II |
Pyrotinib combined with albumin-bound paclitaxel and trastuzumab |
Nanoparticle albumin-bound |
China |
Recruiting |
NCT06143553 |
Shanghai Yizhong Pharmaceutical Co., Ltd |
To compare the clinical efficacy and safety of paclitaxel polymeric micelles for injection with TPC in HER2- metastatic breast cancer subjects |
III |
Paclitaxel, eribulin mesilate, capecitabine, gemcitabine HCl, vinorelbine tartrate, nab-paclitaxel |
Polymeric micelles |
China |
Recruiting |
NCT06199895 |
Liu Huang |
To evaluate the efficacy and safety of paclitaxel polymeric micelles for injection for the treatment of patients with advanced breast and other cancer types |
II |
Paclitaxel polymeric micelles for injection |
Polymeric micelles |
China |
Recruiting |
NCT05949021 |
Mridula George, MD |
To evaluate the efficacy, safety, and exploratory measures of liposomal doxorubicin and carboplatin combination therapy in the adjuvant setting for early stage triple negative breast cancer patients |
II |
Combination of liposomal doxorubicin |
Liposome |
USA |
Recruiting |
5.1. Lipid-based nanoparticles
Liposomal nanoparticles (LNPs) were first described in 1964 as spherical vesicle microparticles comprising single or multiple bilayered membrane structures with an aqueous center core.44 Lipophilic and hydrophilic medications may be incorporated due to the distinct bilayer compositions of the liposomes. Additionally, amphiphilic drugs, such as vincristine and DOX,45 may be encapsulated in the aqueous inner center of LNPs, which has been shown to reduce the cardiotoxicity of DOX compared to that of the unenclosed type.46 Considering the advantages of delivering amphiphilic drugs, it is important to note that the dimensions of LNPs are relatively larger (less than 50 nm), which can be overcome by coating LNPs with polymers. In liposomes, phospholipids are arranged in a bilayer pattern and thus result in a more soluble and stable drug formulation.47 This shows that encapsulating the drug effectively reduces toxicity due to the nontarget distribution of the drug. The biocompatibility of liposomes is optimal, and they are advantageous because of their good biodegradability, low immunogenicity, and low toxicity.48 Chemotherapeutic drugs in the form of LNPs were shown to be effective in clinical trials for treating BC with liposomal formulations, such as the Doxil liposome preparation.49 Doxil is the first anticancer nanomedicine used clinically and comprises a liposome encapsulating DOX. Doxil's PEGylated formulation reduces drug levels in blood without impacting the drug's anticancer activity because it enhances retention time and inhibits premature nanoformulation clearance.50 DOX is usually used for the successful treatment of Kaposi sarcoma and refractory breast and ovarian cancer (USFDA). Among several NPs used for cancer chemotherapy, LNPs are the first nanoparticle platform approved for systemic delivery of chemotherapeutics and for improved further use. There are a number of well-established LPN-based formulations, which include solid lipid nanoparticles (SLNs), nanostructured lipid carriers (NLCs) and many other types of liposomal formulations, which are discussed below.
5.1.1. Solid lipid nanoparticles. SLNs are colloidal particles made of biodegradable, biocompatible and physiological lipids measuring approximately 50 to 1000 nm in size based on the preparation methods and composition; they are solid in nature at room and body temperatures and are safe for use51 SLNs are made of solid fat, surfactants and active pharmaceutical ingredients and are generated from natural or synthetic lipids.12 These NPs are used as an effective approach for improving the oral BA concentration of less aqueous soluble therapeutics due to their many advantages, such as ease of preparation, enhanced drug stability, increased drug content, efficient delivery of drugs and improved long-term stability.52 Compared with those of both MCF-7 and MCF-10A cells, SLNs harboring tamoxifen citrate and camptothecin exhibited improved efficacy.53 The evoked apoptotic mechanism in RES-loaded SLNs significantly represses on BC growth.54
5.1.2. Nanostructured lipid carriers. Nanostructured lipid carriers (NLCs) are improved SLNs identified in the late 1990s; these NLCs contain a mixture of solid (fat) and liquid (oil) lipids that form a matrix.55 A few drawbacks of SLNs, including the ejection of the enclosed drug during storage and decreased loading of the therapeutic agent, could be effectively overcome by the addition of NLCs, which provide improved drug loading and minimal drug loss during storage.56 NLCs can be strongly assimilated by tumor cells and exhibit many favorable conditions, such as high drug encapsulation capacity, controlled drug delivery, improved drug stability and effortless extensive production.57 The poor oral BA content and solubility of quercetin were overcome by incorporating the drug into NLCs, the encapsulation efficiency was approximately 95%, and prolonged delivery of quercetin allowed significant cell death in MCF-7 and MDA-MB-231 cells. Additionally, drugs such as doxorubicin (DOX) and paclitaxel (PTX) were found to be effective against the MCF-7 and SK-OV3 cell lines and their multidrug resistant variants when enclosed in NLCs.58
5.1.3. pH-sensitive liposomes. Due to their ischemic nature and pH of approximately 5 to 6.5, the unusually acidic tumor microenvironment (TME) of neoplastic tissues can be used to establish pH-responsive nanodrug delivery systems since the pH of healthy tissues and blood falls in the range of approximately 7 to 7.4.59 After the discovery of pH-sensitive drug delivery methods in 1980, pH-sensitive liposomal formulations were regarded as trustworthy methods for increasing drug availability at tumor sites (intracellular/extracellular). When used in conjunction with a ligand, this system can increase targeting and clinical effectiveness even more.60 These types of liposomes bear a negative charge and remain steady at neutral pH, but upon attaining acidic conditions at the tumor site, they exhibit pH-stimulated drug delivery via disruption of the lipid bilayer due to various molecular modifications in the lipid portion, such as protonation, deprotonation and decreased stability.80 pH-responsive liposomes of PTX showed rapid release of the drug at low pH, which was more effective in in vitro and in vivo BC models.81 As a result of pH variations, the ionization degree of pH-sensitive components, such as biopolymeric materials or phospolipids, changes due to their sensitivity to low pH, enabling them to accept or donate protons.82 A good example is phosphatidyl dioleoyl phosphoethanolamine (DOPE), which is a natural liposome that has a dual layered structure and a hexagonal structure at neutral and acidic pH values, respectively, leading to membrane rupture and loss of stability.83
5.1.4. Thermosensitive liposomes. The use of thermosensitive liposomes (TSLs) is based on the concept that drug delivery can be facilitated by localized hyperthermia at the BC tumor site by combining the event of superheating with the merits of the liposomal structure. Traditional TSLs containing temperature-sensitive components and liposomes modified with temperature-sensitive polymers are two broad categories of TSLs.84 Extrinsic heat acts as a stimulus for drug delivery at the tumor site, which improves drug localization, reduces systemic side effects and offers integrated thermochemotherapy to tumor tissues.85 Docetaxel (DTX)-loaded liposomes significantly extinguished tumors at a rate 1.3-fold greater than that of tumors treated with drugs alone during in vivo animal studies of human BC in nude mice.86 The pioneering TSL formulation was developed in 1978 by Yatvin and others using DPPC and distearoyl phosphatidylcholine (DSPC) at a molar ratio of 3
:
1.87 Compared with those of nonthermosensitive liposomes, the growth of tumors from patients treated with cisplatin was markedly lessened, as observed in 2 individual tumor models (i.e., MDA-MB-231 and MDA-MB-436).88
5.1.5. Long circulatory liposomes. Generally, liposomes are prone to uptake by accelerated blood clearance and the reticuloendothelial system (RES) in the body after administration, and rapid opsonization results in easy elimination of liposomes. Liposomes have been investigated in a variety of ways to overcome their RES uptake and maintain them in circulation, including changing their size or altering their surface. To extend their circulation time, second-generation liposomes have a tweaked surface made up of glycoprotein, oligo- and polysaccharides, and synthetic polymers.89 Since the majority of liposomes accumulate in the liver and spleen and thereby might increase drug intoxication and side effects in these 2 organs, this issue can be efficiently mastered by formulating long-circulatory/circulating liposomes that are improved by water-soluble polymers such as PEG.90 Once long-term drug-charged circulating liposomes accumulate in cancer tissue, the drug can be liberated concurrently and regularly.91 Anticancer studies of BC cell lines (MDA-MB-231, MCF-7, and SKBR-3) revealed that treatment with liposomes containing glucoevatromonoside derivatives markedly decreased cell survival, and the formulations improved the targeting of these cancer cells.92
5.1.6. Other liposomal nanodrug delivery systems. LPNs present various benefits when modified into self-micro/nanoemulsified drug delivery systems (SMEDDSs/SNEDDSs) and several other formulations other than the abovementioned liposomal preparations, including enhanced biodegradability, biocompatibility, and targeting of therapeutics.93 Payne, a British scholar in 1986, reported the existence of proliposomes for the first time to resolve difficulties such as aggregation, leakage of drugs and oxidative and hydrolytic degradation of phospholipids during storage. Proliposomes are formulated under pressure to minimize agitation, which results in a free-flowing powder by slowly mixing the drug with the liposomal material on the surface of a suitable carrier.94 It is known as a proliposome since the liposomal suspension has yet to be formed and is generated by the addition of water or an aqueous buffer prior to use. Based on the type of phospholipid incorporated in the preparation, proliposomes can be categorized into mixed micellar, liquid crystal and dry granular proliposomes. Curcumin, due to its anti-inflammatory, antioxidant and antitumor properties, has been reported to be use in BC and certain other cancers, yet its therapeutic application is limited owing to its low water solubility, instability, high metabolism and low abundance of BAs. Cationic PEGylated niosomes containing curcumin combined with PTX were produced by Alemi and coworkers to achieve an improved synergistic anticancer effects.95 Remarkably, many other studies have proposed that hybrid liposomes loaded with dipalmitoyl phosphatidylcholine (DPPC) and polyoxyethylene dodecyl ether can cause programmed cell death in various cancer cell lines, including human BC cells, lymphoma cells, and lung cancer cells.96 Immunoliposomes are another type of novel drug delivery tool that includes a combination of liposomes and targeted antibodies. Alteration of liposomes by monoclonal antibodies or their fragments results in immunoliposomes, which can selectively recognize and attach to antigens on the surface of the target site through immunoglobulins at the surface or their fragments, thereby facilitating drug accumulation at the targeted tumor site. These liposomes play a vital role in tumor targeting by penetrating cells either via endocytosis-derived fusion or by direct fusion with the plasma membrane, which decreases the aggregation of drugs in normal tissues, enhances drug efficacy and prevents drug toxicity.97 Compared with DOX-loaded liposomes with PEG-linked surfaces, anti-HER-2 DOX-loaded immunoliposomes are capable of improving the antitumor effects of drugs in xenograft models of BC.98 Third-generation targeted liposomes are prepared by changing to their surface by means of appropriate ligands and can be utilized for active and passive targeting of tumors. In passive targeting, they exhibit increased drug concentrations within the tumor owing to the EPR effect. Functionalized liposomes may carry the medication to a particular location, such as a cell organelle, because of specific receptor-selective associations with various forms of targeting ligands, such as peptides and antibodies.99 Hypoxia-responsive liposomes are primed by the injection of nitroimidazole derivative into the liposomal membrane. These patients exhibited a decrease in the hypoxic metabolism of the tumor and stimulated drug delivery due to liposomal degeneration. Such drug delivery results in oxygen-dependent delivery of the medication (DOX), which was verified by confocal laser scanning microscopy and near-infrared imaging. Liposomes sensitive to hypoxia showed particular cytotoxic effects on hypoxic tumor cells. These findings indicate that hypoxia-sensitive liposomes may be a successful path to cancer treatment.100 In addition to the above-described LPNs, photosensitive liposomes,101 magnetic liposomes,102 and ligand-modified liposomes103 include a few other liposomal drug delivery systems that are left undiscussed.
5.2. Polymeric nanoparticles
Polymer-based or polymeric NPs (PNPs) are made of natural or synthetic polymers and are self-assembled micelles approximately 100 nm in size. Owing to their enhanced durability, these NPs are extremely specialized in nature and exhibit positive rheological behavior. The release of chemotherapeutic agents is triggered after administration by degradation of the polymeric layer.104 The chemotherapeutic agent may be applied to the surface of PNPs through surface adsorption, chemical conjugation or encapsulation centered on the PNPs to target the tumor site.105 PNPs have the potential to charge active substances. In this way, the intracellular distribution of the active drug increases, and the drug is protected from degradation in a rigid matrix.106 In many trials, drugs entrapped against BC with polymeric micelles reportedly demonstrate greater efficacy than other drugs.107 Apart from using natural products, including cellulose and chitosan, as polymers,108 polymers synthetically prepared by nanoprecipitation, emulsification and salting-out methods are also employed for more specific biomedical applications of PNPs.109 PNPs of DOX HCl conjugated with hyaluronic acid and hydroxyethyl chitosan markedly increased the cellular uptake of the anticancer agents studied in HER2+ BC cell lines (MDA-MB- 453, MDA-MB- 435, and MCF-7).110 The various polymeric platforms used for anticancer nanotherapy include solid polymeric NPs, polymeric micelles, polymer conjugates, dendrimers, polymersomes, polyplexes, nanofibers and polymer–lipid hybrid systems.111 Owing to the improved stability offered by these NPs, the chances of RES accumulation and delay in biodegradation are marked as drawbacks. PNPs are efficiently modified to facilitate various benefits of targeting anticancer agents for BC by incorporating biodegradable polymers, immunotherapeutic agents and multiple chemotherapeutic agents.109
5.2.1. Biodegradable polymeric nanoparticles. In nanocarrier platforms, biodegradable polymers are chosen due to their modified release properties, greater solubility and permeability, ability to release BAs, improved encapsulation ability and minimal toxicity. Chitosan, gelatin, polylactic acid (PLA), poly D,L-lactic-co-glycolic acid (PLGA), polycaprolactone, and polyalkyl-cyanoacrylate are widely used biodegradable polymers.112 A hydrophobic anticancer drug will be placed in the central hydrophobic bridging area inside the biodegradable PNP network, which undergoes surface modification through physical adsorption of hydrophilic polymer side chains. After accumulation within the tumor site, the NPs release the enclosed medication just after biodegradation and diffusion processes.113 pH-sensitive biodegradable polymers such as polyethylene oxide (PEO) become readily soluble at a pH below 6.5, enabling tumor-specific delivery of therapeutics such as PTX by encapsulating the drug and releasing it into the acidic breast TME.114 Biodegradable polymers offer extra benefit from oral chemotherapy, as patients may undergo chemotherapy immediately at home and have a better quality of life in the late cancer period. Oral chemotherapy frequently provides palliative care.115 In 2018, Farrokhi and his team constructed a DNAzyme that targets the expression of the BC cell oncogene c-Myc using a cyclodextrin nanocarrier. The formulation blocked the development of SMC cells and the cell line MCF-7 by 30–80%.116
5.2.2. Polymeric micelles. Polymeric micelles are favorable supramolecular DDSs consisting of amphiphilic block copolymers with hydrophobic centers designed for aqueous insoluble drugs and hydrophilic layers loaded with molecules of hydrophilic drugs. Because of its semisolid hydrophobic center consisting of a biodegradable polymer, the polymer micelle measures a diameter of approximately 10–100 nm and is thus suitable for promoting many water-insoluble anticancer treatments.102 Genexol-PM, developed in Korea by Samyang Biopharm, is a micellar nanopolymer formulation of PTX indicated for the treatment of BC and non-small cell lung carcinoma (NSCLC) in South Korea.117,118 It comprises an amphiphilic diblock copolymer, monomethoxy poly(ethylene glycol)-block-poly(D,L-lactide) (mPEG-PDLLA) and PTX. Using an active targeting pathway, Baidya and his colleagues developed chrysin-charged folate-conjugated PF127-F68 mixed micelles to enhance oral BA and anticancer activity against human BC cells in 2019. The folate receptors in BC are activated by this combination. This technique was developed to investigate anticancer behavior in cell lines both in vitro and in vivo. The formulation revealed substantially higher Cmax and AUC0-t and improved anticancer action (5-fold) in an MTT assay (MCF-7).119 Furthermore, in a human BC model, BT474 xenograft mouse, bortezomib (BTZ)-loaded micelles exerted a better antitumor impact than free BTZ and suppressed BTZ in vivo hepatotoxicity.120
5.2.3. Dendrimers. Dendrimers are the tiniest nanomaterials developed with dimensions of only 1.9 nm for G1 and 4.4 nm for G4, rendering their use simpler in some unique circumstances.121 Dendrimers are composed of polymeric star-shaped molecules that are continuously strongly branched and have a rigid 3D structure. They show 3 distinct sections, namely, a central core, branches and an external surface, with multiple surface operational groups.122 The most intensively researched group of materials is the polyamidoamine (PAMAM) dendrimer family, but the diversity of the building blocks is progressively increasing.123 Dendrimers bearing propylene imine (PPI) monomers are commonly employed in the delivery of drugs as well as nucleic acids in BC treatment, and they have also been equipped with ligands to target anti-BC drugs. Said and others synthesized a new water-soluble poly(propylene imine) dendrimer (PPI) modified with 4-sulfo-1,8-naphthalimid units (SNID) and its related structural monomer analog (SNIM) by simple synthesis.124 Gupta and others coupled 5th generation PPI dendrimers with FA to bind folate receptors and successfully achieved DOX delivery.125 These DOX-loaded FA-PPI dendrimers displayed faster release of DOX and greater cellular uptake by MCF-7 cells. Curcumin dendrimers (derived from Curcuma longa) have greater water solubility and have the potential to effectively induce apoptosis in BC cells.126 Thermosensitive branched OEG water soluble dendrimers could resolve the limitations of the currently used dendrimers. OEG dendrimers combined with gemcitabine (GEM) demonstrated beneficial tumor invasion and aggregation because of the longest peripheral PEG segments, resulting in marginally greater anticancer effects than those of GEM-linked PAMAM.127
5.2.4. Multifunctional polymeric nanoparticles. Multifunctional PNPs are formulated for the simultaneous delivery of two or more anticancer agents, such as trastuzumab, incorporated with PTX NPs for targeted BC chemotherapy.128 These codelivery systems have several benefits, including delivering chemotherapeutic agents without harmful adjuvants, promoting synergistic effects, diminishing side effects of the drugs employed and achieving targeted delivery of cancer therapy129 Previously, a multifunctional dendrimer coupled with fluorescein isothiocyanate was formulated for scanning, FA as a tumor marker for targeting cancer cells that overexpress folate receptors and PTX as a chemotherapy medication.130
5.3. Inorganic nanoparticles
In addition to PNPS, metal-based NPs provide considerable versatility for drug distribution and diagnostics. Metallic NPs, including gold (Au NPs) and silver (Ag NPs), and magnetic NPs, including superparamagnetic iron oxide NPs (SPIONs) and quantum dots (QDs), play a helpful and effective role in cancer treatment owing to their low toxicity, fair size-to-volume ratio and outstanding thermal stability. These features have lead to improved targeting, silencing of genes, drug release and analytical assays for diagnosis. Almost all metallic NPs have the same framework, which consists of an electronic, magnetic and optical center and a shield that is primarily an organic surface coating.131 Additionally, metallic NPSs could be used for integrating optical and magnetic imaging in conjunction with fluorescent active molecules.132
5.3.1. Gold and silver nanoparticles. Gold NPs were first identified by Michael Faraday.133 These NPs have dimensions of approximately 130 nm and are stable, with good biocompatibility and no toxicity.134 Herceptin®, a humanized anti-human HER-2 antibody conjugated with PAMAM dendrimers consisting of Au NPs and gadolinium (Gd), may be utilized for the early diagnosis and therapy of human HER2+ BC.135 Sun et al. engineered DOX-loaded Au NPs that are capable of transporting the medication efficiently to BCSCs and substantially enhancing antitumor efficiency.136 Ghosh et al. documented the use of Gloriosa superba tuber extract to prepare gold and silver nanoparticles and reported that AgNPs and AuNPs have excellent anticancer effects on MCF-7 cells.137 An American study by Nima and coworkers recently described the ability of nanotherapeutics (DOX) to treat BC and prostate cancer cells utilizing silver-decorated gold nanorods.138 Hibiscus rosasinensis petal extracts and chitosan were used to produce antioxidants such as vitamin C- and vitamin E-encapsulated NPs and Ag NPs. Chitosan polymers can bind to each other and are biocompatible, biodegradable and cationic in nature. These NPs were proven to be significantly hemocompatible and had a strong encapsulation performance of almost 76%. In fact, slightly greater antitumor behavior toward BC cell lines (MCF-7) was observed.139 Gold NPs can be directed toward BC cell mitochondria and may be successful at triggering apoptotic cell death and activating tumor cell damage, which may be useful in BC photothermal therapy.140
5.3.2. Magnetic nanoparticles and SPIONs. Magnetic NPs can be generated by means of a magnetic field. Magnetic materials such as nickel, cobalt and iron are used to construct these NPs.141 These NPs are being utilized in MRI with the advancement of nanotechnology, owing to their ability to bind to the target site and thus support drug release technology.142 SPIONs are approximately 1 to 100 nm in size and are composed of an inner magnetic core made of magnetite (Fe3O4) or maghemite (γ-Fe2O3). Compared with magnetite, the latter is the most suitable because of its minimal chance of causing Fe(III) toxicity in the body.143 The magnetic core is often shielded by a water-soluble stabilizing layer such as polymers that enables targeted biomolecules to be delivered to the target site. The most widely used biopolymers for stabilization include polysaccharides, PEG, dextran, alginate and polyacrylic acid (PAA).144 SPIONs are concurrently employed in tissue regeneration, immunoassays, magnetic field cell imaging and drug delivery.145 In 2018, Nosrati and others generated methotrexate-conjugated L-lysine-coated Fe2O3 magnetic NPs for BC treatment. This research was performed on BC cells from MCF-7 cells. The preparation had a negative zeta potential and a particle size < 100 nm, indicating major anticancer effects.146 Poller et al. further contrasted the influence of 3 separate forms of SPIONs on BC cells, which differed in scale, form, zeta potential and surface coating. Comparisons of the cellular absorption, magnetic properties and cytotoxic effects of the SPIONs are shown. Among the three kinds of SPIONs involved in BC therapy, namely, dextran-coated (SPIONDEX), lauric acid-coated (SPIONLA) and SPIONLA, which include human serum albumin (SPIONLA-HSA), the SPIONLA demonstrated the maximum cell absorption and cell cytotoxicity against BC cells.147
5.3.3. Quantum dots. Quantum dots (QDs) play a vital role in BC therapy due to their significant optical properties. QDs are fluorescent NPs with a size of approximately 2 to 10 nm that are composed of a core of hundreds to thousands of atoms of elements belonging to groups II and VI (for instance, cadmium, technetium, zinc and selenide) or group III (Eg, tantalum) and V (Eg, indium).148 QDs serve as a powerful diagnostic tool for BC in addition to therapeutics due to their ability to emit narrow symmetrical peaks with minimal overlap between the spectra. QDs that are emitted at different wavelengths were studied in an analysis of the cell lines MCF-7 and BT-474, and it was observed that these two cell lines produce specific amounts of the following 5 biomarkers: ER, PR, EGFR, mTOR and HER2. The QDs were therefore paired with the main antibodies of these protein biomarkers and used for identification by simultaneous quantitative and multicolor detection.149 Nevertheless, in vivo, the use of QDs in imaging and treatment is restricted by the toxic impact of heavy metal cores.150 Additionally, they require coating surfaces with polymers or multilayer ligand shells to provide considerable water solubility owing to their hydrophobicity. The QDs of the somatostatin analog veldoreotide were used to biomap and reduce tumor development in patients with BC and are currently in a phase I clinical trial.151
5.3.4. Mesoporous silica nanoparticles. Mesoporous silica NPs (MSNs) have attracted enormous interest in the field of nanotherapeutic drug delivery because of their unique physicochemical properties.152 Additionally, MSNs possess a large surface area and pore volume and can possibly alter the pore size in addition to having an easily modifiable surface, enabling them to act as effective tools for drug delivery and imaging.153 NPs bearing either medications or genetic material are contained inside the pores of MSNs, whose shape and scale are designed to reduce RES sequestration and improve tumor absorption by increasing vessel width, strong adhesion and cell assimilation.154 A study published by Tsai and others revealed the incorporation of an anti-HER2/neu monoclonal antibody in the form of NPs utilizing green fluorescent MSNs as a DDS to selectively target BC cells.155 Wu et al. produced Bcl-2 siRNA-packed mesoporous polymer nanospheres in 2019 to bind to the BC FA receptor. The NPs were administered competently to BC cells and strongly inhibited the expression of the Bcl-2 mRNA, which is involved in BC cell death.156 Conversely, one significant downside of MSNs is that they lack the capacity to penetrate into the tumor mass.157
5.4. Organic nanoparticles
Organic NPs have been widely utilized and studied for various oncologic purposes in recent years for diagnosis and cancer therapy. Several researchers have explored hybrid organic nanocarriers for drug delivery and scanning and have reported successful decreases in tumor size, efficient concentrations of drugs at active sites and influential diagnoses of cancers.
5.4.1. Carbon-based nanoparticles. Carbon nanomaterials such as fullerene, graphene, carbon nanotubes and carbon dots (CDs) have attracted increased interest in BC therapy because of their special physicochemical, optical and biological properties.158 Fullerenes, carbon nanotubes (CNTs) and graphenes may be fabricated to detect and manage cancer on the surface with imaging tools and drugs, and such carbon-derived NPs possess various desirable qualities, such as a compact scale, large specific surface region, robust useable surface groups, favorable biocompatibility, low toxicity, and distinct optical and thermal characteristics.159
5.4.1.1. Carbon nanotubes. CNTs are fullerene allotropes with thin, long, cylindrical, branched, hollow-framed hydrophobic carbon atom constructs that are insoluble in water and other organic solvents, and their toxicity in a biological medium is the main limiting factor. CNTs possess walls made of layers of graphene rolled at a precise angle and are known as single-walled (SWNT) or multiwalled (MWNT) nanotubes based on single or multiple graphene sheets.26 The amine-functional fullerene nanostructure has outstanding fluorescence properties and has the potential to penetrate in vitro BC cells easily (MCF-7). Fiorillo reported that breast cancer stem cells (BCSCs) are prone to carbon nanotube therapy based on graphene oxide and have good potential to target BCSCs.160 Hence, CNTs are successful transporters for drug distribution. However, the processing of CNTs cannot be performed easily. CNTs also have some disadvantages, such as solubility and biodegradability.161
5.4.1.2. Carbon dots. CDs were introduced in 2004 as a type of carbon-based NP, and in the early period of their development, major studies focused on photoluminescence (PL) by means of several synthetic methods, starting materials and surface modifications.162 The application of CDs in BC treatment occurred for the first time in 2013. Hsu et al. published a study of green tea-derived CDs in 2013, which highlighted cancer cell suppression activity163 using the cancer cell lines MCF-7, MDA-MB-231, and HeLa (human cervical carcinoma) cells. As the accumulation of CDs increased, the percentage cell viability decreased to approximately 20, 18, and 68%, respectively, which was attributed to H2O2 and reactive oxygen species (ROS) generation and reflected the significant suppression of BC cell lines. Further research on BC therapy was subsequently conducted in 2018 by Kong et al. by combining CDs with DOX through electrostatic interactions, and the conjugates generated greater cell absorption and anticancer effects on MCF-7 cells than did free DOX.164
5.4.2. Protein-based (viral) nanoparticles. The use of protein-based NPs is another promising flexible approach for targeted medication delivery. Viral NPs are those corresponding to the protein envelopes or capsids of viruses that are biocompatible and biodegradable, which makes them suitable drug carriers.165 The viral NPs derived from plant viruses and bacteriophages are particularly effective because they exhibit low risks of pathogenicity and undesired side effects in the human body.166 Nonetheless, viral NPs are noninfectious because they lack a viral genome. Potato virus X (PVX) was bonded and propagated to athymic mice harboring human MDA-MB-231 BC xenografts by Le et al. in 2017, and PVX-DOX treatment reduced tumor development.167 Thus, the use of PVX, a form of viral NP that originates from plants, paves the way for malignant therapy using viral NPs. In addition to PVX, influenza viral NPs altered by the securing of glycophosphatidylinositol (GPI) and the HER2 antigen by protein transfer likewise resulted in increased defense against HER2-expressing tumor cell proliferation in a murine BC model, and updated GPI-HER2 influenza viral NPs were added to the development of HER2-specific IgG and improved HER2-specific Th1-type immunity compared with GPI-HER2 vaccination alone.168
5.5. Cell-based nanocarriers
The bulk of the cells used in these systems have been established to have potential in tumor areas, such as the center of tumor hypoxia, so they are generally welcomed. The use of host cells as NP-releasing vehicles to address challenges in anticancer drug targeting for BC is thought to be a very useful method. Monocytes were stocked in vitro with gold nanoshells and then leached onto spheroids of BC tumors with nanoshell-coated macrophages. Furthermore, these experiments showed the use of macrophages for targeted phototherapy focused on the spheroid design of BC tumors.169 Although conventional DDSs, such as liposomes and polymeric NPs, have been used as carriers of anticancer and antifungal agents in recent years, certain negative features, such as biocompatibility and long-term safety issues, prevail.170 These limitations can be efficiently solved by the use of exosome-based drug delivery systems, which exhibit great physiological stability. Since exosomes have a hydrophobic nucleus, they promote the storage of water-soluble drugs. There are three distinct groups of extracellular vesicles, namely, microvesicles (MVs), ranging from 100 to 1000 nm in diameter; apoptotic bodies, ranging from 1 to 5 μm in diameter; and exosomes, ranging from 30 to 100 nm.3 Exosomes are efficient transporters of large molecules such as proteins. For instance, surface modifications develop with exosomes produced from immature dendritic cells in mice, and these cells are modified to establish the Lamp2b exosomal membrane protein, which is fused with the iRGD peptide unique to ac integrin.171 The electroporation-isolated exosomes were assembled with DOX, and the efficiency of encapsulation was estimated to be 20%. These engineered exosomes were intravenously administered to rats, after which DOX entered the tumor, and as a result, tumor development was inhibited.172 DOX-treated exosomes were used to treat BC and TNBC for successful targeting and delivery to αv integrin-positive BC cells and thereby showed a reduction in immunogenicity and toxicity.173
6. Targeting potential of nanoformulations for breast cancer treatment
Nanotechnology nanoformulations show significant promise for transforming the treatment of breast cancer since they can precisely target cancer cells while limiting toxicity and off-target effects. Improved pharmacokinetics, increased solubility of poorly water-soluble drugs, extended circulation time, and the possibility of targeted drug delivery to tumor sites through passive and active mechanisms are just a few benefits these nanoscale drug delivery systems have over traditional therapies.174
One of the key advantages of nanoformulations is their ability to passively target cancerous tissues through the enhanced permeability and retention (EPR) effect. This phenomenon takes advantage of the leaky vasculature and poor lymphatic drainage commonly found in tumors, allowing nanoparticles to preferentially accumulate within the tumor microenvironment.175 By encapsulating chemotherapeutic agents within nanoparticles, drug concentrations at the tumor site can be increased while reducing systemic exposure and associated side effects. Additionally, surface modification of nanoparticles with targeting ligands such as antibodies or peptides enables active targeting of specific molecular markers overexpressed on the surface of breast cancer cells. This targeted approach enhances the selectivity of drug delivery, further improving therapeutic efficacy while minimizing damage to healthy tissues.176
Zhang et al. 2023 developed PLGA shell PANP nanoparticles with perfluoropentane (PFP), Paclitaxel (PTX), and anti-miR-221 inhibitor and monitored them in vivo using ultrasound-triggered PFP vaporization. RAW-PANPs enriched in tumor tissues after injection into tumor-burden mice. Ultrasound cavitation explosion released PTX in the tumor. The release of anti-miR-221 improved tumor cell PTX sensitivity. Therefore, RAW-PANPs suppressed TNBC cell proliferation in vitro and tumor growth and progression in vivo. The treatments did not affect the heart, kidneys, or liver and the study developed a macrophage-carried, ultrasound-triggered, cancer cell-targeted chemotherapeutic system and a miRNA-based approach to improve cancer cell drug sensitivity to treat treatment-resistant TNBC patients. This novel study used macrophages to transport nanoparticles to tumors and ultrasonically break them to release miRNA and PTX.177 In another recent study by Li et al. 2023, autopilot biohybrids (Bif@BDC-NPs) which deliver doxorubicin to tumors were developed. The study involved preparation of albumin-encapsulated DOX nanoparticles (BD-NPs) coated with chitosan for breast cancer chemotherapy and anaerobic Bifidobacterium infantis (Bif) as self-propelled motors form this combination. Bif@BDC-NPs accurately attach hypoxic tumor tissue and promote drug accumulation at the tumor location, causing tumor cell death due to Bif's anaerobic characteristics. Therefore, Bif@BDC-NPs, a bacteria-driven oral drug delivery method, bypasses several physiological barriers and has significant potential for precision solid tumor treatment.178
Polydopamine NPs were formulated to deliver PTX and Trastuzumab to HER2+ breast tumors. PDA NPs, despite not being loaded with taxane or anti-HER2 antibody, have shown remarkable antitumor activity in vitro in HER2+ conventional cell cultures and breast tumor spheroids.179 Encapsulation of diosgenin in PLGA nanoparticles, coated with folic acid-chitosan, yielded a stable formulation with potent anticancer effects against TUBO breast cancer cells. In vitro and in vivo studies demonstrated dose-dependent inhibition of tumor growth and apoptosis induction.180
These studies highlight the potential of nanoformulations to enhance the efficacy of breast cancer treatment by improving targeting specificity, therapeutic outcomes, and diagnostic capabilities. Nanoformulations are as miniature missiles that target breast cancer either by becoming trapped in leaking tumor vessels, which increases the concentration of the substance at the site (passive targeting) or by modifying their surface, they are able to target cancer cells while causing minimal damage to healthy tissue (active targeting). This focused strategy provides promise regarding improved treatment results and diminished adverse effects. Continued research efforts in this field are critical to translating these promising nanoformulation strategies into clinically effective therapies for breast cancer patients, ultimately advancing personalized medicine and improving survival rates.
7. Challenges in the development of nanomedicine for BC treatment
Nanotechnology has great potential for breast cancer treatment, but many barriers must be addressed before its use in clinical practice.
Ensuring the success of nanopharmaceuticals is hampered by economic considerations. The production process and raw material costs are relatively high, which drives up the price of their products. For instance, the production of medications like Paclitaxel and Doxorubicin, which are free pharmaceuticals, is significantly less expensive than that of Abraxane™ and Doxil™.181 It is projected that the complete commercialization process for a novel nanodrug will take around 10–15 years and cost approximately $1 billion. Therefore, in order to justify their higher cost in comparison to traditional therapies, the clinical benefits of nanomedicines must be evident.182 Given that even minute changes in the manufacturing process can have a substantial impact on properties like size, shape, composition, drug loading and release, biocompatibility, toxicity, and in vivo outcome, the scalable and controlled manufacturing of nanomedicines under good manufacturing practices (GMP) conditions poses special challenges. As a result, several techniques should be used to characterize nanomedicine products batch by batch.183
Furthermore, it is crucial to address unique concerns regarding nanomedicines designed for human use, including sterility. Consequently, one of the greatest obstacles in the development of nanomedicines is to identify a method of sterilization that preserves the physicochemical properties and stability of the therapeutic molecules without compromising them. Particular care must be taken when developing nanomedicines utilizing biological molecules, such as proteins, because of their extreme vulnerability to degradation by sterilization methods.184 Particular attention must be paid to the endotoxin contamination in this context. Endotoxins can cause severe health problems, and contamination with endotoxins causes the failure of over thirty percent of nanoformulations during early preclinical development. Consequently, the endotoxin content of nanomedicines must be meticulously assessed utilizing suitable methodologies. Additionally, it is difficult to characterize the storage and stability characteristics (shelf life) of nanomedicine products. Additionally, the characteristics of nanomedicines can be modified through storage in lyophilized form or aqueous solutions.185
It is necessary to evaluate the toxicological effects of nanomaterials; however, certain toxic effects remain largely unknown. The derived toxicological data, with the exception of a few observations that have been reported thus far, are inconsistent and contradictory. The implementation of guidelines to standardize preclinical nanomedicine research is obligatory. Such guidelines would facilitate modeling, quantitative comparisons, reproducibility, and cost-effectiveness, thereby enhancing the utility, effectiveness, and safety of nanoformulations.186 Furthermore, they would aid in the rapid translation of basic research into clinical practice. Furthermore, regulatory concerns play a significant role in the advancement of technologies utilized for the characterization and quality monitoring of nanopharmaceuticals. Regulatory determinations concerning therapeutic nanomedicines are established through the subjective evaluation of risks and benefits by an individual; this tedious procedure can cause regulatory setbacks for nanomedicine products.187 Integrating the efforts of international consortia comprised of clinicians, academics, pharmaceutical companies, and regulatory authorities should be among these actions taken in order to improve the clinical efficacy and patient outcomes of these nanopharmaceuticals that target cancer.188 Numerous nanoformulations, ranging from inorganic to organic nanoparticles, with a variety of formulation and production techniques, great flexibility, and control over size and shape, have been studied in recent publications. They are loaded with active compounds and several chemotherapeutics, and they have been functionalized to enable targeted therapy.198 This inadequate rate of clinical translation, however, works in opposition to the positive outcomes of preclinical research. In fact, there are currently very few drugs based on nanotechnology that are approved for use in clinical trials. In order to enhance clinical trials, Van der Meel and colleagues proposed using smart patient stratification techniques in cancer nanomedicine. These techniques include imaging-based tumor accumulation to select trial candidates and probes and procedures to evaluate the tumor microenvironment.199
Furthermore, they demonstrated that the meticulous rational design of pharmacological combination regimens will enhance the pharmacokinetic and/or pharmacodynamic advantages and suggested the implementation of creative techniques for designing modular (pro)drugs and drug-delivery systems, in addition to library screening, in order to increase the likelihood of successful preclinical testing of formulations. Performance, translation, and exploitation of nanomedicine will all be enhanced by these smart strategies.200
8. Insights on personalized protein corona in BC nanotherapy
The successful translation of nanoscale therapies for breast cancer treatment hinges not only on their intrinsic properties but also on their interactions with biological systems, notably the formation of a protein corona upon exposure to biological fluids. The protein corona, composed of biomolecules such as proteins, lipids, and other biomolecules, plays a pivotal role in determining the fate and functionality of nanoparticles within the body. Recent advancements have highlighted the significance of personalized protein coronas in modulating the safety, efficacy, and therapeutic outcomes of nanoscale materials in cancer therapy.201
8.1. Formation and composition of personalized protein corona
Upon introduction into physiological environments, nanoparticles rapidly adsorb proteins from the surrounding milieu, forming a dynamic protein corona layer. The composition of this corona is highly variable and influenced by factors such as nanoparticle surface properties, size, shape, and surface charge, as well as the unique biological identity of the individual. Recent studies have revealed substantial inter-individual variability in protein corona composition, emphasizing the need for personalized approaches in nanomedicine.202
8.2. Impact on nanoparticle–biological interactions
The protein corona serves as a molecular interface between nanoparticles and biological entities, influencing their interactions with cells, tissues, and physiological barriers. In the context of breast cancer, the protein corona can modulate nanoparticle uptake by cancer cells, intracellular trafficking, and subsequent therapeutic responses. Additionally, the protein corona may affect nanoparticle pharmacokinetics, biodistribution, and clearance profiles, thereby shaping their overall therapeutic efficacy and safety profiles.203
8.3. Implications for precision medicine in breast cancer treatment
The recognition of personalized protein coronas as critical determinants of nanoscale therapy outcomes underscores the importance of integrating precision medicine approaches into the development and optimization of nanoparticle-based breast cancer treatments. By accounting for individual variations in protein corona composition, it may be possible to tailor nanotherapeutic strategies to optimize treatment responses and minimize adverse effects in breast cancer patients.204
8.4. Challenges and future directions
Despite significant progress in understanding the role of personalized protein coronas in nanoscale therapies, several challenges remain. These include the development of robust predictive models for protein corona formation, strategies to mitigate inter-individual variability, and the translation of personalized nanomedicine approaches into clinical practice. Future research efforts should focus on addressing these challenges to realize the full potential of personalized protein corona-based approaches in breast cancer treatment.205
9. Conclusions and future prospects
BC chemotherapy in the form of novel nanotechnology will continue to benefit amidst the increasing challenges faced in tumor treatment. Nanomedicine has a wide range of advantages for cancer patients. It is important to note that the inclusion of nanomaterials results in better stability, improved solubility and controlled release kinetics for chemotherapeutic agents. Nanoformulations have the potential to overcome the significant limitations of conventional BC therapy, as summarized in Table 4. This review clearly describes the various drug delivery systems established for BC treatment and highlights the importance of nanotherapy (Fig. 7). However, the complicated aspects of nanomedicine use have yet to be fully resolved, and additional research is needed. Scientists are constantly working to develop new nanoformulations that require adequate evidence that nanoformulations are therapeutically much better, abundantly stable and rational.
Table 4 Summary of challenges in standard breast cancer treatment and how nanomedicine can help to overcome these challenges
Limitations of standard breast cancer therapy |
Highlights of nanotechnology in BC chemotherapy |
Reference |
Lack of selectivity in drug targeting for BC |
Both passive as well as active targeting increases drug concentration at tumor site and diminishes toxic drug levels in noncancer sites |
189 |
Improper reach of drugs to metastatic organs including brain, bone and lungs |
Nanotherapy has in-born features of brain and bone penetration |
190 |
Unfavorable PK properties like short half-life and rapid clearance |
Approaches like PEGylation can be applied to extend the retention of the drug |
191 |
Influence of the drugs or excipients leading to dose-limiting toxicity, say, surfactants or organic cosolvents |
Solvent-free, surfactant-free nanoformulations can be formulated for controlled-release of drugs |
192 |
Cellular level drug resistance, for instance, increase in efflux transport of drug |
Endocytosis takes place as a result of passive/active transport; some nano preprations inhibit efflux mechanism and codelivery of agents that promote drug resistance |
193 |
TME level drug resistance like low pH, hypoxia, etc. |
Possibility of targeting TME and use of stimulus-sensitive approaches |
194 |
Challenges in destroying cancer stem cells |
Cancer stem cells can be directly targeted |
195 |
Inefficient pharmaceutical characteristics of the drugs such as low water-solubility, poor in vivo stability |
Drug solubilization can be achieved easily by means of nanotechnology and can even protect the unstable drugs |
196 |
Suboptimal dosing schedule, particularly in case of using multiple drugs as combinations |
Dosing schedule can be consciously optimized and delivery of multiple drugs is made better |
197 |
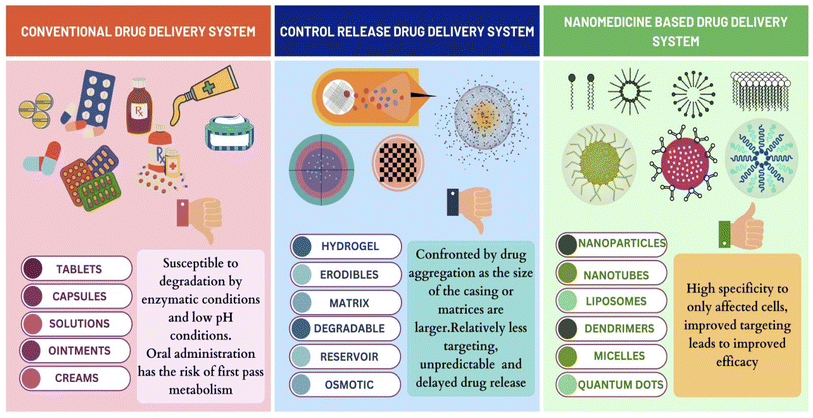 |
| Fig. 7 Comparison of drug delivery in breast cancer. | |
Abbreviations
ABC | Accelerated blood clearance |
API | Active pharmaceutical ingredient |
AUC | Area under the curve |
BA | Bioavailability |
BC | Breast cancer |
BCSC | Breast cancer stem cells |
BTZ | Bortezomib |
CDK | Cyclin-dependent kinase |
CDs | Carbon dots |
Cmax | Peak free drug concentration |
CNT | Carbon nanotubes |
DDS | Drug delivery system |
DEX | Dextran |
DMPC | Dipalmitoylphosphatidylcholine |
DOPE | Dioleoyl phosphoethanolamine |
DOX | Doxorubicin |
DPPC | Dipalmitoyl phosphatidylcholine |
DSPC | Distearoyl phosphatidylcholine |
EPR | Enhanced permeability and retention |
ER | Estrogen receptor |
FA | Folic acid |
Gd | Gadolinium |
GEM | Gemcitabine |
GI | Gastro intestinal |
GPI | Glyco phosphatidyl inositol |
GSTE | Gloriosa superba tuber extract |
HeLa | Human cervical carcinoma |
HER2/ERBB2/EGFR | Human epidermal growth factor receptor/oncogene 2 |
HSA | Human serum albumin |
i.v | Intravenous |
IgG | Immunoglobulin G |
LA | Lauric acid |
LNP | Liposomal nanoparticles |
MAb | Monoclonnal antibody |
MRI | Magnetic resonance imaging |
MSN | Mesoporous silica nanoparticles |
MV | Microvesicles |
nab | Nanoparticle-albumin bound |
neu | Neutrophil |
NLC | Nanostructured lipid carriers |
NP | Nanoparticle |
NSCLC | Non-small-cell lung carcinoma |
PAA | Poly acrylic acid |
PAMAM | Polyamidoamine |
PARP | Poly ADP ribose polymerase |
PEG | Poly ethylene glycol |
PEO | Polyethylene oxide |
PFOA | Perfluorooctanesulfonic acid |
PLA | Poly lactic acid |
PLGA | Poly D,L-lactic-co-glycolic acid |
PNP | Polymeric nanoparticle |
PPI | Propylene imine |
PR | Progesterone receptor |
PTX | Paclitaxel |
PVX | Potato virus X |
QDs | Quantum dots |
RES | Reticuloendothelial system |
ROS | Reactive oxygen species |
SLN | Solid lipid nanoparticles |
SMEDDS | Self-micro emulsifying drug delivery system |
SNEDDS | Self-nano emulsifying drug delivery system |
SPIONs | Super paramagnetic iron oxide nanoparticles |
SWNT/MWNT | Single-walled/multi-walled carbon nanotubes |
TCDD | 2,3,7,8-Tetrachlorodibenzo-p-dioxin |
Tmax | Time taken to reach peak free drug concentration |
TME | Tumor microenvironment |
TNBC | Triple negative breast cancer |
TSL | Thermo-sensitive liposomes |
USFDA | United States food and drug administration |
WHO | World health organization |
Author contributions
Sruthi Laakshmi Mugundhan designed the graphical illustrations and contributed to the writing, conceptualization and publishing process; Mothilal Mohan contributed to the supervision, drafting of the work, revision of the intellectual content and final approval of the manuscript. All the authors read and approved the final manuscript.
Conflicts of interest
There are no conflicts to declare.
References
- S. Nasirizadeh and B. Malaekeh-Nikouei, Solid lipid nanoparticles and nanostructured lipid carriers in oral cancer drug delivery, J. Drug Delivery Sci. Technol., 2020, 55, 101458 CrossRef CAS.
- V. Kulothungan, T. Ramamoorthy, K. Sathishkumar, R. Mohan, N. Tomy, G. J. Miller and P. Mathur, Burden of female breast cancer in India: estimates of YLDs, YLLs, and DALYs at national and subnational levels based on the national cancer registry programme, Breast Cancer Res. Treat., 2024, 4, 1 Search PubMed.
- A. M. Allahverdiyev, E. Parlar, S. Dinparvar, M. Bagirova and E. Ş. Abamor, Current aspects in treatment of breast cancer based of nanodrug delivery systems and future prospects, Artif. Cells, Nanomed., Biotechnol., 2018, 46(sup3), 755–762 CrossRef PubMed.
- S. K. Pindiprolu, P. T. Krishnamurthy, P. K. Chintamaneni and V. V. Karri, Nanocarrier based approaches for targeting breast cancer stem cells, Artif. Cells, Nanomed., Biotechnol., 2018, 46(5), 885–898 CrossRef CAS PubMed.
- P. Y. Liyanage, S. D. Hettiarachchi, Y. Zhou, A. Ouhtit, E. S. Seven, C. Y. Oztan, E. Celik and R. M. Leblanc, Nanoparticle-mediated targeted drug delivery for breast cancer treatment, Biochim. Biophys. Acta, 2019, 1871(2), 419–433 CAS.
- S. Augustine, J. Singh, M. Srivastava, M. Sharma, A. Das and B. D. Malhotra, Recent advances in carbon based nanosystems for cancer theranostics, Biomater. Sci., 2017, 5(5), 901–952 RSC.
- B. Zucchetti, A. K. Shimada, A. Katz and G. Curigliano, The role of histone deacetylase inhibitors in metastatic breast cancer, Breast, 2019, 43, 130–134 CrossRef PubMed.
- S. Ibrahim, T. Tagami, T. Kishi and T. Ozeki, Curcumin marinosomes as promising nano-drug delivery system for lung cancer, Int. J. Pharm., 2018, 540(1–2), 40–49 CrossRef CAS PubMed.
- A. D. Bangham, Liposomes: the Babraham connection, Chem. Phys. Lipids, 1993, 64(1–3), 275–285 CrossRef CAS PubMed.
- R. Pushpalatha, S. Selvamuthukumar and D. Kilimozhi, Nanocarrier mediated combination drug delivery for chemotherapy–A review, J. Drug Delivery Sci. Technol., 2017, 39, 362–371 CrossRef CAS.
- B. Bahrami, M. Hojjat-Farsangi, H. Mohammadi, E. Anvari, G. Ghalamfarsa, M. Yousefi and F. Jadidi-Niaragh, Nanoparticles and targeted drug delivery in cancer therapy, Immunol. Lett., 2017, 190, 64–83 CrossRef CAS PubMed.
- J. L Arias, B. Clares, M. E Morales, V. Gallardo and M. A Ruiz, Lipid-based drug delivery systems for cancer treatment, Curr. Drug Targets, 2011, 12(8), 1151–1165 CrossRef PubMed.
- World Health Organization, Fact Sheets, Breast Cancer, https://www.who.int/news-room/fact-sheets/detail/breast-cancer, (accessed April 2024) Search PubMed.
- N. A. Lakkis, R. M. Abdallah, U. M. Musharrafieh, H. G. Issa and M. H. Osman, Epidemiology of Breast, Corpus Uteri, and Ovarian Cancers in Lebanon With Emphasis on Breast Cancer Incidence Trends and Risk Factors Compared to Regional and Global Rates, Cancer Control, 2024, 31, 10732748241236266 CrossRef PubMed.
- N. Tzenios, M. E. Tazanios and M. Chahine, The impact of BMI on breast cancer–an updated systematic review and meta-analysis, Medicine, 2024, 103(5), e36831 CrossRef CAS PubMed.
- K. M. Cuthrell and N. Tzenios, Breast Cancer: Updated and Deep Insights, Int. Res. J. Oncol., 2023, 6(1), 104–118 Search PubMed.
- American Cancer Society, Cancer Facts and Figures 2024, American Cancer Society, Atlanta, GA, 2024, https://www.cancer.org/research/cancer-facts-statistics/all-cancer-facts-figures/2024-cancer-facts-figures.html, (accessed April 2024) Search PubMed.
- J. W. Zhu, P. Charkhchi, S. Adekunte and M. R. Akbari, What is known about breast cancer in young women?, Cancers, 2023, 15(6), 1917 CrossRef CAS PubMed.
- A. Migowski, P. Nadanovsky and C. Manso de Mello Vianna, Harms and benefits of mammographic screening for breast cancer in Brazil, PLoS One, 2024, 19(1), e0297048 CrossRef CAS PubMed.
- V. Sopik, International variation in breast cancer incidence and mortality in young women, Breast Cancer Res. Treat., 2021, 186, 497–507 CrossRef CAS PubMed.
- Y. Xu, M. Gong, Y. Wang, Y. Yang, S. Liu and Q. Zeng, Global trends and forecasts of breast cancer incidence and deaths, Sci. Data, 2023, 10(1), 334 CrossRef PubMed.
- Cancer Research UK, https://www.cancerresearchuk.org/health-professional/cancer-statistics/statistics-by-cancer-type/breast-cancer/incidence-invasive#heading-Three, (accessed April 2024) Search PubMed.
- S. R. Grobmyer, G. Zhou, L. G. Gutwein, N. Iwakuma, P. Sharma and S. N. Hochwald, Nanoparticle delivery for metastatic breast cancer, Nanomed. Nanotechnol. Biol. Med., 2012, 8, S21–S30 CrossRef CAS PubMed.
- L. Hilakivi-Clarke, Maternal exposure to diethylstilbestrol during pregnancy and increased breast cancer risk in daughters, Breast Cancer Res., 2014, 16, 1 CrossRef PubMed.
- M. Afzal, K. S. Alharbi, N. K. Alruwaili, F. A. Al-Abassi, A. A. Al-Malki, I. Kazmi, V. Kumar, M. A. Kamal, M. S. Nadeem, M. Aslam and F. Anwar, Nanomedicine in treatment of breast cancer–A challenge to conventional therapy, Semin. Cancer Biol., 2021, 69, 279–292 CrossRef PubMed.
- H. J. Han, C. Ekweremadu and N. Patel, Advanced drug delivery system with nanomaterials for personalised medicine to treat breast cancer, J. Drug Delivery Sci. Technol., 2019, 52, 1051–1060 CrossRef CAS.
- G. K. Malhotra, X. Zhao, H. Band and V. Band, Histological, molecular and functional subtypes of breast cancers, Cancer Biol. Ther., 2010, 10(10), 955–960 CrossRef PubMed.
- H. K. Sajja, M. P. East, H. Mao, Y. A. Wang, S. Nie and L. Yang, Development of multifunctional nanoparticles for targeted drug delivery and noninvasive imaging of therapeutic effect, Curr. Drug Discovery Technol., 2009, 6(1), 43–51 CrossRef CAS PubMed.
- Q. Zhan, B. Liu, X. Situ, Y. Luo, T. Fu, Y. Wang, Z. Xie, L. Ren, Y. Zhu, W. He and Z. Ke, New insights into the correlations between circulating tumor cells and target organ metastasis, Signal Transduction Targeted Ther., 2023, 8(1), 465 CrossRef CAS PubMed.
- A. Goldhirsch, W. C. Wood, A. S. Coates, R. D. Gelber, B. Thürlimann and H. J. Senn, Strategies for subtypes—dealing with the diversity of breast cancer: highlights of the St Gallen International Expert Consensus on the Primary Therapy of Early Breast Cancer 2011, Ann. Oncol., 2011, 22(8), 1736–1747 CrossRef CAS PubMed.
- F. K. Al-Thoubaity, Molecular classification of breast cancer: a retrospective cohort study, Ann. Med. Surg., 2020, 49, 44–48 CrossRef PubMed.
- S. Jin and K. Ye, Targeted drug delivery for breast cancer treatment, Recent Pat. Anti-Cancer Drug Discovery, 2013, 8(2), 143–153 CrossRef CAS PubMed.
- R. M. Zaid, P. Mishra, A. S. Noredyani, S. Tabassum, Z. Ab Wahid and A. M. Sakinah, Proximate characteristics and statistical optimization of ultrasound-assisted extraction of high-methoxyl-pectin from Hylocereus polyrhizus peels, Food Bioprod. Process., 2020, 123, 134–149 CrossRef CAS.
- Z. Zhu, Q. Wu, X. Di, S. Li, F. J. Barba, M. Koubaa, S. Roohinejad, X. Xiong and J. He, Multistage recovery process of seaweed pigments: investigation of ultrasound assisted extraction and ultra-filtration performances, Food Bioprod. Process., 2017, 104, 40–47 CrossRef CAS.
- R. X. Zhang, H. L. Wong, H. Y. Xue, J. Y. Eoh and X. Y. Wu, Nanomedicine of synergistic drug combinations for cancer therapy–strategies and perspectives, J. Controlled Release, 2016, 240, 489–503 CrossRef CAS PubMed.
- L. E. Gerlowski and R. K. Jain, Microvascular permeability of normal and neoplastic tissues, Microvasc. Res., 1986, 31(3), 288–305 CrossRef CAS PubMed.
- S. Ashton, Y. H. Song, J. Nolan, E. Cadogan, J. Murray, R. Odedra, J. Foster, P. A. Hall, S. Low, P. Taylor and R. Ellston, Aurora kinase inhibitor nanoparticles target tumors with favorable therapeutic index in vivo, Sci. Transl. Med., 2016, 8(325), 325ra17 Search PubMed.
- P. Yingchoncharoen, D. S. Kalinowski and D. R. Richardson, Lipid-based drug delivery systems in cancer therapy: what is available and what is yet to come, Pharmacol. Rev., 2016, 68(3), 701–787 CrossRef CAS PubMed.
- H. Hashizume, P. Baluk, S. Morikawa, J. W. McLean, G. Thurston, S. Roberge, R. K. Jain and D. M. McDonald, Openings between defective endothelial cells explain tumor vessel leakiness, Am. J. Pathol., 2000, 156(4), 1363–1380 CrossRef CAS PubMed.
- S. K. Hobbs, W. L. Monsky, F. Yuan, W. G. Roberts, L. Griffith, V. P. Torchilin and R. K. Jain, Regulation of transport pathways in tumor vessels: role of tumor type and microenvironment, Proc. Natl. Acad. Sci. U. S. A., 1998, 95(8), 4607–4612 CrossRef CAS PubMed.
- G. Mattheolabakis, B. Rigas and P. P. Constantinides, Nanodelivery strategies in cancer chemotherapy: biological rationale and pharmaceutical perspectives, Nanomedicine, 2012, 7(10), 1577–1590 CrossRef CAS PubMed.
- E. Blanco and M. Ferrari, Emerging nanotherapeutic strategies in breast cancer, Breast, 2014, 23(1), 10–18 CrossRef PubMed.
- V. P. Torchilin, Liposomes as targetable drug carriers, Crit. Rev. Ther. Drug Carrier Syst., 1985, 2(1), 65–115 CAS.
- A. Fritze, F. Hens, A. Kimpfler, R. Schubert and R. Peschka-Süss, Remote loading of doxorubicin into liposomes driven by a transmembrane phosphate gradient, Biochim. Biophys. Acta, 2006, 1758(10), 1633–1640 CrossRef CAS PubMed.
- N. L. Boman, D. Masin, L. D. Mayer, P. R. Cullis and M. B. Bally, Liposomal vincristine which exhibits increased drug retention and increased circulation longevity cures mice bearing P388 tumors, Cancer Res., 1994, 54(11), 2830–2833 CAS.
- G. Zheng, M. Zheng, B. Yang, H. Fu and Y. Li, Improving breast cancer therapy using doxorubicin loaded solid lipid nanoparticles: synthesis of a novel arginine-glycine-aspartic tripeptide conjugated, pH sensitive lipid and evaluation of the nanomedicine in vitro and in vivo, Biomed. Pharmacother., 2019, 116, 109006 CrossRef CAS PubMed.
- M. Kapoor, S. L. Lee and K. M. Tyner, Liposomal drug product development and quality: current US experience and perspective, AAPS J., 2017, 19, 632–641 CrossRef CAS PubMed.
- D. R. Khan, The use of nanocarriers for drug delivery in cancer therapy, J. Cancer Sci. Ther., 2010, 2(3), 58–62 CrossRef CAS.
- Y. C. Barenholz, Doxil®—The first FDA-approved nano-drug: lessons learned, J. Controlled Release, 2012, 160(2), 117–134 CrossRef CAS PubMed.
- M. Abrishami, M. Abrishami, A. Mahmoudi, N. Mosallaei, M. Vakili Ahrari Roodi and B. Malaekeh-Nikouei, Solid lipid nanoparticles improve the diclofenac availability in vitreous after intraocular injection, J. Drug Delivery, 2016, 1368481 Search PubMed.
- S. Cai, Q. Yang, T. R. Bagby and M. L. Forrest, Lymphatic drug delivery using engineered liposomes and solid lipid nanoparticles, Adv. Drug Delivery Rev., 2011, 63(10–11), 901–908 CrossRef CAS PubMed.
- C. Y. Acevedo-Morantes, M. T. Acevedo-Morantes, D. Suleiman-Rosado and J. E. Ramírez-Vick, Evaluation of the cytotoxic effect of camptothecin solid lipid nanoparticles on MCF7 cells, Drug Delivery, 2013, 20(8), 338–348 CrossRef CAS PubMed.
- W. Wang, L. Zhang, T. Chen, W. Guo, X. Bao, D. Wang, B. Ren, H. Wang, Y. Li, Y. Wang and S. Chen, Anticancer effects of resveratrol-loaded solid lipid nanoparticles on human breast cancer cells, Molecules, 2017, 22(11), 1814 CrossRef PubMed.
- N. Naseri, H. Valizadeh and P. Zakeri-Milani, Solid lipid nanoparticles and nanostructured lipid carriers: structure, preparation and application, Adv. Pharm. Bull., 2015, 5(3), 305 CrossRef CAS PubMed.
- Z. R. Huang, S. C. Hua, Y. L. Yang and J. Y. Fang, Development and evaluation of lipid nanoparticles for camptothecin delivery: a comparison of solid lipid nanoparticles, nanostructured lipid carriers, and lipid emulsion, Acta Pharmacol. Sin., 2008, 29(9), 1094–1102 CrossRef CAS PubMed.
- S. Selvamuthukumar and R. Velmurugan, Nanostructured lipid carriers: a potential drug carrier for cancer chemotherapy, Lipids Health Dis., 2012, 11, 1–8 CrossRef PubMed.
- L. Zhang, J. M. Chan, F. X. Gu, J. W. Rhee, A. Z. Wang, A. F. Radovic-Moreno, F. Alexis, R. Langer and O. C. Farokhzad, Self-assembled lipid− polymer hybrid nanoparticles: a robust drug delivery platform, ACS Nano, 2008, 2(8), 1696–1702 CrossRef CAS PubMed.
- S. Karve, A. Bandekar, M. R. Ali and S. Sofou, The pH-dependent association with cancer cells of tunable functionalized lipid vesicles with encapsulated doxorubicin for high cell-kill selectivity, Biomaterials, 2010, 31(15), 4409–4416 CrossRef CAS PubMed.
- S. R. Paliwal, R. Paliwal and S. P. Vyas, A review of mechanistic insight and application of pH-sensitive liposomes in drug delivery, Drug Delivery, 2015, 22(3), 231–242 CrossRef CAS PubMed.
- Y. Lee and D. H. Thompson, Stimuli-responsive liposomes for drug delivery, Wiley Interdiscip. Rev.: Nanomed. Nanobiotechnol., 2017, 9(5), e1450 Search PubMed.
- B. K. Kashyap, V. V. Singh, M. K. Solanki, A. Kumar, J. Ruokolainen and K. K. Kesari, Smart Nanomaterials in Cancer Theranostics: Challenges and Opportunities, ACS Omega, 2023, 8(16), 14290–14320, DOI:10.1021/acsomega.2c07840.
- Y. A. Tereshkina, T. I. Torkhovskaya, E. G. Tikhonova, L. V. Kostryukova, M. A. Sanzhakov, E. I. Korotkevich, Y. Y. Khudoklinova, N. A. Orlova and E. F. Kolesanova, Nanoliposomes as drug delivery systems: safety concerns, J. Drug Targeting, 2022, 30(3), 313–325 CrossRef PubMed.
- G. M. Jensen and D. F. Hodgson, Opportunities and challenges in commercial pharmaceutical liposome applications, Advanced drug delivery reviews, 2020, 154, 2–12 CrossRef PubMed.
- F. Wang, M. Porter, A. Konstantopoulos, P. Zhang and H. Cui, Preclinical development of drug delivery systems for paclitaxel-based cancer chemotherapy, J. Controlled Release, 2017, 267, 100–118 CrossRef CAS PubMed.
- M. J. Nirmala, U. Kizhuveetil, A. Johnson, G. Balaji, R. Nagarajan and V. Muthuvijayan, Cancer nanomedicine: a review of nano-therapeutics and challenges ahead, RSC Adv., 2023, 13(13), 8606–8629 RSC.
- D. Wu, M. Si, H. Y. Xue and H. L. Wong, Nanomedicine applications in the treatment of breast cancer: current state of the art, Int. J. Nanomed., 2017, 16, 5879–5892 CrossRef PubMed.
- H. Chou, H. Lin and J. M. Liu, A tale of the two PEGylated liposomal doxorubicins, OncoTargets Ther., 2015, 13, 1719–1720 Search PubMed.
- A. I. Fraguas-Sánchez, I. Lozza and A. I. Torres-Suárez, Actively targeted nanomedicines in breast cancer: from pre-clinal investigation to clinic, Cancers, 2022, 14(5), 1198 CrossRef PubMed.
- C. H. Li, V. Karantza, G. Aktan and M. Lala, Current treatment landscape for patients
with locally recurrent inoperable or metastatic triple-negative breast cancer: a systematic literature review, Breast Cancer Res., 2019, 21, 1–4 CrossRef CAS PubMed.
- T. Siddiqui, P. Rani, T. Ashraf and A. Ellahi, Enhertu (Fam-trastuzumab-deruxtecan-nxki)–Revolutionizing treatment paradigm for HER2-Low breast cancer, Ann. Med. Surg., 2022, 82, 104665 Search PubMed.
- A. Mandapati and K. E. Lukong, Triple negative breast cancer: approved treatment options and their mechanisms of action, J. Cancer Res. Clin. Oncol., 2023, 149(7), 3701–3719 CrossRef PubMed.
- Nanoparticle Albumin-Bound (Nab) Paclitaxel/Cyclophosphamide in Early-Stage Breast Cancer, NCT00629499, https://clinicaltrials.gov/study/NCT00629499, (accessed April 2024) Search PubMed.
- A Phase III Study of NK105 in Patients With Breast Cancer, NCT01644890, https://clinicaltrials.gov/study/NCT01644890?term=NCT01644890%26rank=1, (accessed April 2024) Search PubMed.
- A Study to Evaluate the Efficacy and Safety of Nanosomal Docetaxel Lipid Suspension in Triple Negative Breast Cancer Patients, NCT03671044, https://clinicaltrials.gov/study/NCT03671044?term=NCT03671044%26rank=1, (accessed April 2024) Search PubMed.
- Treatment of Triple-Negative Breast Cancer With Albumin-Bound Paclitaxel as Neoadjuvant Therapy: A Prospective RCT, NCT04137653, https://clinicaltrials.gov/study/NCT04137653?term=NCT04137653%26rank=1, (accessed April 2024) Search PubMed.
- Single-Arm, Multi-Center Clinical Study of Pyrotinib Maleate Tablets Combined With Albumin-Bound Paclitaxel and Trastuzumab in Neoadjuvant Treatment of Her2-Positive Early or Locally Advanced Breast Cancer, NCT04917900, https://clinicaltrials.gov/study/NCT04917900?term=NCT04917900%26rank=1, (accessed April 2024) Search PubMed.
- Paclitaxel Polymeric Micelles for Injection Versus TPC on the Treatment of HER2-Negative Metastatic Breast Cancer (MBC), NCT06143553, https://clinicaltrials.gov/study/NCT06143553?term=NCT06143553%26rank=1, (accessed April 2024) Search PubMed.
- Clinical Efficacy and Safety of Paclitaxel Polymeric Micelles for Injection in the Treatment of Patients With Taxans-Resistant Pancreatic Adenocarcinoma, Cholangiocarcinoma, Lung Cancer, Gastric Cancer, Esophageal Carcinoma, or Breast Cancer, NCT06199895, https://clinicaltrials.gov/study/NCT06199895?term=NCT06199895%26rank=1, (accessed April 2024) Search PubMed.
- OCTANE: Adjuvant Liposomal Doxorubicin and Carboplatin for Early-Stage Triple-Negative Breast Cancer, NCT05949021, https://clinicaltrials.gov/study/NCT05949021?term=NCT05949021%26rank=1, (accessed April 2024) Search PubMed.
- L. Jiang, L. Li, B. He, D. Pan, K. Luo, Q. Yi and Z. Gu, Anti-cancer efficacy of paclitaxel loaded in pH triggered liposomes, J. Biomed. Nanotechnol., 2016, 12(1), 79–90 CrossRef CAS PubMed.
- S. F. Medeiros, A. M. Santos, H. Fessi and A. Elaissari, Stimuli-responsive magnetic particles for biomedical applications, Int. J. Pharm., 2011, 403(1–2), 139–161 CrossRef CAS PubMed.
- D. D. Ferreira, S. C. Lopes, M. S. Franco and M. C. Oliveira, pH-sensitive liposomes for drug delivery in cancer treatment, Ther. Delivery, 2013, 4(9), 1099–1123 CrossRef CAS PubMed.
- S. Saraf, A. Jain, A. Tiwari, A. Verma, P. K. Panda and S. K. Jain, Advances in liposomal drug delivery to cancer: an overview, J. Drug Delivery Sci. Technol., 2020, 56, 101549 CrossRef CAS.
- P. Pradhan, J. Giri, F. Rieken, C. Koch, O. Mykhaylyk, M. Döblinger, R. Banerjee, D. Bahadur and C. Plank, Targeted temperature sensitive magnetic liposomes for thermo-chemotherapy, J. Controlled Release, 2010, 142(1), 108–121 CrossRef CAS PubMed.
- H. Zhang, W. Gong, Z. Y. Wang, S. J. Yuan, X. Y. Xie, Y. F. Yang, Y. Yang, S. S. Wang, D. X. Yang, Z. X. Xuan and X. G. Mei, Preparation, characterization, and pharmacodynamics of thermosensitive liposomes containing docetaxel, J. Pharm. Sci., 2014, 103(7), 2177–2183 CrossRef CAS PubMed.
- M. B. Yatvin, J. N. Weinstein, W. H. Dennis and R. Blumenthal, Design of liposomes for enhanced local release of drugs by hyperthermia, Science, 1978, 202(4374), 1290–1293 CrossRef CAS PubMed.
- M. Dunne, Y. N. Dou, D. M. Drake, T. Spence, S. M. Gontijo, P. G. Wells and C. Allen, Hyperthermia-mediated drug delivery induces biological effects at the tumor and molecular levels that improve cisplatin efficacy in triple negative breast cancer, J. Controlled Release, 2018, 282, 35–45 CrossRef CAS PubMed.
- A. Jain and S. K. Jain, Advances in tumor targeted liposomes, Curr. Mol. Med., 2018, 18(1), 44–57 CrossRef CAS PubMed.
- X. Zhang, X. Kang, L. Jin, J. Bai, W. Liu and Z. Wang, Stimulation of wound healing using bioinspired hydrogels with basic fibroblast growth factor (bFGF), Int. J. Nanomed., 2018, 4, 3897–3906 CrossRef PubMed.
- E. R. Gomes, M. V. Novais, I. T. Silva, A. L. Barros, E. A. Leite, J. Munkert, A. C. Frade, G. D. Cassali, F. C. Braga, R. M. Pádua and M. C. Oliveira, Long-circulating and fusogenic liposomes loaded with a glucoevatromonoside derivative induce potent antitumor response, Biomed. Pharmacother., 2018, 108, 1152–1161 CrossRef CAS PubMed.
- G. Fontana, L. Maniscalco, D. Schillaci, G. Cavallaro and G. Giammona, Solid lipid nanoparticles containing tamoxifen characterization and in vitro antitumoral activity, Drug Delivery, 2005, 12(6), 385–392 CrossRef CAS PubMed.
- P. Potluri and G. V. Betageri, Mixed-micellar proliposomal systems for enhanced oral delivery of progesterone, Drug Delivery, 2006, 13(3), 227–232 CrossRef CAS PubMed.
- N. I. Payne, C. V. Ambrose, P. Timmins, M. D. Ward and F. Ridgway, Proliposomes: a novel solution to an old problem, J. Pharm. Sci., 1986, 75(4), 325–329 CrossRef CAS PubMed.
- A. Alemi, R. J. Zavar, F. Haghiralsadat, H. Zarei Jaliani, M. Haghi Karamallah, S. A. Hosseini and S. Haghi Karamallah, Paclitaxel and curcumin coadministration in novel cationic PEGylated niosomal formulations exhibit enhanced synergistic antitumor efficacy, J. Nanobiotechnol., 2018, 16, 1–20 CrossRef PubMed.
- S. Sofou and G. Sgouros, Antibody-targeted liposomes in cancer therapy and imaging, Expert Opin. Drug Delivery, 2008, 5(2), 189–204 CrossRef CAS PubMed.
- J. W. Park, K. Hong, D. B. Kirpotin, G. Colbern, R. Shalaby, J. Baselga, Y. Shao, U. B. Nielsen, J. D. Marks, D. Moore and D. Papahadjopoulos, Anti-HER2 immunoliposomes: enhanced efficacy attributable to targeted delivery, Clin. Cancer Res., 2002, 8(4), 1172–1181 CAS.
- N. Parveen, M. A. Abourehab, R. Shukla, P. V. Thanikachalam, G. K. Jain and P. Kesharwani, Immunoliposomes as an emerging nanocarrier for breast cancer therapy, Eur. Polym. J., 2023, 184, 111781 CrossRef CAS.
- Y. Tang, F. Soroush, Z. Tong, M. F. Kiani and B. Wang, Targeted multidrug delivery system to overcome chemoresistance in breast cancer, Int. J. Nanomed., 2017, 671–681 CrossRef CAS PubMed.
- S. Y. Wang, J. Li, Y. Zhou, D. Q. Li and G. M. Du, Chemical cross-linking approach for prolonging diclofenac sodium release from pectin-based delivery system, Int. J. Biol. Macromol., 2019, 137, 512–520 CrossRef CAS PubMed.
- I. Meerovich, M. G. Nichols and A. K. Dash, Low-intensity light-induced drug release from a dual delivery system comprising of a drug loaded liposome and a photosensitive conjugate, J. Drug Targeting, 2020, 28(6), 655–667 CrossRef CAS PubMed.
- I. Meerovich, M. G. Nichols and A. K. Dash, Low-intensity light-induced drug release from a dual delivery system comprising of a drug loaded liposome and a photosensitive conjugate, J. Drug Targeting, 2020, 28(6), 655–667 CrossRef CAS PubMed.
- C. Lin, X. Zhang, H. Chen, Z. Bian, G. Zhang, M. K. Riaz, D. Tyagi, G. Lin, Y. Zhang, J. Wang and A. Lu, Dual-ligand modified liposomes provide effective local targeted delivery of lung-cancer drug by antibody and tumor lineage-homing cell-penetrating peptide, Drug Delivery, 2018, 25(1), 256–266 CrossRef CAS PubMed.
- Z. Hussain, J. A. Khan and S. Murtaza, Nanotechnology: an emerging therapeutic option for breast cancer, Crit. Rev. Eukaryotic Gene Expression, 2018, 28(2), 163–175 CrossRef PubMed.
- A. Kumari, S. K. Yadav and S. C. Yadav, Biodegradable polymeric nanoparticles based drug delivery systems, Colloids Surf., B, 2010, 75(1), 1–8 CrossRef CAS PubMed.
- E. M. Bressler, J. Kim, R. B. Shmueli, A. C. Mirando, H. Bazzazi, E. Lee, A. S. Popel, N. B. Pandey and J. J. Green, Biomimetic peptide display from a polymeric nanoparticle surface for targeting and antitumor activity to human triple-negative breast cancer cells, J. Biomed. Mater. Res., Part A, 2018, 106(6), 1753–1764 CrossRef CAS PubMed.
- M. Zamani, K. Rostamizadeh, H. K. Manjili and H. Danafar, In vitro and in vivo biocompatibility study of folate-lysine-PEG-PCL as nanocarrier for targeted breast cancer drug delivery, Eur. Polym. J., 2018, 103, 260–270 CrossRef CAS.
- U. Hani, M. Rahamathulla, R. A. Osmani, H. Y. Kumar, D. Urolagin, M. Y. Ansari, K. Pandey, K. Devi and S. Yasmin, Recent advances in novel drug delivery systems and approaches for management of breast cancer: a comprehensive review, J. Drug Delivery Sci. Technol., 2020, 56, 101505 CrossRef CAS.
- M. Elmowafy, K. Shalaby, M. H. Elkomy, O. A. Alsaidan, H. A. Gomaa, M. A. Abdelgawad and E. M. Mostafa, Polymeric nanoparticles for delivery of natural bioactive agents: recent advances and challenges, Polymers, 2023, 15(5), 1123 CrossRef CAS PubMed.
- L. Martínez-Jothar, N. Beztsinna, C. F. van Nostrum, W. E. Hennink and S. Oliveira, Selective cytotoxicity to HER2 positive breast cancer cells by saporin-loaded nanobody-targeted polymeric nanoparticles in combination with photochemical internalization, Mol. Pharmaceutics, 2019, 16(4), 1633–1647 CrossRef PubMed.
- R. H. Prabhu, V. B. Patravale and M. D. Joshi, Polymeric nanoparticles for targeted treatment in oncology: current insights, Int. J. Nanomed., 2015, 2, 1001–1018 Search PubMed.
- P. Prabu, A. A. Chaudhari, N. Dharmaraj, M. S. Khil, S. Y. Park and H. Y. Kim, Preparation, characterization, in vitro drug release and cellular uptake of poly(caprolactone) grafted dextran copolymeric nanoparticles loaded with anticancer drug, J. Biomed. Mater. Res., Part A, 2009, 90(4), 1128–1136 CrossRef CAS PubMed.
- S. Niu, G. R. Williams, J. Wu, J. Wu, X. Zhang, H. Zheng, S. Li and L. M. Zhu, A novel chitosan-based nanomedicine for multi-drug resistant breast cancer therapy, Chem. Eng. J., 2019, 369, 134–149 CrossRef CAS.
- H. Devalapally, D. Shenoy, S. Little, R. Langer and M. Amiji, Poly (ethylene oxide)-modified poly (beta-amino ester) nanoparticles as a pH-sensitive system for tumor-targeted delivery of hydrophobic drugs: part 3. Therapeutic efficacy and safety studies in ovarian cancer xenograft model, Cancer Chemother. Pharmacol., 2007, 59, 477–484 CrossRef CAS PubMed.
- A. Pawar, S. Singh, S. Rajalakshmi, K. Shaikh and C. Bothiraja, Development of fisetin-loaded folate functionalized pluronic micelles for breast cancer targeting, Artif. Cells, Nanomed., Biotechnol., 2018, 46(sup1), 347–361 CrossRef CAS PubMed.
- S. Enteshari, J. Varshosaz, M. Minayian and F. Hassanzadeh, Antitumor activity of raloxifene-targeted poly (styrene maleic acid)-poly (amide-ether-ester-imide) co-polymeric nanomicelles loaded with docetaxel in breast cancer-bearing mice, Invest. New Drugs, 2018, 36, 206–216 CrossRef CAS PubMed.
- F. Farrokhi, Z. Karami, S. Esmaeili-Mahani and A. Heydari, Delivery of DNAzyme targeting c-Myc gene using β-cyclodextrin polymer nanocarrier for therapeutic application in human breast cancer cell line, J. Drug Delivery Sci. Technol., 2018, 47, 477–484 CrossRef CAS.
- G. Pillai and M. L. Ceballos-Coronel, Science and technology of the emerging nanomedicines in cancer therapy: a primer for physicians and pharmacists, SAGE Open Med., 2013, 1, 2050312113513759 Search PubMed.
- W. T. Lim, E. H. Tan, C. K. Toh, S. W. Hee, S. S. Leong, P. C. Ang, N. S. Wong and B. Chowbay, Phase I pharmacokinetic study of a weekly liposomal paclitaxel formulation (Genexol®-PM) in patients with solid tumors, Ann. Oncol., 2010, 21(2), 382–388 CrossRef CAS PubMed.
- D. Baidya, J. Kushwaha, K. Mahadik and S. Patil, Chrysin-loaded folate conjugated PF127-F68 mixed micelles with enhanced oral bioavailability and anticancer activity against human breast cancer cells, Drug Dev. Ind. Pharm., 2019, 45(5), 852–860 CrossRef CAS PubMed.
- J. Liu, X. Xu, Y. Li, J. Xu, R. Zhao, S. Liu, J. Wu, L. Zhang and B. Zhang, Bortezomib-loaded mixed micelles realize a “three-in-one” effect for enhanced breast cancer treatment, Biomater. Sci., 2023, 11(14), 4890–4906 RSC.
- Advances in Novel Formulations for Drug Delivery, ed. R. K. Keservani, R. K. Kesharwani and A. K. Sharma, John Wiley & Sons, 2023 Search PubMed.
- Y. Zou, S. Shen, A. Karpus, H. Sun, R. Laurent, A. M. Caminade, M. Shen, S. Mignani, X. Shi and J. P. Majoral, Unsymmetrical Low-Generation Cationic Phosphorus Dendrimers as a Nonviral Vector to Deliver MicroRNA for Breast Cancer Therapy, Biomacromolecules, 2024, 25(2), 1171–1179 CrossRef CAS PubMed.
- L. Xu, H. Wang, W. Xiao, W. Zhang, C. Stewart, H. Huang, F. Li and J. Han, PAMAM dendrimer-based tongue rapidly identifies multiple antibiotics, Sens. Actuators, B, 2023, 382, 133519 CrossRef CAS.
- A. I. Said, D. Staneva and I. Grabchev, New Water-Soluble Poly (propylene imine) Dendrimer Modified with 4-Sulfo-1, 8-naphthalimide Units: Sensing Properties and Logic Gates Mimicking, Sensors, 2023, 23(11), 5268 CrossRef CAS PubMed.
- D. B. Rai, K. Medicherla, D. Pooja and H. Kulhari, Dendrimers-mediated delivery of phytoconstituents, in Nanotechnology Based Delivery of Phytoconstituents and Cosmeceuticals, Springer Nature Singapore, Singapore, 2024, pp. 265–303 Search PubMed.
- H. An, X. Deng, F. Wang, P. Xu and N. Wang, Dendrimers as nanocarriers for the delivery of drugs obtained from natural products, Polymers, 2023, 15(10), 2292 CrossRef CAS PubMed.
- N. N. Sheveleva, A. V. Komolkin and D. A. Markelov, Influence of the chemical structure on the mechanical relaxation of dendrimers, Polymers, 2023, 15(4), 833 CrossRef CAS PubMed.
- B. Sun, B. Ranganathan and S. S. Feng, Multifunctional poly (D, L-lactide-co-glycolide)/montmorillonite (PLGA/MMT) nanoparticles decorated by Trastuzumab for targeted chemotherapy of breast cancer, Biomaterials, 2008, 29(4), 475–486 CrossRef CAS PubMed.
- K. Barzaman, J. Karami, Z. Zarei, A. Hosseinzadeh, M. H. Kazemi, S. Moradi-Kalbolandi, E. Safari and L. Farahmand, Breast cancer: biology, biomarkers, and treatments, Int. Immunopharmacol., 2020, 84, 106535 CrossRef CAS PubMed.
- O. Yuliarti, K. K. Goh, L. Matia-Merino, J. Mawson and C. Brennan, Extraction and characterisation of pomace pectin from gold kiwifruit (Actinidia chinensis), Food Chem., 2015, 187, 290–296 CrossRef CAS PubMed.
- A. Sybachin and V. Pigareva, Ensembles of carboxymethyl cyclodextrins on cationic liposomes as highly efficient nanocontainers for the delivery of hydrophobic compounds, Biochim. Biophys. Acta, Gen. Subj., 2023, 1867(6), 130363 CrossRef CAS PubMed.
- X. He, S. Chen and X. Mao, Utilization of metal or non-metal-based functional materials as efficient composites in cancer therapies, RSC Adv., 2022, 12(11), 6540–6551 RSC.
- K. L. Jetha, A. Vyas, D. Teli, A. Chaudhari, R. Satasiya, V. Patel, S. Soni, S. Modi and V. Apostolopoulos, History of Nanoparticles. Nanocarrier Vaccines: Biopharmaceutics-Based Fast Track Development, 2024, pp. 1–23 Search PubMed.
- Y. Bustanji, J. Taneera, M. H. Semreen, E. Abu-Gharbieh, W. El-Huneidi, M. A. Faris, K. H. Alzoubi, N. C. Soares, B. Albustanji, A. Y. Abuhelwa and R. Abu-Zurayk, Gold nanoparticles and breast cancer: a bibliometric analysis of the current state of research and future directions, OpenNano, 2023, 12, 100164 CrossRef.
- L. Sitia, M. Sevieri, L. Signati, A. Bonizzi, A. Chesi, F. Mainini, F. Corsi and S. Mazzucchelli, HER-2-targeted nanoparticles for breast cancer diagnosis and treatment, Cancers, 2022, 14(10), 2424 CrossRef CAS PubMed.
- V. Jain, H. Kumar, H. V. Anod, P. Chand, N. V. Gupta, S. Dey and S. S. Kesharwani, A review of nanotechnology-based approaches for breast cancer and triple-negative breast cancer, J. Controlled Release, 2020, 326, 628–647 CrossRef CAS PubMed.
- S. Ghosh, A. N. Harke, M. J. Chacko, S. P. Gurav, K. A. Joshi, A. Dhepe, A. Dewle, G. B. Tomar, R. Kitture, V. S. Parihar and K. Banerjee, Gloriosa superba mediated synthesis of silver and gold nanoparticles for anticancer applications, J. Nanomed. Nanotechnol., 2016, 7(4), 1–8 Search PubMed.
- Z. A. Nima, A. M. Alwbari, V. Dantuluri, R. N. Hamzah, N. Sra, P. Motwani, K. Arnaoutakis, R. A. Levy, A. F. Bohliqa, D. Nedosekin and V. P. Zharov, Targeting nano drug delivery to cancer cells using tunable, multi-layer, silver-decorated gold nanorods, J. Appl. Toxicol., 2017, 37(12), 1370–1378 CrossRef CAS PubMed.
- D. Nayak, A. P. Minz, S. Ashe, P. R. Rauta, M. Kumari, P. Chopra and B. Nayak, Synergistic combination of antioxidants, silver nanoparticles and chitosan in a nanoparticle based formulation: characterization and cytotoxic effect on MCF-7 breast cancer cell lines, J. Colloid Interface Sci., 2016, 470, 142–152 CrossRef CAS PubMed.
- M. M. Mkandawire, M. Lakatos, A. Springer, A. Clemens, D. Appelhans, U. Krause-Buchholz, W. Pompe, G. Rödel and M. Mkandawire, Induction of apoptosis in human cancer cells by targeting mitochondria with gold nanoparticles, Nanoscale, 2015, 7(24), 10634–10640 RSC.
- B. Rezaei, P. Yari, S. M. Sanders, H. Wang, V. K. Chugh, S. Liang, S. Mostufa, K. Xu, J. P. Wang, J. Gómez-Pastora and K. Wu, Magnetic nanoparticles: a review on synthesis, characterization, functionalization, and biomedical applications, Small, 2024, 20(5), 2304848 CrossRef CAS PubMed.
- R. Lapusan, R. Borlan and M. Focsan, Advancing MRI with Magnetic Nanoparticles: A Comprehensive Review of Translational Research and Clinical Trials, Nanoscale Adv., 2024, 1–26 Search PubMed.
- V. P. Chavda, A. B. Patel, K. J. Mistry, S. F. Suthar, Z. X. Wu, Z. S. Chen and K. Hou, Nano-drug delivery systems entrapping natural bioactive compounds for cancer: recent progress and future challenges, Front. Oncol., 2022, 12, 867655 CrossRef CAS PubMed.
- V. Popova, E. Dmitrienko and A. Chubarov, Magnetic Nanocomposites and Imprinted Polymers for Biomedical Applications of Nucleic Acids, Magnetochemistry, 2022, 9(1), 12 CrossRef.
- A. P. Shaik, A. S. Shaik, A. Al Majwal and F. A. Al, Blocking interleukin-4 receptor α using polyethylene glycol functionalized superparamagnetic iron oxide nanocarriers to inhibit breast cancer cell proliferation, Cancer Res. Treat., 2017, 49(2), 322 CrossRef CAS PubMed.
- H. Nosrati, N. Rashidi, H. Danafar and H. K. Manjili, Anticancer activity of tamoxifen loaded tyrosine decorated biocompatible Fe 3 O 4 magnetic nanoparticles against
breast cancer cell lines, J. Inorg. Organomet. Polym. Mater., 2018, 28, 1178–1186 CrossRef CAS.
- J. M. Poller, J. Zaloga, E. Schreiber, H. Unterweger, C. Janko, P. Radon, D. Eberbeck, L. Trahms, C. Alexiou and R. P. Friedrich, Selection of potential iron oxide nanoparticles for breast cancer treatment based on in vitro cytotoxicity and cellular uptake, Int. J. Nanomed., 2017, 19, 3207–3220 CrossRef PubMed.
- P. Tiwari, R. P. Shukla, K. Yadav, N. Singh, D. Marwaha, S. Gautam, A. K. Bakshi, N. Rai, A. Kumar, D. Sharma and P. R. Mishra, Dacarbazine-primed carbon quantum dots coated with breast cancer cell-derived exosomes for improved breast cancer therapy, J. Controlled Release, 2024, 365, 43–59 CrossRef CAS PubMed.
- M. Wang, S. Lan, W. Zhang, Q. Jin, H. Du, X. Sun, L. He, X. Meng, L. Su and G. Liu, Anti-Cancer Potency of Copper-Doped Carbon Quantum Dots Against Breast Cancer Progression, Int. J. Nanomed., 2024, 31, 1985–2004 CrossRef PubMed.
- G. G. De la Cruz, L. Rodríguez-Fragoso, P. Rodríguez-Fragoso and A. Rodríguez-López, Toxicity of quantum dots, in Toxicity of Nanoparticles-Recent Advances and New Perspectives, IntechOpen, 2024 Search PubMed.
- X. Lin and T. Chen, A Review of in vivo Toxicity of Quantum Dots in Animal Models, Int. J. Nanomed., 2023, 31, 8143–8168 CrossRef PubMed.
- V. Candela Noguera, Study development and improvement of MCM-41-type MSN synthesis oriented to biomedical applications, Doctoral dissertation, Universitat Politécnica de Valéncia, 2021.
- Y. Feng, Z. Liao, M. Li, H. Zhang, T. Li, X. Qin, S. Li, C. Wu, F. You, X. Liao and L. Cai, Mesoporous silica nanoparticles-based nanoplatforms: basic construction, current state, and emerging applications in anticancer therapeutics, Adv. Healthcare Mater., 2023, 12(16), 2201884 CrossRef CAS PubMed.
- K. Djayanti, P. Maharjan, K. H. Cho, S. Jeong, M. S. Kim, M. C. Shin and K. A. Min, Mesoporous silica nanoparticles as a potential nanoplatform: therapeutic applications and considerations, Int. J. Mol. Sci., 2023, 24(7), 6349 CrossRef CAS PubMed.
- R. Rani, P. Malik, S. Dhania and T. K. Mukherjee, Recent advances in mesoporous silica nanoparticle-mediated drug delivery for breast cancer treatment, Pharmaceutics, 2023, 15(1), 227 CrossRef CAS PubMed.
- A. Lérida-Viso, A. Estepa-Fernández, A. García-Fernández, V. Martí-Centelles and R. Martínez-Máñez, Biosafety of mesoporous silica nanoparticles; towards clinical translation, Adv. Drug Delivery Rev., 2023, 12, 115049 CrossRef PubMed.
- Y. Dutta Gupta, Y. Mackeyev, S. Krishnan and S. Bhandary, Mesoporous silica nanotechnology: promising advances in augmenting cancer theranostics, Cancer Nanotechnol., 2024, 15(1), 9 CrossRef.
- A. Lewinska, A. Radon, K. Gil, D. Błoniarz, A. Ciuraszkiewicz, J. Kubacki, M. Kadziołka-Gaweł, D. Łukowiec, P. Gebara, A. Krogul-Sobczak and P. Piotrowski, Carbon-Coated Iron Oxide Nanoparticles Promote Reductive Stress-Mediated Cytotoxic Autophagy in Drug-Induced Senescent Breast Cancer Cells, ACS Appl. Mater. Interfaces, 2024, 16(12), 15457–15478 CrossRef CAS PubMed.
- M. Zhao, Z. Li, C. Yu, Q. Sun, K. Wang and Z. Xie, Clinically approved carbon nanoparticles for enhanced photothermal-immunotherapy toward cancer metastasis, Chem. Eng. J., 2024, 482, 149039 CrossRef CAS.
- E. Khallaf, S. Mokhtar, L. Adel, A. Y. Ahmed and M. A. Ameen, Assessment of safety margins in breast cancer conserving surgeries after peritumoral
carbon particles injection and intraoperative specimen radiography compared to frozen section pathology, Int. J. Chem. Biol. Sci., 2023, 24(8), 18–25 Search PubMed.
- C. E. Tiron, G. Luta, M. Butura, F. Zugun-Eloae, C. S. Stan, A. Coroaba, E. L. Ursu, G. D. Stanciu and A. Tiron, NHF-derived carbon dots: prevalidation approach in breast cancer treatment, Sci. Rep., 2020, 10(1), 12662 CrossRef CAS PubMed.
- T. Kong, L. Hao, Y. Wei, X. Cai and B. Zhu, Doxorubicin conjugated carbon dots as a drug delivery system for human breast cancer therapy, Cell Proliferation, 2018, 51(5), e12488 CrossRef PubMed.
- N. Vale, S. Silva, D. Duarte, D. M. Crista, L. P. da Silva and J. C. da Silva, Normal breast epithelial MCF-10A cells to evaluate the safety of carbon dots, RSC Med. Chem., 2021, 12(2), 245–253 RSC.
- J. Li, M. Li, L. Tian, Y. Qiu, Q. Yu, X. Wang, R. Guo and Q. He, Facile strategy by hyaluronic acid functional carbon dot-doxorubicin nanoparticles for CD44 targeted drug delivery and enhanced breast cancer therapy, Int. J. Pharm., 2020, 578, 119122 CrossRef CAS PubMed.
- R. Prajapati, E. Garcia-Garrido and Á. Somoza, Albumin-based nanoparticles for the delivery of doxorubicin in breast cancer, Cancers, 2021, 13(12), 3011 CrossRef CAS PubMed.
- A. Sorolla, M. A. Sorolla, E. Wang and V. Ceña, Peptides, proteins and nanotechnology: a promising synergy for breast cancer targeting and treatment, Expert Opin. Drug Delivery, 2020, 17(11), 1597–1613 CrossRef CAS PubMed.
- R. Solanki, P. K. Rajput, B. Jodha, U. C. Yadav and S. Patel, Enhancing apoptosis-mediated anticancer activity of evodiamine through protein-based nanoparticles in breast cancer cells, Sci. Rep., 2024, 14(1), 2595 CrossRef CAS PubMed.
- A. K. Srivastav, P. K. Rajput, J. Jaiswal, U. C. Yadav and U. Kumar, In vitro and in silico investigation of glycyrrhizic acid encapsulated zein nanoparticles: a synergistic targeted drug delivery approach for breast cancer, Int. J. Biol. Macromol., 2024, 131368 CrossRef CAS PubMed.
- Z. Mirza and S. Karim, Nanoparticles-based drug delivery and gene therapy for breast cancer: recent advancements and future challenges, Semin. Cancer Biol., 2021, 69, 226–237 CrossRef CAS PubMed.
- Y. Chang-Qing, L. Jie, Z. Shi-Qi, Z. Kun, G. Zi-Qian, X. Ran, L. Hui-Meng, Z. Ren-Bin, Z. Gang, Y. Da-Chuan and Z. Chen-Yan, Recent treatment progress of triple negative breast cancer, Prog. Biophys. Mol. Biol., 2020, 151, 40–53 CrossRef PubMed.
- A. Mansoori-Kermani, S. Khalighi, I. Akbarzadeh, F. R. Niavol, H. Motasadizadeh, A. Mahdieh, V. Jahed, M. Abdinezhad, N. Rahbariasr, M. Hosseini and N. Ahmadkhani, Engineered hyaluronic acid-decorated niosomal nanoparticles for controlled and targeted delivery of epirubicin to treat breast cancer, Mater. Today Bio, 2022, 16, 100349 CrossRef CAS PubMed.
- Z. Zhang, J. A. Dombroski and M. R. King, Engineering of exosomes to target cancer metastasis, Cell. Mol. Bioeng., 2020, 13, 1–6 CrossRef CAS PubMed.
- S. Safaei, M. Fadaee, O. R. Farzam, A. Yari, E. Poursaei, C. Aslan, S. Samemaleki, D. Shanehbandi, B. Baradaran and T. Kazemi, Exploring the dynamic interplay between exosomes and the immune tumor microenvironment: implications for breast cancer progression and therapeutic strategies, Breast Cancer Res., 2024, 26(1), 57 CrossRef PubMed.
- P. Pandey, F. Khan, R. Maqsood and T. K. Upadhyay, Current Perspectives on Nanoparticle-based Targeted Drug Delivery Approaches in Breast Cancer Treatment, Endocr. Metab. Immune Disord. Drug Targets, 2023, 23(10), 1291–1302 CrossRef CAS PubMed.
- D. Fan, Y. Cao, M. Cao, Y. Wang, Y. Cao and T. Gong, Nanomedicine in cancer therapy, Signal Transduction Targeted Ther., 2023, 8(1), 293 CrossRef PubMed.
- K. Elumalai, S. Srinivasan and A. Shanmugam, Review of the efficacy of nanoparticle-based drug delivery systems for cancer treatment, Biomed. Technol., 2024, 5, 109–122 CrossRef CAS.
- L. Zhang, Z. Ren, J. Lü, X. Mo, J. Lin, Y. Li, W. Ma, P. Liu, Y. Shen, Q. Zhao and L. Qian, Nanoparticles carrying paclitaxel and anti-miR-221 for breast cancer therapy triggered by ultrasound, Cell Death Discovery, 2023, 9(1), 298 CrossRef CAS PubMed.
- J. Li, Q. Wen, J. Dai, B. Wang, Y. Lu, Z. Wu, Y. Fan, F. Zeng, Y. Chen, Y. Zhang and R. Chen, An oral bioactive chitosan-decorated doxorubicin nanoparticles/bacteria bioconjugates enhance chemotherapy efficacy in an in-situ breast cancer model, Int. J. Biol. Macromol., 2024, 5, 131428 CrossRef PubMed.
- C. Nieto, M. A. Vega and E. Martín del Valle, Nature-inspired nanoparticles as paclitaxel targeted carrier for the treatment of her2-positive breast cancer, Cancers, 2021, 13(11), 2526 CrossRef CAS PubMed.
- F. Teymouri and E. Karimi, Development of chitosan-folate modified PLGA nanoparticles for targeted delivery of diosgenin as an anticancer agent, Discover Oncol., 2024, 15(1), 1 CrossRef PubMed.
- F. Rodríguez, P. Caruana, N. De la Fuente, P. Español, M. Gamez, J. Balart, E. Llurba, R. Rovira, R. Ruiz, C. Martín-Lorente and J. L. Corchero, Nano-based approved pharmaceuticals for cancer treatment: present and future challenges, Biomolecules, 2022, 12(6), 784 CrossRef PubMed.
- D. Sun, W. Gao, H. Hu and S. Zhou, Why 90% of clinical drug development fails and how to improve it?, Acta Pharm. Sin. B, 2022, 12(7), 3049–3062 CrossRef CAS PubMed.
- M. Chehelgerdi, M. Chehelgerdi, O. Q. Allela, R. D. Pecho, N. Jayasankar, D. P. Rao, T. Thamaraikani, M. Vasanthan, P. Viktor, N. Lakshmaiya and M. J. Saadh, Progressing nanotechnology to improve targeted cancer treatment: overcoming hurdles in its clinical implementation, Mol. Cancer, 2023, 22(1), 169 CrossRef PubMed.
- T. M. Joseph, M. D. Kar, A. Esmaeili, Ł. Piszczyk, M. S. Hasanin, M. Kattali, J. Haponiuk and S. Thomas, Nanoparticles: taking a unique position in medicine, Nanomaterials, 2023, 13(3), 574 CrossRef CAS PubMed.
- B. Pelaz, C. Alexiou, R. A. Alvarez-Puebla, F. Alves, A. M. Andrews, S. Ashraf, L. P. Balogh, L. Ballerini, A. Bestetti, C. Brendel and S. Bosi, Diverse applications of nanomedicine, ACS Nano, 2017, 11(3), 2313–2381 CrossRef CAS PubMed.
- H. Bahadar, F. Maqbool, K. Niaz and M. Abdollahi, Toxicity of nanoparticles and an overview of current experimental models, Iran. Biomed. J., 2016, 20(1), 1 Search PubMed.
- S. Đorđević, M. M. Gonzalez, I. Conejos-Sánchez, B. Carreira, S. Pozzi, R. C. Acúrcio, R. Satchi-Fainaro, H. F. Florindo and M. J. Vicent, Current hurdles to the translation of nanomedicines from bench to the clinic, Drug Delivery Transl. Res., 2022, 1–26 Search PubMed.
- R. Foulkes, E. Man, J. Thind, S. Yeung, A. Joy and C. Hoskins, The regulation of nanomaterials and nanomedicines for clinical application:
current and future perspectives, Biomater. Sci., 2020, 8(17), 4653–4664 RSC.
- J. K. Patra, G. Das, L. F. Fraceto, E. V. Campos, M. D. Rodriguez-Torres, L. S. Acosta-Torres, L. A. Diaz-Torres, R. Grillo, M. K. Swamy, S. Sharma and S. Habtemariam, Nano based drug delivery systems: recent developments and future prospects, J. Nanobiotechnol., 2018, 16, 1–33 CrossRef PubMed.
- J. M. Metselaar and T. Lammers, Challenges in nanomedicine clinical translation, Drug Delivery Transl. Res., 2020, 10, 721–725 CrossRef PubMed.
- D. Wu, M. Si, H. Y. Xue and H. L. Wong, Nanomedicine applications in the treatment of breast cancer: current state of the art, Int. J. Nanomed., 2017, 16, 5879–5892 CrossRef PubMed.
- F. Yang, Q. He, X. Dai, X. Zhang and D. Song, The potential role of nanomedicine in the treatment of breast cancer to overcome the obstacles of current therapies, Front. Pharmacol, 2023, 14, 1143102 CrossRef CAS PubMed.
- P. Tagde, A. Najda, K. Nagpal, G. T. Kulkarni, M. Shah, O. Ullah, S. Balant and M. H. Rahman, Nanomedicine-based delivery strategies for breast cancer treatment and management, Int. J. Mol. Sci., 2022, 23(5), 2856 CrossRef CAS PubMed.
- S. Jacob, A. B. Nair and J. Shah, Emerging role of nanosuspensions in drug delivery systems, Biomater. Res., 2020, 24, 1–6 CrossRef PubMed.
- Y. Yao, Y. Zhou, L. Liu, Y. Xu, Q. Chen, Y. Wang, S. Wu, Y. Deng, J. Zhang and A. Shao, Nanoparticle-based drug delivery in cancer therapy and its role in overcoming drug resistance, Front. Mol. Biosci., 2020, 7, 193 CrossRef CAS PubMed.
- S. N. Bukhari, Emerging nanotherapeutic approaches to overcome drug resistance in cancers with update on clinical trials, Pharmaceutics, 2022, 14(4), 866 CrossRef CAS PubMed.
- Q. Fernandes, L. Therachiyil, A. Q. Khan, T. Bedhiafi, H. M. Korashy, A. A. Bhat and S. Uddin, Shrinking the Battlefield in Cancer Therapy: Nanotechnology Against Cancer Stem Cells, Eur. J. Pharm. Sci., 2023, 106586 CrossRef CAS PubMed.
- D. V. Bhalani, B. Nutan, A. Kumar and A. K. Singh Chandel, Bioavailability enhancement techniques for poorly aqueous soluble drugs and therapeutics, Biomedicines, 2022, 10(9), 2055 CrossRef CAS PubMed.
- M. Rahman, O. Afzal, S. N. Ullah, M. Y. Alshahrani, A. G. Alkhathami, A. S. Altamimi, S. S. Almujri, W. H. Almalki, E. M. Shorog, M. A. Alossaimi and A. K. Mandal, Nanomedicine-Based Drug-Targeting in Breast Cancer: Pharmacokinetics, Clinical Progress, and Challenges, ACS Omega, 2023, 8(51), 48625–48649 CrossRef CAS PubMed.
- I. Salahshoori, M. Golriz, M. A. Nobre, S. Mahdavi, R. E. Malekshah, A. Javdani-Mallak, M. N. Jorabchi, H. A. Khonakdar, Q. Wang, A. H. Mohammadi and S. M. Mirnezami, Simulation-based approaches for drug delivery systems: navigating advancements, opportunities, and challenges, J. Mol. Liq., 2023, 27, 123888 Search PubMed.
- A. Sinha, F. Z. Simnani, D. Singh, A. Nandi, A. Choudhury, P. Patel, E. Jha, N. K. Kaushik, Y. K. Mishra, P. K. Panda and M. Suar, The translational paradigm of nanobiomaterials: biological chemistry to modern applications, Mater. Today Bio, 2022, 17, 100463 CrossRef CAS PubMed.
- R. Chaudhari, V. Patel and A. Kumar, Cutting-edge Approaches for Targeted Drug Delivery in Breast Cancer: Beyond Conventional Therapies, Nanoscale Adv., 2024, 1–17 Search PubMed.
- S. Waheed, Z. Li, F. Zhang, A. Chiarini, U. Armato and J. Wu, Engineering nano-drug biointerface to overcome biological barriers toward precision drug delivery, J. Nanobiotechnol., 2022, 20(1), 395 CrossRef PubMed.
- M. H. Akhter, H. Khalilullah, M. Gupta, M. A. Alfaleh, N. A. Alhakamy, Y. Riadi and S. Md, Impact of protein corona on the biological identity of nanomedicine: understanding the fate of nanomaterials in the biological milieu, Biomedicines, 2021, 9(10), 1496 CrossRef CAS PubMed.
- S. T. Fateh, F. Mehryab, S. T. Fateh, A. Salehi-Najafabadi and A. R. Aref, Protein corona: challenges and opportunities for cancer therapy, Funct. Nanomater. Cancer Res., 2024, 1, 683–697 Search PubMed.
|
This journal is © The Royal Society of Chemistry 2024 |