DOI:
10.1039/D4RA01830C
(Review Article)
RSC Adv., 2024,
14, 14539-14581
Development of novel transition metal-catalyzed synthetic approaches for the synthesis of a dihydrobenzofuran nucleus: a review
Received
10th March 2024
, Accepted 16th April 2024
First published on 3rd May 2024
Abstract
The synthesis of dihydrobenzofuran scaffolds bears pivotal significance in the field of medicinal chemistry and organic synthesis. These heterocyclic scaffolds hold immense prospects owing to their significant pharmaceutical applications as they are extensively employed as essential precursors for constructing complex organic frameworks. Their versatility and importance make them an interesting subject of study for researchers in the scientific community. While exploring their synthesis, researchers have unveiled various novel and efficient pathways for assembling the dihydrobenzofuran core. In the wake of extensive data being continuously reported each year, we have outlined the recent updates (post 2020) on novel methodological accomplishments employing the efficient catalytic role of several transition metals to forge dihydrobenzofuran functionalities.
1 Introduction
Dihydrobenzofuran scaffolds have been recognized as key structural motifs as they are an integral part of a vast range of biologically active compounds and have attracted significant consideration in the medicinal field.1 The dihydrobenzofuran core is composed of two rings, i.e., the aryl and dihydrofuran ring (Fig. 1). This unique structural feature makes them ideal candidates for the development of novel pharmaceutical agents.2
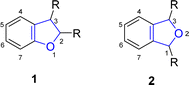 |
| Fig. 1 General structure of dihydrobenzofuran 1 and dihydroisobenzofuran 2. | |
Owing to their high medicinal profile, the synthesis of various naturally occurring products containing a dihydrobenzofuran core has gained significant attention in organic and pharmaceutical chemistry.3 Several bioactive natural products are composed of the 2,3-dihydrobenzofuran framework, such as (+)-decursivine 3,4 (+)-lithospermic acid 4,5 pterocarpan 5,6 (+)-conocarpan 6,7 bisabosqual A 7,8 and caraphenol A 8,9 which have been explored by several synthetic practitioners. They exhibit many biological activities such as anti-malarial, anti-HIV, hepatoprotective, anti-inflammatory and antifungal activities. Similarly, furaquinocins 9 (ref. 10) are also dihydrobenzofurans constituting natural products, which act as antihypertensive agents and inhibit platelet coagulation and aggregation. Furthermore, rubioncolin A 10 and rubioncolin B 12 are used to treat cough, uterine hemorrhage, bladder and kidney stones.11 Cancer is one of the most prevailing and deadly diseases, and researchers are undertaking untiring efforts to discover and develop efficient anti-cancer agents.12–16 (−)-Nocardione 11 (ref. 17) is a Cdc25B tyrosine phosphatase inhibitor that has been observed to display cytotoxic activities (Fig. 2).
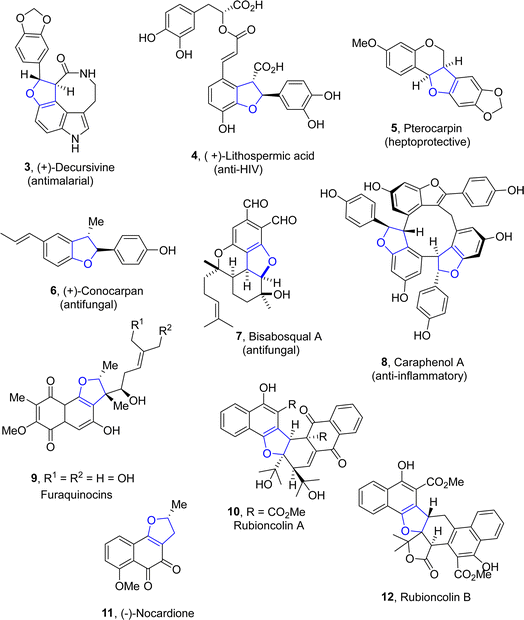 |
| Fig. 2 Structures of some naturally occurring dihydrobenzofurans. | |
Moreover, there are many synthetic dihydrobenzofuran derivatives demonstrating a variety of intriguing medicinal properties, including the imidazolium compound (cytotoxic) 13,18 diesters (anti-leishmaniasis) 14,19 GPR4 agonist 15,20 triazole (antitubercular) 16,21 and the drugs prucalopride 17 (used to treat constipation)22 and efaroxan 18 (α2-adrenoceptor antagonist)23 (Fig. 3).
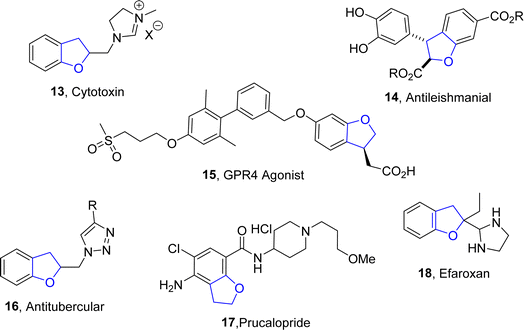 |
| Fig. 3 Structures of some synthetic dihydrobenzofurans. | |
There have been many processes reported for the synthesis of dihydrobenzofurans in the past decades that involve the use of different catalytic systems, i.e., acid or base catalyzed synthesis,24–26 organocatalysis,27 salt catalyzed synthesis28 as well as electrochemical and photochemical synthesis.29,30 Transition metal (TM)-catalyzed reactions involving the synthesis of dihydrobenzofuran skeletons are one of the most reliable and beneficial methods.31 In the past years, several synthetic researchers have designed and reported facile methodologies for the efficient synthesis of dihydrobenzofurans. In this regard, Blum32 et al. in 2014 reported the Ru-catalyzed photochemical synthesis of dihydrobenzofuran derivatives 26 through the reaction of phenols 19 and alkenes 20 via oxidative [3 + 2] cycloadditions. Similarly, Fe-catalyzed facile generation of dihydrobenzofurans via the Claisen rearrangement of allyl aryl ethers 21 was reported by the research group of Sakate33 in 2018. Furthermore, Henry and coworkers34 in 2018 described an Fe and Cu dual catalysis protocol for the synthesis of 2,3-dihydrobenzofurans using substituted phenylethan-2′-ol 22. Ir-catalyzed synthesis of 2,3-dihydrobenzofuran was reported by Ohmura and coworkers35 in 2019, which proceeded via the intramolecular cycloaddition of the C–H bond of o-methyl ether 23 to the C–C double bond. In 2020, the research group of Fang36 disclosed the efficient synthesis of chiral dihydrobenzofuran-3-ols via the Cu-catalyzed intramolecular reaction of aryl pinacol boronic esters 24. In the same year, Li37 et al. envisioned the Ni-catalyzed synthesis of chiral 2,3-dihydrobenzofurans using ortho-substituted aryl halides 25 (Fig. 4). In addition, multiple other TM-catalyzed approaches have also been reported in the literature.38–40
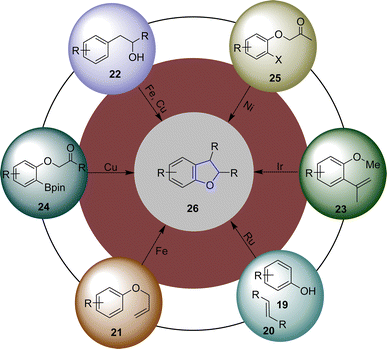 |
| Fig. 4 Different transition metal-induced synthetic routes for the synthesis of dihydrobenzofurans. | |
The TM-catalyzed synthesis of dihydrobenzofuran derivatives has gained tremendous interest in the field of organic chemistry as it exhibits high efficiency, i.e., producing desired products in high yields under ambient reaction conditions.41 Several reviews regarding the synthesis of dihydrobenzofurans have been published to date. In 2019, Laurita et al.42 published a review on the synthesis of 2,3-dihydrobenzofurans covering the data from 2012 to 2019. Similarly, Dapkekar43 et al. in 2022 also reported a review focusing on the methodological developments regarding the synthesis of dihydrobenzofurans and dihydroisobenzofurans. Besides all these published reviews, no particular review has been published concerning solely the TM-catalyzed synthesis of 2,3-dihydrobenzofurans. Herein, the recent data on the synthetic methodologies involving the TM-catalyzed synthesis of dihydrobenzofurans is summarized (reported within 2021–2024).
2 Review of literature
Transition metal-catalyzed transformations represent an advancing domain over the past few years. TM-mediated synthetic pathways have several advantages over conventional protocols.44
2.1. Rh-catalyzed synthesis of 2,3-dihydrobenzofuran derivatives
Rhodium is one of the widely recognized metals in catalysis, which is extensively employed in organic synthesis and industrial processes. Among the range of synthetic methodologies represented for the synthesis of dihydrobenzofuran frameworks, Rh-catalyzed synthesis is a part of the most reliable approaches since they proceed under mild reaction conditions with high yield ranges. TM-catalyzed direct C–H bond functionalization has been proven as a powerful approach for the synthesis of target molecules in an unambiguous and step-economic way.45 In particular, the combination of C–H bond functionalization with C–C bond activation to facilitate the rapid synthesis of functionally diverse frameworks from comparatively simple precursors has garnered considerable focus.46 Considering the significance of utilization of TM-mediated C–H functionalization towards the synthesis of several heterocycles, Zhang47 et al. in 2021 described the Rh(III)-catalyzed construction of cyclic 3-ethylidene-2,3-dihydrobenzofuran skeleton 29 with remarkable regioselectivity and chemoselectivity. In their novel approach, N-phenoxyacetamides 27 were reacted with cyclopropylidenemethyl alkenes 28 via subsequent C–H functionalization and [3 + 2] annulation in the presence of [Cp*RhCl2]2 and NaOAc (as a base) to synthesize desired products 29 in moderate to high yields (37–80%) (Scheme 1).
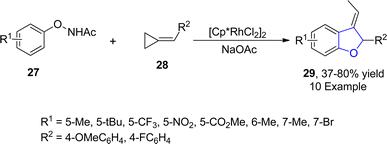 |
| Scheme 1 Synthesis of cyclic 3-ethylidene-2,3-dihydrobenzofuran 29. | |
In 2022, Song48 et al. developed a novel protocol leading towards the synthesis of dihydrobenzofuran derivatives employing coupling partners associated via asymmetric C–H functionalization. In their methodology, substituted phenoxyacetamides 31 were made to react with diazooxindoles 30 in the presence of the rhodium-based catalyst (RhCp*Cl), exploiting cesium carbonate as a base in dioxane solvent. As a result, spirooxindoyl-substituted dihydrobenzofuran derivatives 32 were obtained in 49–76% yields (Scheme 2). The methodology was also employed for the synthesis of various heptacyclic molecules.
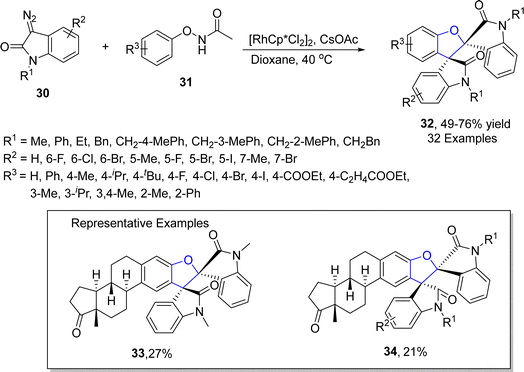 |
| Scheme 2 Synthesis of bispirooxindoyl dihydrobenzofurans derivatives 32. | |
Sun49 et al. in 2022 envisioned another rhodium-catalyzed difunctionalization of p-substituted olefinic arenes 35 to synthesize 2,3-dihydrobenzofuran derivatives 39, 40 and 41. Olefinic arenes 35 were made to react with unsaturated reactants such as isocyanates 38, dioxazolones 37 and internal alkynes 36 via a tandem reaction. In their synthetic methodology, twofold C–H activation of 35 took place at the ortho and meta positions. In the first step, the already present directing group (DG) on 35 resulted in the installation of the alkene coupling partner on the ortho position. This further acted as a relayed directing group for the second activation of C–H, which resulted in the cyclization of olefins at the para position to form the desired products (benzofuran derivative) 39, 40 and 41. Moderate to high yields (40–86%) were obtained when the reaction was carried out in the presence of t-AmOH (as solvent), CsOPiv (as an additive), and AgOAc (as an oxidant) at 120 °C for 12 h (Scheme 3). The mechanism of the reaction was assumed to proceed via the interaction of olefinic arenes 35 with Rh catalyst. Further, alkyne insertion took place to generate intermediate 42, followed by the dissociation of the bonds and C–H bond activation of intermediate 42. In the next step, alkene insertion of 42 resulted in the formation of another intermediate 43, which underwent reductive elimination to furnish the desired products 39 (Scheme 4). The synthetic utility of the above mentioned methodology was also examined.
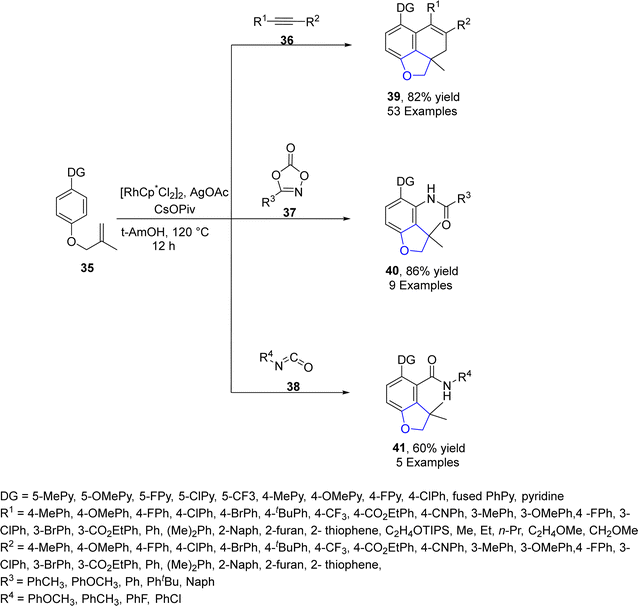 |
| Scheme 3 Synthesis of diverse dihydrobenzofuran derivatives 39–41. | |
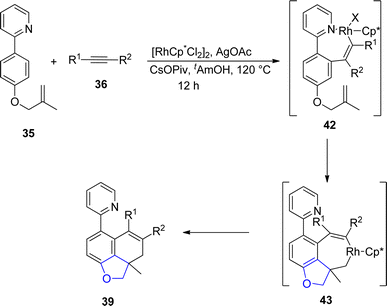 |
| Scheme 4 Proposed mechanism for the synthesis of dihydrobenzofuran derivatives 39. | |
Wei50 et al. in 2022 envisioned a rhodium-catalyzed [3 + 2] annulation of 2-alkenylphenols 44 and N-phenoxyacetamides 45 in the presence of base additive (Zn(OAc)2) along with methanol (as a solvent) to access 2,3-dihydrobenzofurans 48 in excellent yields (90%). The proposed mechanism deduced that intermediate 46 was formed via reversible C–H/N–H bond cleavage of 45 assisted by Rh active catalyst, followed by the insertion of 44, to furnish intermediate 47. After subsequent oxidative addition, intramolecular hydrogen transfer and finally nucleophilic 1,4-addition gave the desired dihydrobenzofuran skeletons 48 (Scheme 5).
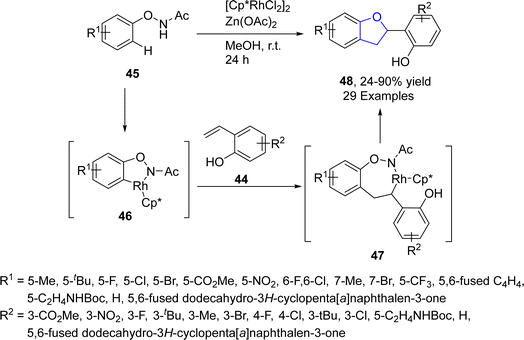 |
| Scheme 5 Synthesis of 2,3-dihydrobenzofuran derivatives 48. | |
In the same year, another rhodium-catalyzed approach for the asymmetric synthesis of dihydrobenzofuran derivatives 51 was put forward by Yu51 et al. In their methodology, aryl-joined alkenes 49 and substituted dioxazolone 37 were subjected to chiral rhodium-promoted carboamidation, using copper acetate as an additive in the presence of AgSbF6 and dichloroethane to attain the enantioselective synthesis of dihydrobenzofurans 51 in 44–83% yield with up to 98.5
:
1.5 enantiomeric ratio. The reaction mechanism was proposed to undergo C–H activation and migratory insertion, followed by oxidative addition to generate intermediate 50. The resulting intermediate 50 was then believed to execute reductive elimination and protonation to achieve enantioenriched 2,3-dihydro-3-benzofuranmethanamides 51 (Scheme 6). To examine the synthetic utility of this novel approach, derivatization of the synthesized compounds was also carried out.
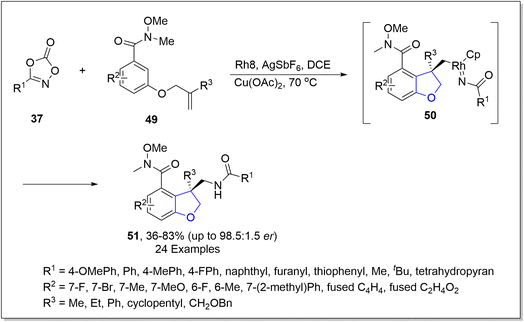 |
| Scheme 6 Asymmetric synthesis of 2,3-dihydro-3-benzofuranmethanamides 51. | |
Sinha52 et al. in 2023 demonstrated a rhodium-promoted synthesis of dihydrobenzofurans 55. Imidazole (directing group)-substituted allyloxy aryls 52 were subjected to intramolecular regioselective hydroarylation in the presence of [RhCp*Cl2]2 catalyst, employing cesium acetate as a base in methanol
:
water (2
:
1) solvent. As a consequence, imidazole-constituting dihydrobenzofurans 55 were attained in moderate to excellent yield (52–91%). The reaction mechanism involved coordination with the rhodium catalyst, followed by the activation of the C–H bond to generate rhodacycle intermediates 53 & 53*. The rhodacycle intermediate 53 was then supposed to undergo migratory insertion to generate the seven-membered intermediate 54, which underwent contraction to furnish the target molecules 55 (Scheme 7).
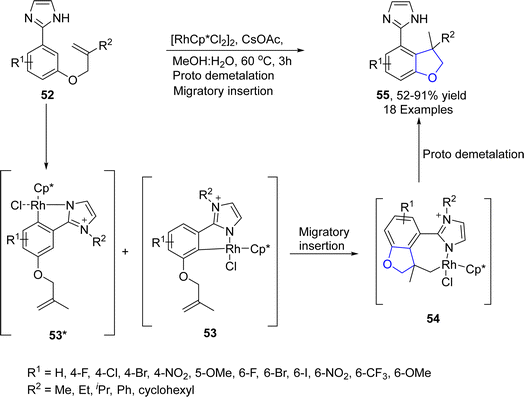 |
| Scheme 7 Synthesis of imidazole-containing dihydrobenzofuran derivatives 55. | |
Oxa-Michael addition reaction is one of the significant reactions of Michael addition for the construction of C–O skeletons and is used for several methodological approaches towards dihydrobenzofurans synthesis.53 In this regard, Zhu54 et al. in 2021 accomplished the synthesis of diverse stereoisomers of asymmetric 2,3-dihydrobenzofurans 58, 59, 60 and 61 via one pot, stereodivergent dual catalysis. In their novel methodology, arylvinyldiazoacetates 56 were reacted with substituted aminophenols 57 in a relay catalytic system to synthesize asymmetric products (58, 59, 60 and 61) in excellent yields (up to 99%) with exceptional enantiomeric and diastereomeric excess (99% ee, 99
:
1 dr respectively). In this regard, an initial C–H functionalization was attained employing a rhodium catalyst consisting of ligand 64, followed by the utilization of other organo-catalysts 62 and 63 that induced oxa-Michael addition to furnish the desired isomers of chiral 2,3-dihydrobenzofurans (58, 59, 60 and 61) (Scheme 8). In addition to gram scale synthesis, the derivatization of the generated products was also carried out as these compounds were transformed into pharmaceutically important and enantiopure amino alcohols.
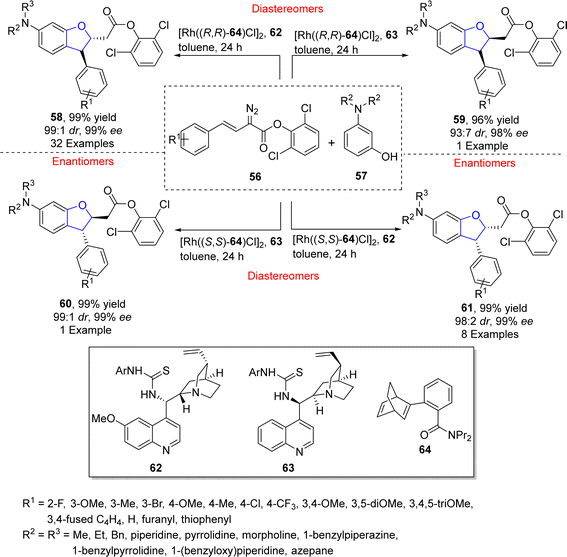 |
| Scheme 8 Synthesis of asymmetric 2,3-disubstituted dihydrobenzofuran derivatives 58–61. | |
Metal carbenes are diverse intermediates that enable particular C–C bond forming transformations including C–H insertion,55 ylide formation,56 and aromatic addition reactions.57 The asymmetric synthesis of 2,3-dihydrobenzofurans involving the insertion of carbene precursors into C(sp3)–H bonds potentially provides access to various crucial compounds like dihydrobenzofurans.58 In this regard, in 2021, Bi59 et al. designed a new method for the efficient assembly of asymmetric fluoroalkyl 2,3-disubstituted dihydrobenzofurans 66 in good to excellent yields (53–99%) with remarkable diastereomeric and enantiomeric ratio (>20
:
1 dr, 68
:
32–98
:
2 er) via a rhodium-catalyzed protocol. In their synthetic approach, fluoroalkyl N-triftosylhydrazones 65 were used as carbene precursors, which were transformed into the desired products 66 via the intramolecular insertion of carbene into the α-C(sp3)–H bond of ether (Scheme 9). This efficient protocol was also used for the gram-scale synthesis of diverse 2,3-dihydrobenzofuran derivatives. Bi and coworkers also synthesized various enantioselective natural and bioactive molecules 67, 68, 69 and 70 employing the above methodology (Fig. 5).
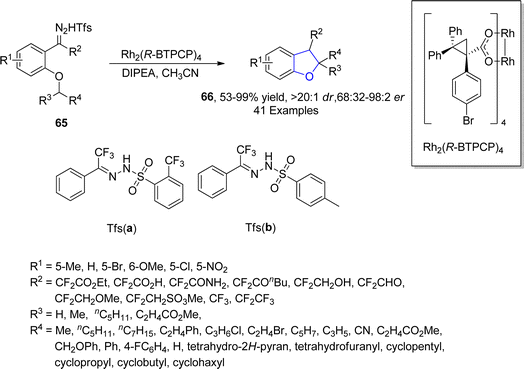 |
| Scheme 9 Synthesis of asymmetric fluoroalkyl dihydrobenzofurans 66. | |
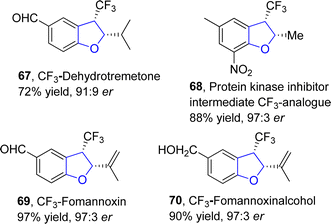 |
| Fig. 5 Synthesized natural and bioactive molecules. | |
Buckley60 et al. in 2021 reported the synthesis of novel diastereoselective dirhodium carboxylate catalysts and employed them in the construction of 2,3-dihydrobenzofuran skeletons 72, 73. In their novel methodology, the stereoselective C–H insertion reaction of aryldiazoacetates 71 took place in the presence of catalytic amount of dirhodium catalyst 74 to furnish 2,3-dihydrobenzofuran units 72 & 73 with excellent trans enantiopurity (84% ee) and trans diastereoselectivity (>91
:
9 dr) in toluene (Scheme 10).
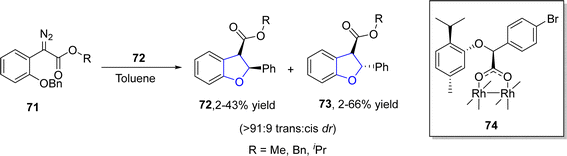 |
| Scheme 10 Synthesis of the 2,3-dihydrobenzofuran skeleton 72 and 73. | |
In 2021, Hong61 et al. accomplished the Rh and asymmetric phosphoric acid 79 catalyzed synthesis of 2,3-dihydrobenzofurans 78 via stereoselective Mannich type interception of phenolic oxonium ylides. In their novel methodology, diazo-containing phenolic derivatives 75 were made to react with imines 76 to synthesize the desired dihydrobenzofuran motifs 78 in low to excellent yield range (35–90%) with exclusive diastereoselectivity (>20
:
1 dr) and enantioselectivity (>99% ee) values. The formation of oxonium ylide intermediate 77 took place by the addition of phenolic OH, followed by the interception of 77 with imines 76 to generate Mannich-type products 78 in the presence of chiral phosphoric acid 77 (Scheme 11). Further transformations of the synthesized compounds were also carried out to demonstrate the synthetic efficacy of the mentioned approach.
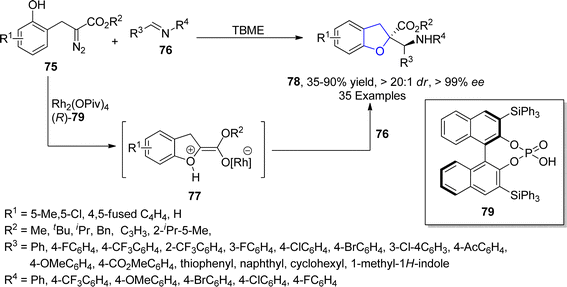 |
| Scheme 11 Synthesis of diastereoselective 2,2-disubstituted dihydrobenzofuran 78. | |
In 2022, Hu62 et al. presented a strategy for the efficient synthesis of 3-hydroxyoxindole incorporating 2,3-dihydrobenzofuran derivatives 83 via a Rh-catalyzed protocol. In their novel approach, diazo-containing phenolic compounds 80 were reacted with isatin 81 through an aldol type addition reaction to afford desired scaffolds 83 in moderate to excellent yields (58–98%) with exclusive diastereoselectivity (81
:
19–95
:
5 dr). Carbene species generated from diazo compound, underwent intramolecular cyclization with hydroxyl group to form oxonium ylide intermediate 82. The isatin 81 added on the Si-face of 82 via aldol-type reaction to furnish desired 2,3-dihydrobenzofuran scaffolds 83 (Scheme 12). Two synthesized compounds 84 and 85 were found to exhibit anticancer activities against human colon cancer cells with 15.99 μM and 14.48 μM IC50 values, respectively (Fig. 6). In order to demonstrate the practicality of the synthetic strategy, several spiro heterocyclic and amide products were also obtained from the synthesized 2,2-disubstituted dihydrobenzofurans 83.
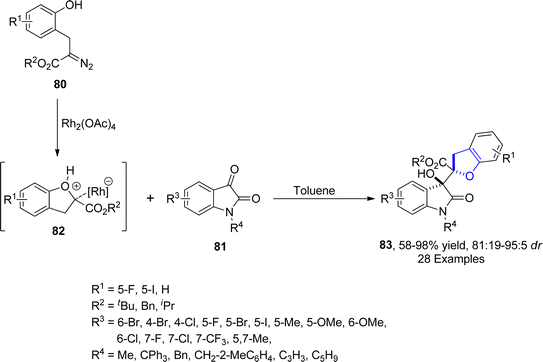 |
| Scheme 12 Synthesis of 3-hydroxyoxindole-containing 2,3-dihydrobenzofuran derivatives 83. | |
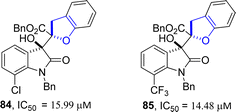 |
| Fig. 6 Structures of compounds 84 & 85 exhibiting anticancer potential. | |
Rh-catalyzed synthesis of heterocycles via [3 + 2] annulation portray an appealing strategy as it offers complete regio- and stereo-control for introducing the functional groups. Related to that, Zhong63 et al. in 2021 demonstrated the synthesis of α-quaternary carbon containing 2,3-dihydrobenzofuran analogues 89 via Rh-catalyzed C–H activation/[3 + 2] annulation. In their novel methodology, N-phenoxy amides 86 were reacted with propargylic monofluoroalkynes 87 in the presence of an Rh catalyst [Cp*RhCl2]2 and NaOAc (used as base) to afford cyclic products 89 in low to high yields (35–78%). Rh-activated C–H activation of 87 took place, followed by the regioselective insertion of alkyne to afford the five-membered species 88. Next, 88 subsequently underwent oxidative addition, reductive elimination and finally bimolecular nucleophilic substitution reaction to furnish 3-alkylidene-2,3-dihydrobenzofuran moiety 89 (Scheme 13). Various spiro compounds were also synthesized from these alkylidene-2,3-dihydrobenzofurans 89 to illustrate the synthetic utility of the reaction.
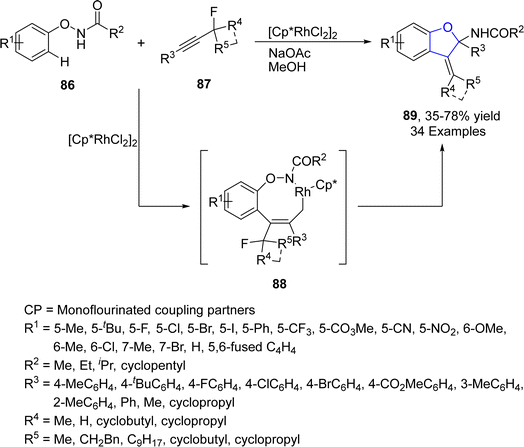 |
| Scheme 13 Synthesis of 3-alkylidene-2,3-dihydrobenzofurans 89. | |
Singh64 et al. in 2021 also reported the Rh-catalyzed, chemodivergent synthesis of 2,3-dihydrobenzofuran derivatives 92 through the coupling of N-phenoxyacetamides 45 with alkylidenecyclopropanes 90 via C–H and C–C bond activation. This transformation was in accordance with the fact that the cyclopropanes are highly reactive in nature and underwent ring opening reactions to give five-membered heterocyclic compounds.65 Polar solvent, i.e., hexafluoroisopropanol (HFIP), induced [3 + 2] annulation to achieve the desired products 92 in moderate to high yield (52–82%). Rh-assisted coupling of 45 and 90 took place, followed by the formation and scission of the 7-membered ring and subsequently β-hydride elimination to afford the intermediate 91. Next, this intermediate 91 underwent the oxidative insertion of the N–O bond, nucleophilic addition and finally deprotonation to achieve 2,3-disubstituted dihydrobenzofuran derivatives 92 (Scheme 14). The gram scale synthesis was also performed in order to demonstrate the efficacy of the synthetic strategy.
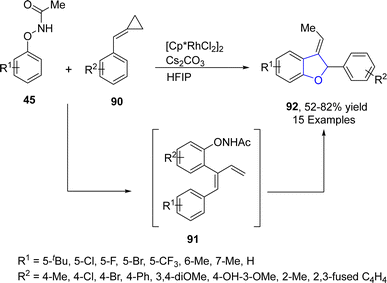 |
| Scheme 14 Synthesis of 3-ethylidenedihydrobenzofuran derivatives 92. | |
In 2021, Paymode and Sharma66 designed a facile approach for the preparation of polycyclic 2,3-dihydrobenzofuran derivatives 95 via a rhodium-catalyzed system. In their synthetic approach, diazo compounds 93 were condensed with 2,3-dihydrofurans/cyclopentenes/cyclohexenes 94 via the [3 + 2] annulation process in the presence of Rh2(TPA)4 to furnish desired dihydrobenzofurans 95 in moderate to high yields (34–85%) (Scheme 15). In the wake of wide-spreading bacterial diseases, synthetic chemists have employed several synthetic pathways to develop efficacious anti-bacterial agents.67 Employing the mentioned methodology, Paymode and Sharma also performed the total synthesis of naturally occurring aflatoxin B2 96a (Fig. 7), which exhibits potent antimitotic and antimicrobial activities.
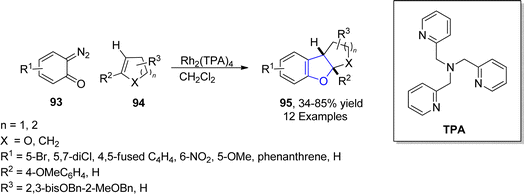 |
| Scheme 15 Synthesis of fused rings containing 2,3-dihydrobenzofuran derivative 95. | |
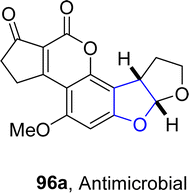 |
| Fig. 7 Structure of aflatoxin B2 96a. | |
Various tailored molecules are synthesized as a result of ring-opening reactions of cyclic compounds.68 Jiang69 et al. in 2022 carried out the rhodium-mediated one pot synthesis of benzofuran-3(2H)-ones 101 by reacting aliphatic alcohols (MeOH) with salicylaldehyde 97 and cyclopropanols 98. After interpreting the screening results, a total of three series of target heterocycles 101 were synthesized in moderate to high yield (32–70%) by independently adding the substitution on one of the three components one by one under optimized conditions ([CpRhCl2]2 catalyst, Cu(OAc)2 oxidant & CsOAc (additive) (Scheme 15). The reaction mechanism was proposed to involve the C–H activation of substituted salicyclaldehydes 97, followed by ligand exchange with aryl cyclopropanols 98, β-carbon elimination and reductive elimination (cyclopropane ring opening) to result in C–H alkylation product 99. The compound 99 was then supposed to be enolized by copper acetate, followed by β-hydride elimination and oxy-Michael addition to give benzofuranones 100. Substituted benzofuranones 100 were then assumed to be reoxidized, followed by methanol involving Michael addition to afford target molecules 101 (Scheme 16).
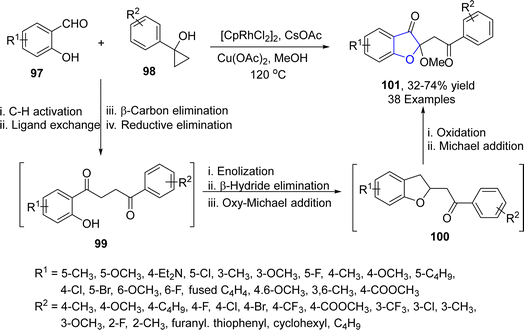 |
| Scheme 16 Synthesis of benzofuran-3(2H)-ones 101. | |
2.2. Pd-catalyzed synthesis of 2,3-dihydrobenzofuran derivatives
Palladium is a valuable gray-white metal, especially used as a catalyst due to its distinct properties such as thermal stability, resistance to poisoning, selectivity and its high surface area. Pd-catalyzed synthesis of five-membered heterocycles has been presented as the most beneficial and reliable synthetic methods as it has diverse functional group tolerance and carried out in mild reaction conditions.70 The classical Heck coupling progresses through different steps including oxidative addition, proceeded by migratory insertion and reductive elimination. Heck coupling has provided novel methodologies towards the sophisticated aromatic substrates from organohalides and alkenes due to the stepwise development of synthetic logic.71 Over the past few decennia, Pd-catalyzed Heck coupling has been widely used as one of the crucial synthetic tools facilitating the straightforward synthesis of molecular heterogeneity and complexity.72 In 2021, Wu73 et al. disclosed an enantioselective, Pd-catalyzed method for the synthesis of 2,3-dihydrobenzofuran derivatives 105. In their novel methodology, aryl iodide-joined alkenes 102 were reacted with o-alkynylanilines 103 in the presence of Pd2(dba)3·CHCl3 along with ligand N-Me–Xu3 via Heck/Cacchi reactions to achieve dihydrobenzofurans in excellent yields (84–97%). Asymmetric σ-alkylpalladium intermediate 104 was formed via intramolecular Heck coupling reaction of Pd activated 102, followed by its reaction with 103 to generate the desired polycyclic products 105 with exceptional enantiomeric excess (84–97% ee) (Scheme 17). The gram scale synthesis was also demonstrated along with the derivatization of the synthesized compounds.
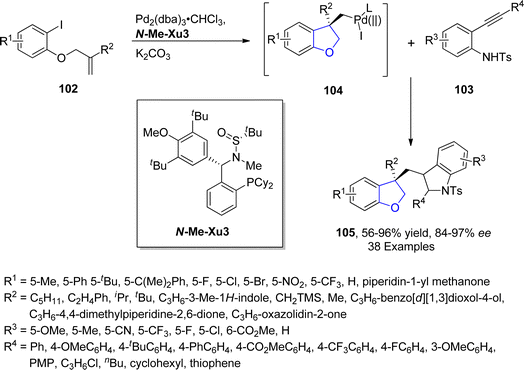 |
| Scheme 17 Synthesis of polycyclic dihydrobenzofurans 105. | |
In 2021, Sreenivasulu and Satyanarayana74 envisioned a Pd-catalyzed, regio- and stereo-selective construction of Z/E isomers of 1,3-dihydroisobenzofurans 108 & 109 via the aryl Heck coupling of o-substituted tertiary alcohols 106 and substituted aryl bromides 107. It is a temperature and time-dependent strategy, in which Z-isomer 108 was formed at 80 °C after 4–6 hours of reaction, whereas stable E-isomer 109 was attained at 100 °C after 8–12 hours of reaction (Scheme 18). Initially, Pd-assisted intermolecular Heck coupling reaction between 106 and 107 took place to generate an intermediate 110, followed by the elimination reaction to form a palladacycle 111. Further, this palladacycle underwent intramolecular oxo-cyclization to furnish the desired heterocyclic products 108 & 109 in moderate to high yields, i.e., 49–84% for Z-isomer and 68–88% for E-isomer, respectively (Scheme 19).
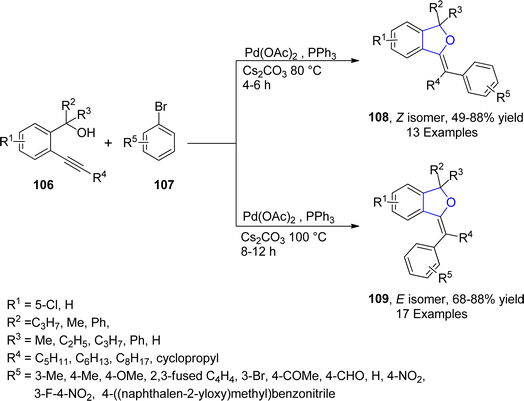 |
| Scheme 18 Synthesis of (Z)/(E)-3-(1-arylalkylidene)-1,3-dihydroisobenzofurans 108 and 109. | |
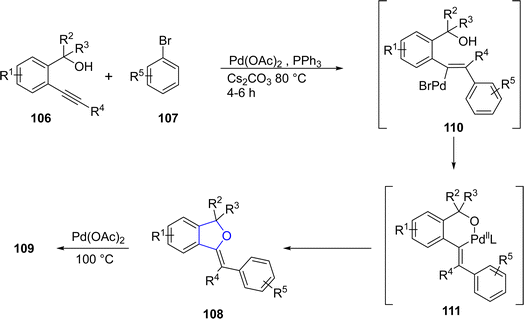 |
| Scheme 19 Proposed mechanism for the synthesis of (Z)/(E)-3-(1-arylalkylidene)-1,3-dihydroisobenzofurans 108 and 109. | |
Pd/XuPhos catalyst is used as an efficient catalytic system for the stereodefined cascade Heck/intermolecular C–H alkylation reactions.75 Taking into consideration the wide synthetic utility of borylated compounds, Wu76 et al. in 2022, also developed a novel palladium promoted Heck/borylation strategy utilizing alkenes fused with aryl iodides 102 as precursors. Their synthetic protocol involved the treatment of aromatic rings substituted alkenes 102 and B2pin2 112 in the presence of Pd2(dba)3·CHCl3 catalyst exploiting N-Me–Xu3 as a ligand (having 3,5-di-tert-butyl-4-methoxyphenyl group), using cesium carbonate as a base in diethyl ether and water. This protocol resulted in the efficacious and enantioselective synthesis of dihydrobenzofuran based boronic esters 113 (52–98%) with excellent enantiomeric excess (53–97% ee). This highly efficient synthetic strategy also paved routes towards the accomplishment of chromane, indoline and indane boronic esters (Scheme 20).
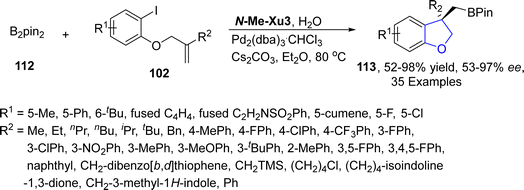 |
| Scheme 20 Synthesis of 2,3-dihydrobenzofuranyl boronic esters 113. | |
In 2022, Guo77 et al. successfully designed a new Pd-catalyzed method for the synthesis of substituted 3,3-disubstituted-2,3-dihydrobenzofurans via the reaction of olefin-tethered aryl iodides 102 with α,β-unsaturated ketones 114 and substituted styrenes 115. In their one-pot synthetic methodology, two sequential Heck couplings took place, leading to the construction of dihydrobenzofuran skeletons 116 and 117. Good to high yields, i.e., 87% (116) and 78% (117), with exclusive E/Z selectivity (20
:
1) values of target molecules were achieved when Pd(PhCN)2Cl2 was subjected as a catalyst along with Cy2NMe (base) in MeCN (solvent) (Scheme 21). The reaction mechanism was proposed to proceed via the reaction of reduced Pd species [Pd(0)] and aryl iodides 102 to form an intermediate 118, which underwent subsequent intramolecular insertion reaction and olefin insertion reaction to form another intermediate 119. Next, reductive elimination reaction finally generated the desired dihydrofuran products 117 (Scheme 22).
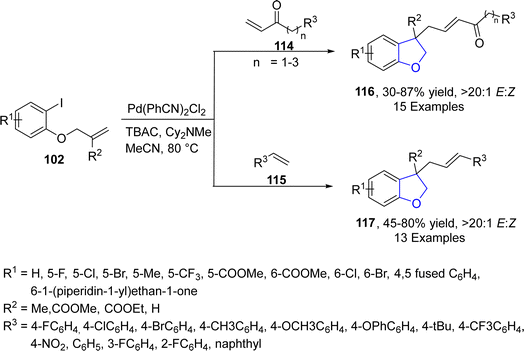 |
| Scheme 21 Synthesis of 3,3-disubstituted-2,3-dihydrobenzofuran 116 and 117. | |
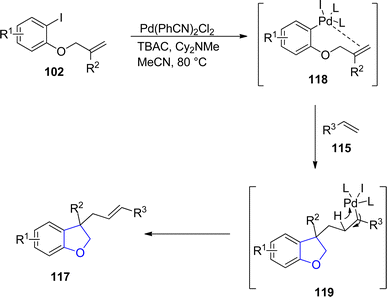 |
| Scheme 22 Proposed mechanism for the synthesis of 3,3-disubstituted dihydrobenzofurans 117. | |
Spirocyclic organic compounds have acquired a significant place in medicinal chemistry due to their vast biological potential.78 Marchese79 et al. in 2022 devised a modular method for the preparation of 2,3-dihydrobenzofuran-based spirocyclic compounds 121, 122 and 123 via palladium catalysis. In their synthetic approach, o-substituted aryl iodides 120 were transformed into corresponding bis-heterocyclic spirocycles 121, 122 and 123 by following the Mizoroki–Heck-type reaction pathway in the presence of Cs2CO3 (used as base). Initially, the oxidative addition of 120 took place, followed by migratory insertion and C–H activation to form an intermediate, which on reductive elimination generated spirocyclic dihydrobenzofurans 121, 122 and 123. Low to high yields (31–94%) were observed using this methodological approach (Scheme 23).
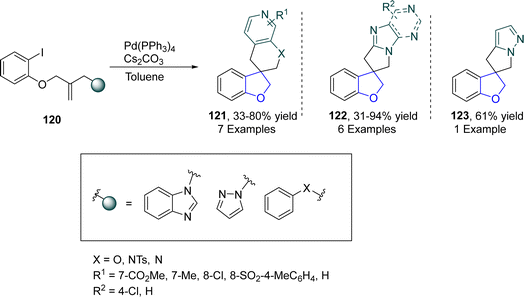 |
| Scheme 23 Synthesis of heterocyclic spiro-2,3-dihydrobenzofurans 121, 122 and 123. | |
Another palladium-catalyzed method for dihydrobenzofurans was given by Kang80 et al. in 2022. They carried out the palladium-mediated aminocarbonylation by treating aryl iodide-linked alkenes 124 with diversely substituted nitro compounds 125 and 126. The reaction took place smoothly utilizing PdCl2 as a catalyst, Mo(CO)6 for the release of CO, triphenylphosphine as the ligand, DIPEA as base in tetrahydrofuran solvent without any addition of additive and reducing agent (Scheme 24). The synthetic pathway was proposed to follow the certain steps, i.e., oxidative addition, intramolecular carbopalladation, linking and integration of CO to form intermediate 131. This was followed by the attack of amine (obtained by the reduction of substituted nitro compound) and reductive elimination to gain dihydrobenzofuran derivatives 127 and 128 in 51–88% yields (Scheme 25).
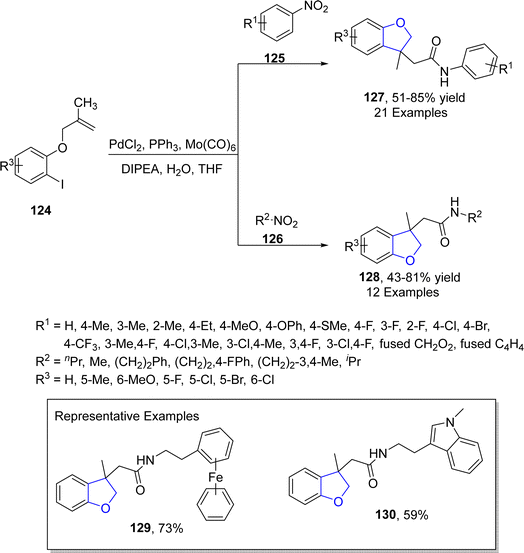 |
| Scheme 24 Synthesis of carbamoyl-substituted 2,3-dihydrobenzofurans 127 and 128. | |
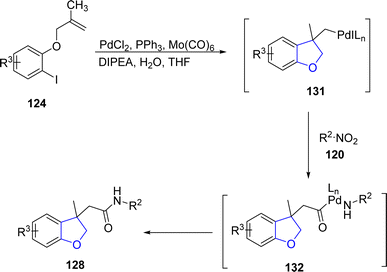 |
| Scheme 25 Proposed mechanism for the synthesis of carbamoyl-substituted 2,3-dihydrobenzofurans 128. | |
In 2023, another palladium-promoted asymmetric synthesis of dihydrobenzofurans via Heck/Tsuji–Trost was put forward by Zhang's group.81 In the newly developed synthetic pathway, substituted dienes 133 were treated with halo-substituted phenols 134 using Pd2dba3·CHCl3 as catalyst and TY-Phos as ligand using sodium phenoxide in dichloromethane, which afforded alkenyl-substituted dihydrobenzofurans 135 in 35–99% yield with enantiomeric excess (73–97 ee) (Scheme 26). To demonstrate the practicality of the novel synthetic approach, the gram scale synthesis of 135 was performed. Moreover, the derivatization of the synthesized compounds towards the synthesis of natural products, i.e., tremetone and fomannoxin, was also performed.
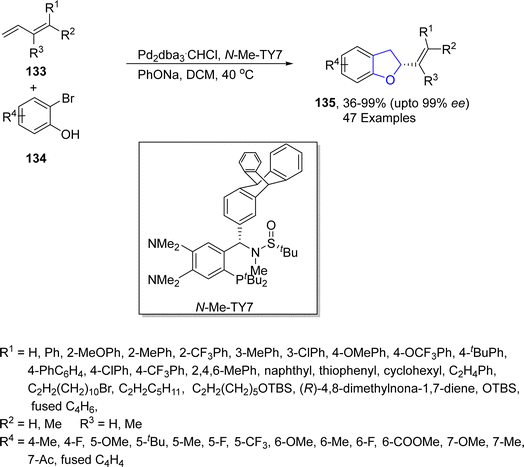 |
| Scheme 26 Synthesis of 2,3-dihydrobenzofurans 135. | |
The Pd-catalyzed synthesis of 2,3-dihydrobenzofurans involving the insertion of carbene precursors into C(sp3)–H bonds potentially provides access to various crucial compounds like dihydrobenzofurans.82 In 2023, Ding83 et al. reported a rarely explored 1,5-Pd/H shift to synthesize dihydrobenzofurans. They treated alkynes-substituted compounds 136 (obtained from 2-bromo-3-hydroxy benzaldehydes) with R–B(OH)2/R–BPin, substituted alkynols 137 and N-sulfonyl hydrazones 138 via palladium catalyzed (PdCl2 & Pd(OAc)2 respectively) cascade reaction. The reaction proceeded via the 1,5-Pd/H shift approach between the vinyl and acyl moiety to furnish acyl palladium species. The acyl palladium intermediates were then subjected to decarbonylation, followed by the reaction with nucleophiles to yield dihydrobenzofuran derivatives 139, 140 and 141 in efficient yields via decarbonylative alkenylation, arylation and alkynylation (Scheme 27). The novel synthetic strategy highlights the significant synthetic efficacy, facilitating the production of disubstituted and polycyclic products.
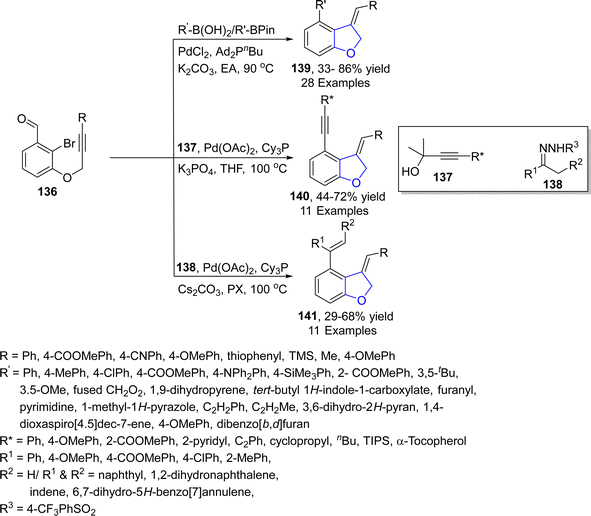 |
| Scheme 27 Synthesis of 2,3-dihydrobenzofurans 139–141. | |
Annulation reactions simply refer to the process of combining two or more molecular fragments, often cyclic structures, to form bridged or fused ring systems. Pd-catalyzed annulation reactions involving the synthesis of arene-fused furan heterocycles have tremendous importance in organic synthesis.84 In this perspective, Zhou85 et al. in 2021 prepared a library of polycyclic dihydrobenzofurans 146 via Pd-catalyzed annulation reaction. In their novel methodology, alkenyl ethers 142 and alkynyl oxime ethers 143 underwent cyclization reaction in the presence of Pd(OAc)2 along with CuCl2 (used as an oxidant) and tetrabutyl ammonium bromide (as co-catalyst) to afford 2,3-dihydrobenzofuran derivatives 146 in moderate to excellent yields (41–86%) (Scheme 28). This synthetic approach covers a broad range of substrate scope (32 examples). Various synthetic transformations of the synthesized compounds took place to illustrate the synthetic efficiency of the procedure.
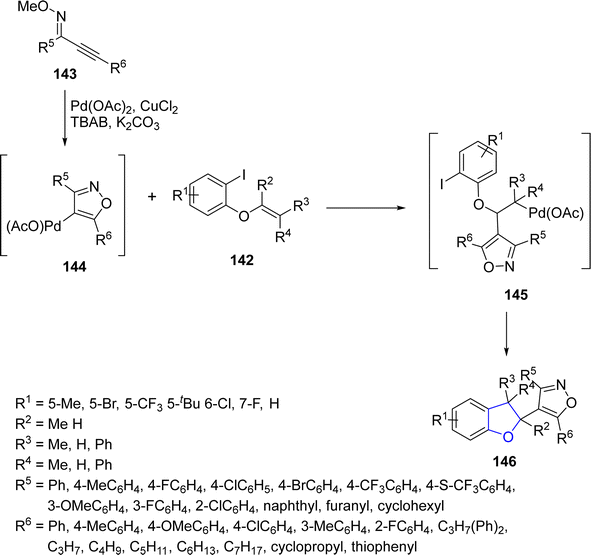 |
| Scheme 28 Synthesis of polycyclic 2-isoxazolyl-2,3-dihydrobenzofurans 146. | |
Similarly, in 2022, Houghtling86 et al. reported a palladium-catalyzed protocol for the synthesis of variety of dihydrobenzofuran derivatives 148, in which a novel urea ligand 149 was utilized to enhance the product yield (50–72%). In this context, substituted 2-bromophenols 134 were treated with 1,3-dienes 147 in the presence of NaOtBu (as base) in a 9
:
1 ratio of PhMe and anisole (used as solvent) at 110 °C (Scheme 29).
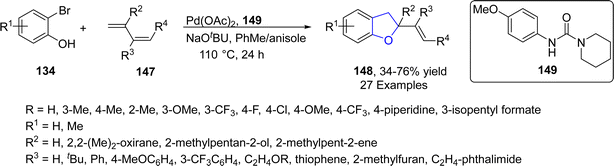 |
| Scheme 29 Synthesis of 2,3-dihydrobenzofurans 148. | |
In 2023, Sun87 et al. reported the palladium-catalyzed chiral [4 + 1] cyclization reaction to synthesize enantioselective dihydrobenzofurans 152. In their synthetic methodology, cyclic vinyl methylene ketone 151 and leaving group substituted aryl ethers 150 were subjected to a series of allylation reaction and C–H activation reaction in the presence of palladium catalyst and ligand L, utilizing cesium fluoride as a base in 2-methyl tetrahydrofuran and acetone. As a result, the enantiomeric synthesis of a library of dihydrobenzofurans 152 was achieved in 38–89% yield up to 99
:
1 enantiomeric ratio. The reaction mechanism was supposed to involve the palladium mediated decarboxylation of substituted vinyl methylene carbonate, oxidative process, O-nucleophilic conversion, asymmetric concerted metalation–deprotonation, protonation and reductive elimination to yield target molecules (Scheme 30). Various derivatives of 152 were synthesized in order to elaborate the synthetic efficiency.
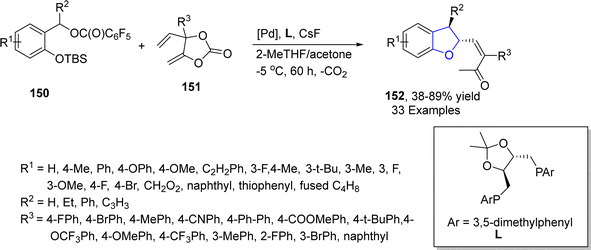 |
| Scheme 30 Synthesis of 2,3-dihydrobenzofurans 152. | |
In recent times, synthesis of various heterocycles and carbocycles have been achieved by employing alkyne cyclization. In these cyclization reactions, palladium-based catalysts have gained great significance. In 2023, Sun70 et al. reported the synthesis of dihydrobenzofurans 145 by treating tosyl hydrazones 143 and aryl-substituted joined alkynes 144 in the presence of bis(triphenylphosphine)palladium(II) dichloride catalyst, using Cs2CO3 as a base and tricyclohexylphosphine PCy3 as a ligand in toluene, followed by the addition of dienophile, i.e., dimethylbut-2-ynedioate. The reaction mechanism involved the intramolecular carbopalladation, migratory insertion, γ-hydride elimination and Diels–Alder ([4 + 2] cycloaddition) reaction to furnish the spirocyclobutane-substituted dihydrobenzofurans 145 (Scheme 31).
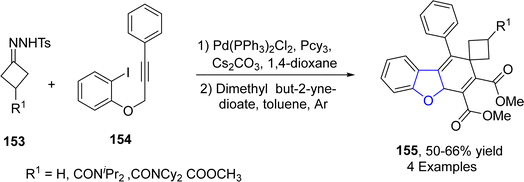 |
| Scheme 31 Synthesis of polycyclic 2,3-dihydrobenzofurans 155. | |
Wu88 et al. in 2021 developed a Pd-catalyzed protocol for the synthesis of 2,3-dihydrobenzofuran derivatives 158 via C(sp3)–H and C(sp2)–H intramolecular coupling. In their novel methodology, alkyl phenyl ethers 156 were used as efficient starting materials, which underwent subsequent C(sp3)–H and C(sp2)–H bond activation and reductive elimination in the presence of 1,4-dibenzoquinone (BQ), AgOAc and LiOAc (as base) to furnish desired heterocyclic products 158 in moderate to excellent yields (33–99%) (Scheme 32). The developed protocol was also utilized further for the gram scale synthesis of target molecules.
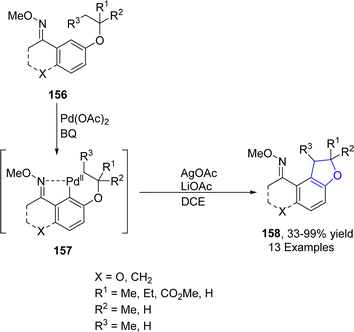 |
| Scheme 32 Synthesis of fused cyclic dihydrobenzofurans 158. | |
Reddy89 et al. in 2021 proposed a Pd-catalyzed highly efficient protocol for the preparation of 2,2,3-trisubstituted dihydrobenzofuran derivatives 162 via intramolecular condensation. In their novel methodology, 2-hydroxyphenyl-substituted enones 159 were reacted with diazo compound 160 in the presence of [Pd(cinnamyl)Cl]2 along with MeSO3H (used as an additive) to afford dihydrobenzofurans 162 in moderate to excellent yields (51–91%). Active Pd catalyst interacted with diazo compounds 160 to give carbene species, which on nucleophilic addition with 159 gave oxonium ylides that existed in equilibrium with the corresponding zwitterions 161. Zwitterions 161 were then trapped via Michael addition to afford desired products 162 (Scheme 33). Further, a number of substituted dibenzofuran products were also synthesized in order to demonstrate the synthetic efficiency of the novel approach.
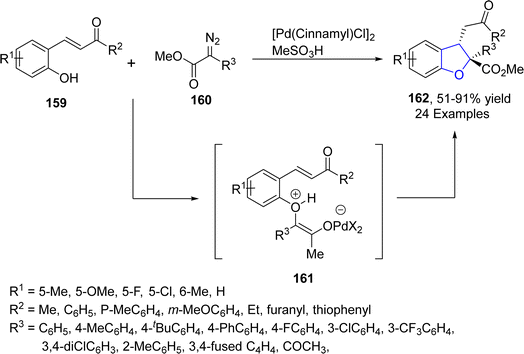 |
| Scheme 33 Synthesis of 2,2,3-trisubstituted dihydrobenzofuran derivatives 162. | |
2.3. Cu-catalyzed synthesis of 2,3-dihydrobenzofuran derivatives
Copper-based catalysts are most active, cheap and widely used catalysts in organic transformations and have various oxidation states. The reductive elimination of Cu(III) to generate C–X (X = heteroatom) bond is well studied in the literature.90 Cu-catalyzed synthesis also plays a crucial role in the synthesis of diverse dihydrobenzofuran scaffolds.91 Synthesis via deconstructive insertion reaction involving the generation of new structures via the bond cleavage of easily accessible scaffolds has recently attained growing attention from synthetic practitioners.92 In this regard, Zheng93 et al. in 2022 reported the Cu-catalyzed synthesis of pyridine-fused dihydrobenzofuran derivatives 167. In their synthetic protocol, coumarins 163 and oximes 164 were reacted in the presence of CuBr (catalyst) along with Li2CO3 (used as an additive) to afford 2,3-dihydrobenzofurans 167 in low to high yields (16–85%) via the destructive insertion of 164 into 163. This synthetic methodology provides broad functional group tolerance. Cu-assisted oxime-Cu-iminyl radical was converted into α-carbon radical, which underwent radical addition to give intermediate 165, followed by intramolecular addition to carbonyl group and oxidation to yield the desired products 167 (Scheme 34). The synthesized compounds were further transformed into a number of useful products to illustrate the proficiency of the developed synthetic route.
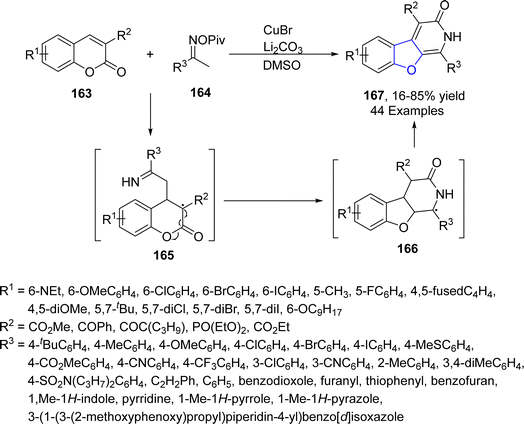 |
| Scheme 34 Synthesis of 2,3-dihydrobenzofuran-fused pyridones 167. | |
[3 + 2] cycloaddition reactions are pericyclic reactions, which involve the addition of a double bond system to a three-atom ring system. These cycloaddition reactions are amongst the most convenient synthetic protocols for the synthesis of five-membered heterocyclic ring compounds.94 Considering this, in 2021, Jing95 et al. described the synthesis of a range of enantioselective 2,3-dihydrobenzofurans 170 via the Cu/SPDO-catalyzed synthetic route. In their novel approach, quinone esters 168 and substituted styrenes 169 underwent [3 + 2] cycloaddition in the presence of SPDO-ligated Cu(OTf)2 to furnish the desired dihydrobenzofuran moieties 170 in good to excellent yields (86–96%) with extraordinary enantioselectivities (86–99% ee) (Scheme 35). Natural products 171 and 172 were also synthesized by utilizing the mentioned methodological strategy (Fig. 8).
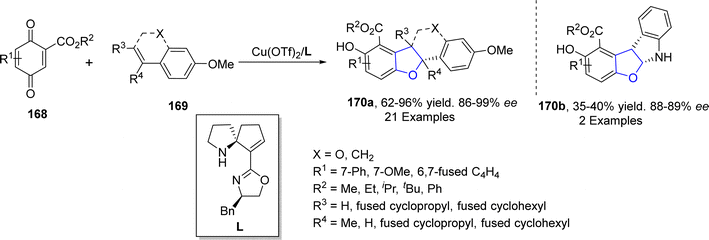 |
| Scheme 35 Synthesis of 2-aryl-2,3-dihydrobenzofurans 170. | |
 |
| Fig. 8 Structure of asymmetric natural products 171 and 172. | |
An enantioselective and diastereoselective pathway towards the synthesis of dihydrobenzofurans 163 was given by Zhu96 et al. in 2023. They exploited copper(II)/SPDO complex (chiral spirocyclic pyrrolidine (oxazoline)) catalyst for carrying out asymmetric [3 + 2] cycloaddition reaction between 2,3-dihydrofuran 173 and quinone esters 168 utilizing copper triflate Cu(OTf)2 and ligand L in toluene, wet tetrahydrofuran or mestylene solvent, to enable the asymmetric synthesis of benzofuran derivatives 175. The reaction mechanism was suggested to move forward with the formation of intermediate 174, on the coordination of substrates and catalyst. Intermediate 174 was then believed to undergo further transformations to furnish the synthesis of target molecules (Scheme 36). The developed synthetic route was further explored towards the synthesis of olefin variants-substituted dihydrobenzofurans (A & B) (Fig. 9) and naturally-occurring aflatoxins 96, i.e., (−)aflatoxin B2 96a & (−)dihydroaflatoxin D2 96b (Fig. 10).
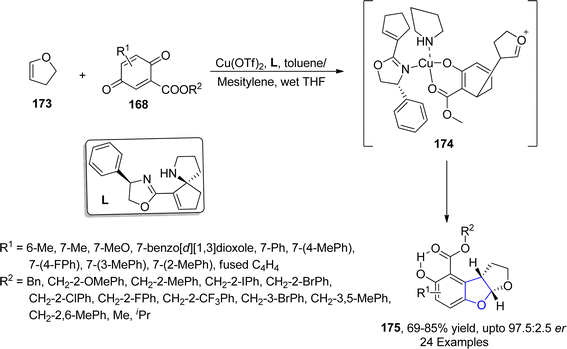 |
| Scheme 36 Synthesis of 2,3,3a,8a-tetrahydrofuro[2,3-b]benzofurans 175. | |
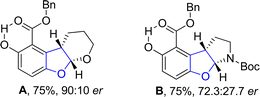 |
| Fig. 9 Structures of olefin variant-substituted dihydrobenzofurans. | |
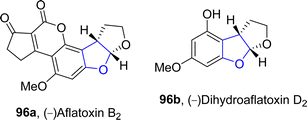 |
| Fig. 10 Structures of naturally-occurring dihydrobenzofuran derivatives 96a and 96b. | |
The five-membered O-containing compound, i.e., 2-benzylidene-1-benzofuran-3-one, called aurone, showed a number of pharmacological activities like antidiabetic, antiviral and anticarcinogenic.97 In 2021, Devi et al.98 employed copper bromide-promoted synthesis of these 2-benzylidene-1-benzofuran-3-one derivatives 179. For this purpose, they furnished chalcones 179 by treating aromatic aldehydes 177 with substituted acetophenone 176 utilizing montmorillonite K10 clay as a catalyst. The synthesized chalcones 179 were then subjected to copper bromide cyclization by employing dimethylformamide/water (7
:
3) as a solvent to attain substituted benzofuranone heterocycles 179 (Scheme 37).
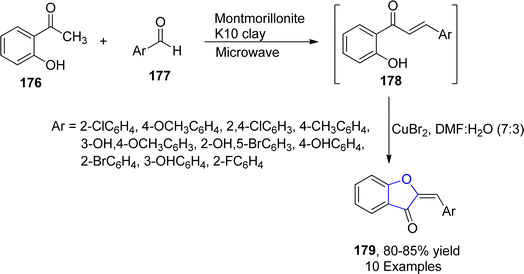 |
| Scheme 37 Synthesis of 2-benzylidene-1-benzofuran-3-ones 179. | |
In the same year, Mitsudo99 et al. designed an efficient Cu-catalyzed protocol for the synthesis of dihydrofuran-fused thienoacenes 182 and 183 via C–O cyclization. In their novel methodology, 2-(benzo[b]thiophen-2-yl)phenols 180 and 2-(benzo[b]thiophen-3-yl)phenols 181 underwent the degenerative cyclization process to afford thieno[3,2-b]furans 182 (12–86%) or thieno[2,3-b]furans 183 (53–95%) respectively. Similarly, substrates 181 afforded heteroacenes 184 and 185 having π-expanded system via double C–O cyclization. The catalytic amount of Cu(OAc)2, NaOAc (as base) and PhCOOH (as acid) were used to achieve the efficient yields of target molecules (Schemes 38 and 39).
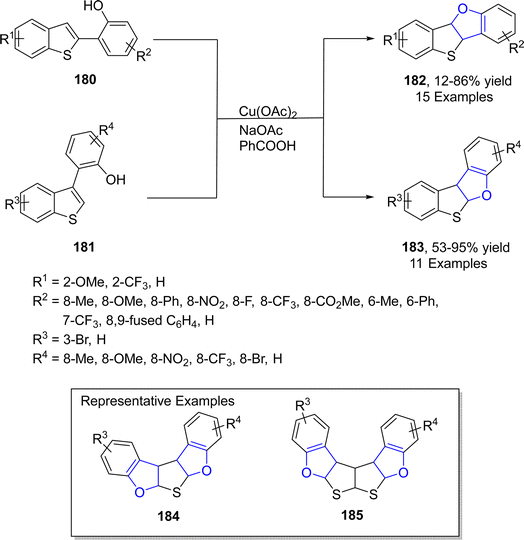 |
| Scheme 38 Synthesis of furan-fused thienoacenes 182–185. | |
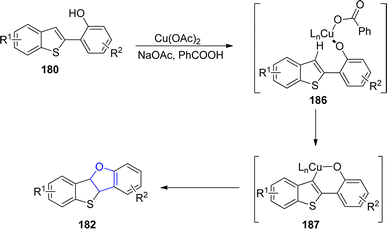 |
| Scheme 39 Proposed mechanism for the synthesis of furan-fused thienoacenes 182. | |
Oxidative cross coupling is a strategy where two phenolic moieties are coupled together via an oxidative process to generate new C–C bonds.100 Cu-catalyzed intermolecular oxidative cross coupling reactions have attracted much attention in organic synthesis to generate new dihydrobenzofuran units. In 2021, Dong101 et al. developed a biomimetic, Cu-catalyzed synthesis of neolignane analogs comprising of 2,3-dihydrobenzofuran units 190. In their novel methodology, substituted para-alkenyl phenols 188 were cross coupled with a number of electron rich phenols 20 to furnish 8-5′ neolignan correspondents 190 exclusively. Cu-assisted radical formation of phenols (188, 20) took place, which coupled to generate quinone methide intermediate 189, followed by the addition of –OH to 189 to afford the desired 2,3-dihydrobenzofuran skeletons 190 in excellent yields (24–95%) with notable diastereoselectivity values (>20
:
1 dr) (Scheme 40). Through the developed synthetic route, Licarin A 191 was also synthesized, which exhibits anti-inflammatory activity (Fig. 11).
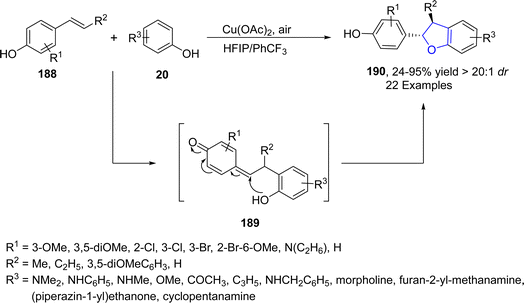 |
| Scheme 40 Synthesis of neolignan analogs comprising of 2,3-dihydrobenzofuran 190. | |
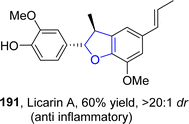 |
| Fig. 11 Structure of licarin A 191. | |
2.4. Ni-catalyzed synthesis of 2,3-dihydrobenzofuran derivatives
Nickel, which is also regarded as the “spirited horse” of TM catalysis, is a well-known metal catalyst used in organic synthesis.102 Enantioselective Ni-catalyzed reductive Heck coupling of tethered alkenes has been utilized to furnish a range of benzene-fused heterocyclic rings bearing a quaternary stereogenic center.103 In reference to this, Cerveri104 et al. in 2021, synthesized a library of asymmetric 2,3-dihydrobenzofuran-3-ylacetic acids 193 via a tandem, enantioselective Ni-catalyzed Heck cross coupling reaction. In their novel methodology, CO2 fixation took place when o-aryliodines 102 were cyclized in the presence of [(L)2Ni(H2O)Cl]Cl (used as pre-catalyst), Zn (as reducing agent), tetrabutylammonium iodide (TBAI) and trimethylsilyl chloride (TMSCl) (as additives) to afford asymmetric dihydrobenzofuran derivatives 193. Complex formation of compound 102 and NiL-precatalyst was carried out to form intermediate 192, followed by subsequent Zn-mediated reduction and CO2 insertion to afford the target compounds in moderate to high yields (47–69%) with up to 99% enantiomeric excess (Scheme 41).
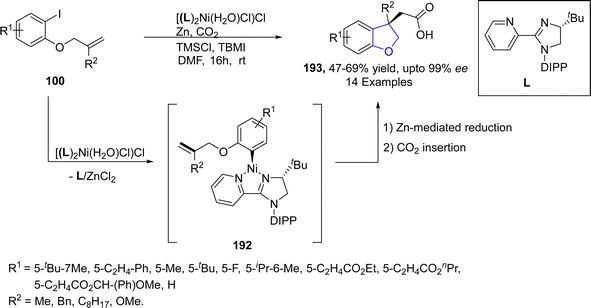 |
| Scheme 41 Synthesis of 2,3-dihydrobenzofuran-3-ylacetic acids 193. | |
Pyrano-fused cyclic organic compounds are ubiquitously employed in medicinal chemistry owing to their unique biologically active nature. There are mainly two types of these heterocycles based on the point of attachment of oxygen atom, i.e., C3–C2 & C2–C1. In 2022, Bhardwaj105 et al. reported a novel and efficient methodology by reacting enopyranoses 195 and iodine substituted phenols 194 under the action of nickel catalyst using cesium carbonate as a base in the presence of triphenylphosphine and dimethylformamide. This one-pot synthetic approach resulted in the straightforward synthesis of pyrano cis-fused dihydrobenzofurans 198 (47–75% yield). The reaction mechanism was proposed to proceed via the oxidative addition and carbometallation to form an intermediate 196, followed by β-OAc elimination and allylic rearrangement to furnish the desired dihydrobenzofurans 197 (Scheme 42).
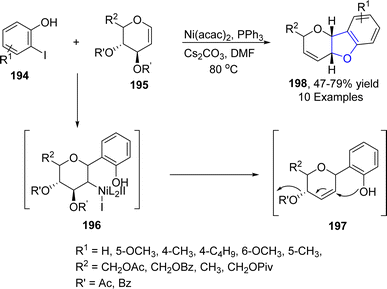 |
| Scheme 42 Synthesis of pyrano cis-fused dihydrobenzofurans 198. | |
As a key component of several dicarbon-functionalization reactions, alkene aryl-acylation or aryl-carbamoylation facilitates the synthesis of structurally significant heterocycles comprising of carbonyl compounds.106 In this respect, Wang107 et al. in 2022 described a novel approach to afford dihydrobenzofuran derivatives 200 employing nickel-catalyzed aryl carbamoylation and aryl acylation of aryl iodide-joined alkenes 124. Aryl iodide-joined alkenes 124 were subjected to treatment with aryl-substituted isocyanates 199 via Ni-catalyzed aryl carbamoylation to result in the efficient synthesis of substituted dihydrobenzofurans 200 (62–73%) (Scheme 43).
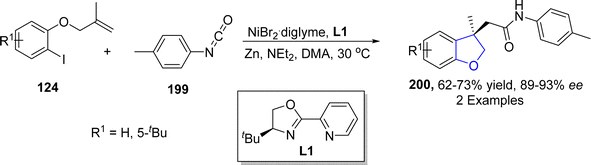 |
| Scheme 43 Synthesis of substituted dihydrobenzofurans 200. | |
In 2021, Lin108 et al. reported the Ni-catalyzed asymmetric synthesis of 2,3-dihydrobenzofuran derivatives 204 via the reductive aryl allylation process. In their synthetic approach, aryl iodides 102 were reacted with cyclic vinyl ethylene carbonates 203 to achieve cyclic products 204 in moderate to good yields (46–67%) with remarkable enantioselectivity values (>98% ee). Alkene-tethered aryl iodides 102 underwent oxidative addition with Ni catalyst to generate intermediate 201, followed by olefinic migratory insertion to form cyclic intermediate 202. Next, vinyl ethylene carbonates 203 were oxidatively added to the intermediate 202, proceeded by reductive elimination to access the desired dihydrobenzofurans 204 (Scheme 44).
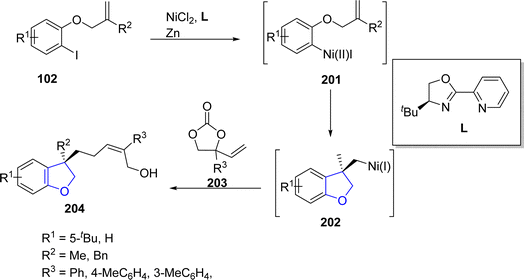 |
| Scheme 44 Synthesis of 2,3-dihydrobenzofuran 204. | |
Geng109 et al. in 2021 reported the Ni-catalyzed synthesis of 2,3-dihydrobenzofuran derivatives 207 via carbonylative synthesis. In their novel methodology, ortho-substituted aryl iodides 124 reacted with alkyl halides 205 in the presence of Ni(acac)2 (as a catalyst), 4,4′-di-tert-butyl-2,2′-bipyridyl (L) (as ligand), Mo(CO)6 (as carbonylating agent) and Mn (as reductant) to furnish desired products 207 in low to excellent yield (38–87%). In the first step, activated Ni was oxidatively added to ortho-substituted aryl iodide 124, followed by intramolecular addition to the carbonyl group and addition of alkyl halide 205 to generate intermediate 206. The intermediate 206 was further subjected to reductive elimination to afford the 2,3-dihydrobenzofuran derivatives 207 (Scheme 45).
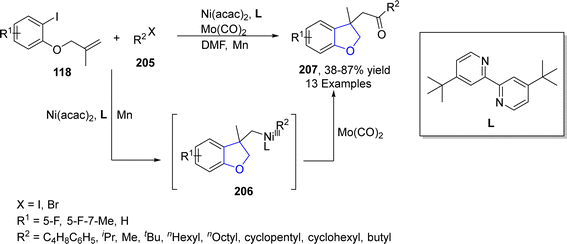 |
| Scheme 45 Synthesis 2,3-dihydrobenzofuran derivatives 207. | |
Electrification is an optimistic approach of replacing traditional energy-demanding chemical processes with greener substitutes and thus alleviating carbon emissions.110 Utilizing this strategy, Déjardin111 et al. in 2021, proposed an electroreductive, Ni-catalyzed synthesis of 2,3- dihydrobenzofuran derivatives 211 based on domino reaction. In this regard, intramolecular carbonickelation of propargylic aryl halides 208 was carried out, followed by the subsequent cyclization and nucleophilic addition of benzaldehydes 209 to furnish substituted 2,3-dihydrobenzofuran 211 (11–90%). The reaction was highly stereo and regio-selective. Maximum yield (90%) was observed when the reaction proceeded at 80 °C in the presence of DMF (as a solvent) (Scheme 46).
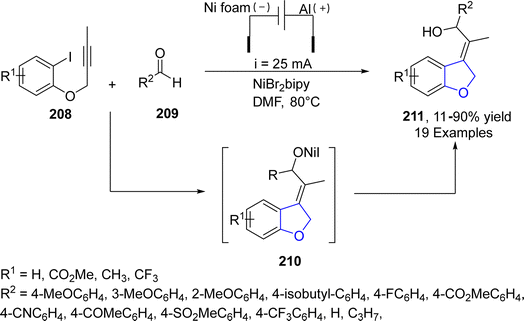 |
| Scheme 46 Synthesis of 2,3-dihydrobenzofuran derivatives 211. | |
2.5. Au-catalyzed synthesis of 2,3-dihydrobenzofuran derivatives
Au-containing catalytic systems are extensively studied in organic synthesis as they have exclusive low temperature oxidation activity. Au-catalyzed synthesis of heterocycles via [3 + 2] annulation portrays an appealing strategy as it offers complete regio- and stereo-control for introducing the functional groups. Utilizing this strategy, Liang112 et al. in 2021 described the synthesis of polycyclic dihydrobenzofuran derivatives 215 via gold and Cu-catalyzed tandem reaction. In their synthetic approach, propargyl alcohols 212 were coupled with pyridylhomopropargylic alcohols 213 via a number of processes, i.e., 5-endo–dig cyclization, Meyer–Schuster rearrangement and Friedel–Crafts-type reactions to furnish 2,3-dihydrobenzofurans 215 in low to high yields (14–81%) (Scheme 47).
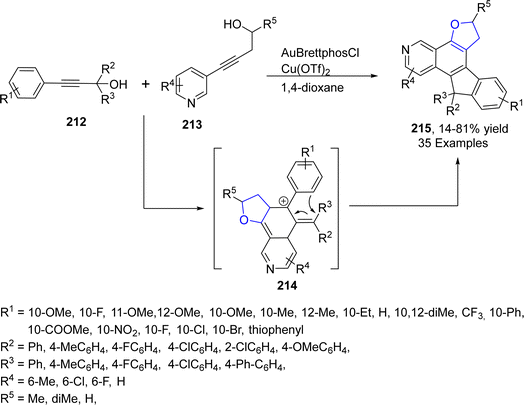 |
| Scheme 47 Synthesis of polycyclic dihydrobenzofuran derivatives 215. | |
In 2023, Morita113 et al. carried out the efficient synthesis of dihydrobenzofurans by involving gold-based NHC catalyst (permethylated β-cyclodextrin-tagged N-heterocyclic carbene–gold catalyst) in water. They utilized the NHC catalyst due to the hydrophobic nature of β-CD,114 which was expected to enhance the solubility of substrates with non-polar moieties in water. Moreover, NHC-based gold catalyst was assumed to activate the alcoholic functionality in benzylic alcohols 217 and carbonyl functionality in p-quinones 216 to facilitate the synthesis of dihydrobenzofurans 197. Substituted p-quinones 216 were treated with isoeugenols via [3 + 2] cycloaddition reaction in the presence of β-CD–NHC–AuCl catalyst, utilizing AgNTf2 (as an additive) in the excess of water to result in the efficient asymmetric synthesis of target molecules 218 in 31–81% yield (Scheme 48).
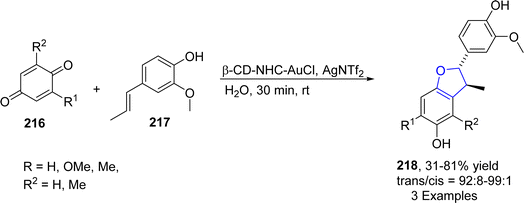 |
| Scheme 48 Synthesis of 2,3-disubstituted dihydrobenzofurans 218. | |
Wang115 et al. in 2021 accomplished the gold-catalyzed [2 + 3] cycloaddition of phenols 20 and substituted-1,3-enynes 219 to furnish dihydrobenzofuran scaffolds 221 in low to high yields (25–81%). In their synthetic methodology, the ortho-selectivity of phenols 20 was attained for the first time via electrophilic aromatic substitution employing 219 as an α-oxo vinyl gold carbenoid analogue. Catalytic amounts of tBuXPhosAuCl (phosphine ligated aurum catalyst) and 2,6-dichloropyridine N-oxide 222 (as an additive) were utilized in dichloroethane to achieve the desired products 221. Gold and ligand 222 assisted 1,3-enynes 219 underwent concerted SN2 reaction and proto-deauration upon the addition of substituted phenols 20 to generate intermediate 220. The intermediate 220 was converted to the final product 221 via oxa-Michael addition (Scheme 49).
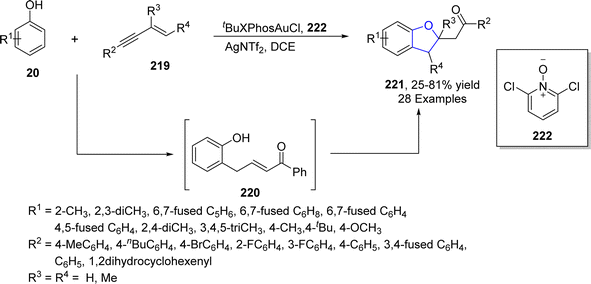 |
| Scheme 49 Synthesis of 2,3-dihydrobenzofuran derivatives 221. | |
Du116 et al. in 2023 disclosed a gold-catalyzed, one-pot construction of benzofuran-3(2H)-one skeletons 226 using the phenomenon of cycloisomerization of alkynyl phenols 223. In their novel methodology, o-alkynyl phenols 223 were reacted with substituted alcohols 224 in the presence of Ph3PAuCl (catalyst), Selectfluor (as an oxidant) and TfOH (as an additive) to afford benzofuranones 226 in moderate to good yields (22–76%). o-Alkynyl phenols 223 were activated and oxidatively added to its phenolic part to form a cyclic intermediate, which was oxidized by Selectfluor. In the next step, reductive elimination took place, followed by the nucleophilic substitution reaction to generate intermediate 225. This intermediate then underwent ketal hydrolysis to furnish the final products 226 (Scheme 50).
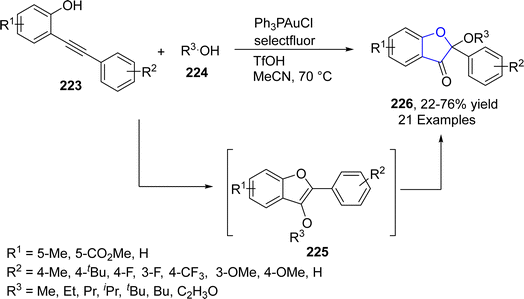 |
| Scheme 50 Synthesis of benzofuran-3(2H)-ones 226. | |
2.6. Ru-catalyzed synthesis of 2,3-dihydrobenzofuran derivatives
Ruthenium, though being the rarest earth metal, is widely used as a catalyst in organic transformations. The Ru-catalyzed synthesis of dihydrobenzofuran derivatives via C–C and C–H bond functionalization has gained tremendous attention in organic synthesis. Directing groups accompanied enantioselective functionalization of C–H bonds promoted by TM catalysts has gained significant utility in several organic transformations. In 2023, Sau117 et al. carried out the synthesis of chiral dihydrobenzofuran derivatives 228 via ruthenium-catalyzed C–H functionalization of 3-(allyloxy)benzamides 227 in the presence of AgSbF6, using copper acetate (as an additive) in 1,2-dichloroethane. The synthesized optically active dihydrobenzofurans were then subjected to treatment with substituted alkynes 36 in acetic acid to afford dihydrobenzofuran-fused isoquinolinones 228 in high enantiomeric excess (up to 97
:
3 er) along with a side product 229 (Scheme 51).
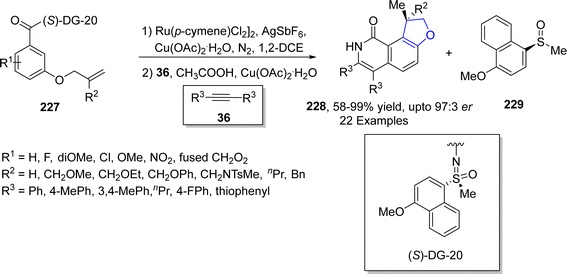 |
| Scheme 51 Synthesis of dihydrobenzofuran-fused isoquinolinones 228. | |
Similarly, Pannilawithana et al.118 utilized ruthenium catalyst-mediated synthesis of dihydrobenzofuran heterocycles by treating varied phenols 20 with substituted aldehydes 230. The coupling reaction was efficiently carried out in the presence of carbon monoxide and dichloroethane as CO addition greatly enhanced the yield of benzofuran derivatives 231 (64–93%) in comparison to alkylation products 232 (side product) (Scheme 52).
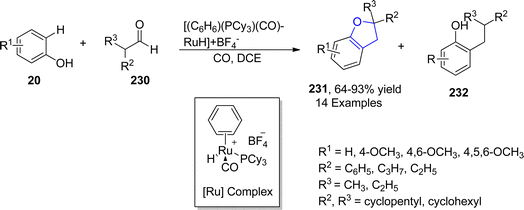 |
| Scheme 52 Synthesis of dihydrobenzofuran heterocycles 231. | |
Utilizing the annulation reaction strategy, another ruthenium-catalyzed approach for the facile synthesis of dihydrobenzofurans was demonstrated by Phukon119 et al. in 2023. In the developed synthetic methodology, substituted naphthols 234 were made to react with substituted sulfoxonium ylides 233 via the cyclization reaction, which involved the employment of 1,4-dioxane (as a source of –CH2) and copper acetate to synthesize dihydronaphthofurans 235 in 51–68% yield (Scheme 53).
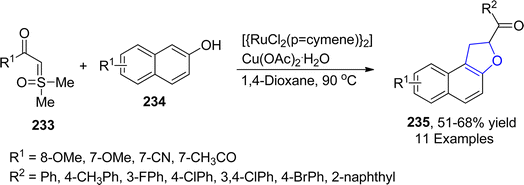 |
| Scheme 53 Synthesis of dihydronaphthofurans 235. | |
In 2021, Yuan120 et al. successfully designed a multicomponent, photoredox catalytic protocol that was applied for the construction of dihydrobenzofuran ring systems 240. For this purpose, 2-vinyl phenols 44, N-alkoxypyridinium salts 236 and sulfur ylides 237 were coupled in the presence of Ru(bpy)3Cl2·6H2O as an efficient photocatalyst along with CuI/ligand (L) in DABCO. Ru catalyst facilitated the formation of sulfonium ylide and alkoxy radical that got transformed into benzylic radical intermediate 238 by reacting with in situ formed 2-vinylphenolate. Benzylic intermediate 238 further interacted with sulfonium ylides 237 to form 239, followed by nucleophilic substitution reaction to furnish the desired cyclized products 240 in low to good yields (26–74%) (Scheme 54).
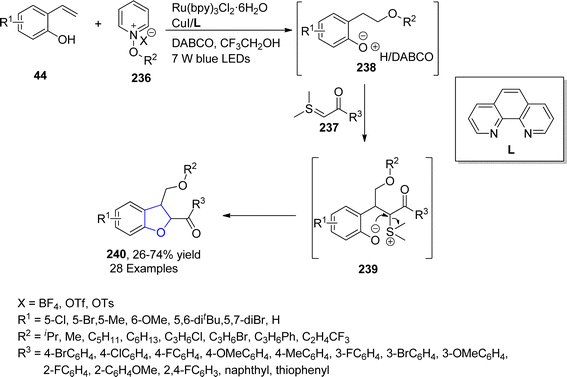 |
| Scheme 54 Synthesis of dihydrobenzofuran derivatives 240. | |
2.7. Ir-catalyzed synthesis of 2,3-dihydrobenzofuran derivatives
Iridium is the most corrosion resistance metal, which displays variable oxidation states and is utilized as a good catalyst in several organic transformations. Cross-dehydrogenative coupling (CDC) is an efficient approach to afford various organic frameworks by involving the formation of C–C bond via two inequal C–H bonds. This synthetic transformation is highly ecofriendly and requires a short duration.121 The synthesis of several sophisticated heterocycles can be achieved by the intramolecular cross-dehydrogenative coupling (first given by Fagnou and Liégault), which involves the fusion of alkyl groups to the aromatic ring. Kusaka122 et al. in 2022 presented the enantioselective synthesis of dihydrobenzofurans 242 exploiting intramolecular CDC C(sp2)–H/C(sp3)–H. They carried out this approach on silyl-based aryl ethers 241 using iridium-based (S)-DTBM-SEGPHOS as a catalyst and tert-butyl ethylene (TBE) as a hydrogen collector involving p-xylene as a solvent to furnish enantioenriched dihydrobenzofuran derivatives 243 in 53–86% yield range with up to 99% ee. The reaction was proposed to proceed via oxidative addition of 241, followed by the removal of hydrogen and ligand exchange, leading towards the synthesis of aryliridium intermediate 242. The intermediate 242 then underwent cyclization and reductive elimination to give dihydrobenzofurans 243 in high enantiomeric excess (up to 99% ee) (Scheme 55).
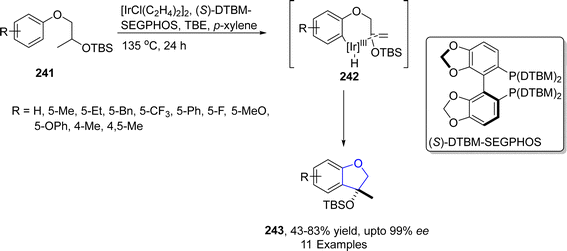 |
| Scheme 55 Synthesis of dihydrobenzofuran derivatives 243. | |
TM-catalyzed intramolecular straightforward addition of aromatic C–H bonds to olefinic bonds, named hydroarylation, has presented facile pathways towards the cyclic compounds with efficient atom-economy.123 Utilizing the intramolecular hydroarylation strategy, in 2021, Sakamoto and Nishimura124 described the Ir-catalyzed, enantioselective synthesis of 2,3-dihydrobenzofuran derivatives 248 via C–H activated intramolecular hydroarylation. In their novel methodology, they used one-pot protocol to attain desired cyclic products 248 in good to excellent yields (62–99%). Pd-catalyst was used to generate m-cinnamyloxyphenyl ketones 247 from allyl carbonate 246, followed by the Ir-catalyzed protocol to afford 2,3-dihydrobenzofuran compounds 248. A bisphosphine ligand, i.e., (S,S)-QuinoxP*, was used to attain higher enantioselectivities (>98% ee) (Scheme 56).
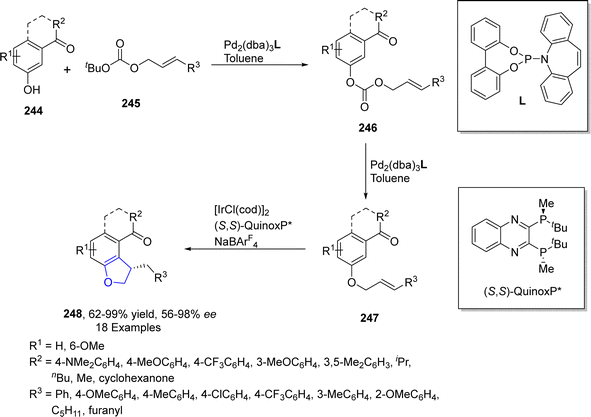 |
| Scheme 56 Synthesis of 2,3-dihydrobenzofuran derivatives 248. | |
In 2021, Ohmura125 et al. reported the synthesis of enantioselective 2,3-dihydrobenzofuran scaffolds 251 via iridium-catalyzed intramolecular hydroarylation. In their novel methodology, tert-butyldimethylsilyloxy-substituted aryl ethers 249 acted as starting materials that were transformed into dihydrobenzofurans 251 (featuring a stereogenic carbon at C3 position) in low to excellent yields (18–85%) with significant enantioselectivity values (33–99%) (Scheme 57).
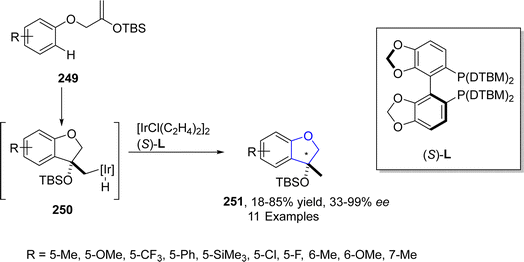 |
| Scheme 57 Synthesis of 2,3-dihydrobenzofuran 251. | |
2.8. Fe-catalyzed synthesis of 2,3-dihydrobenzofuran derivatives
Being inexpensive and earth friendly, iron (Fe) and its salts have become highly enticing. These parameters led the researchers to increase focus towards the iron-based catalysis for temperate and green reactions.126 Bashir127 et al. in 2023 proposed a facile method for the synthesis of 2,3-dihydrobenzofurans 254 via [3 + 2] cycloaddition. In this regard, substituted hydroquinones 252 were made to react with N-arylated cyclic enamines 253 in the presence of a biomimetic catalyst, i.e., hemin (an iron embedded porphyrin) with an oxidant partner, i.e., tBuOOH. Low to high yields (27–91%) were obtained by employing different catalytic loadings, CH2Cl2 (solvent) at room temperature for 10 hours (Scheme 58). The synthesized compounds were evaluated for their anti-cancerous potential against MCF-7 cancer cells, among which the compound with 2-propyne N-substitution exhibited potent IC50 value = 27.73 μM.
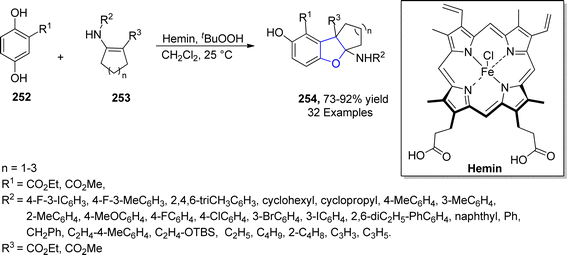 |
| Scheme 58 Synthesis of 2,3-dihydrobenzofuran 254. | |
In 2021, Zhang128 et al. reported the iron(III)-mediated, free radical-catalyzed cascade protocol for the synthesis of naphthodihydrofurans 258. In their novel approach, naphthoquinones 255 were reacted with allyl alcohols 256 in the presence of Fe(acac)3 and Ph2SiH2 (used as reductant) in EtOH to access naphthodihydrofurans 258 in moderate to excellent yields (47–93%). Alkyl radical formed from 256 and naphthoquinones 255 underwent Michael addition and single electron transfer process to form intermediate 257, which was dehydrated and cyclized to yield desired products 258 (Scheme 59). The gram scale synthesis of 258 was performed to scale up the reaction and structurally important 3,3-dimethyl-2,3-dihydronaphtho[1,2-b]furan-4,5-diones were also generated from the synthesized naphthodihydrofurans 258.
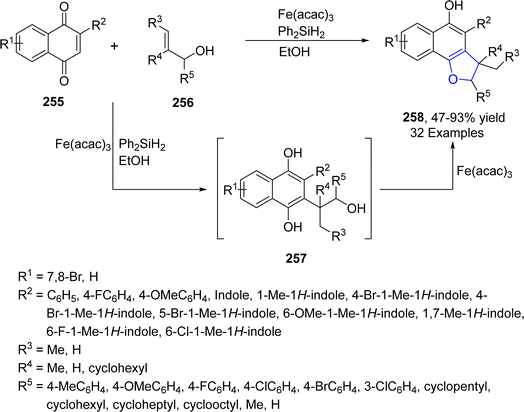 |
| Scheme 59 Synthesis of naphthodihydrofurans 258. | |
2.9. Ag-catalyzed synthesis of 2,3-dihydrobenzofuran derivatives
Ag-catalyzed [3 + 2] annulation reactions are the most convenient synthetic strategies for the synthesis of five-membered heterocyclic ring compounds.94 In this context, in 2023, Guo129 et al. executed visible-light promoted, additive-free synthesis of dihydrobenzofurans 261 and 262. They treated substituted phenols 20 with styrenes 259 and N-acylindoles 260 in the presence of nanoparticles-based silver phosphate (Ag3PO4) in dichloromethane or HFIP solvent, to accomplish the synthesis of dihydrobenzofurans and indolines constituting dihydrobenzofurans 261 and 262, respectively. This visible light-induced synthetic route proved to be high yielding and efficient due to the recyclable nature of nanoparticles-supported silver phosphate catalyst. On the catalytic surface, the stabilized radical cations of both substrates underwent [3 + 2] cross coupling reaction to yield the target molecules. Initially, the radical formation of substrates took place, which resulted in the formation of the radical cation intermediate. This intermediate radially eliminates a proton to furnish the desired 2,3-dihydrobenzofuran derivatives 261 and 262 (Scheme 60). The proposed mechanism of the reaction was supposed to be initiated with the formation of radical species 265, which cross coupled with the radical cation of 259 to form an intermediate 266. Finally, this intermediate led to the synthesis of target molecules 261 upon deprotonation and cyclization (Scheme 61).
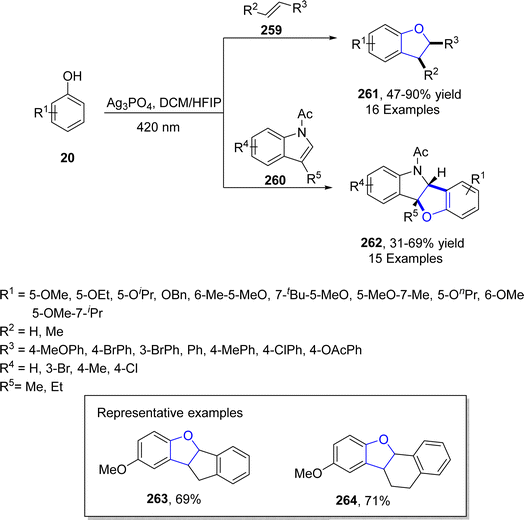 |
| Scheme 60 Synthesis of dihydrobenzofurans 261 and 264. | |
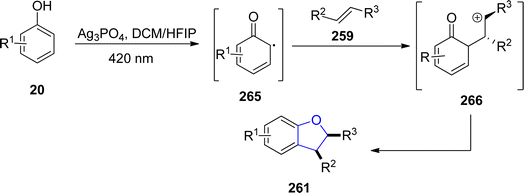 |
| Scheme 61 Proposed mechanism for the synthesis of dihydrobenzofurans 261. | |
In 2021, Dias and coworkers130 also optimized a silver-catalyzed method for the synthesis of 2,3-dihydrobenzofuran neolignans 269 and 270 via oxidative coupling strategy. In their synthetic approach, methyl p-coumarates 267 and methyl ferulates 268 were transformed into 269 and 270, respectively, in the presence of Ag2O (used as catalyst) and azobisisobutyronitrile (as radical inhibitor) in acetonitrile. These conditions furnished significant stability between conversion and selectivity (with 45% conversion and more than 71% selectivity) of dihydrobenzofurans 269 and 270 (Scheme 62).
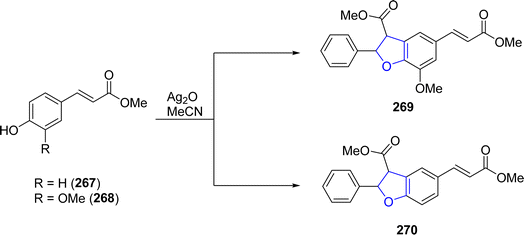 |
| Scheme 62 Synthesis of 2,3-dihydrobenzofuran neolignans 269 and 270. | |
2.10. Pt-catalyzed synthesis of 2,3-dihydrobenzofuran derivatives
Platinum (Pt) catalysts depict astounding capacity as a carbon monoxide (CO) oxidation catalyst in the catalytic converter on account of their exceptional stability and tailoring adaptability. They are also widely utilized as electrodes in electrosynthesis. Electrosynthesis has gained significant weightage in organic synthesis owing to the green applicability of electrons in proceeding redox reactions. However, its industrial usage is limited due to the bulk waste generation as a result of using concentrated supporting electrolytes.131 Regarding to this, Okamoto132 et al. in 2023 reported the synthesis of dihydrobenzofuran derivatives 271 by employing an electrochemical approach utilizing ultra-low electrolyte concentration (0.001–0.01 M). For the first time, they treated alkenes 21 and phenols 20 via electrochemically-induced [3 + 2] cycloaddition in the presence of flow microreactor using HFIP as the solvent. Bu4NPF6 (tetrabutylammonium hexafluorophosphate) was added with dichloromethane and acetic acid to be used as an electrolyte in the chemical reaction. Their synthetic route was highly productive and environment friendly owing to the complete elimination of waste production, thereby giving substituted dihydrobenzofurans 271 in 21–91% yield. Phenoxonium cations of 20 were formed anodically, which were further reacted with 21 to afford 2,3-dihydrobenzofuran derivatives 271 (Scheme 63).
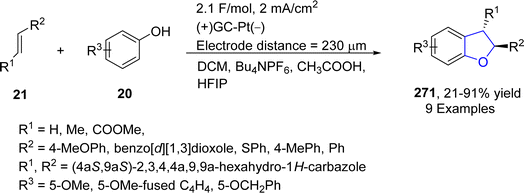 |
| Scheme 63 Synthesis of dihydrobenzofuran 271. | |
In 2023, Guan's133 group reported another catalyst free, environmentally benign electrochemical synthesis of dihydrobenzofurans 275. They treated substituted aminophenols 272 with diverse variety of olefins via an electrochemically-mediated approach in the presence of nBu4NBF4 in acetonitrile solvent to carry out the synthesis of target molecules in effective yields (33–99%). The developed route was found to be highly economical as it avoids the use of any catalyst, oxidizing agent and additive. The proposed mechanism of this reaction involved the anodic oxidation of substituted aminophenols 272 via single electron transfer, followed by an addition reaction with alkenes 273 to form intermediate 274. The resulting intermediate was then supposed to form the carbocation, which ultimately afforded dihydrobenzofurans 275, followed by intramolecular cyclization (Scheme 64).
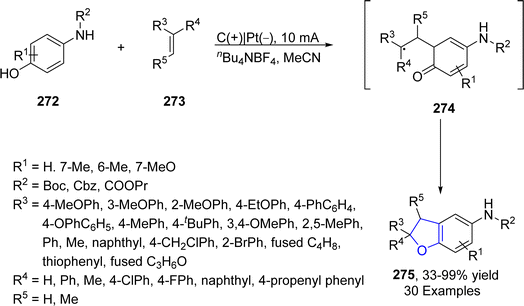 |
| Scheme 64 Synthesis of dihydrobenzofurans 275. | |
2.11. V-catalyzed synthesis of 2,3-dihydrobenzofuran derivatives
Vanadium (V), being a transition metal, has the capability to change its oxidation state and is used as an efficient catalyst for many reaction systems. Utilizing the V-catalyzed synthetic protocol, Wang134 et al. in 2023, treated the substituted aryl acetates 277 and dihydroxy substituted naphthoic acid esters 276 via the [3 + 2] cascade reaction to afford dihydrobenzofuran derivatives 279 in 20–88% yield. The reaction was executed in the presence of catalytic isothiourea and vanadium oxide acetate utilizing hydrogen peroxide, n-Bu4NHSO4 and B(OMe)3 in 1,4-dioxane. The postulated mechanism involved the formation of C1-ammonium enolate and removal of proton, followed by Michael addition and lactonization to furnish dihydrobenzofuran derivatives 279 (Scheme 65).
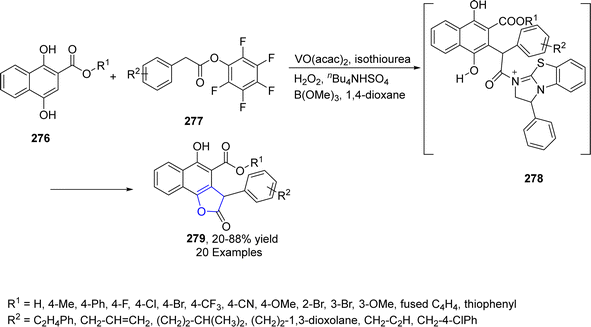 |
| Scheme 65 Synthesis of polycyclic dihydrobenzofuran 279. | |
2.12. W-catalyzed synthesis of 2,3-dihydrobenzofuran derivatives
Owing to their inexpensive and reusable characteristics, tungsten (W) catalysts are abundantly used in organic synthesis. Another visible-light promoted synthetic route to achieve dihydrobenzofurans was given in 2023 by Gowda135 et al. For this purpose, diversely substituted aromatic aldehydes 281 were made to react with alkynyl aryl ethers 280 exploiting TBADT (tetrabutylammonium decatungstate), which is a photocatalyst, responsible for carrying out 1,5-hydrogen atom transfer. The reaction protocol involved the use of visible light (390 nm) in acetonitrile solvent to afford dihydrobenzofurans 283 (in 48–76% yield range) via the activation of C–H bond without any addition of additive. The plausible mechanism of this reaction was believed to include the excitation of photocatalyst via visible light, followed by the removal of proton from aromatic aldehydes 281 to give the acyl radical. The resulting radical was then assumed to execute the addition reaction with alkynyl aryl ethers 280 proceeded by 1,5-transfer of hydrogen atom to give rise to intermediate 282. This intermediate then finally gave substituted dihydrobenzofurans 283 via exo–dig radical involving cyclization and subsequent reduction (Scheme 66).
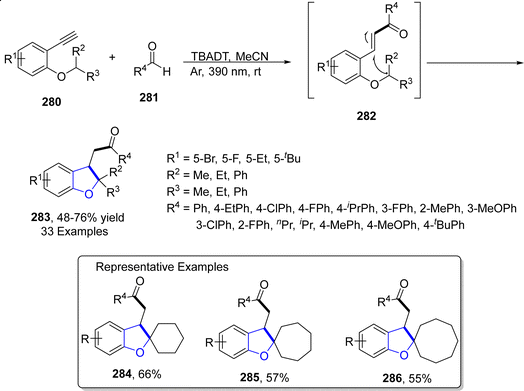 |
| Scheme 66 Synthesis of dihydrobenzofuran derivatives 283. | |
2.13. Co-catalyzed synthesis of 2,3-dihydrobenzofuran derivatives
Cobalt (Co)-based catalyst have high reducibility, high oxygen mobility and thermal stability due to which they are extensively studied and used in the field of organic synthesis. Tian136 et al. in 2021 reported a facile one-pot, photosensitized and cobalt-catalyzed approach for the synthesis of 2,3-dihydrobenzofuran derivatives 289. In their synthetic methodology, 2-propynolphenols 287 underwent semi-hydrogenation and intramolecular cyclization in the presence of a photosensitizer, i.e., Ir[dF(CF3)ppy]2(dtbbpy)PF6 and a cobalt catalyst (Co(OAc)2·4H2O), to access 2,3-dihydrobenzofuran units 289. This methodology covers diverse functionalities (18 examples) and gave moderate to excellent yields (54–98%). Phenolic hydroxyl played a vital role for the intramolecular cyclization with (Z)-alkene, which was transformed from alkyne group of 287. As per the suggested mechanism, activated Co species underwent hydrometallation with alkyne part of 287, proceeded by protonation and oxidative addition (with phenolic hydroxyl group). The resulting intermediate was converted into intermediate 288 on migratory insertion and yielded the final products 289 via reductive elimination (Scheme 67).
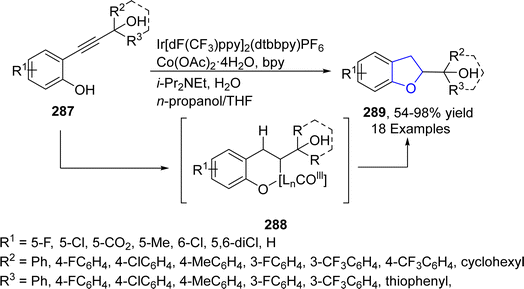 |
| Scheme 67 Synthesis of dihydrobenzofuran derivatives 289. | |
2.14. Dual metal-catalyzed synthesis of 2,3-dihydrobenzofuran derivatives
2.14.1. Pd and Cu-catalyzed synthesis of 2,3-dihydrobenzofuran derivatives. Phthalans are structurally dihydrobenzofurans constituting organic scaffolds, which are of significant contribution in the pharmaceutical industry. In 2022, Huang137 et al. reported a facile approach to procure a diverse range of substituted phthalans 294 utilizing Sonogashira coupling. Their synthetic strategy involved the reaction of substituted aryl halides 290, nucleophiles 291 and substituted triynes 292 in the presence of catalytic amount of palladium tetrakis(triphenylphosphine) and copper iodide using diisopropyl amine as the base in tetrahydrofuran. The reaction was assumed to propagate via oxidative addition and palladium promoted coupling, followed by reductive elimination and base-catalyzed propargyl–allenyl isomerization to generate intermediate 293. Intermediate 293 was then supposed to undergo PDDA cyclization, followed by subsequent regioselective nucleophilic addition to construct a wide variety of 1,3-dihydroisobenzofurans (phthalans) 294 in moderate to excellent yields (40–92%) (Scheme 68).
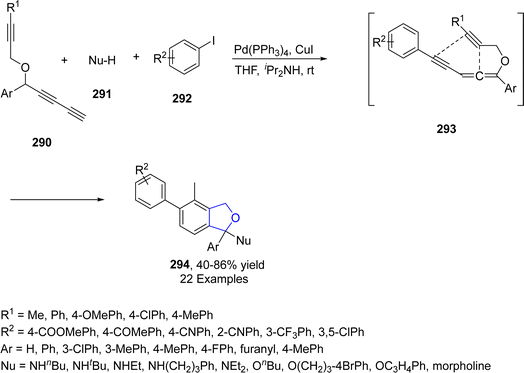 |
| Scheme 68 Synthesis of substituted phthalans 294. | |
2.14.2. Pd and Ni-catalyzed synthesis of 2,3-dihydrobenzofuran derivatives. Dual catalysis is one of the most dynamic strategies for the advancement of chemical reactions in organic synthesis. Lian138 et al. in 2022 devised a dual catalytic protocol to synthesize 2,3-dihydrobenzofurans 298 containing gem-difluorovinyl framework. In their synthetic approach, the coupling of gem-difluorovinyl tosylates 296 and alkynyl bromoarenes 295 occurred in the presence of Pd and Ni catalyst along with ZnI2 (as an additive) and Zn (as reductant) in DMF at 100 °C. As interpreted by the proposed mechanism, alkynyl bromoarenes 295 underwent subsequent oxidative addition and cyclization process in the presence of Pd(0) species. Next, Ni(0)-catalyzed oxidative addition of gem-difluorovinyl tosylates 296 took place, proceeded by the transmetallation step to generate gem-difluorovinyl zinc intermediate, which interacted with Pd-mediated alkynyl bromoarenes species to form intermediate 297. This intermediate underwent reductive elimination to furnish the desired 2,3-dihydrobenzofuran derivative 298 (Scheme 69).
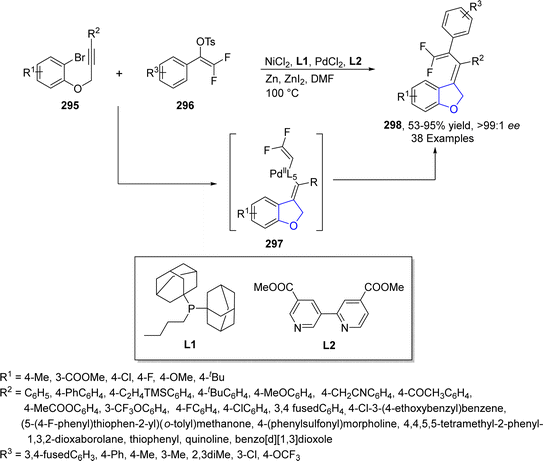 |
| Scheme 69 Synthesis of 2,3-dihydrobenzofurans 298. | |
2.14.3. Ti and Ni-catalyzed synthesis of 2,3-dihydrobenzofuran derivatives. Ni-catalyzed stereoselective reductive dicarbofunctionalization of alkenes is the most facile approach for the rapid generation of several asymmetric cyclic frameworks.139 Similarly, various catalysts comprising of titanium (Ti) play a crucial role in the development of dihydrobenzofuran core structure.140 In 2023, Zhao141 et al. also utilized haloarene-substituted alkenes 300 for the asymmetric synthesis of dihydrobenzofurans 301. They reacted aromatic rings-joined alkenes with substituted benzyl alcohols 299 by employing Ni(COD)2 and titanium chloride (TiCl4) as catalysts in the presence of ligand, manganese, 2,6-lutidine in tetrahydrofuran to achieve the enantioenriched dihydrobenzofurans 301 in 31–51% yield with 75–87% ee (Scheme 70). The synthetic strategy was also utilized to furnish the dihydrobenzofuran derivatives 302 and 303 from clinically approved drugs, i.e., bexarotene (used against T-cell lymphoma cancer) and probenecid (used in the treatment of gout), respectively.
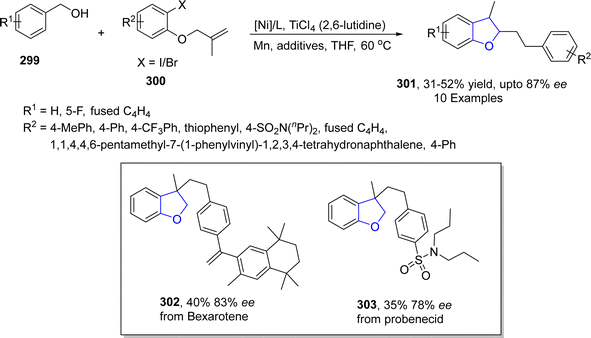 |
| Scheme 70 Synthesis of 2,3-dihydrobenzofurans 301. | |
3 Conclusion
This review article elucidates the latest developments in the transition metal-catalyzed synthesis of polysubstituted dihydrobenzofuran derivatives. Due to their exemplary output, cost effectiveness, and diversification, transition metal-mediated one-pot synthesis involving cutting-edge methodologies, i.e., multiple C–C/C–O bond-forming processes in an intermolecular or intramolecular approach, annulation and insertion reactions, have been rigorously explored towards the accomplishment of these heterocycles. Various transition metals, i.e., Rh, Pd, Cu, Co, Ni, Fe, Ag, Au, W, Ir, Pt, V and Ru-catalyzed robust methodologies have been scrutinized towards the synthesis of 2,3-dihydrobenzofurans derivatives along with their mechanistic details. It is aimed that this communication, by providing a comprehensive perspective of the synthetic protocols available, will assist to provoke attention in the study of dihydrobenzofurans in pharmaceutical and medicinal chemistry.
Conflicts of interest
There are no conflicts to declare.
Acknowledgements
A. Irfan extends his appreciation to the Deanship of Research and Graduate Studies at King Khalid University for funding this work through Large Research Project under grant number (RGP2/130/45). A. R. Chaudhry is thankful to the Deanship of Graduate Studies and Scientific Research at the University of Bisha, for supporting this work through the Fast-Track Research Support Program.
References
- A. Radadiya and A. Shah, Bioactive Benzofuran Derivatives: An insight on Iead Developments, Radioligands and Advances of the Last Decade, Eur. J. Med. Chem., 2015, 97, 356–376 CrossRef CAS PubMed.
- R. J. Nevagi, S. N. Dighe and S. N. Dighe, Biological and Medicinal Significance of Benzofuran, Eur. J. Med. Chem., 2015, 97, 561–581 CrossRef CAS PubMed.
- R. Munir, A. F. Zahoor, U. Nazeer, M. A. Saeed, A. Mansha, A. Irfan and M. U. Tariq, Gilman Reagent Toward the Synthesis of Natural Products, RSC Adv., 2023, 13(50), 35172–35208 RSC.
- H. Qin, Z. Xu, Y. Cui and Y. Jia, Total Synthesis of (±)-Decursivine and (±)-Serotobenine: A Witkop Photocyclization/Elimination/O-Michael Addition Cascade Approach, Angew. Chem., Int. Ed., 2011, 19(50), 4447–4449 CrossRef PubMed.
- D. H. Wang and J. Q. Yu, Highly Convergent Total Synthesis of (+)-Lithospermic Acid via a Late-Stage Intermolecular C–H Olefination, J. Am. Chem. Soc., 2011, 133(15), 5767–5769 CrossRef CAS PubMed.
- Q. Huang, P. Wang, Y. Tian, N. Song, S. Ren, J. Tao, K. Hang and M. Li, Synthesis of (±)-Pterocarpin and its Thia-and Aza-Analogues in a Modular Manner, Synlett, 2015, 26(10), 1385–1390 CrossRef CAS.
- Y. Natori, H. Tsutsui, N. Sato, S. Nakamura, H. Nambu, M. Shiro and S. Hashimoto, Asymmetric synthesis of Neolignans (−)-Epi-conocarpan and (+)-Conocarpan via Rh (II)-Catalyzed C−H Insertion Process and Revision of the Absolute Configuration of (−)-Epi-Conocarpan, J. Org. Chem., 2009, 74(11), 4418–4421 CrossRef CAS PubMed.
- C. W. am Ende, Z. Zhou and K. A. Parker, Total Synthesis of (±)-Bisabosqual A, J. Am. Chem. Soc., 2013, 135(2), 582–585 CrossRef CAS PubMed.
- N. E. Wright and S. A. Snyder, 9-Membered Carbocycle Formation: Development of Distinct Friedel–Crafts Cyclizations and Application to a Scalable Total Synthesis of (±)-Caraphenol A, Angew. Chem., Int. Ed., 2014, 53(13), 3409–3413 CrossRef CAS PubMed.
- S. Panthee, S. Takahashi, H. Takagi, T. Nogawa, E. Oowada, M. Uramoto and H. Osada, Furaquinocins I and J: Novel Polyketide Isoprenoid Hybrid Compounds From Streptomyces Reveromyceticus SN-593, J. Antibiot., 2011, 64(7), 509–513 CrossRef CAS PubMed.
- Y. F. Qiaos, K. Takeya, H. Itokawa and Y. Iitaka, Three Novel Naphthohydroquinone Dimers from Rubia Oncotricha, Chem. Pharm. Bull., 1990, 38(10), 2896–2898 CrossRef.
- S. Eckhardt, Recent progress in the development of anticancer agents, Curr. Med. Chem.: Anti-Cancer Agents, 2002, 2(3), 419–439 CrossRef CAS PubMed.
- I. Shahzadi, A. F. Zahoor, A. Rasul, N. Rasool, Z. Raza, S. Faisal, B. Parveen, S. Kamal, M. Zia-ur-Rehman and F. M. Zahid, Synthesis, anticancer, and computational studies of 1, 3, 4-oxadiazole-purine derivatives, J. Heterocycl. Chem., 2020, 57(7), 2782–2794 CrossRef CAS.
- I. Shahzadi, A. F. Zahoor, A. Rasul, A. Mansha, S. Ahmad and Z. R. Synthesis, hemolytic studies, and in silico modeling of novel acefylline–1, 2, 4-triazole hybrids as potential anti-cancer agents against MCF-7 and A549, ACS Omega, 2021, 6(18), 11943–11953 CrossRef CAS PubMed.
- V. Gandin, P. Khalkar, J. Braude and A. P. Fernandes, Organic selenium compounds as potential chemotherapeutic agents for improved cancer treatment, Free Radical Biol. Med., 2018, 127, 80–97 CrossRef CAS PubMed.
- R. Akhtar, A. F. Zahoor, A. Rasul, M. Ahmad, M. N. Anjum, M. Ajmal and Z. Raza, Design, synthesis, in silico study and anticancer potential of novel n-4-piperazinyl-ciprofloxacin-aniline hybrids, Pak. J. Pharm. Sci., 2019, 32(5), 2215–2222 Search PubMed.
- T. Otani, Y. Sugimoto, Y. Aoyagi, Y. Igarashi, T. Furumai, N. Saito, T. Asao and T. Oki, New Cdc25B Tyrosine Phosphatase Inhibitors, Nocardiones A and B, Produced by Nocardia sp. TP-A0248: Taxonomy, Fermentation, Isolation, Structural Elucidation and Biological Properties, J. Antibiot., 2000, 53(4), 337–344 CrossRef CAS PubMed.
- W. Chen, X. D. Yang, Y. Li, L. J. Yang, X. Q. Wang, G. L. Zhang and H. B. Zhang, Design, Synthesis and Cytotoxic Activities of Novel Hybrid Compounds Between Dihydrobenzofuran and Imidazole, Org. Biomol. Chem., 2011, 9(11), 4250–4255 RSC.
- S. Van Miert, S. Van Dyck, T. J. Schmidt, R. Brun, A. Vlietinck, G. Lemière and L. Pieters, Antileishmanial Activity, Cytotoxicity and QSAR Analysis of Synthetic Dihydrobenzofuran Lignans and Related Benzofurans, Bioorg. Med. Chem., 2005, 13(3), 661–669 CrossRef CAS PubMed.
- N. Negoro, S. Sasaki, S. Mikami, M. Ito, M. Suzuki, Y. Tsujihata, R. Ito, A. Harada, K. Takeuchi, N. Suzuki, J. Miyazaki, T. Santou, T. Odani, N. Kanzaki, M. Funami, T. Tanaka, A. Kogame, S. Matsunaga, T. Yasuma and Y. Momose, Discovery of TAK-875: A Potent, Selective, and Orally Bioavailable GPR40 agonist, ACS Med. Chem. Lett., 2010, 1(6), 290–294 CrossRef CAS PubMed.
- R. P. Tripathi, A. K. Yadav, A. Ajay, S. S. Bisht, V. Chaturvedi and S. K. Sinha, Application of Huisgen (3+ 2) Cycloaddition Reaction: Synthesis of 1-(2, 3-Dihydrobenzofuran-2-yl-methyl [1, 2, 3]-triazoles) and their Antitubercular Evaluations, Eur. J. Med. Chem., 2010, 45(1), 142–148 CrossRef CAS PubMed.
- J. E. Frampton, Prucalopride, Drugs, 2009, 69, 2463–2476 CrossRef CAS PubMed.
- C. B. Chapleo, P. L. Myers, R. C. Butler, J. A. Davis, J. C. Doxey, S. D. Higgins, M. Myers, A. G. Roach and C. F. Smith, Alpha-Adrenoceptor Reagents. 2. Effects of Modification of the 1, 4-benzodioxan Ring System on Alpha-Adrenoreceptor Activity, J. Med. Chem., 1984, 27(5), 570–576 CrossRef CAS PubMed.
- Z. Chen, W. Huang, Y. Su, H. Jiang and W. Wu, Lewis/Brønsted Acid-Mediated Cyclization/Amidation of 1, 6-enynes with Nitriles: Access to 3-enamide Substituted Dihydrobenzofurans, Chem. Commun., 2023, 59(30), 4523–4526 RSC.
- H. Rouh, Y. Tang, T. Xu, Q. Yuan, S. Zhang, J. Y. Wang, S. Jin, Y. Wang, J. Pan, H. L. Wood, J. D. Mcdodald and G. Li, Aggregation-induced synthesis (AIS): asymmetric synthesis via chiral aggregates, Research, 2022, 2022, 9865108 CrossRef CAS PubMed.
- S. Zhou, B. Cai, C. Hu, X. Cheng, L. Li and J. Xuan, Visible Light and Base Promoted OH Insertion/Cyclization of Para-Quinone Methides with Aryl Diazoacetates: An Approach to 2, 3-Dihydrobenzofuran Derivatives, Chin. Chem. Lett., 2021, 32(8), 2577–2581 CrossRef CAS.
- M. Xiang, C. Y. Li, J. Zhang, Y. Zou, Z. C. Huang, W. S. Li, Y. Wang, F. Tian and L. X. Wang, Organocatalyst-promoted Diastereoselective and Enantioselective Michael Addition/Hemiketalization Reaction between Hydroxymaleimide and Quinone, Asian J. Org. Chem., 2021, 10(7), 1713–1717 CrossRef CAS.
- K. Röser, A. Scheucher, C. Mairhofer, M. Bechmann and M. Waser, Oxidative Decarboxylative Ammonium Hypoiodite-catalysed Dihydrobenzofuran Synthesis, Org. Biomol. Chem., 2022, 20(16), 3273–3276 RSC.
- K. M. Dawood and T. Fuchigami, Electrochemical Partial Fluorination of Organic Compounds. 74. Efficient Anodic Synthesis of 2-Fluoro-and
2, 3-Difluoro-2, 3-dihydrobenzofuran Derivatives, J. Org. Chem., 2004, 69(16), 5302–5306 CrossRef CAS PubMed.
- K. S. O'Callaghan, D. Lynch, M. Baumann, S. G. Collins and A. R. Maguire, Flow Photolysis of Aryldiazoacetates Leading to Dihydrobenzofurans Via Intramolecular C–H Insertion, Org. Biomol. Chem., 2023, 21(23), 4770–4780 RSC.
- L. Gonnard, A. Guérinot and J. Cossy, Transition Metal-Catalyzed α-Alkylation of Amines by C(sp3)–H Bond Activation, Tetrahedron, 2019, 75(2), 145–163 CrossRef CAS.
- T. R. Blum, Y. Zhu, S. A. Nordeen and T. P. Yoon, Photocatalytic Synthesis of Dihydrobenzofurans by Oxidative [3+ 2] Cycloaddition of Phenols, Angew. Chem., 2014, 126(41), 11236–11239 CrossRef.
- S. S. Sakate, S. H. Shinde, G. B. Kasar, R. C. Chikate and C. V. Rode, Cascade Synthesis of Dihydrobenzofuran via Claisen Rearrangement of Allyl Aryl Ethers Using FeCl3/MCM-41 Catalyst, J. Saudi Chem. Soc., 2018, 22(4), 396–404 CrossRef CAS.
- M. C. Henry, H. M. Senn and A. Sutherland, Synthesis of Functionalized Indolines and Dihydrobenzofurans by Iron and Copper Catalyzed Aryl C–N and C–O Bond Formation, J. Org. Chem., 2018, 84(1), 346–364 CrossRef PubMed.
- T. Ohmura, S. Kusaka, T. Torigoe and M. Suginome, Iridium-Catalyzed C(sp3)–H Addition of Methyl Ethers across Intramolecular Carbon–Carbon Double Bonds Giving 2, 3-Dihydrobenzofurans, Adv. Synth. Catal., 2019, 361(19), 4448–4453 CrossRef CAS.
- C. Ni, J. Gao and X. Fang, Cu (i)-Catalyzed Asymmetric Intramolecular Addition of Aryl Pinacolboronic Esters to Unactivated Ketones: Enantioselective Synthesis of 2, 3-Dihydrobenzofuran-3-ol Derivatives, Chem. Commun., 2020, 56(17), 2654–2657 RSC.
- Y. Li, W. Li, J. Tian, G. Huang and H. Lv, Nickel-Catalyzed Asymmetric Addition of Aromatic Halides to Ketones: Highly Enantioselective Synthesis of Chiral 2, 3-Dihydrobenzofurans Containing a Tertiary Alcohol, Org. Lett., 2020, 22(14), 5353–5357 CrossRef CAS PubMed.
- R. K Rit, K. Ghosh, R. Mandal and A. K. Sahoo, Ruthenium-Catalyzed Intramolecular Hydroarylation of Arenes and Mechanistic Study: Synthesis of Dihydrobenzofurans, Indolines, and Chromans, J. Org. Chem., 2016, 81(18), 8552–8560 CrossRef PubMed.
- S. Agasti, S. Maity, K. J. Szabo and D. Maiti, Palladium-Catalyzed Synthesis of 2, 3-Disubstituted Benzofurans: An Approach Towards the Synthesis of Deuterium Labeled Compounds, Adv. Synth. Catal., 2015, 357(10), 2331–2338 CrossRef CAS PubMed.
- M. Szlosek-Pinaud, P. Diaz, J. Martinez and F. Lamaty, Efficient Synthetic Approach to Heterocycles Possessing the 3, 3-Disubstituted-2,3-Dihydrobenzofuran Skeleton via Diverse Palladium-Catalyzed Tandem Reactions, Tetrahedron, 2007, 63(16), 3340–3349 CrossRef CAS.
- L. Yang, X. Liang, Y. Ding, X. Li, X. Li and Q. Zeng, Transition Metal-Catalyzed Enantioselective Synthesis of Chiral Five-and Six-Membered Benzo O-heterocycles, Chem. Rec., 2023, 23(11), e202300173 CrossRef CAS PubMed.
- T. Laurita, R. D'Orsi, L. Chiummiento, M. Funicello and P. Lupattelli, Recent advances in synthetic strategies to 2, 3-dihydrobenzofurans, Synthesis, 2020, 52(10), 1451–1477 CrossRef CAS.
- A. B. Dapkekar, C. Sreenivasulu, D. Ravi Kishore and G. Satyanarayana, Recent advances towards the synthesis of dihydrobenzofurans and dihydroisobenzofurans, Asian J. Org. Chem., 2022, 11(5), e202200012 CrossRef CAS.
- P. Gandeepan, T. Müller, D. Zell, G. Cera, S. Warratz and L. Ackermann, 3d transition metals for C–H activation, Chem. Rev., 2018, 119(4), 2192–2452 CrossRef PubMed.
- T. W. Lyons and M. S. Sanford, Palladium-catalyzed Ligand-Directed C–H Functionalization Reactions, Chem. Rev., 2010, 110(2), 1147–1169 CrossRef CAS PubMed.
- S. Cui, Y. Zhang and Q. Wu, Rh (iii)-Catalyzed
C–H Activation/Cycloaddition of Benzamides and Methylenecyclopropanes: Divergence in Ring Formation, Chem. Sci., 2013, 4(9), 3421–3426 RSC.
- H. Zhang, S. Lin, H. Gao, K. Zhang, Y. Wang, Z. Zhou and W. Yi, Chemodivergent Assembly of Ortho-Functionalized Phenols with Tunable Selectivity Via Rhodium (III)-Catalyzed and Solvent-Controlled CH activation, Commun. Chem., 2021, 4(1), 81 CrossRef CAS PubMed.
- X. Song, K. Wang, L. Xue, H. Yu, X. Zhang, R. Lee and X. Fan, Coupling Partner-Dependent Unsymmetrical C–H Functionalization of N-phenoxyacetamides Leading to Sophisticated Spirocyclic Scaffolds, Org. Chem. Front., 2022, 9(17), 4583–4590 RSC.
- L. Sun, Y. Zhao, B. Liu, J. Chang and X. Li, Rhodium III-Catalyzed Remote Difunctionalization of Arenes Assisted by a Relay Directing Group, Chem. Sci., 2022, 13(24), 7347–7354 RSC.
- Y. Wei, H. Xu, F. Chen, H. Gao, Y. Huang and W. Yi and Zhou, Specific Assembly of Dihydrobenzofuran Frameworks via Rh (iii)-Catalysed C–H Coupling of N-Phenoxyacetamides with 2-alkenylphenols, New J. Chem., 2022, 46(12), 5705–5711 RSC.
- W. Yu, C. Chen, L. Feng, T. Xia, C. Shi, Y. Yang and B. Zhou, Rhodium (III)-Catalyzed Asymmetric 1, 2-Carboamidation of Alkenes Enables Access to Chiral 2, 3-Dihydro-3-Benzofuranmethanamides, Org. Lett., 2022, 24(9), 1762–1767 CrossRef CAS PubMed.
- N. Sinha, P. Mistry, S. Das, T. Datta and B. Roy, Intramolecular Hydroarylation of Arenes via Imidazole-Directed C–H Activation in Aqueous Methanol Using Rhodium (III) as the Catalyst and Mechanistic Study, J. Org. Chem., 2023, 88, 8969–8983 CrossRef CAS PubMed.
- Y. Wang and D. M. Du, Recent Advances in Organocatalytic Asymmetric Oxa-Michael Addition Triggered Cascade Reactions, Org. Chem. Front., 2020, 7(20), 3266–3283 RSC.
- D. X. Zhu, J. G. Liu and M. H. Xu, Stereodivergent Synthesis of Enantioenriched 2, 3-Disubstituted Dihydrobenzofurans via a One-Pot C–H Functionalization/Oxa-Michael Addition Cascade, J. Am. Chem. Soc., 2021, 143(23), 8583–8589 CrossRef CAS PubMed.
- H. M. Davies and R. E. Beckwith, Catalytic Enantioselective C–H Activation by Means of Metal–Carbenoid-Induced C–H Insertion, Chem. Rev., 2003, 103(8), 2861–2904 CrossRef CAS PubMed.
- A. Padwa and S. F. Hornbuckle, Ylide Formation from the Reaction of Carbenes and Carbenoids with Heteroatom Lone Pairs, Chem. Rev., 1991, 91(3), 263–309 CrossRef CAS.
- S. E. Reisman, R. R. Nani and S. Levin, Buchner and beyond: Arene cyclopropanation as applied to natural product total synthesis, Synlett, 2011, 2011(17), 2437–2442 CrossRef.
- A. Tinoco, V. Steck, V. Tyagi and R. Fasan, Highly Diastereo-and Enantioselective Synthesis of Trifluoromethyl-Substituted Cyclopropanes via Myoglobin-Catalyzed Transfer of Trifluoromethylcarbene, J. Am. Chem. Soc., 2017, 139(15), 5293–5296 CrossRef CAS PubMed.
- X. Zhang, P. Sivaguru, G. Zanoni, X. Han, M. Tong and X. Bi, Catalytic Asymmetric C (sp3)–H Carbene Insertion Approach to Access Enantioenriched 3-fluoroalkyl 2, 3-Dihydrobenzofurans, ACS Catal., 2021, 11(22), 14293–14301 CrossRef CAS.
- A. M. Buckley, D. C. Crowley, T. A. Brouder, A. Ford, U. B. Rao Khandavilli, S. E. Lawrence and A. R. Maguire, Dirhodium Carboxylate Catalysts from 2-Fenchyloxy or 2-Menthyloxy Arylacetic Acids: Enantioselective C–H Insertion, Aromatic Addition and Oxonium Ylid Formation/Rearrangement, ChemCatChem, 2021, 13(20), 4318–4324 CrossRef CAS PubMed.
- K. Hong, S. Dong, X. Xu, Z. Zhang, T. Shi, H. Yuan, X. Xu and W. Hu, Enantioselective Intermolecular Mannich-type Interception of Phenolic Oxonium Ylide for the Direct Assembly of Chiral 2, 2-disubstituted Dihydrobenzofurans, ACS Catal., 2021, 11(12), 6750–6756 CrossRef CAS.
- K. Hong, X. Yang, Z. Zhang, X. Xie, X. Lv, X. Xu and W. Hu, Diastereoselective Aldol-Type Interception of Phenolic Oxonium Ylides for the Direct Assembly of 2, 2-disubstituted Dihydrobenzofurans, Org. Biomol. Chem., 2022, 20(22), 4635–4639 RSC.
- X. Zhong, S. Lin, H. Gao, F. X. Liu, Z. Zhou and W. Yi, Rh (III)-Catalyzed Redox-Neutral C–H Activation/[3+ 2] Annulation of N-phenoxy Amides with Propargylic Monofluoroalkynes, Org. Lett., 2021, 23(6), 2285–2291 CrossRef CAS PubMed.
- A. Singh, A. Dey and C. M. Volla, Rh (III)-Catalyzed Stereoselective C–C Bond Cleavage of ACPs with N-Phenoxyacetamides: The Critical Role of the Nucleophilic Directing Group, J. Org. Chem., 2021, 86(15), 10474–10483 CrossRef CAS PubMed.
- U. Nazeer, A. Mushtaq, A. F. Zahoor, F. Hafeez, I. Shahzadi and R. Akhtar, Cloke–Wilson Rearrangement: A Unique Gateway to Access Five-Membered Heterocycles, RSC Adv., 2023, 13(50), 35695–35732 RSC.
- D. J. Paymode and I. Sharma, Rhodium-Catalyzed [3+ 2]-Annulation of Ortho-Diazoquinones with Enol Ethers: Diversity-Oriented Total Synthesis of Aflatoxin B2, Eur. J. Org Chem., 2021, 2021(13), 2034–2040 CrossRef CAS.
- A. F. Zahoor, M. Yousaf, R. Siddique, S. Ahmad, S. A. R. Naqvi and S. M. A. Rizvi, Synthetic strategies toward the synthesis of enoxacin-, levofloxacin-, and gatifloxacin-based compounds: A review, Synth. Commun., 2017, 47(11), 1021–1039 CrossRef CAS.
- S. Ahmad, A. F. Zahoor, S. A. R. Naqvi and M. Akash, Recent trends in ring opening of epoxides with sulfur nucleophiles, Mol. Diversity, 2018, 22(1), 191–205 CrossRef CAS PubMed.
- J. Jiang, J. Liu, Z. Yang, L. Zheng and Z. Q. Liu, Three-Component Synthesis of Benzofuran-3 (2H)-ones with Tetrasubstituted Carbon Stereocenters via Rh (III)-Catalyzed C–H/C–C Bond Activation and Cascade Annulation, Adv. Synth. Catal., 2022, 364(15), 2540–2545 CrossRef CAS.
- J. Sun, H. Ye, H. Zhang and X. X. Wu, Palladium-Catalyzed Cyclization Coupling with Cyclobutanone-Derived N-Tosylhydrazones: Synthesis of Benzofuran-3-Cyclobutylidenes and Spirocyclobutanes, J. Org. Chem., 2023, 88(3), 1568–1577 CrossRef CAS PubMed.
- M. L. Han, W. Huang, Y. W. Liu, M. Liu, H. Xu, H. Xiong and H. X. Dai, Pd-Catalyzed Asymmetric Dearomatization of Indoles via Decarbonylative Heck-type Reaction of Thioesters, Org. Lett., 2020, 23(1), 172–177 CrossRef PubMed.
- Y. Madich, R. Álvarez and J. M. Aurrecoechea, Palladium Catalyzed Regioselective 5-Exo-O-Cyclization/Oxidative Heck Cascades from o-Alkynylbenzamides and Electron-Deficient Alkenes, Eur. J. Org Chem., 2014, 2014(28), 6263–6271 CrossRef CAS.
- Y. Wu, B. Xu, G. Zhao, Z. Pan, Z. M. Zhang and J. Zhang, Palladium/Xu-Phos Catalyzed Enantioselective Tandem Heck/Cacchi Reaction of Unactivated Alkenes, Chin. J. Chem., 2021, 39(12), 3255–3260 CrossRef CAS.
- C. Sreenivasulu and G. Satyanarayana, Time and Temperature Dependent Palladium-Catalyzed Stereo-and Regioselective Alkoxy-arylation of Triple Bonds: Synthesis of (E)/(Z)-1, 1-Disubstituted-3-(1-Phenylalkylidene)-1, 3-dihydroisobenzofurans, J. Org. Chem., 2021, 86(12), 8182–8196 CrossRef CAS PubMed.
- C. Fang, Q. Wang, B. Xu, Z. Zhang and J. Zhang, Palladium/XuPhos-Catalyzed Enantioselective Cascade Heck/Intermolecular C(sp2)–H Alkylation Reaction, Chem. Sci., 2024, 15, 5573–5580 RSC.
- Y. Wu, L. Wu, Z. M. Zhang, B. Xu, Y. Liu and J. Zhang, Enantioselective Difunctionalization of Alkenes by a Palladium-Catalyzed Heck/borylation Sequence, Chem. Sci., 2022, 13(7), 2021–2025 RSC.
- J. M. Guo, Z. Y. Mao, C. H. Liu, S. Y. Yang and B. G. Wei, Palladium-Catalyzed Sequential Heck Reactions of Olefin-Tethered Aryl Iodides with
Alkenes, J. Org. Chem., 2022, 87(17), 11838–11845 CrossRef CAS PubMed.
- K. Babar, A. F. Zahoor, S. Ahmad and R. Akhtar, Recent synthetic strategies toward the synthesis of spirocyclic compounds comprising six-membered carbocyclic/heterocyclic ring systems, Mol. Diversity, 2021, 25, 2487–2532 CrossRef CAS PubMed.
- A. D. Marchese, A. G. Durant and M. Lautens, A Modular Approach for the Palladium-Catalyzed Synthesis of Bis-Heterocyclic Spirocycles, Org. Lett., 2021, 24(1), 95–99 CrossRef PubMed.
- Y. Kang, J. L. Lu, Z. Zhang, Y. K. Liang, A. J. Ma and J. B. Peng, Palladium-Catalyzed Intramolecular Heck/Aminocarbonylation of Alkene-Tethered Iodobenzenes with Nitro Compounds: Synthesis of Carbamoyl-Substituted Benzoheterocycles, J. Org. Chem., 2022, 88(8), 5097–5107 CrossRef PubMed.
- Y. Tu, B. Xu, Q. Wang, H. Dong, Z. M. Zhang and J. Zhang, Palladium/TY-Phos-Catalyzed Asymmetric Heck/Tsuji–Trost Reaction of o-Bromophenols with 1, 3-Dienes, J. Am. Chem. Soc., 2023, 145(8), 4378–4383 CrossRef CAS PubMed.
- A. Tinoco, V. Steck, V. Tyagi and R. Fasan, Highly Diastereo-and Enantioselective Synthesis of Trifluoromethyl-Substituted Cyclopropanes via Myoglobin-Catalyzed Transfer of Trifluoromethylcarbene, J. Am. Chem. Soc., 2017, 139(15), 5293–5296 CrossRef CAS PubMed.
- M. Ding, P. Ou, X. Li, Y. Yu, M. Niu, Y. Yang, Y. Huang, W. Zhi-Xiang and X. Huang, Alkyne Insertion Enabled Vinyl to Acyl 1, 5-Palladium Migration: Rapid Access to Substituted 5-Membered-Dihydrobenzofurans and Indolines, Angew. Chem., Int. Ed., 2023, 62(18), e202300703 CrossRef CAS PubMed.
- R. V. Rozhkov and R. C. Larock, Synthesis of Dihydrobenzofurans via Palladium-Catalyzed Annulation of 1, 3-dienes by o-iodoaryl Acetates, J. Org. Chem., 2010, 75(12), 4131–4134 CrossRef CAS PubMed.
- F. Zhou, C. Li, M. Li, Y. Jin, H. Jiang, Y. Zhang and W. Wu, Synthesis of 2-isoxazolyl-2, 3-Dihydrobenzofurans via Palladium-Catalyzed Cascade Cyclization of Alkenyl Ethers, Chem. Commun., 2021, 57(39), 4799–4802 RSC.
- K. E. Houghtling, A. M. Canfield and S. M. Paradine, Convergent Synthesis of Dihydrobenzofurans via Urea Ligand-Enabled Heteroannulation of 2-Bromophenols with 1, 3-Dienes, Org. Lett., 2022, 24(31), 5787–5790 CrossRef CAS PubMed.
- H. Sun, H. He, S. F. Ni and W. Guo, Asymmetric (4+ 1) Annulations by Cascade Allylation and Transient σ-Alkyl-Pd (II) Initiated Allylic Csp3–H Activation, Angew. Chem., Int. Ed., 2023, 62(51), e202315438 CrossRef CAS PubMed.
- Z. Wu, Z. Wu, X. Sun, W. Qi, Z. Zhang and Y. Zhang, Palladium-Catalyzed Intramolecular Cross-Coupling of Unactivated C(sp3)–H and C(sp2)–H Bonds, Org. Lett., 2021, 23(18), 7161–7165 CrossRef CAS PubMed.
- P. M. Reddy, K. Ramachandran and P. Anbarasan, Palladium-Catalyzed Diastereoselective Synthesis of 2, 2, 3-trisubstituted Dihydrobenzofurans via Intramolecular Trapping of O-ylides with Activated Alkenes, J. Catal., 2021, 396, 291–296 CrossRef CAS.
- N. Bortolamei, A. A. Isse, V. B. Di Marco, A. Gennaro and K. Matyjaszewski, Thermodynamic Properties of Copper Complexes Used as Catalysts in Atom Transfer Radical Polymerization, Macromolecules, 2010, 43(22), 9257–9267 CrossRef CAS.
- M. L. Rao and S. S. Islam, Copper-Catalyzed Synthesis of 1-(2-benzofuryl)-N-heteroarenes from o-hydroxy-gem-(dibromovinyl) benzenes and N-heteroarenes, Org. Biomol. Chem., 2021, 19(41), 9076–9080 RSC.
- S. A. Morris, J. Wang and N. Zheng, The Prowess of Photogenerated Amine Radical Cations in Cascade Reactions: From Carbocycles to Heterocycles, Acc. Chem. Res., 2016, 49(9), 1957–1968 CrossRef CAS PubMed.
- T. Y. Zheng, Y. Q. Zhou, N. Yu, Y. L. Li, T. Wei, L. Peng, Y. Ling, K. Jiang and Y. Wei, Deconstructive Insertion of Oximes into Coumarins: Modular Synthesis of Dihydrobenzofuran-Fused Pyridones, Org. Lett., 2022, 24(12), 2282–2287 CrossRef CAS PubMed.
- M. Ríos-Gutiérrez and L. R. Domingo, Unravelling the mysteries of the [3+ 2] cycloaddition reactions, Eur. J. Org Chem., 2019, 2019(2–3), 267–282 CrossRef.
- Z. R. Jing, D. D. Liang, J. M. Tian, F. M. Zhang and Y. Q. Tu, Enantioselective Construction of 2-Aryl-2, 3-Dihydrobenzofuran Scaffolds Using Cu/SPDO-Catalyzed [3+ 2] Cycloaddition, Org. Lett., 2021, 23(4), 1258–1262 CrossRef CAS PubMed.
- J. X. Zhu, J. M. Tian, Y. Y. Chen, X. J. Hu, X. Han, W. Chen, Z. Yang, X. Bao, X. Ye, H. Chen, F. M. Zhang, H. Wang and Y. Q. Tu, Enantioselective Synthesis of 2, 3, 3a, 8a-Tetrahydrofuro [2, 3-b] benzofuran Scaffolds Enabled by Cu (II)/SPDO-Catalyzed [3+ 2] Cycloaddition of 2, 3-Dihydrofuran and Quinone Esters, J. Org. Chem., 2023, 88(20), 14670–14675 CrossRef CAS PubMed.
- G. Sui, T. Li, B. Zhang, R. Wang, H. Hao and W. Zhou, Recent advances on synthesis and biological activities of aurones, Bioorg. Med. Chem., 2021, 29, 115895 CrossRef CAS PubMed.
- A. P. Devi, J. Pandey, U. Bhardwaj, N. Dhingra, R. Kant and K. L. Ameta, Green Synthesis and in silico, Neuraminidase Study of Some Substituted 2-Benzylidene-1-Benzofuran-3-Ones, SSRN, 2021, DOI:10.2139/ssrn.3911206.
- K. Mitsudo, Y. Kobashi, K. Nakata, Y. Kurimoto, E. Sato, H. Mandai and S. Suga, Cu-Catalyzed Dehydrogenative C–O Cyclization for the Synthesis of Furan-Fused Thienoacenes, Org. Lett., 2021, 23(11), 4322–4326 CrossRef CAS PubMed.
- C. Liu, D. Liu and A. Lei, Recent advances of transition-metal catalyzed radical oxidative cross-couplings, Acc. Chem. Res., 2014, 47(12), 3459–3470 CrossRef CAS PubMed.
- K. Dong, C. Y. Zhao, X. J. Wang, L. Z. Wu and Q. Liu, Bioinspired Selective Synthesis of Heterodimer 8–5′ or 8–O–4′ Neolignan Analogs, Org. Lett., 2021, 23(7), 2816–2820 CrossRef CAS PubMed.
- V. P. Ananikov, Nickel: The “Spirited Horse” of Transition Metal Catalysis, ACS Catal., 2015, 5(3), 1964–1971 CrossRef CAS.
- F. Yang, Y. Jin and C. Wang, Nickel-catalyzed asymmetric intramolecular reductive Heck reaction of unactivated alkenes, Org. Lett., 2019, 21(17), 6989–6994 CrossRef CAS PubMed.
- A. Cerveri, R. Giovanelli, D. Sella, R. Pedrazzani, M. Monari, O. N. Faza, O. C. S. Lopez and M. Bandini, Enantioselective CO2 Fixation via a Heck-Coupling/Carboxylation Cascade Catalyzed by Nickel, Chem.–Eur. J., 2021, 27(28), 7657–7662 CrossRef CAS PubMed.
- M. Bhardwaj, B. Rasool and D. Mukherjee, Ni-Catalyzed Domino Transformation of Enopyranoses and 2-iodo phenols/anilines to Pyrano Cis Fused Dihydro-benzofurans/indoles, Chem. Commun., 2022, 58(50), 7038–7041 RSC.
- S. Xu, K. Wang and W. Kong, Ni-Catalyzed Reductive Arylacylation of Alkenes Toward Carbonyl-Containing Oxindoles, Org. Lett., 2019, 21(18), 7498–7503 CrossRef CAS PubMed.
- Y. Jin, P. Fan and C. Wang, Nickel-Catalyzed Reductive Asymmetric Aryl-Acylation and Aryl-Carbamoylation of Unactivated Alkenes, CCS Chem., 2022, 4(5), 1510–1518 CrossRef CAS.
- Z. Lin, Y. Jin, W. Hu and C. Wang, Nickel-Catalyzed Asymmetric Reductive Aryl-Allylation of Unactivated Alkenes, Chem. Sci., 2021, 12(19), 6712–6718 RSC.
- H. Q. Geng, W. Wang and X. F. Wu, Nickel-Catalyzed Carbonylative Synthesis of Dihydrobenzofurans, Catal. Commun., 2021, 148, 106170 CrossRef CAS.
- M. He, Y. Sun and B. Han, Green Carbon Science: Efficient Carbon Resource Processing, Utilization, and Recycling Towards Carbon Neutrality, Angew. Chem., 2022, 134(15), e202112835 CrossRef.
- C. Déjardin, A. Renou, J. Maddaluno and M. Durandetti, Nickel-Catalyzed Electrochemical Cyclization of Alkynyl Aryl Iodide and the Domino Reaction with Aldehydes, J. Org. Chem., 2021, 86(13), 8882–8890 CrossRef PubMed.
- X. S. Li, D. T. Xu, Z. J. Niu, M. Li, W. Y. Shi, C. T. Wang, W. X. Wei and Y. M. Liang, Gold-Catalyzed Tandem Annulations of Pyridylhomopropargylic Alcohols with Propargyl Alcohols, Org. Lett., 2021, 23(3), 832–836 CrossRef CAS PubMed.
- N. Morita, H. Chiaki, K. Tanaka III, Y. Hashimoto, O. Tamura and N. Krause, Sustainable Chemical Synthesis of 2, 3-Dihydrobenzofurans/1, 2, 3-Trisubstituted Indanes in Water using a Permethylated β-Cyclodextrin-Tagged N-Heterocyclic Carbene–Gold Catalyst, Synlett, 2023, 34(12), 1425–1432 CrossRef CAS.
- D. Prochowicz, A. Kornowicz and J. Lewiński, Interactions of Native Cyclodextrins with Metal Ions and Inorganic Nanoparticles: Fertile Landscape for Chemistry and Materials science, Chem. Rev., 2017, 117(22), 13461–13501 CrossRef CAS PubMed.
- Y. J. Wang, Y. Zhang, Z. Qiang, J. Y. Liang and Z. Chen, Gold Catalyzed Efficient Preparation of Dihydrobenzofuran from 1, 3-enyne and Phenol, Chem. Commun., 2021, 57(94), 12607–12610 RSC.
- W. Du, R. Yang, J. Wu and Z. Xia, Flexible Synthesis of Benzofuranones from ortho-Alkynyl Phenols or Benzofurans, Eur. J. Org Chem., 2023, 26(8), e202201497 CrossRef CAS.
- S. Sau, K. Mukherjee, K. Kondalarao, V. Gandon and A. K. Sahoo, Probing Chiral Sulfoximine Auxiliaries in Ru (II)-Catalyzed One-Pot Asymmetric C–H Hydroarylation and Annulations with Alkynes, Org. Lett., 2023, 25(42), 7667–7672 CrossRef CAS PubMed.
- N. Pannilawithana, B. Pudasaini, M. H. Baik and C. S. Yi, Experimental and Computational Studies on the Ruthenium-Catalyzed Dehydrative C–H Coupling of Phenols with Aldehydes for the Synthesis of 2-Alkylphenol, Benzofuran, and Xanthene Derivatives, J. Am. Chem. Soc., 2021, 143(33), 13428–13440 CrossRef CAS PubMed.
- J. Phukon, P. Bhorali, S. Changmai and S. Gogoi, Hydroxyl-Directed Ru (II)-Catalyzed Synthesis of Fused Dihydrofurans Using 1, 4-Dioxane and Sulfoxonium Ylides as Annulating Agents, Org. Lett., 2023, 25(1), 215–219 CrossRef CAS PubMed.
- F. Yuan, D. M. Yan, P. P. Gao, D. Q. Shi, W. J. Xiao and J. R. Chen, Photoredox-Catalyzed Multicomponent Cyclization of 2-Vinyl Phenols, N-Alkoxypyridinium Salts, and Sulfur Ylides for Synthesis of Dihydrobenzofurans, ChemCatChem, 2021, 13(2), 543–547 CrossRef CAS.
- Y. Liao, F. Liu and Z. J. Shi, Recent Progress in the Oxidative Coupling of Unactivated Csp3–H Bonds with Other C–H Bonds, Chem. Commun., 2021, 57(98), 13288–13296 RSC.
- S. Kusaka, T. Ohmura and M. Suginome, Iridium-Catalyzed Enantioselective Intramolecular Cross-Dehydrogenative Coupling of Alkyl Aryl Ethers Giving Enantioenriched 2, 3-Dihydrobenzofurans, Chem. Lett., 2022, 51(6), 601–604 CrossRef CAS.
- T. Shibata, S. Takayasu, S. Yuzawa and T. Otani, Rh (III)-Catalyzed C–H Bond Activation along with “Rollover” for the Synthesis of 4-Azafluorenes, Org. Lett., 2012, 14(19), 5106–5109 CrossRef CAS PubMed.
- K. Sakamoto and T. Nishimura, Enantioselective Synthesis of 3-substituted Dihydrobenzofurans Through Iridium-Catalyzed Intramolecular Hydroarylation, Org. Biomol. Chem., 2021, 19(3), 684–690 RSC.
- T. Ohmura, S. Kusaka and M. Suginome, Iridium-Catalyzed Enantioselective Intramolecular Hydroarylation of Allylic Aryl Ethers Devoid of a Directing Group on the Aryl Group, Chem. Commun., 2021, 57(99), 13542–13545 RSC.
- K. C. Majumdar, N. De, T. Ghosh and B. Roy, Iron-catalyzed synthesis of heterocycles, Tetrahedron, 2014, 70(33), 4827–4868 CrossRef CAS.
- M. A. Bashir, X. Chen, T. Wang, H. Guo and H. Zhai, Synthesis of Cyclopenta[b]Benzofurans via Biomimetic Oxidative Phenol–enamine [3 + 2] Cycloaddition, Org. Chem. Front., 2023, 10(5), 1213–1218 RSC.
- H. Zhang, B. Wang, H. Xu, F. Y. Li and J. Y. Wang, Synthesis of Naphthodihydrofurans via an Iron (iii)-Catalyzed Reduction Radical Cascade Reaction, Org. Chem. Front., 2021, 8(21), 6019–6025 RSC.
- L. Guo, G. Chen, H. Li, C. H. Tung and Y. Wang, Visible-Light-Driven [3+ 2] Cyclization of Phenols with Indoles and Olefins Using Recyclable Ag3PO4 Nanoparticles, Green Chem., 2023, 25(18), 7102–7108 RSC.
- H. J. Dias, M. L. Rodrigues and A. E. Crotti, Optimization of the Reaction Conditions for the Synthesis of Dihydrobenzofuran Neolignans, J. Braz. Chem. Soc., 2021, 32, 20–28 CAS.
- J. Huo, Z. Wang, C. Oberschelp, G. Guillén-Gosálbez and S. Hellweg, Net-zero transition of the global chemical industry with CO2-feedstock by 2050: feasible yet challenging, Green Chem., 2023, 25(1), 415–430 RSC.
- K. Okamoto, N. Shida and M. Atobe, Electrochemical [3+ 2] Cycloaddition Proceeding at Low Electrolyte Concentration in Laminar-Flow Microreactor, ChemElectroChem, 2023, e202300386, DOI:10.1002/celc.202300386.
- Y. H. Yang, Y. F. Tan, Y. N. Zhao, Y. H. He and Z. Guan, Electrosynthesis of 5-Aminocoumaran Derivatives from Olefins and Aminophenols, Org. Lett., 2023, 25(46), 8205–8209 CrossRef CAS PubMed.
- H. Wang, Y. Zhou, Y. Xie, Y. Liu, Y. Li, H. Zhang and J. Long, Synthesis of Functionalized 3-Aryl-3H-benzofuranone Derivatives from Aryl Acetate via [3+ 2] Annulation of 1, 4-Dihydroxy-2-naphthoic Acid Ester, Org. Biomol. Chem., 2023, 21(8), 1821–1826 RSC.
- P. S. Gowda, D. S. Sharada and G. Satyanarayana, A TBADT Photocatalyst-Enabled Radical-Induced Cyclization Pathway to Access Functionalized Dihydrobenzofurans, Chem. Commun., 2023, 59(59), 9094–9097 RSC.
- W. F. Tian, Y. Zhu, Y. Q. He, M. Wang, X. R. Song, J. Bai and Q. Xiao, Hydroxyl Assisted, Photoredox/Cobalt Co-catalyzed Semi-Hydrogenation and Tandem Cyclization of o-Alkynylphenols for Access to 2, 3-Dihydrobenzofurans, Adv. Synth. Catal., 2021, 363(3), 730–736 CrossRef CAS.
- W. Huang, H. Wang, B. Liu, R. Shen and S. Zhu, Synthesis of 1, 1, 4, 5-Tetrasubstituted Phthalans via Pd-Catalyzed Three-Component Reactions of Haloarenes, Alkynes, and Protic Nucleophiles, Org. Lett., 2022, 24(47), 8651–8656 CrossRef CAS PubMed.
- H. Sun, B. Xiong, Y. Yang, J. Liu, X. Zhang and Z. Lian, Gem-Difluorovinylation of Alkynyl Bromoarenes via Dual Nickel-/Palladium-Catalyzed Cross-Electrophile Coupling, Org. Chem. Front., 2022, 9(2), 305–310 RSC.
- K. E. Poremba, S. E. Dibrell and S. E. Reisman, Nickel-Catalyzed Enantioselective Reductive Cross-Coupling Reactions, ACS Catal., 2020, 10(15), 8237–8246 CrossRef CAS PubMed.
- J. Wu, Y. Liu and M. C. Kozlowski, Visible-Light TiO2-Catalyzed Synthesis of Dihydrobenzofurans by Oxidative [3+ 2] Annulation of Phenols with Alkenyl Phenols, Chem. Sci., 2024 10.1039/D4SC00723A.
- C. Zhao, Z. Ge, J. Hu, H. Tian and X. Wang, Enantioselective Reductive Aryl-Benzylation of Alkenes by a Nickel-Titanium Bimetallic System, Cell Rep., 2023, 4(7) DOI:10.1016/j.xcrp.2023.101474.
|
This journal is © The Royal Society of Chemistry 2024 |
Click here to see how this site uses Cookies. View our privacy policy here.