DOI:
10.1039/D4RA01745E
(Paper)
RSC Adv., 2024,
14, 10726-10735
Luminescence properties of a green-emitting mechanoluminescent phosphor CaSrGa4O8:xTb3+ without pre-excitation†
Received
6th March 2024
, Accepted 21st March 2024
First published on 2nd April 2024
Abstract
In this study, both mechanoluminescence (ML) and long persistent luminescence (LPL) characteristics were first observed in CaSrGa4O8 doped with Tb3+ ions, which confirmed that CaSrGa4O8 is a high-quality host for luminescent material research. Notably, the samples show stronger mechanoluminescent intensity with increasing Tb3+ doping. Additionally, the introduction of Tb3+ led to a shift of the thermoluminescence peak towards higher temperatures and a substantial increase in its intensity, suggesting that Tb3+ doping enhances the overall trap concentration and introduces deeper trap energy levels. Presumably, the free carriers in the system recombine upon mechanical stimulation, releasing energy that is transferred to Tb3+ ions. Investigations into the intrinsic structure, matrix effects, and trap evolution of the material confirmed that deep and shallow traps are responsible for the observed ML and LPL phenomena, respectively. The elucidation of the unique luminescent properties of the material provides us with some guidance for the development of new multi-functional luminescent materials.
1. Introduction
Mechanoluminescent (ML) materials, which emit light under the mechanical stimulation of external forces, emerge as a significant innovation in this context. Characterized by their linearity, sensitivity, repeatability, and ductility, ML materials efficiently harness mechanical energy, which is abundant in daily life.1 Mechanoluminescent (ML) materials are stimulation-responsive materials, which can produce different detectable signals in rapid response to various external stimuli (including stretching, compression, bending, etc.).2–5 In recent years, ML materials have shown broad application prospects in the fields of illumination display,6 information anti-counterfeiting,7 visual mechanical sensing,8 structural defects monitoring,9–11 and genetic detection,12 and its potential application fields include damage detection,13 self-diagnosis,14 defect recording,15 earthquake prediction,16 etc., attracting widespread research interest. After securing patents for layered mechanoluminescent materials in doped CaZnOS systems, our continuous research has been carried out to broaden the luminescence range and application potentials of mechanoluminescent materials.17–19 A novel application involves coating structures with a composite of ML materials and elastic polymers. This technique enables real-time visual observation of surface stress distribution across scales, from nanometers to kilometers. It is particularly beneficial for urban building safety, allowing for visualizing invisible defects and cracks in macro-infrastructure such as high-pressure vessels, pipelines, bridges, and buildings. The emission spectrum of ML phosphors ranges from ultraviolet to near-infrared.20,21
Recent advancements spotlight that alkali earth metal gallate compounds, known for their abundant structures, low phonon energy, high flexibility of doping sites in the structures and appropriate bandwidth for adjusting the defect levels with abundant traps, may constitute a large group of host compounds for exploration of ML materials. Their low synthesis temperature and relatively high stability will also be an advantage.22 Their cost-effectiveness and eco-friendliness have become a central focus of contemporary research efforts.23–28 Rare earth ions have strong light absorption capacity and high photoelectric conversion efficiency.29 Given that green is one of the colors most easily captured by the human eye, developing green-emitting ML materials has become a priority.30,31 Among a variety of rare earth ions or transition metal ions, Tb3+ belongs to the typical 5D4–7FJ (J = 0, 1, 2, 3, 4, 5, 6) transition, with their rich emission levels and effective activation in green luminescent materials, are particularly promising.31 In addition, Tb3+ also has a cross-relaxation transition, which provides theoretical support for luminescence with colorful evolution.32 In consideration of the above discussions, CaSrGa4O8 (CSGO) has been used as a mechanoluminescent host in our research, and it has already shown good perspectives in other doping systems.33–35
In this work, a series of green emission phosphors Ca1−xSrGa4O8:xTb3+ (CSGOT) have been successfully synthesized. With the concentration of Tb3+ doped, these phosphors exhibit a rich energy level spectrum in the range of 450–650 nm, indicating diverse energy level transitions. This new class of mechanoluminescent materials, which requires no pre-excitation, shows great potential for application.
2. Experimental section
2.1. Materials and synthesis
Polycrystalline powders with the formula of Ca1−xSrGa4O8:xTb3+ (x = 0, 0.03, 0.05, 0.07, 0.09 and 0.11) were synthesized by convention a high-temperature solid-state reaction. The starting materials Ca2CO3 (Aladdin, 99.99%), Sr2O3 (Aladdin, 99.95%), Ga2O3 (Aladdin, 99.99%), Tb4O7 (Aladdin, 99.99%) were weighted out according to the stoichiometric ratio. These powders were then mixed and ground in an agate mortar using anhydrous ethanol for about 30 min. After mixing, the powders were placed in a crucible and dried in an oven for 15 min to evaporate any remaining anhydrous ethanol. The dried mixtures were then transferred to a corundum crucible and sintered at 1280 °C for 10 hours, with a heating rate of 5 °C min−1 under air. Once the furnace cooled naturally to room temperature, the products were removed and ground into fine powders for subsequent measurements.
Then, 1.5 g of Polydimethylsiloxane (PDMS) precursor, 0.15 g of curing agent, and 1.0 g of synthesized phosphor were weighed and combined to create the composite elastomer. The mixture was stirred mechanically for 10 min. Subsequently, it was cured in an oven at 80 °C for 2 hours, resulting in the Ca1−xSrGa4O8:xTb3+/PDMS composite elastomer, ready for ML examining.
2.2. Characterization
The phase purity of each sample was checked by X-ray diffraction patterns (XRD) collected on a powder X-ray diffractometer (Cu Kα, λ = 1.54178 Å) with a scanning step size of 0.02626°, range of 10–90°. The morphology and elemental distribution of the powder samples were analyzed by scanning electron microscopy (SEM, FEG-450) equipped with energy dispersive X-ray spectroscopy (EDS). The diffuse reflectance (DR) spectra were measured by using a UV-VIS-NIR spectrophotometer (U-3900H Hitachi) and calibrated with BaSO4 as the standard reference. Photoluminescence excitation (PLE), photoluminescence (PL) and the time-gated photoluminescence decay curve were recorded via a fluorescence spectrometer (FLS1000 Edinburgh) equipped with a 450 W Xe lamp as the steady-state excitation source. The weight of the measured samples was constant (200 mg). The long afterglow decay curves and thermoluminescence (TL) curves were recorded using a fluorescence spectrometer and thermo-luminescence meter (TOSL-3DS). Before the TL measure, the samples were first exposed to the radiation from the UV light for 5 min and then heated from room temperature to 200 °C at a rate of 2 °C s−1. X-ray photoelectron spectroscopy (XPS) (ESCALAB 250xi, ThermoFisher) was used to analyze the chemical bonding states. The mechanoluminescence (ML) spectra were recorded by a motion controller, a photocounting system (QE-Pro Ocean Optics) and a computer.
3. Results and discussion
3.1. Phase, structure and morphology
The XRD patterns of Ca1−xSrGa4O8:xTb3+ (x = 0, 0.03, 0.05, 0.07, 0.09 and 0.11) samples are shown in Fig. 1(a). According to the Hume-Rothery rules, a solid solution is viable if the atomic radius difference between the solute and solvent ions is within 30%. The ionic radius difference is calculated using the formula (1):36,37 |
 | (1) |
where Dr is the percentage difference of radius, Rm (CN) is the radius of the main ion to be replaced, and Rd (CN) is the radius of the doped ion. Considering the valence and ionic radius of Tb3+ ions (for CN = 6, r = 0.92 Å) and Ca2+ ions (for CN = 6, r = 1.00 Å), and the larger difference with Sr2+ ions (for CN = 6, r = 1.18 Å), Tb3+ ions are more likely to replace the Ca2+ ions in the lattice. The calculated values of Dr (Ca2+–Tb3+) = 8%, and Dr (Sr2+–Tb3+) = 22.03%, respectively, indicating a preferential replacement of Ca2+ by Tb3+ in the Ca1−xSrGa4O8:xTb3+ structure. Without the sufficient crystal size for single crystal X-ray diffraction, experimental data was consistent with the structural data obtained by Wang's group confirming that the doping with Tb3+ ions did not introduce any impurity phase in Fig. 1(a). By indexing the XRD data for Ca0.93SrGa4O8:0.07Tb3+, it is obtained that a = 15.841(6) Å, b = 8.311(4) Å, c = 9.028(5) Å, β = 90.60(3)°, V = 1188.6(6) Å3. Refined cell lattice parameters (a/b/c/β/V) with different concentrations of Tb3+ ions doping shown in Fig. S1(a–e).† The experimental and computational XRD patterns of crystal structure model of Ca0.93SrGa4O8:0.07Tb3+ shown in Fig. 1(b), indicating the purities of the phases and the correctness of the crystal structure model proposed in monoclinic system with space group P21.34
 |
| Fig. 1 (a) XRD patterns of the Ca1−xSrGa4O8:xTb3+. (b) Comparison of experimental XRD pattern of Ca0.93SrGa4O8:0.07Tb3+ sintered at 1280 °C with the calculated pattern. (c) SEM micrograph of Ca0.93SrGa4O8:0.07Tb3+ (scale bar: 30 μm; magnification: 1.2k×). (d) EDS energy spectrum of Ca0.93SrGa4O8:0.07Tb3+. (e) SEM micrograph of Ca0.93SrGa4O8:0.07Tb3+ (scale bar: 2 μm; magnification: 20k×), the SEM mapping images of elements O, Sr, Ca, Ga and Tb, respectively. (f) Diffuse reflectance spectra of CaSrGa4O8. (g) Diffuse reflectance spectra of Ca0.93SrGa4O8:0.07Tb3+. | |
The surface morphology, grain size and crystallinity of phosphors play an important role in the luminescence properties of phosphors. The microscopic morphology of Ca1−xSrGa4O8:xTb3+ was characterized by SEM at 1.2k× and 20k× magnification in Fig. 1(c and e). The phosphor particles shown in Fig. 1(e) have irregular polyhedral morphology and range in size from several microns. The SEM image of captured Ca0.93SrGa4O8:0.7Tb3+ shows no agglomeration and no impurities. This is suitable for producing excellent ML phenomena. The EDS Spectroscopy conducted on the selected area in Fig. 1(d and e) demonstrated the uniform distribution of Ca, Sr, Ga, O, and Tb within the analyzed region. This observation provides convincing evidence that the desired sample was successfully synthesized, confirming that the dopant ions were effectively incorporated into the CSGO crystal structure. The uniform distribution of elements further confirms the formation of well-integrated and chemically homogeneous materials, thereby enhancing the reliability and quality of the synthesis process.
Fig. 1(f) displays the diffuse reflectance spectra of CaSrGa4O8 across a wavelength range from 200 nm to 800 nm. When compared to the diffuse reflectance spectra of Ca0.93SrGa4O8:0.07Tb3+ shown in Fig. 1(g), it can be deduced that the absorption band for Ca0.93SrGa4O8:0.07Tb3+ in the 240–260 nm range is due to host absorption, while the absorption band in the 290–350 nm range is attributed to the transition absorption of Tb3+ by Fig. S2.†
This diagram is used to calculate the band gap (Eg) of both CaSrGa4O8 and Ca0.93SrGa4O8:0.07Tb3+. The Eg can be calculated based on the following eqn (2):35
In this equation,
hν represents the energy of the incident photon,
α is the Kubelka–Munk function corresponding to absorbance, and
A is a constant. The Kubelka–Munk function can be determined by the following formula
(3):
35 |
 | (3) |
R denotes the reflectance. Based on these calculations, the optical bandgap of the host material CaSrGa
4O
8 is estimated to be 4.80 eV, while that of the doped Ca
0.93SrGa
4O
8:0.07Tb
3+ is reduced to 4.45 eV.
The narrowing of the optical bandgap in the doped material is significant. It suggests that electrons are more easily excited from the valence band to the conduction band under ultraviolet (UV) excitation, potentially leading to enhanced photoluminescence (PL) emission.36 Additionally, the impurity energy levels introduced by the doped Tb3+ ions may attributed to enhanced energy transfer between the [GaO4] group and Tb3+ ions, leading to more excited electrons returning to the ground state to this bandgap narrowing. This alteration in the bandgap could significantly impact the ML properties and the intensity of ML emissions. These changes underscore the influence of doping on the electronic structure and optical properties of these materials, with potential implications for their practical applications in fields.
3.2. PL/LPL properties of Ca1−xSrGa4O8:xTb3+
In Ca1−xSrGa4O8:xTb3+, the excitation and emission spectra of Tb3+ ions are depicted in Fig. 2(b), while the undoped sample is presented in Fig. 2(a). Excitation spectra of Ca1−xSrGa4O8:xTb3+ (x = 0.03, 0.05, 0.07, 0.09 and 0.11) monitored at 544 nm as shown in Fig. S2.† When excited at 254 nm in Fig. 2(b), the phosphors display a series of sharp emission lines that are characteristic of the internal 4f transitions of Tb3+ ions, specifically from the 5D3 and 5D4 levels to the 7FJ levels (where J = 3, 4, 5, and 6). The broad excitation spectra, observed by monitoring the emission peaks of the 5D4–7F5 transition at 544 nm, show an asymmetric shape with an acromion and a maximum at approximately 280 nm and 254 nm, respectively. The energy level of the acromion at 280 nm aligns well with the energy absorbed by the main lattice, suggesting an effective energy transfer from the [GaO4] group to the Tb3+ ion.38 The broad peak at 254 nm is primarily due to the combined effects of bulk absorption of Tb3+ and the 4f–5d inter-configuration transition of Tb3+, which is spin-allowed. The excitation lines within the 300–400 nm range represent optical transitions within the 4f electron configuration of the Tb3+ ion, and their weak intensity is likely due to the correspondingly low vibrational intensity. Notably, the 254 nm excitation peak is significantly stronger than the right acromion. This observation underscores the strong absorption and efficient energy utilization at this wavelength, which is critical for the photoluminescent properties of these materials. This characteristic makes the Ca1−xSrGa4O8:xTb3+ phosphors particularly suitable for applications requiring efficient excitation and bright luminescence, such as in lighting and display technologies.
 |
| Fig. 2 (a) PLE and PL spectra of the CaSrGa4O8 at room temperature. (b) PLE and PL spectra of the Ca0.93SrGa4O8:0.07Tb3+ at room temperature. (c) The PL spectra of Ca1−xSrGa4O8:xTb3+. (d) Dependence of emission peak (544 nm) intensities versus Tb3+ ion content. (e) PL decay curves under 254 nm excitation of the Ca1−xSrGa4O8:xTb3+. (f) PL decay curves of Ca1−xSrGa4O8:xTb3+ (λex = 254 nm, λem = 544 nm). | |
The emission spectra of Ca1−xSrGa4O8:xTb3+ phosphors, as shown in Fig. 2(c), reveal that the luminescence intensity is significantly influenced by the concentration of Tb3+ doping. Concentration quenching of Tb3+ is observed, indicating that there is an optimal doping concentration for achieving maximum luminescence intensity. This optimal concentration is found to be 0.07 for Tb3+. To differentiate the forbidden transitions from the 5D4 to 7FJ (J = 3, 4, 5, and 6) levels in Tb3+ ions, the emission decay curve at 544 nm under 254 nm excitation of CSGOT was measured, with the results presented in Fig. 2(d). These decay curves fit well with second-order exponential functions, described by equation:
|
 | (4) |
where
I is the emission intensity,
A1 and
A2 are the fitting constants, and
τ1 and
τ2 are the fast and slow decay lifetimes, respectively. The mean luminous lifetime can be evaluated using the following formula.
|
 | (5) |
The PL attenuation measurements indicate that CSGOT follows a double exponential decay behavior. The average lifetime of CSGOT, as shown in Fig. 2(e), gradually decreases with varying doping ion concentrations, suggesting complex radiative and non-radiative decay dynamics of the two excited states. Despite these changes, the average lifetime remains at the millisecond level. As the Tb3+ ion concentration increases, the τ* value decreases from 11.83 ms to 5.27 ms in Fig. 2(f), which is attributed to enhanced energy transfer between the [GaO4] group and Tb3+ ions, leading to more excited electrons returning to the ground state and it is also a simple concentration quenching effect.30
The LPL spectra exhibit similar trends and peak shapes, confirming their origin from the same luminescent Tb3+ center in Fig. 3(a). The LPL emission spectra of the samples, depicted in Fig. 3(b), display excellent persistent afterglow performance. The behavior suggests the presence of multiple traps in the Ca1−xSrGa4O8:xTb3+ phosphors. The LPL intensity decreases with increasing Tb3+ ion doping constantly above x = 0.07, due to the nature and concentration of defects.
 |
| Fig. 3 (a) LPL spectra of the Ca1−xSrGa4O8:xTb3+ and the insets show the LPL emission intensity of the Ca1−xSrGa4O8:xTb3+ (λex = 254 nm, λem = 544 nm). (b) LPL lifetime curves of CaSrGa4O8 and Ca0.93SrGa4O8:0.07Tb3+. (c) Photographs of the CaSrGa4O8 and Ca0.93SrGa4O8:0.07Tb3+ under daylight, 254 nm UV light excitation and after the removal of the UV light excitation, 365 nm UV light excitation and after the removal of the UV light excitation. | |
Under ultraviolet excitation, the undoped phosphor emits blue light, while the doped Tb3+ samples exhibit bright green light emission. Notably, an excellent green afterglow is observed after the cessation of UV excitation, highlighting the material's potential for applications requiring persistent luminescence in Fig. 3(b). Under ultraviolet excitation, the Ca1−xSrGa4O8:xTb3+ phosphors absorb energy, leading to the excitation of free electrons from the valence band to the conduction band. Once in the conduction band, these electrons move freely before being captured by traps within the crystal lattice. The electrons trapped in shallow traps begin to be released back into the conduction band upon cessation of the ultraviolet excitation. This release is facilitated by thermal perturbations. These freed electrons then undergo non-radiative recombination with holes (the absence of electrons in the valence band). During this recombination process, the energy is transferred to the Tb3+ ions. The transfer of energy to Tb3+ ions result in the strong afterglow phenomenon, as depicted in Fig. 3(c). This afterglow is a result of the phosphorescence process, where the delayed recombination of electrons and holes leads to prolonged emission of light after the initial excitation source (in this case, UV light) has been removed.
3.3. ML properties of Ca1−xSrGa4O8:xTb3+
PDMS is commonly utilized as a base material in the fabrication of ML films. Its popularity in this application stems from two key attributes: high transparency and an effective stress transfer capability. These properties make PDMS an ideal medium for observing and studying ML phenomena.39,40 The ML intensity data and images are shown in Fig. 4 and 5, respectively.
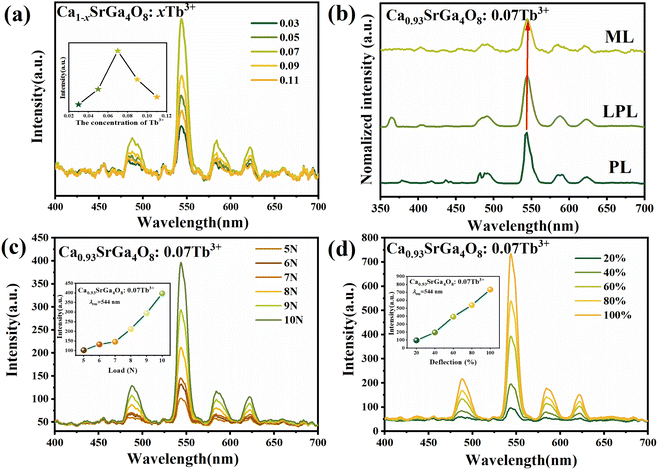 |
| Fig. 4 (a) ML spectra of the Ca1−xSrGa4O8:xTb3+ (the ML emission peak of the Ca1−xSrGa4O8:xTb3+, λem = 546 nm, F = 6 N, acrylic pen as a direct-acting medium, an approximate friction rate of 3 mm per second). (b) ML, LPL, and PL spectra of the Ca0.93SrGa4O8:0.07Tb3+. (c) ML spectra of the Ca0.93SrGa4O8:0.07Tb3+ with different load. (d) ML spectra of the Ca0.93SrGa4O8:0.07Tb3+ different deflection. | |
To accurately assess the ML properties of the phosphor and differentiate them from LPL behavior, the phosphors and PDMS were combined to make an elastic film for the ML test in Fig. 5(a), and the ML spectra of the phosphor were measured without any prior UV pre-irradiation. This approach ensures that the observed ML effects are purely due to mechanical stimulation rather than residual effects of earlier light exposure. The ML spectra exhibited a series of green emission peaks corresponding to the electron transitions of 5D4 to 7FJ (J = 3, 4, 5, and 6) in Tb3+ ions. The experimental results revealed that the ML intensity varied with the concentration of Tb3+ ions, first increasing and then decreasing, peaking at a concentration of x = 0.07 in Fig. 4(a). This trend aligns with the observed patterns in both the LPL and PL spectra, as shown in Fig. 4(b). In Fig. 4(c) and (d), a notable correlation between ML intensity and applied force is observed in the studied film. Specifically, the ML intensity exhibits a progressive increase in response to escalating applied forces. This trend continues until the film reaches a critical force threshold, beyond which it fails. Notably, within a certain force range, the luminous intensity demonstrates a linear relationship with the applied force, indicating a direct and proportional increase in light emission with escalating mechanical stress. This trend is consistently replicated in both figures, underscoring the reproducibility of mechanoluminescent response under varying mechanical stimuli.
 |
| Fig. 5 (a) Preparation process of mechanoluminescent film. (b) The ML photos of Ca0.93SrGa4O8:0.07Tb3+ (compress and stretch). (c) The multi-mode anti-counterfeiting model: using a glass sleeve to write C, S, G, O, respectively. | |
An interesting observation regarding the ML spectrum is its slight redshift compared to the PL spectrum, shown in Fig. 4(b). By applying mechanical stimuli on the particles of CSGOT, lattice shrinks and crystal field strengthens. Consequently, crystal field splitting of 5d energy level of Tb3+ ions increases and the 5d lowest energy level decreases, resulting in the spectral redshift of Tb3+.36 This redshift in the ML spectrum under mechanical stress highlights the sensitivity of Tb3+ doped CaSrGa4O8 phosphors to changes in their physical environment, underlining their potential in applications requiring responsive luminescent materials. When stretching or compression force is applied to the flexible film, the bright ML phenomenon is displayed in Fig. 5(b). Characters such as Ca, Sr, Ga, and O are written on the ML film, and the note mapping is also clearly visible. The gray value can be obtained by analyzing the picture, and then the force of the sample can be more intuitively understood in Fig. 5(c).
3.4. TL/XPS properties of Ca1−xSrGa4O8:xTb3+
The luminescence emission spectrum of the Ca1−xSrGa4O8:xTb3+ phosphors demonstrate characteristic line emissions of Tb3+ in the 50–200 °C temperature range. This observation confirms that the stored energy is released from heat-activated traps and then recombines with ionized Tb3+ ions. With an increase in Tb3+ content, there is a notable decrease in the level of shallow traps. This reduction facilitates the easier release of charge carriers under mechanical stimulation, leading to enhanced mechanoluminescent behavior.36 The average lifetime of CSGOT, as shown in Fig. 2(e), gradually decreases with varying doping ion concentrations in a deeper understanding of the capture and release of electrons in the sample, thermoluminescence spectroscopy tests were conducted, focusing on excitation and decay time. The results, depicted in Fig. 6(a) and (c), show that initially when the traps are almost empty, there is a weak thermoluminescence intensity and emission of green light. However, as the irradiation time increases, both the green emission and thermoluminescence intensity of the sample also increase. This trend suggests that with prolonged excitation, more electrons are trapped, leading to a rise in the thermoluminescence peak. The consistent peak position across different tests indicates that electrons are captured at a similar rate in various traps. After several minutes of excitation, the peak intensity of thermoluminescence stabilizes across all samples, signifying that the traps have reached their electron capacity. Furthermore, as the decay time increases, the thermoluminescence peak shifts towards higher temperatures. Fig. 6(b) and (d) reveal that electron release is faster in shallow traps. In CSGOT, despite higher TL peak intensity, the shallower traps allow trapped electrons to escape more easily to the conduction band (CB), resulting in LPL emission and faster decay.
 |
| Fig. 6 (a and b) TL curves of CaSrGa4O8. (c and d) TL curves of Ca0.93SrGa4O8:0.07Tb3+. (e) TL curves of the Ca1−xSrGa4O8:xTb3+ (f) XPS survey spectrum of Ca0.93SrGa4O8:0.07Tb3+ and the insets show the XPS analysis of the O 1s orbital for the Ca0.93SrGa4O8:0.07Tb3+. | |
As the Tb3+ doping concentration increases, the depth of the shallow trap's defect level significantly decreases in Fig. 6(e), implying that trapped carriers are more readily released by thermal vibration or mechanical force. This change is attributable to both intrinsic defect
and extrinsic defects
. The introduction of Tb3+ ions also lead to a shift in the thermoluminescence peak towards higher temperatures, along with a significant increase in its intensity. This shift and intensity increase indicate that Tb3+ doping not only enhances the overall concentration of traps within the material but also introduces additional deep trap energy levels. These deep trap levels are likely responsible for the observed changes in the thermoluminescence characteristics, reflecting a more complex energy landscape for carrier dynamics within the material. The presence of these deeper traps and the shift in thermoluminescence behavior underscores the significant impact of Tb3+ doping on the material's electronic structure and its potential applications in devices that rely on carrier trapping and release mechanisms.
X-ray photoelectron spectroscopy (XPS) is a valuable analytical tool for analyzing the valence states of elements in a sample and recording changes in oxygen vacancy numbers and the surrounding coordination environment. After testing, the data were calibrated to a C 1s electron peak at 284.8 eV. XPS spectroscopy performed at binding energies of 0–1350 eV on the synthesized Ca0.93SrGa4O8:0.07Tb3+ phosphor revealed clear signals of Ca, Sr, Ga, O, and Tb elements in Fig. 6(f). The O 1s orbit could be decomposed into two peaks at 530.98 eV (OI) and 531.78 eV (OII). The lower binding energy component (OI) is primarily due to bridge oxygen (lattice oxygen), while the OII peak, which is about 0.8 eV higher in binding energy than OI, is related to oxygen vacancies. This finding indicates an increase in oxygen vacancies, corroborating the thermoluminescence data that associate shallow traps with oxygen vacancies in the samples.
3.5. ML application demonstration and mechanism investigation
Under ultraviolet excitation, the CIE coordinates of the samples with different doping concentrations remained basically unchanged, showing significant green emission in Fig. 7(a). Without mechanical stress, free electrons and holes in the Ca1−xSrGa4O8:xTb3+ phosphors move randomly within their respective conduction and valence bands. However, under mechanical force stimulation, these free electrons and holes combine in proximity to each other, releasing energy in the process. This released energy is then transferred to the Tb3+ ions, leading to the occurrence of the ML phenomenon, as depicted in Fig. 7(b). By studying the intrinsic structure, matrix effects, and trap evolution, it is confirmed that deep trap and shallow trap are the causes of the observed ML and LPL, respectively.
 |
| Fig. 7 (a) CIE chromaticity diagram corresponding to the Ca1−xSrGa4O8:xTb3+ emission intensity. (b) ML processes of the Ca1−xSrGa4O8:xTb3+ in the energy level diagram. | |
4. Conclusion
In summary, Ca1−xSrGa4O8:xTb3+ phosphors have been synthesized and their optical luminescent properties have been investigated. The addition of Tb3+ introduces a wealth of trap energy levels and brings excellent long afterglow and stress luminescence properties. Moreover, deep and shallow traps are identified as the primary causes of the observed ML and LPL phenomena, respectively, highlighting the critical role of trap-controlled luminescence processes in optical properties, which brings more possibilities for the materials in note mapping and stress sensing.
Author contributions
Rui Shi: conceptualization, visualization, formal analysis, writing – original draft, project administration. Wei Liu: supervision, data curation, formal analysis, visualization, investigation, writing – review and editing, project administration. Lin Li and Huan Li: data curation, formal analysis, investigation. Zhijun Zhang: supervision, writing – review and editing, project administration. Guanghui Rao and Jingtai Zhao: funding acquisition, project administration, supervision, writing – review and editing.
Conflicts of interest
The authors declare no conflict of interest.
Acknowledgements
This work is supported by the fund from Guangxi Science and Technology Base and Talent Special Project (2020AC18005), Innovation Project of Guangxi Graduate Education (YCSW2022267), Central Government Guided Local Science and Technology Development Fund Project (ZY22096009) and Foundation for Guangxi Bagui scholars.
References
- Z. Wang, S. Zhao, Y. X. Wang, F. Wang, A. A. Ansari and R. Lv, Anal. Bioanal. Chem., 2023, 1–10 DOI:10.1007/s00216-023-04968-1.
- B. Chen, X. Zhang and F. Wang, Acc. Mater. Res., 2021, 2, 364–373 CrossRef CAS.
- J. C. G. Bünzli and K. L. Wong, J. Rare Earths, 2018, 36, 1–41 CrossRef.
- Y. Xie and Z. Li, Mater. Chem. Front., 2020, 4, 317–331 RSC.
- S. M. Jeong, S. Song, H. Kim, K. Joo and H. Takezoe, Adv. Funct. Mater., 2016, 26, 4848–4858 CrossRef CAS.
- S. M. Jeong, S. Song, K. I. Joo, J. Kim, S. H. Hwang, J. Jeong and H. Kim, Energy Environ. Sci., 2014, 7, 3338–3346 RSC.
- M. Y. Wang, H. Wu, W. B. Dong, J. Y. Lian, W. X. Wang, J. Y. Zhou and J. C. Zhang, Inorg. Chem., 2022, 61, 2911–2919 CrossRef CAS PubMed.
- Z. X. Zhou, K. H. Liu, Z. Y. Ban and W. Z. Yuan, Composites, Part A, 2022, 154, 106806 CrossRef CAS.
- N. Terasaki and C. N. Xu, Jpn. J. Appl. Phys., 2009, 48, 04C150 CrossRef.
- S. Krishnan, H. Van der Walt, V. Venkatesh and V. B. Sundaresan, J. Intell. Mater. Syst. Struct., 2017, 28, 2458–2464 CrossRef CAS.
- N. Terasaki, Y. Fujio, Y. Sakata, S. Horiuchi and H. Akiyamaet, J. Adhes., 2018, 94, 867–879 CrossRef CAS.
- H. Song, S. Timilsina, J. Jung, T. S. Kim and S. Ryu, ACS Appl. Mater. Interfaces, 2022, 14, 30205–30215 CrossRef CAS PubMed.
- C. J. Chen, Y. X. Zhuang, D. Tu, X. D. Wang, C. F. Pan and R. J. Xie, Nano Energy, 2020, 68, 104329 CrossRef CAS.
- Y. Jia, M. Yei and W. Jia, Opt. Mater., 2006, 28, 974–979 CrossRef CAS.
- N. Terasaki and C. N. Xu, IEEE Sens. J., 2013, 13, 3999–4004 Search PubMed.
- F. T. Freund, J. Sci. Explor., 2003, 17, 37–71 Search PubMed.
- Y. Zhou, Y. L. Yang, Y. T. Fan, W. Yang, W. B. Zhang, J. F. Hu, Z. J. Zhang and J. T. Zhao, J. Mater. Chem. C, 2019, 7, 8070–8078 RSC.
- Y. L. Yang, T. Li, F. Guo, J. Y. Yuan, C. H. Zhang, Y. Zhou, Q. L. Li, D. Y. Wan, J. T. Zhao and Z. J. Zhang, Inorg. Chem., 2022, 61, 4302–4311 CrossRef CAS PubMed.
- Z. J. Zhang, J. T. Zhao, H. H. Chen, Z. Y Man and X. X. Yang, Chinese Pat., 201110155312.6, 2011 Search PubMed.
- Y. Y. Du, Y. Jiang, T. Y. Sun, J. X. Zhao, B. L. Huang, D. F Peng and F. Wang, Adv. Mater., 2019, 31, 1807062 CrossRef PubMed.
- T. L. Wang, F. Liu, Z. Q. Wang, J. Zhang, S. S. Yu, J. X. Wu, J. H. Huang, W. J. Wang and L. Zhao, Dalton Trans., 2022, 51, 12290–12298 RSC.
- T. Wang, X. H. Xu, D. C. Zhou, Y. Yang, J. B. Qiu and X. Yu, Inorg. Chem., 2016, 55, 894–901 CrossRef CAS PubMed.
- A. Bessière, S. Jacquart, K. Priolkar, A. Lecointre, B. Viana and D. Gourier, Opt. Express, 2011, 19, 10131–10137 CrossRef PubMed.
- O. Q. De Clercq, L. I. D. J. Martin, K. Korthout, J. Kusakovski, H. Vrielinck and D. Poelman, J. Mater. Chem. C, 2017, 5, 10861–10868 RSC.
- A. Abdukayum, J. T. Chen, Q. Zhao and X. P. Yan, J. Am. Chem. Soc., 2013, 135, 14125–14133 CrossRef CAS PubMed.
- F. Liu, Y. F. Chen, Y. J. Liang and Z. W. Pan, Opt. Lett., 2016, 41, 954–957 CrossRef PubMed.
- H. C. Sun, Q. Zhu and J. G. Li, Ceram. Int., 2022, 48, 9640–9650 CrossRef CAS.
- X. H. Zhang, D. Yang, S. F. Wu, X. M. Xu, R. H. Ma, D. F. Peng, Z. L. Wang and S. H. Wang, Dalton Trans., 2022, 51, 10457–10465 RSC.
- F. Wang and X. G. Liu, Acc. Chem. Res., 2014, 47, 1378–1385 CrossRef CAS PubMed.
- C. C. Wang, Z. C. Liu, A. N. Yakovlev, T. T. Hu, T. G. Cherkasova, X. D. Zhu, Y. Liu, J. Zhang, D. Y. Liu and X. Yu, RSC Adv., 2023, 13, 16405–16412 RSC.
- J. Lin, Y. Huang, J. Zhang, F. J. Shi, S. Y. Wei, J. M Gao, Z. X. Huang, X. X. Ding and C. C. Tang, Mater. Chem. Phys., 2008, 108, 440–444 CrossRef CAS.
- X. Yu, S. B. Wang, Y. C. Zhu, J. J. Liang, J. B. Qiu, X. H. Xu and W. Lu, J. Alloys Compd., 2017, 701, 774–779 CrossRef CAS.
- Y. H. Chen, P. Fan, Z. F. Xu, Q. Luo, Z. J. He, Q. Q. Miao, C. Huang, X. He, Q. Zhang, Z. G. Wang, X. G. Li and L. Li, Anomalous Intense 5d0-7f4 Emission, the Adjustable Emission and Energy Transfer in CaSrGa4O8: Eu3+/Bi3+, SSRN, 2022, 4093971 Search PubMed.
- C. C. Zhu, L. Q. Tao, Z. R. Peng, G. Y. Wang, Y. X. Huang, S. M. Zou, H. Sun, Y. F. Zhao, X. P. Chen and T. L. Ren, Adv. Funct. Mater., 2021, 31, 2103255 CrossRef CAS.
- S. S. Ding, P. Chen, H. J. Guo, P. Feng, Y. P. Zhou, Y. H. Wang and J. L. Sun, J. Energy Chem., 2022, 69, 150–160 CrossRef CAS.
- Y. Xiao, P. X. Xiong, S. Zhang, K. Chen, S. H. Tian, K. Chen, S. H. Tian, Y. S. Sun, P. S. Shao, K. X. Qin, M. G. Brik, S. Ye, D. D. Chen and Z. M. Yan, Chem. Eng. J., 2023, 453, 139671 CrossRef CAS.
- B. L. Xiao, P. X. Xiong, S. Wu, D. L. Jiang, K. Chen, Y. Xiao, P. S. Shao and Y. Z. Wang, Adv. Opt. Mater., 2023, 11, 2300911 CrossRef CAS.
- T. Hu, Y. Gao, B. Wang, T. Yu, D. W. Wen, Y. Cheng and Q. G. Zeng, J. Mater. Chem. C, 2022, 10, 9554–9562 RSC.
- S. Y. Qin, J. L. Bian, Y. Han, Z. D. Ma, B. Liu, J. C. Zhang, X. H. Xua and Z. F. Wang, Mater. Res. Bull., 2022, 145, 111535 CrossRef CAS.
- G. G. Zhang, Y. L. Sun, B. K. Qian, H. Gao and D. Zou, Polym. Test., 2020, 90, 106670 CrossRef CAS.
|
This journal is © The Royal Society of Chemistry 2024 |