DOI:
10.1039/D4RA01665C
(Paper)
RSC Adv., 2024,
14, 11939-11948
Novel ultrasonic technology for advanced oxidation processes of water treatment
Received
4th March 2024
, Accepted 3rd April 2024
First published on 15th April 2024
Abstract
Textile wastewater accounts for a significant proportion of industrial wastewater worldwide. In particular, dye wastewater accounts for a large proportion and consists of non-degradable dyes, which are substances resistant to biodegradation. Methylene blue is a representative example of such non-degradable dyes. It is not biologically degraded and exhibits toxicity. Various methods for their decomposition are currently being studied. Advanced oxidation processes (AOPs), which generate highly reactive hydroxyl radicals that oxidize and degrade pollutants, have been actively studied. Particularly, the photocatalytic degradation method using TiO2 nanoparticles is one of the most actively studied fields; however, there are still concerns regarding the toxicity of nanoparticles. Research is currently being conducted on AOPs using the cavitation phenomenon of ultrasonic waves. However, achieving high efficiency using existing ultrasonic equipment is difficult. Therefore, in this study, we evaluated a new water treatment technology through AOPs using a focused ultrasonic system with a cylindrical piezoelectric ceramic structure. After determining the optimal conditions for degradation, the degradation process was evaluated as a useful tool for mitigating the toxicity of methylene blue. We found that, under the optimal conditions of 100 W intensity at a frequency of 400 kHz, this system is a helpful instrument for degradation and a new water treatment technology suitable for removing ecotoxicity and genotoxicity.
Introduction
Textile manufacturing generates considerable amounts of dye wastewater. It is estimated that wastewater generated from the textile industry accounts for a significant portion of water pollution, among which dye wastewater is known to contribute substantially.1–5 Wastewater poses a major challenge in terms of environmental sustainability, and reducing its emissions is crucial.6–8
Methylene blue, an organic dye, is commonly used as a dye and indicator.9–12 It is a non-degradable dye that does not degrade easily by natural methods such as microbial degradation or photolysis, and undegraded methylene blue accumulates in the environment when discharged, such as in aquatic environments.9,13,14 Additionally, the presence of methylene blue in the aquatic environment can lead to toxicity in aquatic organisms, which can affect humans.13–18
Various methods have been studied to degrade these non-degradable substances. Among these, advanced oxidation processes (AOPs) are some of the most notable water treatment technologies. AOPs are treatment technologies that remove pollutants from wastewater using hydroxyl radicals with strong oxidation properties.19–21 Hydroxyl radicals are among the most powerful oxidizing agents currently known. They consist of one oxygen atom and one hydrogen atom and have highly reactive properties, allowing them to readily react with organic materials. When organic pollutants react with hydroxyl radicals, mineralization occurs, degrading them into water or carbon dioxide.20,22 Several studies have elucidated the degradation path of methylene blue through AOPs. Methylene blue is ultimately degraded into harmless substances, including H2O and CO2, through AOPs. As a result of several AOPs, methylene blue, with its complex structure, is commonly converted into a harmless substance.9,23,24 Thus, this reaction can convert contaminated organic matter into harmless substances. Therefore, AOPs using this radical have attracted attention as useful processes for removing pollutants from wastewater.
The AOPs technology using TiO2 photocatalytic nanoparticles is one of the most actively researched.25,26 This process is based on the photocatalytic activity of TiO2. When ultraviolet (UV) light irradiates TiO2, an electron–hole pair is formed, which is a helpful process that can effectively oxidize organic pollutants by producing highly reactive radicals and molecules such as hydroxyl radicals, peroxide radicals, and hydrogen peroxide.25,26 However, the use of TiO2 nanoparticles requires further evaluation. There are concerns regarding the potential toxicity of TiO2 to aquatic environments.27–34 TiO2 nanoparticles readily bind to heavy metals such as cadmium, and nanoparticles attached to cadmium are taken up by aquatic organisms, which causes genetic toxicity in the species. Studies on TiO2 toxicity have been reported.35 Therefore, to use TiO2 particles in AOPs technology, discussions on the filtration of particles must also be conducted.
AOPs technology using ultrasonication has attracted considerable attention.36,37 This method has recently attracted much attention because it degrades pollutants using only ultrasonic energy and does not require the addition of additional catalysts, such as photocatalytic particles.38–41 AOPs technology using ultrasonic energy is gaining attention as a valuable degradation technology because both the physical and chemical effects of ultrasonic waves can be expected. The physical and chemical effects of ultrasound are based on cavitation.42–45
The physical degradation effect of ultrasonication is achieved through the collapse of cavitation bubbles. When ultrasonic energy is continuously applied to the liquid, the generation and collapse of cavitation bubbles occur in succession. When a cavitation bubble collapses, high-temperature and high-pressure energy is released around it. In this process, pollutants such as organic dyes are damaged and degraded by the released energy.46,47
The chemical degradation effect of ultrasonication, also known as sonochemistry, is achieved by the collapse of air bubbles generated via cavitation. When bubbles collapse, highly reactive oxidants, such as hydroxyl radicals, are produced because of the degradation of water molecules in the liquid. The generated radicals react with pollutants, such as organic dyes, and eventually degrade them into harmless substances, such as water and carbon dioxide. Therefore, a high-diffusion process using ultrasonic waves with physical and chemical effects has gained recognition as a valuable technology for water treatment.46–48
However, several challenges are associated with implementing AOPs technology using the currently available ultrasonic instruments. Bath- and horn-type ultrasonic equipment, which are widely used, face difficulties in controlling noise and heat generation and have a relatively limited frequency range. Notably, these devices generate energy unevenly owing to the interference between the internal sound waves. Therefore, predicting the optimal effect of AOPs water treatment using the existing ultrasonic equipment is difficult.
In this study, water treatment was performed using a novel ultrasonic technology to address this issue; a focused ultrasonic system was used to research more efficient and optimized degradation methods and processes than the conventional ultrasonic degradation processes. Unlike existing ultrasound systems, focused ultrasonic systems have cylindrical piezoelectric ceramic structures. A piezoelectric ceramic with a cylindrical structure concentrates energy in the center and is transferred to the sample uniformly and strongly compared to existing ultrasonic equipment. This focused approach was employed to predict the degradation effect of organic dyes.49,50
This study aimed to demonstrate that water treatment technology using a focused ultrasonic system is more effective than other methods. Simultaneously, we aimed to determine the optimal conditions of focused ultrasonic equipment for water treatment technology. Therefore, this study was conducted to evaluate the resolution of each process method through a methylene blue degradation experiment and to assess the toxicity of the degraded methylene blue.
Experimental
Materials
The methylene blue used in the experiment was a high-purity analytical-grade methylene blue solution (M2661, 0.1%; SAMCHUN Chemicals, Co., Ltd, Pyeongtaek, South Korea), which was diluted to a concentration of 10 ppm. At this time, methylene blue was diluted in tertiary deionization water, and deionization water was obtained through the Direct-Q(R) 3 UV Water Purification System (ZRQSVP3EU, Merck Millipore, Burlington, VT, USA). All experiments were conducted using 100 mL of methylene blue diluted to 10 ppm. In addition, the TiO2 particles Evonik's P25 powder.
Methods and analysis
UV/TiO2 degradation. In the UV/TiO2 degradation experiment, TiO2, in which the mode value (the value of the highest peak in the particle-size distribution) was dispersed to approximately 83 nm was used. TiO2, dispersed in 100 mL of methylene blue at 10 ppm, was added to achieve a concentration of 0.05 g L−1. Thereafter, for the adsorption of TiO2 particles and methylene blue, the solution was stirred at 200 rpm for 30 min in a dark room. The sample was irradiated with a Bio-Link crosslinker (BLX; Vilber Lourmat, Colégien, France) at a UV power of 5 × 8 watts, and a degradation experiment was conducted by irradiating the sample with UV-A.
Ultrasonic degradation. The Bath-type sonicator, previously a commercial equipment, was used in this study using a device from MUJIGAE. The frequency range of the equipment is in the 40 kHz range and has a power output of approximately 100 W. Similarly, the cuphorn-type sonicator is an existing commercial device; in this study, Branson's equipment was used. Cuphorn used a 3′′ Diameter Cup Horn for ultrasonication. The cell disruptors (No. 101-147-048) and power supply equipment used were Branson's 550 equipment. The frequency range of the equipment was 20 kHz, and the power was 110 W. In both equipment experiments, tap water was used as the cooling medium to regulate energy transfer and heat generation. The experiment involved fixing the methylene blue sample to prevent it from floating in a bath and cup horn containing tap water, followed by operating the equipment to irradiate the ultrasonic waves. During this process, ice was continuously added to the cooling water to control the heat generation. However, in both types of equipment, the area affected by the frequency was not constant, and the frequency wavelengths offset each other, resulting in an energy imbalance. Additionally, significant noise and difficulties in controlling heat generation make it impractical for extended use.To address this issue, this study introduced a focused ultrasonication system (FS-R01K1; FUST Lab, Daejeon, South Korea). Unlike the two pieces of equipment mentioned above, this equipment can use a high-frequency ultrasonic range of 100 kHz or more. In this study, experiments were conducted in three frequency regions (340, 400, and 700 kHz) and three power conditions: 60, 100, and 150 W. As previously mentioned, tap water was used as the cooling water to control the ultrasonic transmission stores and heat generation. Ultrasonic waves are transmitted from generators (NF, WF 1974) to cylindrical piezoelectric ceramics attached to the focused ultrasonic waves through amplifiers (NF, HAS 4014). In this case, the ultrasonic energy was concentrated at the center through the cooling water, creating a high-temperature and high-pressure state. Consequently, strong and uniform energy is transmitted inside, and the generation and collapse of cavitation bubbles are repeated.49,50 When a cavitation bubble collapses, the intense energy latent in the bubble is released outward, and the energy physically and chemically affects the methylene blue molecule. In the experiment, methylene blue samples were circulated through the equipment at a rate of 5.0 mL min−1 using a pump (LongerPump, WT600-1F) to irradiate ultrasonic waves, and cooling water was also circulated at a rate of 10.0 mL min−1 using the same pump to control heat generation and transfer ultrasonic energy to the sample.
UV-visible. After the experiment, absorbance was measured using a UV-visible spectrophotometer (UV-180, Shimadzu, Kyoto, Japan) to evaluate the degradation of methylene blue. The absorption was measured between 800 and 500 nm, and plastic cells unaffected by transmission were used in the corresponding wavelength bands. The resolution was evaluated based on the peak measured at approximately 665 nm, which is the absorption wavelength band of methylene blue, and the peak shift phenomenon caused by the oxidation of methylene blue was considered during the advanced oxidation process. During the measurement, samples were collected and measured at approximately 2 mL, and in the case of a sample using TiO2, the measurement was carried out after the TiO2 was removed using a cylinder filter. Degradation (%) was calculated using the following equation, according to the concept that the absorbance of the Beer–Lambert law is proportional to the concentration:49,51
where A0 is the absorbance of undegraded methylene blue, and A is the absorbance of degraded methylene blue. Degradation (%) was calculated through the corresponding calculation formula here.
Toxicity evaluation
Daphnia survival rate. The MB toxicity of methylene blue was evaluated using a customized Waterfela-Tox Test Kit (Suncheon, Korea). Five milliliters of 2× ecotoxicity test medium (1 L ultrapure water, 240 mg calcium sulfate dihydrate, 240 mg magnesium sulfate, 16 mg potassium chloride, and 384 mg sodium bicarbonate) were divided into 20 test tubes. A stock of standard pollutants (potassium dichromate, K2Cr2O7; 6, 3, 1.5, 0.75 mg L−1) or measurement substances (methylene blue-containing solution) was added to 5 mL each (final concentration of ecotoxicity test medium, 1×; final concentration of potassium dichromate, 3, 1.5, 0.75, and 0.375 mg mL−1). Five D. magna neonates (born within 24 h) were placed in each test tube, and the tubes were carefully inverted several times for mixing (each group comprised two tubes, including 10 D. magna). After 24 h, the live D. magna cells in each test tube were counted and compared. The experiment was repeated three times, and statistical analysis was performed using a t-test.
Sample preparation. Five samples were evaluated for toxicity at 10 ppm concentration of methylene blue; two samples decomposed for 2 h and 3 h by UV + TiO2, and one sample of methylene blue was treated for 2 h by focused sonication. The Daphnia toxicity assessment was conducted in three repeated experiments, and the criteria for toxicity assessment were evaluated for the water flea survival rate over 24 h.
Genotoxicity
Chemicals and reagents. Distilled water (DW; Gibco, USA) was used as the negative reagent. Six positive reagents were purchased from Sigma-Aldrich (USA): sodium azide (SA), 2-nitrofluorene (2-NF), 9-aminoacridine (9-AA), 4-nitroquinolone N-oxide (4NQO), 2-aminoanthracene (2-AA), and benzo[a]pyrene (BP).To prepare the metabolic activation system (S9; Molecular Toxicology Inc., USA) mix, the Aroclor 1254-induced Sprague-Dawley rat liver S9 metabolic activation system was mixed with a cofactor (Wako Pure Chem. Ind. Ltd, Tokyo, Japan).52
Sample preparation. Five samples were evaluated for toxicity: methylene blue at untreated 10 ppm and 20 ppm concentrations, a sample decomposed by the UV + TiO2 method for 1 h, and a sample treated by the focused sonication method for 1 h. All samples were prepared in 100 mL volume, and each sample was repeatedly tested and evaluated twice.
In vitro bacterial reverse mutation test (Ames test). The Ames test was carried out according to the OECD test guidelines 471, which are based on the method of Maron and Ames with minor modifications.53 Briefly, S. typhimurium strains TA98, TA100, TA1535, and TA1537 and E. coli WP2uvrA (Molecular Toxicology, Inc.) were used as tester strains in the presence or absence of 5% v/v Aroclor 1254-induced rat liver S9 metabolic system (S9 mix). Based on the dose-range test results, 10 and 20 ppm per plate were selected as the maximum concentration for the Ames test of MB (UV + TiO2) and MB (focused ultrasonication).
Results
Comparison of degradation methods
The Fig. 1a and c illustrates a comparison of degradation percent and rate when methylene blue containing TiO2 nanoparticles, which are photocatalytic particles, were degraded for 1 h based on the three types of ultrasonic equipment and the UV irradiation degradation method. When TiO2 was added in methylene blue, approximately 79.5% of methylene blue was degraded under UV irradiation. In the case of ultrasonic equipment, bath-type equipment did not degrade methylene blue, Cuphorn equipment degraded approximately 12.1%, and focused ultrasonic equipment degraded 87.6%. Existing ultrasonic equipment showed a slightly lower methylene blue resolution; however, in the case of focused ultrasonic waves, it was confirmed that the degradation was better than that of the UV/TiO2 process.
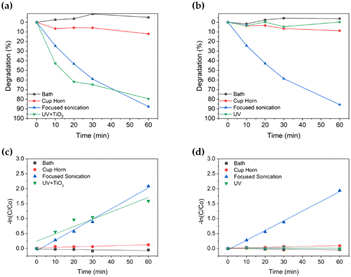 |
| Fig. 1 Degradation (%) comparison according to degradation type; (a): TiO2 used; (b): TiO2 not used. And degradation rate [−ln(C/C0)] comparison according to degradation type; (c): TiO2 used; (d): TiO2 not used. | |
Fig. 1b and d shows an experimental result that does not contain TiO2 particles, which are photocatalytic. When TiO2 was omitted, methylene blue showed minimal degradation under UV irradiation and bath-type conditions. In the cup horn type, degradation occurred in approximately 8.8% of cases, but the degree was insignificant. However, in the case of focused ultrasound, approximately 85.6% of the dye and methylene blue were degraded to a level slightly different from that in the presence of TiO2 particles. The results of the color degradation experiment with the focused ultrasonic method were notably favorable. In the case of the UV type, the degradation performance varied greatly depending on the presence or absence of TiO2. For the cup horn type, the degradation performance differed slightly depending on the presence or absence of TiO2. For the bath type, the methylene blue solution showed minimal degradation in either case without a difference in the presence or absence of TiO2.
Therefore, in the degradation experiment of methylene blue, according to the degradation method, the existing ultrasonic equipment exhibited minimal degradation; however, in the case of focused ultrasonic waves, it was confirmed that the resolution was outstanding, regardless of whether TiO2 was used.
Effects of focused ultrasound conditions
Frequency condition. Fig. 2a and c displays the experimental results of the degradation of methylene blue-containing TiO2 particles for 1 h according to the frequency range of the focused ultrasonication system. The conditions, other than the frequency, were the same. After 1 h, the degradation of methylene blue was approximately 20.3% at 340 kHz, 78.4% at 700 kHz, and 87.6% at 400 kHz. Methylene blue degradation was more pronounced at 400 kHz than at other frequencies. At 700 kHz, the degradation was superior to that at 340 kHz, but the resolution was approximately 10% different from that at 400 kHz. At 340 kHz, although TiO2 particles were present, degradation did not occur as well as in other frequency regions.
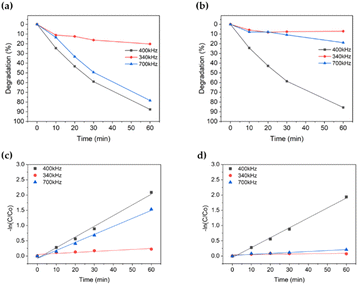 |
| Fig. 2 Degradation (%) comparison based on frequency; (a): TiO2 used; (b): TiO2 not used. And degradation rate [−ln(C/C0)] comparison based on frequency; (c): TiO2 used; (d): TiO2 not used. | |
Fig. 2b and d presents the experimental results for the methylene blue without TiO2 particles. Other than those for TiO2, the conditions were the same as before. Consequently, 340 kHz was degraded by 7.1%, 700 kHz by 18.9%, and 400 kHz by 85.6%. Similar to TiO2, the highest degradation of methylene blue was observed at 400 kHz. In the absence of TiO2, methylene blue degradation was significantly enhanced at 400 kHz. In the case of 340 kHz, the resolution was considerably lower, and in the case of 700 kHz, the resolution was significantly lower than in the previous results.
Therefore, regardless of the presence or absence of TiO2 in the focused ultrasound process, the 400 kHz frequency range was optimal.
Power condition. Fig. 3a and c shows the results of the experiments with the degradation performance of methylene blue in accordance with each power condition at a frequency of 400 kHz when TiO2 particles are present. The results showed that the resolutions were 41.6% at 150 W, 87.6% at 100 W, and 93.0% at 60 W. For 150 W, the largest power intensity, the resolution was less than 50%, and the degradation was the slowest. Both 60 and 100 W exhibited more than 80% resolution, with the optimal degradation at 60 W by a narrow margin. Even at the highest power intensity of 150 W, the degradation performance was the worst under the corresponding conditions. However, at 60 and 100 W, even though the power was lower than 150 W, the degradation performance of methylene blue was notably high.
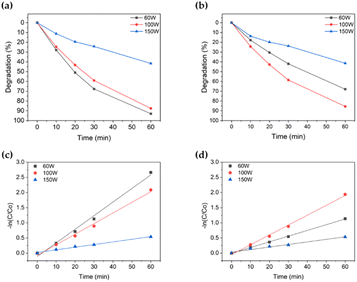 |
| Fig. 3 Degradation (%) comparison according to power; (a): TiO2 used; (b): TiO2 not used. And degradation rate [−ln(C/C0)] comparison according to power; (c): TiO2 used; (d): TiO2 not used. | |
Fig. 3b and d displays the experimental results when TiO2 is not present. Methylene blue showed 41.4% degradation performance at 150 W, 67.9% at 60 W, and 85.6% at 100 W. In the absence of TiO2, the highest resolution was achieved at 100 W, followed by 60 W, with an approximately 20% difference between them. The resolution at 150 W was the lowest. Additionally, depending on the presence or absence of TiO2, the degradation performance was similar at 100 and 150 W, but it differed by approximately 20% at 60 W.
In summary, at a frequency of 400 kHz, the resolution at 100 W was excellent regardless of the presence or absence of TiO2, and at 60 W, the resolution differed by approximately 25%, depending on the presence or absence of TiO2. In the case of 150 W, with the highest power strength, the degradation occurred at the slowest rate in all cases. Therefore, it was confirmed that the optimal condition for decomposing methylene blue is at a strength of 100 W in the frequency range of 400 kHz using the focused ultrasonication system.
Toxicity assessment
Ecotoxicology
Comparison of UV/TiO2 and focused ultrasonication system methods. The ecotoxicity of the degraded methylene blue was evaluated for methylene blue degradation at a strength of 100 W under 400 kHz conditions using a focused ultrasonication system without TiO2 and its degradation using UV/TiO2 photocatalytic methods (Table 1). Additionally, toxicity evaluation was conducted in a standard contaminated solution containing K2Cr2O7 as a comparative experimental group.
Table 1 Methylene blue sample information
|
Degradation methods |
Degradation time (h) |
Sample 1 |
X |
0 |
Sample 2 |
UV/TiO2 |
2 |
Sample 3 |
Focused sonication |
2 |
Fig. 4 presents the experimental results for ecotoxicology. In all repeated experiments, not all Daphnia survived for 24 h in methylene blue at 10 ppm without degradation. In the first experiment, only two Daphnia survived for 24 h when methylene blue was degraded by the UV/TiO2 method for 2 h. However, seven Daphnia survived methylene blue degradation for 2 h by focused ultrasound, and the survival rate was significantly higher than that of methylene blue degraded by other methods.
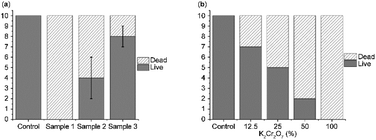 |
| Fig. 4 Comparison of ecotoxicity when methylene blue is degraded by UV/TiO2 method and the focused ultrasonication method (treatment after 24 h); (a) Daphnia toxicity of experimental solution (repeated 3 times) (b) standard contaminated solution for comparison. | |
In the second experiment, Daphnia survived when methylene blue was degraded by the UV/TiO2 method for 2 h, and nine Daphnia survived when the focused ultrasonication system degraded methylene blue. The survival rate in methylene blue degraded by the UV/TiO2 method was higher than that in the first experiment. However, it did not reach the level of toxicity removal of methylene blue degraded by the focused ultrasonication system.
In the third experiment, five Daphnia survived in methylene blue degraded by the UV/TiO2 method at 2 h, and eight Daphnia survived in methylene blue degraded by focused ultrasonic waves. Therefore, when comparing the two methods, the survival rate of Daphnia in the methylene blue degraded by focused ultrasound remained high.
In the experiment involving the standard solution, which served as a comparative group, when the ratio of K2Cr2O7 was 12.5, 25, 50, then 100, 7, 5, 2, and 0% water fleas survived, respectively. Compared with previous experiments, methylene blue, which has not been degraded, has a similar level of toxicity when the concentration of the contaminated solution was 100%, and methylene blue, which has been degraded by UV/TiO2 has a similar level of toxicity when the concentration of the contaminated solution is approximately 25–50%. The toxicity of methylene blue degraded by the focused ultrasonication system was lower than that of the 12.5% concentration of the contaminated solution.
As a result, compared to methylene blue degraded by the UV/TiO2 method, it was confirmed that toxicity was significantly lower when degraded by focused ultrasonic waves during the same degradation time. Therefore, the degradation method using the focused ultrasonication system is useful in terms of degradation and toxicity.
To determine whether differences in methylene blue decomposition by the UV/TiO2 method or the focused ultrasound method affected ecotoxicity, we evaluated the acute toxicity using Daphnia. To determine whether the experimental conditions for the Daphnia toxicity kit were appropriate, Daphnia were exposed to K2Cr2O7, a toxic standard substance, and their survival rates were determined. K2Cr2O7 induced the death of Daphnia in a concentration-dependent manner, and the IC50 value of K2Cr2O7 was also obtained at 0.75 mg L−1, similar to the value in the OECD guidelines (Fig. 5A). This suggested that the conditions for assessing D. magna toxicity were appropriate. Next, Daphnia were exposed to the decomposed methylene blue solution for 2 h using UV/TiO2 or focused ultrasound (Fig. 5B). Although all Daphnia were killed when exposed to a methylene blue solution without decomposition, approximately 45% survived when exposed to a methylene blue decomposition solution using the UV/TiO2 method. Remarkably, 80% of the Daphnia survived when exposed to a methylene blue decomposition solution using the focused ultrasound method, suggesting that methylene blue was effectively decomposed by the focused ultrasound method, lowering its ecological toxicity.
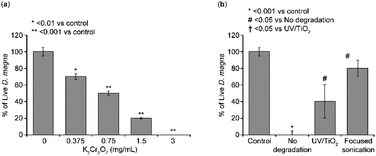 |
| Fig. 5 Comparison of ecotoxicity when methylene blue is degraded by UV/TiO2 method and the focused ultrasonication method (treatment after 24 h); (a) Daphnia toxicity in K2Cr2O7; (b) in methylene blue solution. | |
Comparison of sonicated time of focused ultrasonication system. The Based on the above experiments, when the focused ultrasonication system conditions were the same, toxicity evaluation of Daphnia according to degradation time was conducted. The result revealed that not all water fleas survived for 24 h in methylene blue that did not undergo degradation (Fig. 6). However, most Daphnia survived 30 min of focused ultrasonic degradation. Eight Daphnia survived at 30 and 60 min, and when the degradation time was more than 90 min, all Daphnia survived for 24 h. Compared to the above standard contaminated solution, the toxicity level was within 12.5% of the contaminated solution concentration for up to 60 min. After 90 min of degradation, toxicity was not detected for the control solution, indicating no harm to the Daphnia.
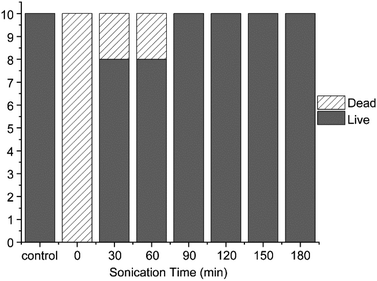 |
| Fig. 6 Comparison of ecotoxicity according to treatment time when methylene blue was treated with the focused ultrasonication system with optimal conditions (treatment after 24 h). | |
The outcomes of the ecotoxicity experiments demonstrate that using focused ultrasound for degrading methylene blue is an effective process for removing toxicity compared to other degradation methods. Furthermore, as the degradation time increases, the toxicity of methylene blue is completely removed.
Genotoxicity
In vitro bacterial reverse mutation test (Ames test)
The To evaluate the mutagenic potential, we subjected methylene blue to a bacterial reverse mutation test with S. typhimurium (TA98, TA100, TA1535, and TA1537) and E. coli WP2uvrA in the presence and absence of the rat liver S9 system at concentrations up to 20 ppm per plate (Fig. 7A and B). The dose-finding test revealed that, except for the S. typhimurium TA98 strain, there was no significant increase in the number of revertant colonies that met the positive criteria, regardless of the presence of the metabolic activation system. Furthermore, in the case of S. typhimurium TA98 treated with the metabolic activation system, a concentration-dependent increase in the number of revertant colonies was observed. Consequently, based on the findings of the dose-finding test, two concentration groups (10 and 20 ppm) were selected to assess the effectiveness of the methylene blue reverse mutation reduction method in the S. typhimurium TA98 strain when the metabolic activation system was applied.
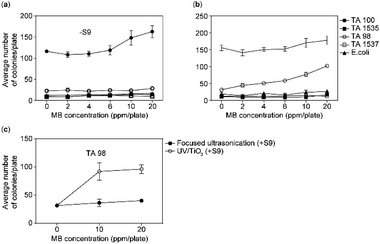 |
| Fig. 7 In vitro bacterial reverse mutation test of methylene blue (MB). The mean of revertant colonies without (a) and with S9 mix (b) in MB-treated bacteria strains. (c) Comparison of mutagenicity detection of degradation methods using Salmonella typhimurium TA98. | |
In this experiment, an increase in the number of revertant colonies was observed when methylene blue was applied in the concentration groups of S. typhimurium TA98 strain with a metabolic activation system. When two different toxicity reduction methods (focused sonication and UV/TiO2) were applied, the increase in revertant colonies in the UV/TiO2 treatment group, a conventional toxicity reduction method, was similar to that observed in the methylene blue treatment group. However, no increase in revertant colony count was observed in the focused sonication treatment group, which is a novel toxicity reduction method.
To measure the activity of the metabolic activation system, positive control substances 2-NF and BP, which require metabolic activation, were used. In the case of S. typhimurium TA98, significant mutagenicity was confirmed when a metabolic activation system was applied. The revertant colony count in the negative control group fell within the range of the historical control data, whereas in all positive control groups, the revertant colony count showed a significant increase (at least two-fold higher) compared to the negative control group.53 This indicated that the test was performed appropriately and supported the validity of the results.
Discussion
Comparison of degradation methods
The methods adopted for the degradation of methylene blue in the experiment were the UV light and the ultrasonic degradation method. The ultrasonic equipment used were bath, cup horn type, and a new focused ultrasonication system. The experiment results demonstrated that regardless of the presence or absence of TiO2 particles (which are photocatalytic), the focused ultrasonication method was superior to other methods. Additionally, the UV irradiation method showed a significant difference in resolution based on the presence or absence of TiO2 particles, whereas the other existing ultrasonic devices did not achieve sufficient degradation. Consequently, we confirmed that the focused ultrasonication system is an excellent instrument for degrading methylene blue.
Comparison of conditions of the focused ultrasonication system
Based on the initial results, an experiment was conducted to determine the optimal conditions for focused ultrasonication in the degradation of methylene blue. In this experiment, the variables considered were ultrasonic frequency and power. Among the various conditions tested, the best degradation occurred at 400 kHz frequency with 100 W power, regardless of TiO2. Therefore, it was established that the optimal conditions for focusing ultrasound on degraded methylene blue were 400 kHz frequency and 100 W power. The discussion on chemical effects is ongoing, but this study's findings are supported by research indicating superior chemical effects typically within the range of 300–500 kHz.54
Ecotoxicity
Based on the degradation experiment, we compared the ecotoxicity of methylene blue degradation using the UV/TiO2 method and the optimal conditions for the focused ultrasonic method. The result of the toxicity evaluation showed that, for the same degradation time, the survival rate of Daphnia was better under focused ultrasonication conditions. Additionally, the longer the degradation time through focused ultrasonication, the higher the survival rate of Daphnia, and after a specific time, all Daphnia survived. Therefore, toxicity was removed when methylene blue was degraded under the optimal conditions of the focused ultrasonication system.
Genotoxicity
The in addition to ecotoxicity, the genotoxicity when methylene blue was degraded using the UV/TiO2 method and the optimal conditions for focused ultrasonication were compared. Genotoxicity was evaluated for the five strains, and significant results were obtained for TA98. Consistent with the findings in the ecotoxicity experiment, the methylene blue degraded by the UV/TiO2 method showed genotoxicity, while the one degraded by the focused ultrasonication system did not show genotoxicity. Consequently, when methylene blue is degraded under the optimal conditions of focused ultrasound, its toxicity may be eliminated.
Conclusions
The In this study, we investigated the optimal degradation method of methylene blue, a representative non-degradable organic dye found in dye wastewater that is toxic and adversely affects aquatic environments. Among the various methods for methylene blue degradation, an optimal method is the advanced oxidation, which uses the physical and chemical effects of the cavitation phenomenon generated through ultrasonic waves. Therefore, a focused ultrasound system capable of maximizing this effect was used in this study. The degradation experiment compared various methods, such as photocatalysts and conventional commercial ultrasonic equipment. Among these, focused ultrasonic waves proved most effective in degrading methylene blue. Notably, among the various conditions of focused ultrasound, when the frequency of 400 kHz and the intensity of 100 W are satisfied, focused ultrasound degrades approximately 85.6% of methylene blue in 1 h, even in the absence of TiO2, a photocatalytic particle. Therefore, it was found that the degradation method using focused ultrasound is more efficient than other methods, and the optimal conditions for methylene blue degradation are a frequency of 400 kHz and a power of 100.
Furthermore, the potential residual toxicity in the degraded methylene blue was evaluated. The result of the toxicity evaluation showed that when degraded for the same duration, methylene blue degraded by focused ultrasound showed little ecotoxicity and genotoxicity, converse to methylene blue degraded by photocatalyst. Regarding ecotoxicity, all Daphnia survived for 24 h from 90 min after the focused ultrasonication degradation time. Genotoxicity was also absent when methylene blue was degraded for 1 h by the photocatalytic reaction for the same duration as focused ultrasound, compared to the TA98 strain. The findings of the toxicity evaluation confirmed that methylene blue degraded by focused ultrasound can remove ecotoxicity and genotoxicity that may affect the aquatic environment. Therefore, it is also a very useful instrument for mitigating toxicity.
In summary, this study reveals a useful AOP system that can remove toxicity along with optimal degradation methods and conditions for methylene blue. Additionally, it provides information on new water treatment technologies. Based on the findings of this study, we intend to further explore the degradation methods for various non-degradable materials, including dyes such as methylene blue. This study further suggests approaches to eliminate toxic substances affecting the aquatic environment, significantly reducing water pollution worldwide.
Conflicts of interest
There are no conflicts to declare.
Acknowledgements
We would like to express our gratitude to Dr Sung-Kyu Lee (E-mail: leesungkyu43@gmail.com) for providing valuable insights and consultation on the assessment of water fleas and genotoxicity of methylene blue-treated water undergoing decomposition by focused ultrasonic technology. This work was supported by the “Technical start-up corporation fostering project” through the Commercialization Promotion Agency for R&D Outcomes (COMPA) grant funded by the Korean government (MSIT) (No. 2023 Incubating 1_08) and by the Technology Innovation Program (Development of smart ultrasound equipment and application technology for the manufacture of monodisperse nanoemulsions for nanopharmaceuticals (grant number 20023461)) funded by the Ministry of Trade, Industry, and Energy (MOTIE, Korea). Furthermore, support was provided by the Korea Institute of Toxicology under grant No. 171195885.
Notes and references
- V. Şimşek, R. Z. Yarbay, V. Marttin and Ü. D. Gül, Treatment of textile dye via economic fungi/MCM-41 bio-based adsorbent: application of neural network approach, J. Clean. Prod., 2023, 421, 138448, DOI:10.1016/j.jclepro.2023.138448.
- A. Gürses, C. Doğar, M. Yalçin, M. Açikyildiz, R. Bayrak and S. Karaca, The adsorption kinetics of the cationic dye, methylene blue, onto clay, J. Hazard. Mater., 2006, 131, 217–228 CrossRef PubMed.
- S. J. Allen and B. Koumanova, Decolourisation of water/wastewater using adsorption, J. Univ. Chem. Technol. Metall., 2005, 40, 175–192 CAS.
- J. Volmajer Valh and A. M. L. Marechal, Decoloration of textile wastewater, in Dyes Pigments, New York, NY, 2009 Search PubMed.
- W. M. Fong, A. C. Affam and W. C. Chung, Synthesis of Ag/Fe/CAC for colour and COD removal from methylene blue dye wastewater, Int. J. Environ. Sci. Technol., 2020, 17, 3485–3494, DOI:10.1007/s13762-020-02720-1.
- Tech, in Organic Pollutants Ten Years After the Stockholm Convention—Environmental and Analytical Update, ed. C. Zaharia, D. Suteu and T. Puzyn, Rijeka, Shanghai, 2012, pp. 55–86 Search PubMed.
- N. Abidi, J. Duplay, A. Jada, E. Errais, M. Ghazi, K. Semhi and M. Trabelsi-Ayadi, Removal of anionic dye from textile industries' effluents by using Tunisian clays as adsorbents. Zeta potential and streaming-induced potential measurements, C. R. Chim., 2019, 22, 113–125, DOI:10.1016/j.crci.2018.10.006.
- S. Pandey, J. Y. Do, J. Kim and M. Kang, Fast and highly efficient removal of dye from aqueous solution using natural locust bean gum based hydrogels as adsorbent, Int. J. Biol. Macromol., 2020, 143, 60–75, DOI:10.1016/j.ijbiomac.2019.12.002.
- I. Khan, K. Saeed, I. Zekker, B. Zhang, A. H. Hendi, A. Ahmad, S. Ahmad, N. Zada, H. Ahmad, L. A. Shah, T. Shah and I. Khan, Review on methylene blue: its properties, uses, toxicity and photodegradation, Water, 2022, 14, 242, DOI:10.3390/w14020242.
- Z. Derakhshan, M. A. Baghapour, M. Ranjbar and M. Faramarzian, Adsorption of methylene blue dye from aqueous solutions by modified pumice stone: kinetics and equilibrium studies, Health Scope, 2013, 2, 136–144, DOI:10.17795/jhealthscope-12492.
- F. N. Allouche and N. Yassaa, Potential adsorption of methylene blue from aqueous solution using green macroalgae Posidonia oceanica, in Proceedings of the IOP Conference Series, Materials Science and Engineering, International Conference on Functional Materials and Chemical Engineering (ICFMCE 2017), Dubai, UAE, 2017, vol. 323, p. 012006 Search PubMed.
- T. H. Han, M. M. Khan, S. Kalathil, J. Lee and M. H. Cho, Simultaneous enhancement of methylene blue degradation and power generation in a microbial fuel cell by gold nanoparticles, Ind. Eng. Chem. Res., 2013, 52, 8174–8181, DOI:10.1021/ie4006244.
- L. Sun, D. Hu, Z. Zhang and X. Deng, Oxidative degradation of methylene blue via PDS-based advanced oxidation process using natural pyrite, Int. J. Environ. Res. Public Health, 2019, 16, 4773, DOI:10.3390/ijerph16234773.
- M. Contreras, C. D. Grande-Tovar, W. Vallejo and C. Chaves-López, Bio-removal of Methylene Blue from aqueous solution by Galactomyces geotrichum KL20A, Water, 2019, 11, 282, DOI:10.3390/w11020282.
- A. H. Jawad, A. S. Abdulhameed and M. S. Mastuli, Acid-factionalized biomass material for methylene blue dye removal: a comprehensive adsorption and mechanism study, J. Taibah Univ. Sci., 2020, 14, 305–313, DOI:10.1080/16583655.2020.1736767.
- L. F. Cusioli, H. B. Quesada, A. T. A. Baptista, R. G. Gomes and R. Bergamasco, Soybean hulls as a low-cost biosorbent for removal of methylene blue contaminant, Environ. Prog. Sustain. Energy, 2020, 39, e13328, DOI:10.1002/ep.13328.
- P. P. Staroń, J. Chwastowski and M. Banach, Sorption
behavior of methylene blue from aqueous solution by raphia fibers, Int. J. Environ. Sci. Technol., 2019, 16, 8449–8460, DOI:10.1007/s13762-019-02446-9.
- Y. A. R. Lebron, V. R. Moreira and L. V. de Souza Santos, Biosorption of methylene blue and eriochrome black T onto the brown macroalgae Fucus vesiculosus: equilibrium, kinetics, thermodynamics and optimization, Environ. Technol., 2021, 42, 279–297, DOI:10.1080/09593330.2019.1626914.
- M. Cheng, G. Zeng, D. Huang, C. Lai, P. Xu, C. Zhang and Y. Liu, Hydroxyl radicals based advanced oxidation processes (AOPs) for remediation of soils contaminated with organic compounds: a review, Chem. Eng. J., 2016, 284, 582–598, DOI:10.1016/j.cej.2015.09.001.
- E. M. Cuerda-Correa, M. F. Alexandre-Franco and C. Fernández-González, Advanced oxidation processes for the removal of antibiotics from water. An overview, Water, 2019, 12, 102, DOI:10.3390/w12010102.
- Y. Lee and U. von Gunten, Oxidative transformation of micropollutants during municipal wastewater treatment: Comparison of kinetic aspects of selective (chlorine, chlorine dioxide, ferrate VI, and ozone) and non-selective oxidants (hydroxyl radical), Water Res., 2010, 44, 555–566, DOI:10.1016/j.watres.2009.11.045.
- W. R. Haag and C. C. D. Yao, Rate constants for reaction of hydroxyl radicals with several drinking water contaminants, Environ. Sci. Technol., 1992, 26, 1005–1013, DOI:10.1021/es00029a021.
- A. Houas, Photocatalytic degradation pathway of methylene blue in water, Appl. Catal., B, 2001, 31, 145–157, DOI:10.1016/S0926-3373(00)00276-9.
- C. Minero, M. Lucchiari, D. Vione and V. Maurino, Fe(III)-enhanced sonochemical degradation of methylene blue in aqueous solution, Environ. Sci. Technol., 2005, 39, 8936–8942, DOI:10.1021/es050314s.
- L. Zhu, M. Hong and G. W. Ho, Fabrication of wheat grain textured TiO2/CuO composite nanofibers for enhanced solar H2 generation and degradation performance, Nano Energy, 2015, 11, 28–37, DOI:10.1016/j.nanoen.2014.09.032.
- S. Ali, S. A. Khan, I. Khan, Z. H. Yamani, M. Sohail and M. A. Morsy, Surfactant-free synthesis of ellipsoidal and spherical shaped TiO2 nanoparticles and their comparative photocatalytic studies, J. Environ. Chem. Eng., 2017, 5, 3956–3962, DOI:10.1016/j.jece.2017.07.066.
- M. Shakeel, F. Jabeen, S. Shabbir, M. S. Asghar, M. S. Khan and A. S. Chaudhry, Toxicity of nano-titanium dioxide (TiO2-NP) through various routes of exposure: a review, Biol. Trace Elem. Res., 2016, 172, 1–36, Doi: DOI:10.1007/s12011-015-0550-x, Epub 2015 November 11, http://www.ncbi.nlm.nih.gov/pubmed/26554951.
- J. Chen, X. Dong, J. Zhao and G. Tang, In vivo acute toxicity of titanium dioxide nanoparticles to mice after intraperitioneal injection, J. Appl. Toxicol., 2009, 29, 330–337, DOI:10.1002/jat.1414.
- L.-L. Guo, X.-H. Liu, D.-X. Qin, L. Gao, H.-M. Zhang, J.-Y. Liu and Y.-G. Cui, Effects of nanosized titanium dioxide on the reproductive system of male mice, Zhonghua Nan ke Xue, 2009, 15, 517–522 CAS.
- N. Li, Y. Duan, M. Hong, L. Zheng, M. Fei, X. Zhao, J. Wang, Y. Cui, H. Liu and J. Cai, Spleen injury and apoptotic pathway in mice caused by titanium dioxide nanoparticles, Toxicol. Lett., 2010, 195, 161–168, DOI:10.1016/j.toxlet.2010.03.1116.
- E.-Y. Moon, G.-H. Yi, J.-S. Kang, J.-S. Lim, H.-M. Kim and S. Pyo, An increase in mouse tumor growth by an in vivo immunomodulating effect of titanium dioxide nanoparticles, J. Immunot., 2011, 8, 56–67, DOI:10.3109/1547691X.2010.543995.
- C. Moon, H.-J. Park, Y.-H. Choi, E.-M. Park, V. Castranova and J. L. Kang, Pulmonary inflammation after intraperitoneal administration of ultrafine titanium dioxide (TiO2) at rest or in lungs primed with lipopolysaccharide, J. Toxicol. Environ.
Health, Part A, 2010, 73, 396–409, DOI:10.1080/15287390903486543.
- J. A. Shin, E. J. Lee, S. M. Seo, H. S. Kim, J. L. Kang and E. M. Park, Nanosized titanium dioxide enhanced inflammatory responses in the septic brain of mouse, Neuroscience, 2010, 165, 445–454, DOI:10.1016/j.neuroscience.2009.10.057.
- S. Alarifi, D. Ali, A. A. Al-Doaiss, B. A. Ali, M. Ahmed and A. A. Al-Khedhairy, Histologic and apoptotic changes induced by titanium dioxide nanoparticles in the livers of rats, Int. J. Nanomed., 2013, 8, 3937–3943, DOI:10.2147/IJN.S47174.
- J. Wang, Y. Nie, H. Dai, M. Wang, L. Cheng, Z. Yang, S. Chen, G. Zhao, L. Wu, S. Guang and A. Xu, Parental exposure to TiO2 NPs promotes the multigenerational reproductive toxicity of Cd in Caenorhabditis elegans via bioaccumulation of Cd in germ cells, Environ. Sci.: Nano, 2019, 6, 1332–1342 RSC.
- O. S. Bayomie, H. Kandeel, T. Shoeib, H. Yang, N. Youssef and M. M. H. El-Sayed, Novel approach for effective removal of methylene blue dye from water using fava bean peel waste, Sci. Rep., 2020, 10, 7824, DOI:10.1038/s41598-020-64727-5.
- Ü. Geçgel, G. Özcan and G. Ç. Gürpınar, Removal of methylene blue from aqueous solution by activated carbon prepared from pea shells (Pisum sativum), J. Chem., 2013, 2013, 1–9 CrossRef.
- E. A. Serna-Galvis, J. Porras and R. A. Torres-Palma, A critical review on the sonochemical degradation of organic pollutants in urine, seawater, and mineral water, Ultrason. Sonochem., 2022, 82, 105861, DOI:10.1016/j.ultsonch.2021.105861.
- P. Liu, Z. Wu, A. V. Abramova and G. Cravotto, Sonochemical processes for the degradation of antibiotics in aqueous solutions: a review, Ultrason. Sonochem., 2021, 74, 105566, DOI:10.1016/j.ultsonch.2021.105566.
- E. Calcio Gaudino, E. Canova, P. Liu, Z. Wu and G. Cravotto, Degradation of antibiotics in wastewater: new advances in cavitational treatments, Molecules, 2021, 26, 617, DOI:10.3390/molecules26030617.
- J. Wang, Z. Wang, C. L. Z. Vieira, J. M. Wolfson, G. Pingtian and S. Huang, Review on the treatment of organic pollutants in water by ultrasonic technology, Ultrason. Sonochem., 2019, 55, 273–278, DOI:10.1016/j.ultsonch.2019.01.017.
- Y. Deng and R. Zhao, Advanced oxidation processes (AOPs) in wastewater treatment, Curr. Pollut. Rep., 2015, 1, 167–176, DOI:10.1007/s40726-015-0015-z.
- P. Cintas and J.-L. Luche, Green chemistry, Green Chem., 1999, 1, 115–125, 10.1039/A900593E.
- R. J. Wood, J. Lee and M. J. Bussemaker, A parametric review of sonochemistry: control and augmentation of sonochemical activity in aqueous solutions, Ultrason. Sonochem., 2017, 38, 351–370, DOI:10.1016/j.ultsonch.2017.03.030.
- N. Tran, P. Drogui and S. K. Brar, Sonochemical techniques to degrade pharmaceutical organic pollutants, Environ. Chem. Lett., 2015, 13, 251–268, DOI:10.1007/s10311-015-0512-8.
- K. Lee, Ultrasound. Its Chemical, Physical, and Biological Effects, ed. K. S. Suslick, VCH, New York, vol. 1988 xiv, p. 336, illus. $65, Science, 1989, 243, p. 1499. doi: DOI:10.1126/science.243.4897.1499-a, http://www.ncbi.nlm.nih.gov/pubmed/17839755.
- Current Trends in Sonochemistry, ed. G. J. Price, The Royal Society of Chemistry, Cambridge, UK, 1992 Search PubMed.
- A. Weissler, Advances in sonochemistry, volume I, J. Acoust. Soc. Am., 1994, 96, 613, DOI:10.1121/1.410458.
- S.-Y. Kim, T.-G. Lee, S.-A. Hwangbo and J.-R. Jeong, Effect of the TiO2 colloidal size distribution on the degradation of methylene blue, Nanomaterials, 2023, 13, 302, DOI:10.3390/nano13020302.
- S. A. Hwangbo, M. Kwak, J. Kim and T. G. Lee, Novel surfactant-free water dispersion technique of TiO2 NPs using focused ultrasound system, Nanomaterials, 2021, 11, 427, DOI:10.3390/nano11020427.
- A. Mohamed, R. El-Sayed, T. A. Osman, M. S. Toprak, M. Muhammed and A. Uheida, Composite nanofibers for highly efficient photocatalytic degradation of organic dyes from contaminated water, Environ. Res., 2016, 145, 18–25, DOI:10.1016/j.envres.2015.09.024.
- A. Magdy, E. Sadaka, N. Hanafy, M. A. El-Magd, N. Allahloubi and M. El Kemary, Green tea ameliorates the side effects of the silver nanoparticles treatment of Ehrlich ascites tumor in mice, Mol. Cell. Toxicol., 2020, 16, 271–282, DOI:10.1007/s13273-020-00078-6.
- S.-M. Bak, S.-M. Back, D. Y. Kim, N.-Y. Jeung, N.-Y. Kim, J.-Y. Yang, K.-H. Han, Y.-B. Kim, B. S. Min, B.-S. Lee and S.-H. Park, Genotoxicity assessment of root extracts of Paeonia lactiflora Pall, Mutat. Res., Genet. Toxicol. Environ. Mutagen., 2023, 886, 503579, DOI:10.1016/j.mrgentox.2022.503579.
- S. Koda, T. Kimura, T. Kondo and H. Mitome, A standard method to calibrate sonochemical efficiency of an individual reaction system, Ultrason. Sonochem., 2003, 10, 149–156, DOI:10.1016/S1350-4177(03)00084-1.
|
This journal is © The Royal Society of Chemistry 2024 |