DOI:
10.1039/D4RA01631A
(Paper)
RSC Adv., 2024,
14, 22470-22479
Food wastewater treatment using a hybrid biofilm reactor: nutrient removal performance and functional microorganisms on filler biofilm and suspended sludge†
Received
5th March 2024
, Accepted 2nd July 2024
First published on 16th July 2024
Abstract
In this study, a laboratory-scale hybrid biofilm reactor (HBR) was constructed to treat food wastewater (FWW) before it is discharged into the sewer. The chemical oxygen demand (COD) of 29
860 mg L−1 in FWW was degraded to 200–350 mg L−1 using the HBR under the operating parameters of COD load 1.68 kg m−3 d−1, hydraulic retention time (HRT) of 426.63 h, dissolved oxygen (DO) of 8–9 mg L−1, and temperature of 22–23 °C. The biomass of biofilm on the surface of filler was 2.64 g L−1 for column A and 0.91 g L−1 for column O. Microbial analysis revealed richer and more diverse microorganisms in filler biofilms compared to those in suspended sludge. The hybrid filler was conducive to the development of functional microbial species, including phyla Firmicutes, Actinobacteriota, and Chloroflexi, and genus level norank_f_JG30-KF-CM45, which will improve FWW treatment efficiency. Moreover, the microorganisms on the filler biofilm had more connections and relationships than those in the suspended sludge. The combination of an up-flow anaerobic sludge bed (UASB) and HBR was demonstrated to be an economical strategy for practical applications as a shorter HRT of 118.34 h could be obtained. Overall, this study provides reliable data and a theoretical basis for the application of HBR and FWW treatments.
1. Introduction
Food wastewater (FWW) is ubiquitous during the washing, compression, storage, and disposal of food waste.1 Organic matter in the FWW can be recycled via anaerobic digestion to produce biogas.2 However, owing to the complex anaerobic digestion process and the low price of natural gas, biogas production from FWW is not economically favorable.3 Hence, many factories discharge generated FWW into sewers. However, certain countries impose restrictions on the discharge concentration of chemical oxygen demand (COD) in FWW. For example, the maximum discharge concentration of COD regulated by the integrated wastewater discharge standard in China is 500 mg L−1. Reducing the COD concentration in FWW before it is discharged into the sewer is necessary.
FWW contains high levels of organic matter, nitrogen, and phosphorus, although there are few refractory substances.4 Therefore, FWW has good biodegradability and is suitable for biological treatment. Biological treatment technologies include activated sludge and biofilm processes. The conventional activated sludge process is not suitable for decentralized FWW treatment because of its complex process conditions and large surface area.5 Biofilm systems feature a small area and short process flow. However, challenges include low load impact resistance, unstable treatment effect, difficulty in control, and high operation cost.6,7
Hybrid biofilm reactors (HBR) integrate activated sludge and biofilm processes by adding biological fillers to the activated sludge reaction tank. The two processes work together to remove pollutants from wastewater.8–10 On the one hand, HBR has the characteristics of an activated sludge process with sufficient solid–liquid contact, high removal efficiency of organic pollutants, and stable quality of effluent.11 On the other hand, the introduction of lightweight large porosity suspended fillers results in several advantages. Firstly, the high fill rate of fillers (60% to 90%) to form a fixed bed allows the system to cope with a high load influent, which significantly shortens the hydraulic retention time (HRT) and thus reduces the size and footprint of the reactor and construction costs.9 Secondly, the fixed bed fillers can retain a large amount of suspended solids, without the need to include sedimentation tanks. The retained pollutants and excess biofilm can be discharged from the reactor through the aeration backwash.12 The large porosity of the fillers provides sufficient space for biofilm growth, thus increasing the biomass, improving the pollutant removal capacity, fully meeting the needs of nitrifying bacteria, and reducing the high correlation between nitrogen removal efficiency and sludge age. These attributes improve the efficacy of the system in denitrifying and removing phosphorus.10,13,14 Once again, the fixed bed allows the formation of a higher pollutant concentration gradient within the reactor and, at the same time, a longer oxygen traveling path, high oxygen utilization, and low energy consumption, as no packing fluidization is required.14,15 Therefore, the HBR process offers the advantages of both suspended sludge and biofilm processes. However, to the best of our knowledge, few studies have reported on the treatment of FWW using HBR technology.
In this study, a laboratory-scale HBR was constructed. Removal of nutrients and the microbial response of the HBR under different influent loads were investigated through continuous long-term experiments. The findings provide reliable data support and a theoretical basis for the application of HBR and the treatment of FWW.
2. Materials and methods
2.1. Experimental materials
The food waste treatment machine that was used was fed 30 kg of restaurant food waste every day and generated 500 L of FWW. The FWW characteristics and discharge standards (regulated by the integrated wastewater discharge standard of China, GB 8978-1996) are shown in Table S1.†
2.2. Experimental setup and operations
As shown in Fig. 1, the HBR consisted of an anoxic (A) column and an aerobic (O) column. Both columns had an internal diameter of 0.1 m. The effective height was 0.5 m for column A and 1.0 m for column O. Column O was continuously aerated; as a result, its dissolved oxygen (DO) did not fall below 4. Aeration backflushing was performed once a day for 5 min in column A. Columns A and O was filled with a lightweight type C4 filler with a filling ratio of 70%. In the procedure, FWW first enters the bottom of column A via a peristaltic pump and then flows by gravity from the top of column A to the bottom of column O (the level of column A is approximately 0.5 m above column O). The reflux ratio from column O to column A is 200%. The effluent is discharged into the municipal sewer from the top of column O.
 |
| Fig. 1 Hybrid biofilm reactor. | |
The experimental phases and operating parameters are listed in Table 1. In start-up I, FWW diluted by 3 times is fed into the HBR systems to start the reactor and allow the formation of biofilms on the surface of the fillers. Subsequently, in start-up II, the inflow of diluted FWW is increased from 0.7344 to 1.8000 L d−1 to domesticate the microorganisms. In phase I, undiluted FWW was pumped into the reactor to investigate the treatment effects. In phase II, the inflow was decreased and two-thirds of the mixture in the reactor was replaced with tap water to quickly reduce the load of the HBR system and investigate suitable operating conditions for FWW treatment.
Table 1 Experimental phase and operating parameters
Experimental phase |
Influent |
Inflow quantity (L d−1) |
COD concentration (mg L−1) |
COD load (kg COD per m3 per d) |
HRT (h) |
Start-up I |
FWW diluted 3 times |
0.7344 |
9953 ± 116 |
0.62 |
384.80 |
Start-up II |
FWW diluted 3 times |
1.8000 |
9953 ± 116 |
1.52 |
157.00 |
Phase I |
FWW |
1.0512 |
29 860 ± 276 |
2.67 |
268.84 |
Phase II |
FWW |
0.6624 |
29 860 ± 276 |
1.68 |
426.63 |
2.3. Determination of microbial activity
The nitrification and denitrification activities of suspended sludge and biofilms on fillers in phase II were measured using the jar test. Specifically, a 500 mL mixture with a filler filling rate of 70% was collected from the HBR system and separated. The fillers were separated from the sludge mixture, and the residual impurities were cleaned. For suspended sludge, the sludge mixture was centrifuged, suspended in deionized water, and centrifuged again. This was repeated three times to remove residual pollutants from the suspended sludge. A 500 mL Erlenmeyer flask was used as the reaction vessel. When testing the nitrification activity, the initial concentration of ammonia nitrogen was 20 mg L−1, and 40 mg L−1 of sodium bicarbonate was added to provide alkalinity. The reaction mixture was continuously aerated to maintain the DO concentration of approximately 8 mg L−1. When the denitrification activity was tested, the initial concentration of nitrate nitrogen (NO3−–N) was 40 mg L−1 and the initial COD concentration was 240 mg L−1. Before the reaction, the reaction solution was aerated with argon to remove DO. During the reaction, the flask was sealed to maintain an anaerobic environment. Samples were collected every 10 min to determine the COD, NH4+–N, NO3−–N, and nitrite nitrogen (NO2−–N) concentrations until they no longer changed.
2.4. DNA extraction and high-throughput sequencing
Suspended sludge and biofilm samples on the filler at the end of phase I (day 74) and phase II (day 120) were collected for microbial sequencing analysis. Three parallel acquisitions of each sample were tested in triplicate to reduce testing errors. Biofilm samples were collected by the ultrasonication of fillers at 4 °C for 15 min. The suspended sludge and peeled biofilm samples were washed thrice with sterile water, centrifuged, and freeze-dried for DNA extraction. DNA extraction, PCR amplification and sequencing, quality control of raw data, and operational taxonomic unit (OTU) analysis were based on our previous study.4
2.5. Analytical methods
The parameters of water quality including COD, NH4+–N, NO3−–N, NO2−–N, and TN were measured according to standard method.16 The conventional indexes including DO and temperature were tested using a multifunctional water-quality device (Multi 3620 IDS, WTW, Germany). The determination of suspended sludge concentration and biofilm mass is carried out using the weighing method, the peeling of biofilm on the fillers is achieved through the combined action of hot alkali and ultrasound as detailed in Methods S1.†
2.6. Statistical analyses
The experimental data were processed and visualized using Origin 2020 and the R language tool (version 4.2.2). The alpha diversity differences in the microbial community structure were compared using Welch's t-test and Wilcoxon rank-sum test for data that did not satisfy normality and homogeneity. Principal component analysis (PCA) at the OTU level was performed using the analysis of similarities (ANOSIM) inter group difference test method. Permutational multivariate analysis of variance (PERMANOVA) was performed to test the significance of the PCA results. Differences in phylum and genus-level microbial community composition were analyzed using the Wilcoxon rank-sum test with a confidence level of 95%. Microbial network analysis was performed using molecular ecological networks based on the random matrix theory method.17 Significant correlations among the OTUs (R > 0.7 or ≤0.7, P < 0.01) were defined based on Spearman's correlation coefficients. The networks were visualized using Gephi software (version 0.9.7) and the topological parameters of the networks were calculated using the R package igraph.
3. Results
3.1. Removal of nutrients of FWW by HBR
3.1.1. Nutrients removal. As shown in Fig. 2, the HBR was fed with 3 times diluted FWW at a low flow rate during the start-up I (day 1–13). After 13 days of operation, the COD concentration of the HBR effluent was stable below 200 mg L−1 (Fig. 2c), and the concentration of TN in the effluent was approximately 50 mg L−1 (Fig. 2d). The HBR had a significant pollutant removal effect, which indicated a successful start-up. In start-up II (day 14–26), the inflow quantity was increased to 1.8 L d−1, aiming to cultivate and domesticate the microorganisms in the HBR system. After 13 days of domestication, the system showed significant degradation of FWW and the effluent COD concentration was below 500 mg L−1 (Fig. 2c), which is below the limit of effluent discharge standard. Therefore, the HBR was considered successfully started during the start-up phase.
 |
| Fig. 2 (a) COD load and HRT at different experimental phases, (b) temporal trends in DO and temperature; nutrient-removal profiles of (c) COD, (d) TN, (e) NH4+–N, (f) NO3−–N and NO2−–N. (The cylindrical bar represents the effluent concentration, and the hollow circle represents the removal rate.) | |
In phase I (day 26–74), undiluted FWW was pumped into the HBR at a loading of 2.66 kg COD per m3 per d to test the performance of the HBR in treating high concentrations FWW. After a long period of continuous operation, it was found that the effluent COD concentration was 900–1400 mg L−1 at DO concentrations of 4–6 mg L−1 (Fig. 2c), which was higher than the effluent discharge standard limit of 500 mg L−1 for COD. The effluent NH4+–N concentration was 14.21–148.42 mg L−1 at a load of 0.12 kg m−3 d−1 (Fig. 2e). Unexpectedly, the effluent NO3−–N concentration was very low, less than 2 mg L−1 for most of the time, and it was only when the aeration disc was clogged resulting in low DO that the effluent NO3−–N concentration was high, with a maximum of 29.23 mg L−1 (Fig. 2f). Thus, it is evident that the O column of HBR undergoes simultaneous nitrification and denitrification in phase I. It has been reported that due to the structural characteristics of the biofilm in HBR and the limitation of oxygen diffusion, the biofilm on the surface of the fillers can form aerobic–anoxic–anaerobic zone from outside to inside. In addition, the detachment of biofilm can allow some microorganisms in the anaerobic zone to enter the aerobic environment of suspended activated sludge, and the attachment of biofilm can also allow some microorganisms in the aerobic environment to enter the anoxic/anaerobic environment.18–20 Therefore, HBR has the advantages of both microbial suspension and attached growth, providing a suitable environment for various microorganisms associated with nitrogen removal.
In phase II (day 75–120), the inflow quantity was decreased with a COD load of 1.68 kg m−3 d−1 to examine the suitable working conditions of the HBR for FWW treatment (Fig. 2a). As the HRT increased (426.63 h) due to the lower FWW intake, two-thirds of the reaction solution of the HBR was replaced with dechlorinated tap water to enable a rapid reduction of the reaction solution concentration (COD concentration of the O-column was diluted to 623 mg L−1), and thus the treatment capacity of the HBR for FWW at lower loads was examined as soon as possible. During the continuous operation of phase II, the effluent COD concentration was basically in the range of 200–350 mg L−1 (Fig. 2c), and stably lower than 500 mg L−1. The higher than 500 mg L−1 on days 85–91 was due to the clogging of the tube from column A to column O on day 84, resulting in the accumulation of solution at the top of column A. After dredging, the accumulated solution entered into the column O at one time, resulting in the increase of the effluent COD concentration. Inconsistently with phase I, the effluent NH4+–N in phase II was approximately 12–20 mg L−1 (Fig. 2e), the effluent NO2−–N concentration was stable below 1 mg L−1 (Fig. 2f), and the effluent NO3−–N concentration was increasing and gradually stabilized around 200 mg L−1 (Fig. 2f), which indicated that the nitrification of the column O in phase II was significant, and the simultaneous nitrification and denitrification were weakened. The possible reason for this phenomenon is that the DO concentration was unstable in phase I due to the increased viscosity of the reaction solution caused by long HRT,21 which promoted the occurrence of simultaneous nitrification and denitrification;22,23 while in phase II, after diluting the reaction solution and reducing the FWW intake, the aeration effect was good, and the DO concentration was stably maintained at 8–9 mg L−1, this ensures excellent nitrification effect.
3.1.2. Fillers biofilm. The status of the fillers at each phase of HBR is shown in Fig. S1.† fillers in column A showed black colour due to anoxia, while that in column O showed earthy yellow colour. At the end of phase II, the biomass of biofilm on the surface of fillers was measured: 37.73 mg g−1 (2.64 g L−1) for column A and 12.96 mg g−1 (0.91 g L−1) for column O. The biomass of biofilm in column A was almost three times higher than that in column O. This is due to the fact that the fillers in column A was in a fixed-bed state without aeration, which is favourable for the growth of the microorganisms attached to the fillers. The fillers in column O is continuously under aeration, the flow of the reaction solution and the mutual collision between the fillers were not conducive to the attachment and growth of microorganisms on the surface of the fillers.
3.1.3. Activity of fillers biofilms and suspended sludge. As shown in Fig. S2a,† the denitrification effect of the biofilms that formed on the fillers in column A was poor; the NO3−–N concentration showed a regular decreasing trend in the first 30 min and then no longer decreased, indicating that the contribution of the fillers biofilm in column A to the removal of NO3−–N in the HBR was limited. The denitrification effect of the suspended sludge in column A was evident and 40 mg L−1 of NO3−–N was completely removed within 150 min. The finding indicates that the removal of NO3−–N (approximately 220 mg L−1) from the O-column reflux solution by column A of the HBR was mainly performed using the suspended sludge in phase II. Because aerobic denitrification simultaneously occurred in column O in phase III, the denitrification activities of filler biofilms and suspended sludge in column O were examined. These denitrification effects were poor in phase II (Fig. S2b†).The nitrification activities of the filler biofilms and suspended sludge are shown in Fig. S2c.† Biofilm microorganisms on the fillers degraded 20 mg L−1 of NH4+–N to 10.35 mg L−1 in 90 min, representing a removal rate of 48.25%. The suspended sludge could only degrade to 16.05 mg L−1 in 90 min, representing a removal rate of 24.75%. Both the biofilms of the suspended sludge participated in the removal of NH4+–N; the removal by biofilm microorganisms was approximately twice that of the suspended sludge. The oxygen consumption rates of column O biofilms and suspended sludge were determined; the oxygen consumption rate of biofilms and suspended sludge was comparable (0.00196 versus 0.00194 mg O2 per L per s, respectively; Fig. S2d†).
3.2. Microbial analysis of the HBR system
3.2.1. Microbial community structure. 16S rRNA high-throughput sequencing was performed to explore the microbial community structure and diversity of filler biofilms and suspended sludge in the HBR system. The effective sequences were divided into 3412 OTUs with 97% similarity. A Venn diagram was drawn to show the number of shared and unique OTUs in different samples (Fig. 3a). The species number of OTU levels in the filler biofilm were higher than those in the suspended sludge. In addition, filler biofilms displayed greater diversity and richness (Table S2†) than suspended sludge in columns A and O (Fig. 3b and c). These results indicate that the hybrid biofilm is beneficial for microbial colonization, which can enrich the species number and diversity of microorganisms in the HBR system, which is consistent with previous research results.10,24,25 PCA and PERMANOVA further indicated a significant difference (P < 0.01) in the microbial community between the filler biofilm and suspended sludge (Fig. 3d).
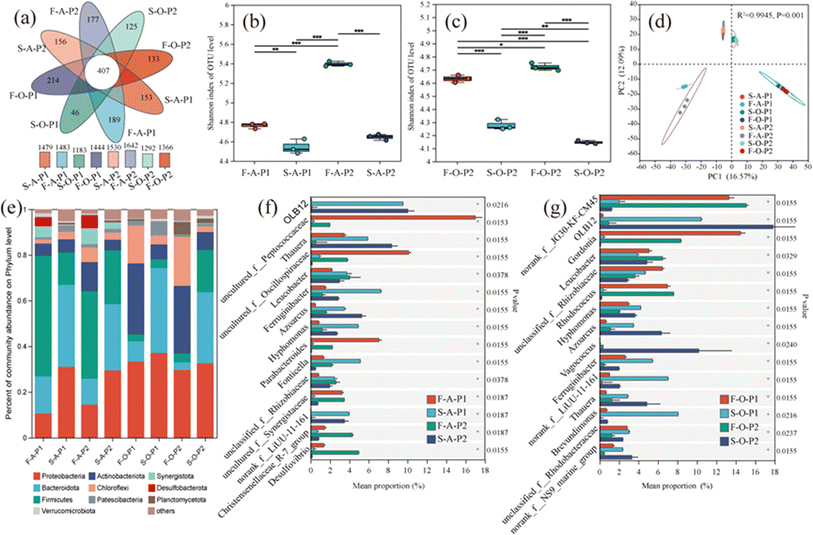 |
| Fig. 3 Microbial community composition and diversity: (a) Venn diagram shows the number of OTUs shared and unique in different samples; alpha diversity (Shannon index) of column A (b) and column O (c); PCA analysis (d); microbial community composition on phylum level (e), and difference analysis of species on genus level of column A (f) and column O (g). (*P < 0.05, **P < 0.01, ***P < 0.001). | |
The composition of the microbial community at the phylum level is shown in Fig. 3e. The main phyla were Proteobacteria, Bacteroidetes, Firmicutes, Actinobacteria, and Chloroflexi. These phyla are commonly detected in wastewater treatment reactors.10 The filler biofilms and suspended sludge harbored similar microbial phyla; however, the relative abundances of the phyla differed significantly between biofilms and suspended sludge, and between columns A and O (Fig. 3e). Firmicutes and Synergistota were more abundant in filler biofilms than in suspended sludge in column A. The opposite was observed in column O. The abundances of Proteobacteria and Bacteroidetes in the suspended sludge were higher than those in the filler biofilms. The abundance of Actinobacteria and Chloroflexi increased in phase II and was more pronounced in the filler biofilms. In addition, at the genus level, microbial communities in filler biofilms and suspended sludge differed significantly (P < 0.05) in columns A (Fig. 3f) and O (Fig. 3g) in the two phases. The main genera identified were OLB12, norank_f__JG30-KF-CM45, Leucobacter, Thauera, unclassified_f__Rhizobiaceae, Gordonia, Ferruginibacter, Hyphomonas, Azoarcus, and uncultured_f__Reptococcaceae. OLB12 was the dominant genus in the suspended sludge samples, whereas norank_f__JG30-KF-CM45 and Gordonia were the dominant genera in filler biofilms of column O samples. Uncultured_f__Reptococcaceae were prevalent in the fillers of column A during phase I.
3.2.2. Microbial degradation pathways of nutrients in FWW. PICRUSt2 prediction was used to analyze the microbial metabolism pathways and gene functions.26 Fig. 4a shows the abundance of microbial metabolism pathways in level 2 based on PICRUSt2 prediction. It can be seen that the metabolism is the main pathways in level 2, followed by the environmental information processing, genetic information processing, and cellular processes. Most of the metabolism pathways were enhanced in the fillers of column O in both phase I and phase II, such as cellular community – prokaryotes, membrane transport, metabolism of terpenoids and polyketides, metabolism of other amino acids, xenobiotics biodegradation and metabolism, lipid metabolism, metabolism of cofactors and vitamins, energy metabolism, amino acid metabolism, carbohydrate metabolism, global and overview maps. This result indicates that the fillers in column O undertakes greater roles in various metabolic pathways, which is due to hybrid fillers is beneficial to microbial colonization.10 The abundance heatmap of carbohydrate metabolic pathways is shown in Fig. 4b, the abundance of carbohydrate metabolism genes is provided in Table S3.† Pyruvate metabolism, glyoxylate and dicarboxylate metabolism, glycolysis/gluconeogenesis, and propanoate metabolism are the main carbohydrate metabolic pathways.27 The abundance of carbohydrate metabolism genes in column O is higher than that in column A. In column A, the abundance of carbohydrate metabolism genes in the sludge is higher than that in the fillers, while in column O, the abundance of carbohydrate metabolism genes in the sludge is higher than that in the fillers. On the whole, the fillers in column O expressed more carbohydrate metabolism genes in two phases, this result is consistent with the result of oxygen consumption rate (Fig. S2d†), which indicates that the fillers in column O have a higher metabolic activity, thus promotes the degradation of organic compounds in FWW (Fig. S3c†). The nitrogen metabolism process is depicted in Fig. 4c, the abundance of nitrogen metabolism genes in the HBR system is exhibited in Table S4.† The abundance of nitrification genes in the fillers is higher that in the sludge, especially in the fillers of column O, this is because the fillers provide a better environment for colonization of nitrifying bacteria.10 For denitrification genes, the abundance in column O is higher than column A, especially in the fillers of column O, such as narG, narZ, nxrA (Table S4†). Therefore, the simultaneous nitrification and denitrification effect in column O was mainly conducted by the fillers in the HBR system.23 These results indicates that the microorganisms on the fillers biofilm plays a crucial role in the degradation of nutrients from FWW.
 |
| Fig. 4 (a) The abundance of microbial metabolism pathways in level 2 based on PICRUSt2 analysis, (b) the abundance heatmap of carbohydrate metabolism genes in HBR system, and (c) nitrogen metabolism process in HBR system. | |
3.2.3. Microbial network structure in HBR system. In order to reflect the interactions between species, the microbial network structure was analyzed based on the correlation between top 100 genera (Fig. 5). It was observed that most of the nodes in column A belonging to Firmicutes, and Proteobacteria occupies a dominant position in column O, this is consistent with the phylum level community composition (Fig. 3e). The network analysis topology features between different groups are summarized in Table S5.† The number of nodes and edges in the fillers is greater than that in the sludge, this indicates that fillers cause more associations between microbial species. Previous studies have also confirmed that bacteria on the fillers biofilm interact more than those on the suspended sludge.10 In addition, the positive links in the fillers is greater than that in the sludge. Positive links are generally associated with the cross-feeding, co-colonization, or niche overlap.28 Therefore, the involvement of hybrid fillers in activated sludge process might have stimulated cross-feeding or co-colonization between species in the microbial networks. The modularity index for fillers samples is also greater than that of the sludge samples, suggesting that the networks of fillers microorganisms have better modular structures. On the whole, the microorganisms on the fillers biofilm have more connections and relationships between them than those on the suspended sludge. Therefore, the HBR process has advantages in nutrients removal from FWW compared to single activated sludge process.
 |
| Fig. 5 Microbial networks in HBR system. The size of each node is proportional to their abundance in the microbial community, the color of each node is determined by the phylum they belong to. Red edges indicate positive relationships between two individual nodes, while green edges indicate negative relationships. | |
4. Discussion
4.1. Performance of HBR on COD degradation in FWW
The COD removal performance of the HBR under different operating conditions is summarized in Table 2. A comparison of the COD removal in the FWW between the HBR and other biological treatment processes is represented in Table S6.† In condition 3, the COD concentration in FWW was reduced from 19
860 to <1400 mg L−1, with a removal rate of 96.7–97.3%. Compared to the results of Xiao et al.,29 the HBR is economical because it achieves similar effluent concentrations and removal rates at lower temperatures (Table S6†). In terms of the performance of various processes, HBR displays higher COD removal at higher influent COD concentrations than MBR,30 anaerobic membrane reactors,21 and anaerobic dynamic membrane reactors.31 Under condition 4, the effluent COD concentration was as low as 194–458 mg L−1 although the HRT was 426.63 h (discussed later).
Table 2 The treatment effect of HBR on COD removal in FWW under different operating conditions
Conditions |
Inlet concentration (mg L−1) |
Inflow (L d−1) |
COD load (kg m−3 d−1) |
HRT (h) |
DO (mg L−1) |
Temperature (°C) |
Effluent concentration (mg L−1) |
Removal rate (%) |
1 |
9953 ± 116 |
0.7344 |
0.62 |
384.80 |
4–7 |
19–21 |
150–200 |
98.5–99.4 |
2 |
9953 ± 116 |
1.800 |
1.52 |
157.00 |
2.5–7 |
17.5–18.5 |
480–800 |
92.5–95.3 |
3 |
29 860 ± 276 |
1.0512 |
2.67 |
268.84 |
4–6 |
22–23 |
900–1400 |
96.7–97.3 |
4 |
29 860 ± 276 |
0.6624 |
1.68 |
426.63 |
8–9 |
22–23 |
194–458 |
96.6–98.2 |
4.2. Roles of columns A and O in the HBR system
In phase I, the concentration of NO3−–N in the influent of column A (effluent of column O) was 0.55 to 5.92 mg L−1 (Fig. 2f). The limited NO3−–N was removed by column A; that is, the amount of COD degraded by denitrification was limited. However, column A contributed 12.2% of the total COD removal as calculated from the percentage contribution of the two columns to the total COD removal (Table S7†). Therefore, the role of column A in phase I may be to degrade the COD through anaerobic digestion.32 During phase II, the NO3−–N concentration in the influent of column A (the effluent of column O) continuously increased to 220 mg L−1 (Fig. 2f). However, the NO3−–N concentration in the effluent of column A was lower than 2 mg L−1 (Fig. S3e†); therefore, significant denitrification occurred in column A, as confirmed by the previous study,14 which consumed a portion of COD in FWW. In summary, at higher COD loads (phase I), column A facilitates the removal of part of the COD by anaerobic digestion. If there is a demand for TN removal from FWW, the HBR can be operated at a lower COD load, such as the conditions in phase II.
The operational status of column O is shown in Fig. S3.† The main role of column O was to degrade COD in the FWW. Column O contributed 87.8% of the total COD removal during phase I. In phase II, approximately 12
000 mg L−1 of COD in the influent (effluent of column A) was reduced to approximately 350 mg L−1 at a volumetric loading of 2.52 kg COD per m3 per d, and HRT of 284.42 h, with a removal rate of approximately 97% (Fig. S3c†). In phase I, the TN in the influent was degraded to <50 mg L−1 by simultaneous nitrification and denitrification, with a removal rate of approximately 90% (Fig. 2d). In phase II, simultaneous denitrification was weakened due to the increase in DO (Fig. 2b),22,23 and approximately 850 mg L−1 of NH4+–N in the influent was converted to approximately 220 mg L−1 of NO3−–N by nitrification, which was removed from the HBR system by denitrification after refluxing to column A. The findings demonstrate the crucial role of column O in the removal of COD and TN from FWW.
4.3. Functional microorganisms in filler biofilms and suspended sludge
Proteobacteria, Bacteroidetes, Firmicutes, and Actinobacteria were the predominant phyla in the HBR system (Fig. 3e). It is generally agreed that Proteobacteria and Firmicutes play crucial roles in carbon metabolism and nitrogen cycle,33 Bacteroidota is active in nitrogen mineralization.34 Actinobacteria are usually associated with reduced NO3−–N levels.33 At the genus level, the dominant genus detected in the sludge included OLB12 and Thauera. OLB12 is simultaneously capable of nitrification-denitrification and phosphorus removal.35,36 Thauera is an aerobic denitrifier.37 These facts explain the simultaneous nitrification and denitrification in column O of the HBR in phase I. OLB12 was less abundant in the filler biofilms than in suspended sludge, which differs from the results of the aerobic moving bed biofilm reactor system.38 The dominant genera in the fillers of column O, norank_f_JG30-KF-CM45, are associated with COD degradation39 and denitrification.40 In the current study, the functional microorganisms in the filler biofilms of column O played greater roles in various metabolic pathways, especially carbohydrate and nitrogen metabolism. Hybrid fillers may form a micro-ecological environment that contributes to the development of different microbial species, thus improving FWW treatment efficiency.41 Therefore, the significant reduction in organic matter and nitrogen in the FWW by the HBR system is mainly due to the involvement of hybrid fillers, which are beneficial for microbial colonization.10 Overall, HBR provides a more efficient and economical solution for treatment of highly concentrated FWW than conventional biological treatment technologies such as membrane bioreactors.21
4.4. Prospects for practical applications
In this study, the HRT of the HBR was 296.14 h in phase I and 426.63 h in phase II. A long HRT is required for extensive COD removal from wastewater.42,43 However, a shorter HRT is expected because it is related to the footprint of the device. An anaerobic reactor is economical and effective for COD removal.44 Hence, an anaerobic reactor can be combined with HBR considering that column A contributed approximately 12% of COD removal at the anaerobic condition in phase I (Table S7†). The up-flow anaerobic sludge blanket (UASB) is the most commonly used technology for the anaerobic treatment of organic wastewater (Table S8†).45 The UASB reactor can be set up before the HBR to remove a portion of the COD in the FWW through anaerobic digestion. As shown in Table S9,† the organic volumetric loading rate of the UASB can be up to 2.5–13.6 kg COD per m3 per d at 25 °C, which is much higher than that of the activated sludge or biofilm processes. The combination of UASB and HBR is an economical strategy for industrial applications.
A flowchart of the UASB-HBR process and the envisioned performance of the combined UASB and HBR process for treating FWW are shown in Fig. S4.† The determined COD concentration of FWW after degreasing was 30
000 mg L−1, the COD removal rate of UASB was 85%, and the volumetric load of COD removal was 10 kg COD per m3 per d. Hence the HRT of UASB was approximately 61.2 h, and the concentration of COD in the effluent was approximately 4500 mg L−1. The effluent from the UASB entered the HBR; therefore, the COD removal efficiency could be calculated according to condition 4 in Table 2. The calculated volumetric load of COD removal was 1.68 kg COD per m3 per d, COD removal rate was 97%, HRT was 57.14 h, and the effluent COD concentration was <500 mg L−1. Therefore, a high concentration of FWW can be degraded to meet the discharge standard in 118.34 h by the combined UASB-HBR process.
5. Conclusion
An HBR was constructed for the treatment of highly concentrated FWW. The FWW COD of 29
860 mg L−1 was degraded to 200–350 mg L−1 by HBR under the operating parameters of the COD load of 1.68 kg m−3 d−1, HRT 426.63 h, DO 8–9 mg L−1, and temperature 22–23 °C. Column A of the HBR system removed 12.2% of the COD by anaerobic digestion and denitrification, and simultaneous nitrification-denitrification was observed in column O. The microorganism population of filler biofilms was richer and more diverse than the population of suspended sludge because the hybrid fillers were conducive to the development of functional microbial species, phyla Firmicutes, Actinobacteria, and Chloroflexi, and genus norank_f_JG30-KF-CM45, thus improving the treatment efficiency of FWW. Moreover, the microorganisms on the filler biofilms were more interconnected and interrelated than those in suspended sludge. The study findings provide reliable data and a theoretical basis for the application of HBR in the treatment of FWW.
Data availability
The data supporting this article have been included as part of the ESI.†
Author contributions
Zhenjun Tian: conceptualization, investigation, validation, writing – original draft. Ying Xiong: writing – review & editing. Guowen Li: methodology, writing – review & editing. Xiaoxin Cao: resources, writing – review & editing. Xin Li: conceptualization, methodology. Caili Du: visualization, methodology. Lieyu Zhang: supervision, funding acquisition, writing – review & editing.
Conflicts of interest
The authors declare that they have no known competing financial interests or personal relationships that could have appeared to influence the work reported in this paper.
Acknowledgements
We would like to thank the Beijing Science and Technology New Star Cross Cooperation Project (Project No. 20230484456) for the financial supports of this work.
References
- Y. Tang, Y. Li, L. Zhan, D. Wu, S. Zhang, R. Pang and B. Xie, Sci. Total Environ., 2022, 805, 150158 CrossRef CAS PubMed.
- W. Wang, Y. Zhang, T. M. Yin, L. Zhao, X. J. Xu, D. F. Xing, R. C. Zhang, D. J. Lee, N. Q. Ren and C. Chen, Sci. Total Environ., 2023, 905, 167142 CrossRef CAS PubMed.
- J. A. Arzate, M. Kirstein, F. C. Ertem, E. Kielhorn, H. Ramirez Malule, P. Neubauer, M. N. Cruz-Bournazou and S. Junne, Chem. Ing. Tech., 2017, 89, 686–695 CrossRef CAS.
- Z. Tian, G. Li, Y. Xiong, X. Cao, H. Pang, W. Tang, Y. Liu, M. Bai, Q. Zhu, C. Du, M. Li and L. Zhang, J. Environ. Manage., 2023, 345, 118434 CrossRef CAS.
- M. Lares, M. C. Ncibi, M. Sillanpää and M. Sillanpää, Water Res., 2018, 133, 236–246 CrossRef CAS PubMed.
- F. G. Meng, S. Q. Zhang, Y. Oh, Z. B. Zhou, H. S. Shin and S. R. Chae, Water Res., 2017, 114, 151–180 CrossRef CAS PubMed.
- C. Wang, M. Ding, T. C. A. Ng and H. Y. Ng, Chem. Eng. J., 2023, 466, 143226 CrossRef CAS.
- F. Hou, T. Zhang, Y. Peng, X. Cao, H. Pang, Y. Shao, X. Lu, J. Yuan, X. Chen and J. Zhang, Front. Environ. Sci. Eng., 2021, 16, 33 CrossRef.
- J. Wang, H. Shi and Y. Qian, Process Biochem., 2000, 36, 297–303 CrossRef.
- M. Godzieba, M. Zubrowska-Sudol, J. Walczak and S. Ciesielski, Sci. Rep., 2022, 12, 12558 CrossRef CAS.
- M. Azari, U. Walter, V. Rekers, J. D. Gu and M. Denecke, Chemosphere, 2017, 174, 117–126 CrossRef CAS.
- L. Zhang, M. Liu, S. Zhang, Y. Yang and Y. Peng, Chemosphere, 2015, 140, 114–118 CrossRef CAS.
- H. S. Lee, S. J. Park and T. I. Yoon, Process Biochem., 2002, 38, 81–88 CrossRef CAS.
- G. Güneş, E. Hallaç, M. Özgan, A. Ertürk, D. Okutman Taş, E. Çokgor, D. Güven, I. Takacs, A. Erdinçler and G. Insel, Bioprocess Biosyst. Eng., 2019, 42, 379–390 CrossRef.
- K. S. Jewell, P. Falås, A. Wick, A. Joss and T. A. Ternes, Water Res., 2016, 105, 559–567 CrossRef CAS PubMed.
- APHA, American Public Health Association, Washington DC, 2017 Search PubMed.
- Y. Deng, P. Zhang, Y. Qin, Q. Tu, Y. Yang, Z. He, C. W. Schadt and J. Zhou, Environ. Microbiol., 2016, 18, 205–218 CrossRef CAS.
- N. Seetha, R. Bhargava and P. Kumar, Bioresour. Technol., 2010, 101, 3060–3066 CrossRef CAS PubMed.
- E. Walters, A. Hille, M. He, C. Ochmann and H. Horn, Water Res., 2009, 43, 4461–4468 CrossRef CAS PubMed.
- I. W. Lo, K. V. Lo, D. S. Mavinic, D. Shiskowski and W. Ramey, J. Environ. Sci., 2010, 22, 953–960 CrossRef CAS PubMed.
- Y. He, P. Xu, C. Li and B. Zhang, Water Res., 2005, 39, 4110–4118 CrossRef CAS.
- S. M. Hocaoglu, G. Insel, E. U. Cokgor and D. Orhon, Bioresour. Technol., 2011, 102, 4333–4340 CrossRef CAS.
- H. Chen, X. Zhao, Y. Cheng, M. Jiang, X. Li and G. Xue, Environ. Sci. Technol., 2018, 52, 1404–1412 CrossRef CAS PubMed.
- J. Zhang, Y. Peng, X. Li and R. Du, Water Res., 2022, 208, 117856 CrossRef CAS PubMed.
- J. Yuan, X. Deng, X. Xie, L. Chen, C. Wei, C. Feng and G. Qiu, ISME Communications, 2024, 4, ycae011 CrossRef.
- G. M. Douglas, V. J. Maffei, J. R. Zaneveld, S. N. Yurgel, J. R. Brown, C. M. Taylor, C. Huttenhower and M. G. I. Langille, Nat. Biotechnol., 2020, 38, 685–688 CrossRef CAS PubMed.
- L. Chen, H. Chen, Z. Hu, Y. Tian, C. Wang, P. Xie, X. Deng, Y. Zhang, X. Tang, X. Lin, B. Li, C. Wei and G. Qiu, Water Res., 2022, 216, 118258 CrossRef CAS.
- K. Faust and J. Raes, Nat. Rev. Microbiol., 2012, 10, 538–550 CrossRef CAS.
- X. Xiao, W. Shi and W. Ruan, Processes, 2019, 7, 879 CrossRef CAS.
- Y. Wang, X. Huang and Q. Yuan, Process Biochem., 2005, 40, 1733–1739 CrossRef CAS.
- M. Galib, E. Elbeshbishy, R. Reid, A. Hussain and H. S. Lee, J. Environ. Manage., 2016, 182, 477–485 CrossRef CAS.
- W. W. Li, H. Q. Yu and B. E. Rittmann, Nature, 2015, 528, 29–31 CrossRef CAS PubMed.
- T. Fang, T. Wang, M. Zhao, L. Bai, Y. Deng and W. Ruan, J. Environ. Manage., 2023, 325, 116531 CrossRef CAS PubMed.
- Y. Duan, M. K. Awasthi, H. Wu, J. Yang, Z. Li, X. Ni, J. Zhang, Z. Zhang and H. Li, Bioresour. Technol., 2022, 346, 126647 CrossRef CAS.
- Y. Li, R. Dong, J. Guo, L. Wang and J. Zhao, J. Environ. Manage., 2022, 313, 115028 CrossRef CAS PubMed.
- F. Iannacone, F. Di Capua, F. Granata, R. Gargano and G. Esposito, Bioresour. Technol., 2020, 310, 123453 CrossRef CAS.
- E. Scholten, T. Lukow, G. Auling, R. M. Kroppenstedt, F. A. Rainey and H. Diekmann, Int. J. Syst. Evol. Microbiol., 1999, 49, 1045–1051 CrossRef CAS.
- X. Xing, X. Yuan, Y. Zhang, C. Men, Z. Zhang, X. Zheng, D. Ni, H. Xi and J. Zuo, J. Environ. Manage., 2023, 345, 118763 CrossRef CAS.
- S. Wongkiew, T. Koottatep, C. Polprasert, P. Prombutara, W. Jinsart and S. K. Khanal, Waste Manage., 2021, 125, 67–76 CrossRef CAS PubMed.
- X. Huang, Y. Xing, H. Wang, Z. Dai and T. Chen, Front. Microbiol., 2022, 12, 811697 CrossRef.
- T. Wu, S. S. Yang, L. Zhong, J. W. Pang, L. Zhang, X. F. Xia, F. Yang, G. J. Xie, B. F. Liu, N. Q. Ren and J. Ding, Sci. Total Environ., 2023, 856, 158977 CrossRef CAS PubMed.
- K.-Y. Kim, W. Yang, P. J. Evans and B. E. Logan, Bioresour. Technol., 2016, 221, 96–101 CrossRef CAS PubMed.
- N. Fazli, N. S. A. Mutamim, N. M. A. Jafri and N. A. M. Ramli, J. Environ. Chem. Eng., 2018, 6, 4339–4346 CrossRef CAS.
- S. Mishra, V. Singh, B. Ormeci, A. Hussain, L. Cheng and K. Venkiteshwaran, J. Environ. Manage., 2023, 327, 116898 CrossRef CAS PubMed.
- A. Ahmad and A. S. Senaidi, Environ. Sci. Pollut. Res., 2023, 30, 48703–48720 CrossRef CAS PubMed.
|
This journal is © The Royal Society of Chemistry 2024 |
Click here to see how this site uses Cookies. View our privacy policy here.