DOI:
10.1039/D4RA01548G
(Paper)
RSC Adv., 2024,
14, 15048-15057
Improving photoluminescence properties and reducing recombination of CsPbBr3 perovskite through lithium doping
Received
29th February 2024
, Accepted 23rd April 2024
First published on 8th May 2024
Abstract
This study investigates the impact of lithium doping on the structural and photophysical properties of spin-coated CsPbBr3 perovskite thin films. The deposited films display a pristine structure, preferentially growing along the (220) direction, and exhibit high-quality green photoluminescence at around 530 nm. The doping leads to an improvement in the optical properties of the films, as evidenced by a stronger photoluminescence (PL) intensity compared to undoped CsPbBr3, particularly at temperatures below 200 K. The increase in PL intensity suggests a decrease in defects and surface passivation. Additionally, the decrease in the power-law exponent β from 1.6 to 1.0 indicates a reduction in non-radiative recombination, likely due to trap states filling with free electrons induced by the doping. Overall, doping with lithium reduces non-radiative recombination, fills trap states, and reduces band tail/activation energy, leading to improved optoelectronic properties of the films. This investigation provides insights into the photophysical properties of the Li–CsPbBr3 absorber layer and the recombination mechanism, and helps to unravel new methods for the development of high-stability, high-performance perovskite thin-film solar cells and optoelectronic devices.
Introduction
Cesium lead halide perovskites show better moisture, oxygen, and thermal stabilities,1–3 along with improved mechanical properties when organic cation (MA+/FA+) is substituted in perovskite solar cells,4 allowing them to withstand temperatures up to 300 °C.5 CsPbBr3 exhibits remarkably high carrier mobility, large diffusion length, low trap density, and excellent light absorption.6–9 In addition, CsPbBr3 materials are known to have excellent optoelectronics characteristics, such as high quantum yield, excellent photophysical properties and strong light absorption,10 which allow them to be successfully applied in photodetectors,11,12 colored-light-emitting diodes13,14 solar cells11,13 and luminescent solar concentrators.14 Furthermore, due to the bonding–antibonding interaction between the conduction and valence bands, CsPbBr3 is a defect-tolerant semiconductor material that can maintain its general qualities despite the presence of defects.15 Nevertheless, its remarkable photophysical properties, including lifetime, diffusion length, and recombination of carriers, can be affected by the defect density, and hence limit the device performance due to non-radiative losses.16–18 The typical trap state density in solution-processed perovskite films is about 1015–1016 cm−3, which is comparable to the density of photogenerated or photoinjected carriers in solar cells or LEDs under device working conditions.19,20 J. Kang and his colleagues performed theoretical calculations on CsPbBr3. They suggested that the dominant defects can introduce shallow transition levels, and proposed that Br-poor growth conditions are a key factor for reducing the defect density.15 Doping with alkali cations is a good way to reduce defects and therefore lower non-radiative recombination in perovskite materials. Various reports demonstrated the effect of doping on surface passivation, enlarging grain sizes, and passivating grain boundaries, as well as in reducing non-radiative losses and defect state density.21,22 In particular, doping with alkali metal (Cs+, Rb+, Li+, K+) has been used as an effective way to reduce non-radiative losses in perovskite materials, either by grain and surface passivation or by suppressing the trapping of photo-carriers.23–25 Alkaline doping (Rb+, K+, Na+, …) enhances perovskite film performance, with less trap states and grain boundary passivation increasing the built-in potential, which as result leads to superior power conversion efficiency.21,26–28 In fact, it has been observed that the alkaline metals Na+ and K+ exhibit a positive influence on the characteristics of solar cells, particularly in enhancing the power conversion efficiency (PCE). For instance, the PCE showed significant improvement, rising from 15.56% to 18.16% and 17.81% with 1% Na doping and K doping respectively. As reported by the authors Wangen et al.,21 this observed enhancement can be attributed to certain positive morphological changes in the CH3NH3PbI3 perovskite materials. Rubidium has proven to be an efficient alkali metal for various optoelectronic devices, such as LEDs, as reported by Shi et al.29 Their research revealed that the incorporation of Rb+ in FAPbBr3 significantly enhances the photoluminescence quantum yield (PLQY) by reducing trap density. Consequently, this improvement augments the performance of PeLEDs (perovskite light emitting diodes). An optimum PCE of 25.6% (certified 25.2%) has been reached for formamidinium lead triiodide (FAPbI3) films, which have 450 hours of stability.30 However, organic–inorganic perovskites suffer from device degradation due to hydrate formation.31 The bandgap of CsPbX3 (X: I, Br, Cl) can be adjusted from the visible to the ultraviolet by using different halide ions.32,33 Among them, CsPbBr3 with a 2.3 eV bandgap demonstrates superior phase stability under ambient conditions34 with PCE values ranging from 2% to 8.29%.35–38 Several studies have concentrated on exploring the impact of lithium on perovskites. For instance, H. Wu et al.39 utilized LiBr within colloidal CsPbX3 (X = Br and I) perovskite quantum dots as a novel agent aimed at mitigating defects for excited electrons. This approach significantly reduced non-radiative recombination, leading to enhanced performance. Their findings suggest that an optimal concentration of LiBr not only diminishes defects, but also enhances phase stability by mitigating halide migration effects. Another study conducted by Dazhao et al.40 showed the self-crystallization of CsPbBr3 nanocrystals within borosilicate glass through lithium doping. They elucidated that the introduction of lithium into CsPbBr3 nanocrystals facilitated self-crystallization, which was primarily attributed to alterations in the glass network structure and an enhancement in the degree of phase separation within the glass.
In the present study, the impact of different fractions of Li doping on the structural, morphological, optical and photoluminescence properties is investigated. The mechanism of lithium doping on photocarrier recombination and its impact on deep defect trap states in CsPbBr3 films are examined with the temperature and power dependence in photoluminescence characterizations. These findings also clarify the nature of the surface trap states and the role of lithium in the surface passivation of perovskite films.
Experimental details
Synthesis and characterization
Preparation of the substrate. FTO substrates were cleaned under sonification for 15 min, with 2% Hellmanex solution, acetone, and ethanol, respectively. Perovskite solution preparation: CsPbBr3 films were synthetized from the appropriate molar ratio of CsBr (purity = 99.999%), LiBr (purity > 99%), and PbBr2 (purity ≥ 98%), which were purchased from Sigma-Aldrich. The precursors were dissolved in 48% DMSO and 52% DMF under an inert atmosphere in an Ar filled glove box (O2 and H2O < 2 ppm). The solution was rigorously stirred at 80 °C for 24 hours.
Perovskite film deposition. The films were elaborated within the glove box via a spin-coating process at 5000 rpm for 30 s. Subsequently, the samples were dried and annealed at 90 °C for 30 min. Finally, the synthetized thin films were stored in the glove box before any analysis.
Perovskite film characterizations. A RIGAKU Ultima IV X-ray diffractometer was employed for structural studies using the Bragg–Bentano configuration. The surface morphology of the synthetized perovskite films was recorded using an environmental scanning electron microscope FESEM (Quanta 200 FEI). The optical absorption was measured using an Ocean Optics HR4000 spectrophotometer equipped with a Si-CCD detector. Photoluminescence characterizations were carried out using a He–Cd laser source and a back-thinned Si-CCD detector.
Results and discussion
Fig. 1a presents the X-ray analysis of the pristine and doped CsPbBr3 films. The obtained patterns reveal several diffraction peaks, centered at 15.21, 21.6 and 30.6°, assigned respectively to the (110), (020), and (220) lattice planes, as shown in Fig. 1a. The asterisk symbol indicates the substrate contribution, and no binary phases were found within the detection limit of the instrument. The (220) peak intensity is relatively high compared to the literature data,39–41 indicating preferential growth along this direction for the perovskite films. Furthermore, an increase in the (110) and (220) peak intensities is observed with doping, resulting in improved crystallinity of the synthetized films. The crystal structure of the obtained XRD results can be assigned to the cubic structure related to the space group Pm
m.42 Pawley refinement (Fig. 1d) was used to extract the lattice parameters of the undoped and Li+ doped CsPbBr3, as presented in Table 1. After a thorough analysis of the XRD results, it was observed that the incorporation of 0 to 5% Li alkali into the perovskite matrix results in a slight shift towards lower angle, as illustrated in Fig. 1b. This shift will notably influence the d-spacing according to Bragg's law and consequently affect the lattice parameters of the cubic structure. Fig. 1c illustrates the evolution of the lattice volume with the incorporation of Li into the CsPbBr3 lattice, showing a consistent linear increase in volume with increasing Li content. This crystal expansion supported by higher lattice parameters was also reported by Z. Tang et al.,43 who attributed it to the smaller ionic radius of the Li+ alkali ions.
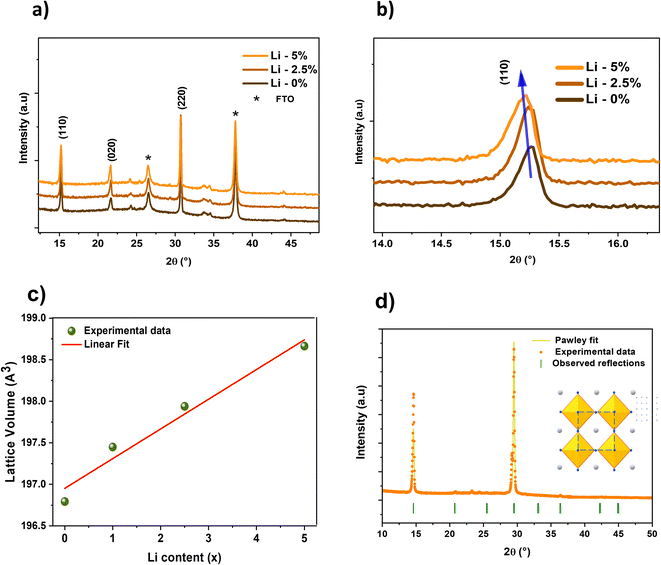 |
| Fig. 1 (a) XRD pattern of spin coated CsPbBr3 films with different Li contents (0, 2.5 and 5%). (b) Expansion of the XRD pattern within the angular range of 14–16.5°. (c) Lattice parameter evolution as a function of Li content. (d) X-ray diffraction pattern of CsPbBr3: experimental and refinement data. | |
Table 1 Lattice parameters and RP of undoped CsPbBr3 and doped Li%–CsPbBr3
Material |
CsPbBr3 |
2.5% Li–CsPbBr3 |
5% Li–CsPbBr3 |
Space group |
Pm m |
Pm m |
Pm m |
a (Å) |
5.817 ± 0.001 |
5.828 ± 0.001 |
5.835 ± 0.001 |
Rp |
7.78% |
5.14% |
4.15% |
The FESEM images of undoped and doped 5% Li–CsPbBr3 films are illustrated in Fig. 2a and b. A rougher and more compact film with a smaller grain size are observed for the pristine film (0% Li+). In addition, its surface is well covered with regular grains distributed uniformly. In contrast, the doped film presents a surface covered with large regular grains. In fact, the grains sizes range from 300 to 600 nm. However, it suffers from the presence of pinholes, which may be attributed to the evaporation of DMF/DMSO as discussed in our recent works.44 Upon comparing the morphology of both films, it is evident that the incorporation of Li+ contributes to the enlargement of the grain size.
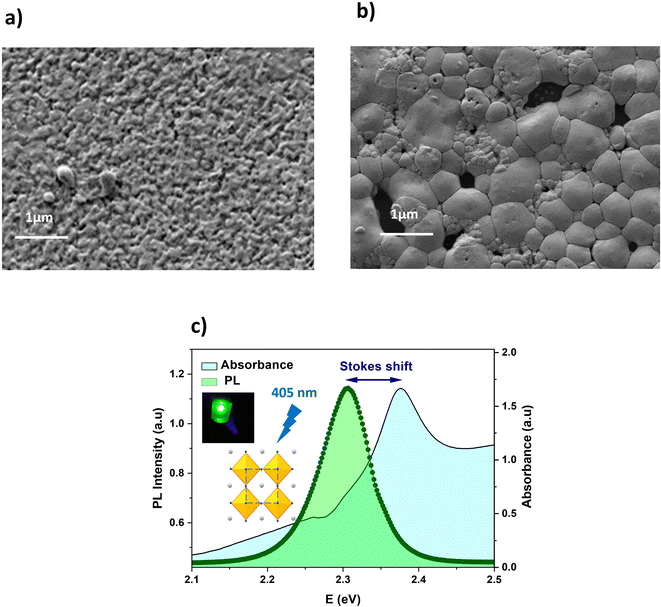 |
| Fig. 2 (a) SEM image of undoped CsPbBr3 film, (b) SEM image of 5% Li–CsPbBr3 film, and (c) room temperature absorption and PL spectrum of CsPbBr3 thin film. | |
The room temperature optical absorption and photoluminescence emission relationships for the spin coated CsPbBr3 film are illustrated in Fig. 2c. The sample exhibits a continuous increase in absorbance to reach an absorption edge at 2.37 eV and small excitonic features around 2.26 eV. The PL measurements reveal a sharp emission peak centered at 2.3 eV with a slight full width at half maximum (FWHM) of 20.66 nm. Stokes shifts are regularly observed in perovskite semiconductors and can be understood through a spectral diffusion/exciton relation process.45 The inset shows the CsPbBr3 crystal structure and the high green PL emission under an excitation of 405 nm.
The normalized photoluminescence spectra were evaluated at several temperatures from 30 K to 300 K for the CsPbBr3 and 5% Li–CsPbBr3 films, as illustrated in Fig. 3a and 2b, respectively. For both samples, the intensity of the PL peak decreases with increasing temperature, and the emission peak is blue-shifted and the FWHM values are enlarged due to the enhanced coupling with optical phonons46,47 and can be attributed to the phonon-assisted broadening effect.48 Furthermore, the PL intensity of the Li-doped CsPbBr3 films was stronger than that of undoped CsPbBr3 for operating temperatures below 200 K, which could be linked to an improvement in the optical properties of the films after doping. A slight shift is observed in the PL peak with temperature for the undoped and doped films, which suggests smaller differentiation in the bandgap.
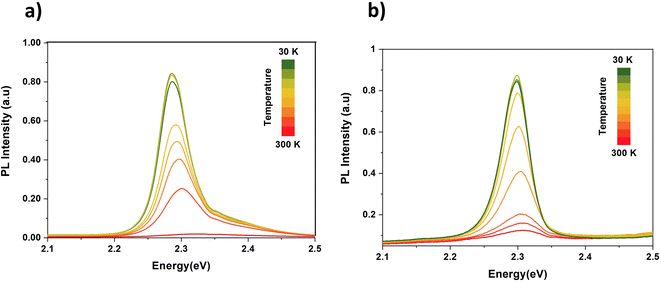 |
| Fig. 3 Temperature dependent photoluminescence spectra in the temperature range of 30 K to 300 K for (a) CsPbBr3 and (b) 5% Li–CsPbBr3. | |
To provide more information regarding the quality of the Li–CsPbBr3 films, the exciton (electron)–phonon interaction processes, and the presence and nature of defects in their surfaces, temperature-dependent photoluminescence was carried out. Fig. 3a and b display the evolution of the integrated PL intensity for the undoped CsPbBr3 and 5% Li–CsPbBr3 films, respectively, at temperatures ranging from 30 to 300 K. The decrease observed in the integrated PL intensity with temperature suggests the presence of thermal quenching.49 Exciton dissociations are activated by thermal energy, which make them competitive with the radiative recombination.
A modified Arrhenius formula was used to fit the temperature-dependent integrated PL intensities, as presented in Fig. 4a. With increasing temperature, the integrated PL intensity decreases, which suggests the appearance of thermal quenching.49 The same exponential trend is observed in CsPbBr3 nanocrystals and quantum dots.46,50
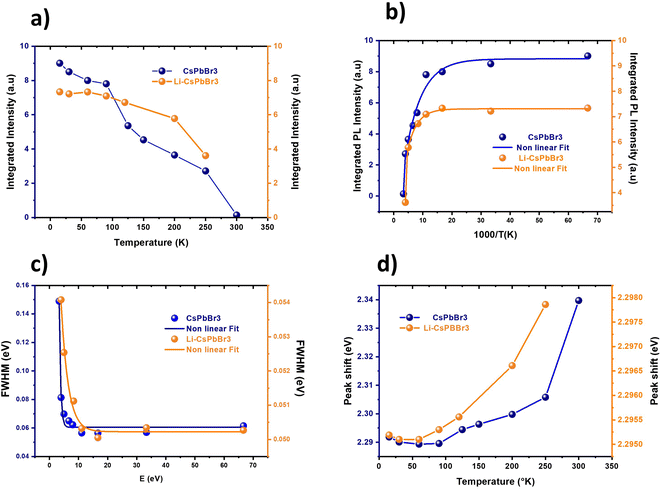 |
| Fig. 4 (a and b) Evolution of integrated PL intensity with temperature for CsPbBr3 and 5% Li–CsPbBr3. (c) FWHM of the PL peak as a function of temperature for the CsPbBr3 and 5% Li–CsPbBr3 samples. (d) Peak shift of the CsPbBr3 and 5% Li–CsPbBr3 films with temperature. | |
The activation energy was extracted from the Arrhenius equation:51,52
|
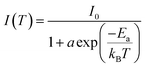 | (1) |
where
I0 represents the integrated PL intensity near 0 K,
Ea is the activation energy of the non-radiative recombination process,
a is related to the density of non-radiative recombination centers, and
kB is the Boltzmann constant.
The obtained exciton binding energy for CsPbBr3 and 5% Li–CsPbBr3 were found to be 60.50 and 50.22 meV, respectively. The obtained values are close to the ones reported in the literature.46,53,54
Emission linewidth can provide details about temperature-independent inhomogeneous broadening due to elemental composition, shape, or size dispersion. The contribution of exciton interactions with optical and acoustic phonons can also be provided by temperature-dependent homogenous broadening and are mainly due to lattice vibrations.54 The mechanism of electron–phonon coupling is ascribed to the Boson model55 and evaluated by fitting the FWHM of the PL emission with temperature as presented in Fig. 4c using the equation below:
|
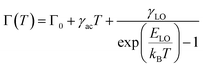 | (2) |
where
Γ0 refers to the inhomogeneous broadening, which involves the scattering process from disorder and lattice imperfections,
56 and
γac is the contribution of the exciton–acoustic phonon coupling coefficient. The third term represents the contribution of exciton interactions with longitudinal optical phonons, in which
γLO is the coupling coefficient of the exciton–optical phonon,
ELO represents the phonon energy and
kB is the Boltzmann constant. The contribution of impurities was neglected in this calculation because of the minimal trap states in CsPbBr
3. It was found that the Gauss-fitted FWHM of the PL band increases with temperature.
Table 2 summarizes the fitting results for Γ0, γac, γLO and ELO. The exciton–LO phonon coupling is the dominant contribution in homogenous linewidth broadening due to the polar nature of CsPbBr3. Typically, the interactions between LO phonons and electrons are stronger than those between acoustic phonons and electrons.57 The acoustic phonon interaction seems to have a negligible contribution to the FWHM of the PL emission.
Table 2 Extracted PL linewidth broadening parameters
Sample |
Γ (meV) |
γac (meV K−1) |
γLO (meV) |
ELO (meV) |
CsPbBr3 |
61.55 |
1.40 |
84.8 |
33.43 |
5% Li–CsPbBr3 |
50.22 |
0.24 |
51.96 |
1.23 |
The PL peak shifted to higher values with temperature for both the spin-coated films, as shown in Fig. 4d, which could be due to lattice dilatation and crystallite reorientation.9 The peak position increases moderately for the doped film, which may be due to the enhanced electron–phonon interactions. It is well known that the thermal expansion of the lattice reduces the interaction between the valence orbitals Br 4p and Pb 6s, thus increasing the bandgap.54
To obtain more in-depth information on the charge recombination processes of the 5% Li-doped CsPbBr3 films, the photoluminescence of the films was studied under varying excitation power densities from 50 to 350 mW cm−2 using a 450 nm laser. The PL spectra are shown in Fig. 5a for CsPbBr3 and Fig. 5b for 5% Li–CsPbBr3. The results illustrate an increase in the PL intensity with lower excitation power density for the two samples. In addition, the PL intensities of doped film are two times stronger than the pristine ones, which sustain our results on a decrease of defects, and surface passivation.
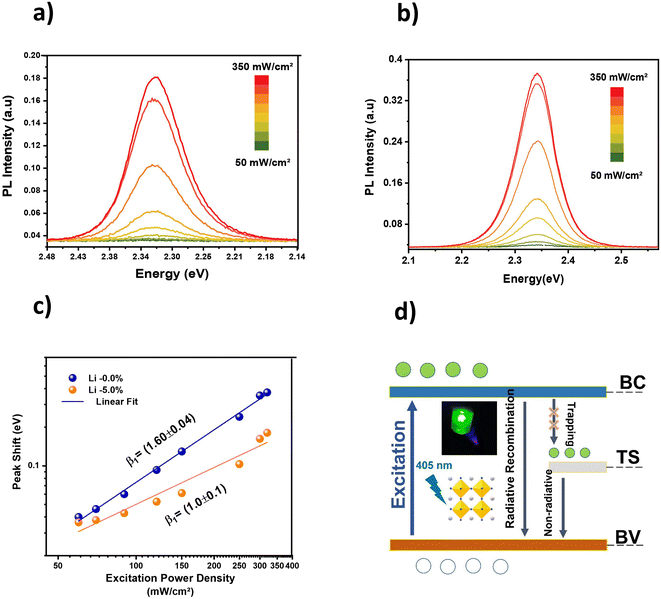 |
| Fig. 5 Power-dependent PL spectra excitation intensity in the range of 50–350 mW cm−2 for (a) CsPbBr3; and (b) 5% Li–CsPbBr3. (c) Double-logarithmic PL plots for CsPbBr3 and 5% Li–CsPbBr3 as a function of excitation power density. (d) Schematic illustrating the reduction of the non-radiative recombination process after Li-doping of CsPbBr3 films. | |
Under non-resonant excitation conditions, the PL intensity in direct-bandgap semiconductors has a power-law dependence on the excitation density (IEx.), according to the equation below:57
with
β = 2 for band-to-band transitions due to exciton or free carrier recombination, 1 <
β < 2 for exciton recombination, which includes free excitons and bound excitons, and
β < 1 for free-to-bound recombination and donor–acceptor pairs.
52
As shown in Fig. 5c, the peak shift has a dependence on the excitation power density, which implies the excitonic nature of the emission. This linear tendency diverges slowly for the Li–CsPbBr3 films. The power-law exponent β was found to be 1.6 ± 0.04 and 1 ± 0.1 for the undoped and 5% Li–CsPbBr3 films. This means that the CsPbBr3 films are governed by exciton recombination, while for the doped CsPbBr3, there is interference between bound pair excitons, free excitons, free-to-bound recombination, and donor–acceptor pair. There is no abrupt increase in the integrated PL intensity below 175 mW cm−2 with excitation power density because of spontaneous radiation instead of amplified spontaneous emission or other optical non-linear phenomena.58
The decrease of β from 1.6 to 1.0 indicates a decay in non-radiative recombination, as doping induces trap states filled by free electrons. A similar effect has been observed in the doping of MAPbI3 films by Li, as reported by Fang et al.20
For better illustration of this effect, Fig. 5d shows a schematic of the radiative and non-radiative processes in 5% Li–CsPbBr3, specifically, the reduction of the non-radiative recombination process after the incorporation of Li in the CsPbBr3 films. On the other hand, radiative recombination for the undoped CsPbBr3 films occurs for the electrons located in the conduction band (CB) after having been excited with energies higher than the bandgap. Two recombination processes occur, the first when the electrons return directly to the valence band (VB), and the second when the electrons are trapped in the defect band (DB) located near the bandgap, and subsequently follow a non-radiative path when returning to the VB. For the doped films, the presence of Li in CsPbBr3 film prevents the trapping of electrons by inducing a new energy level (TS), which is more favorable to the radiative recombination path, and thus leads to an increase in the PL emission.
It is clearly seen from the photoluminescence investigations that doping with lithium reduces non-radiative recombination by filling trap states as well as reducing band tail/activation energy. Considering the amount of defect states filled by Li-doping, the trap density was calculated using the equations below, and the obtained values are summarized in Table 3.
|
Reduction in trap state = log10(Nt) − (Li% × log10(Nt))
| (4) |
|
Calculated trap density (cm−3) Nc_t = antilog10(reduction in trap state)
| (5) |
where,
Nt is the trap density of CsPbBr
3 and
Nc_t is the calculated trap state after Li doping.
Table 3 Deep defect trap states vs. Li doping in CsPbBr3 thin films
Doping of Li (%) |
Calculated trap density Nc_t (cm−3) |
0 |
5.0 × 1017 |
2.5 |
1.5 × 1017 |
5 |
6.0 × 1016 |
The results show fewer trap states after doping with Li, which may be due to fewer grain boundaries. The same behavior has been observed for doping with other alkali metals in perovskite solar cells, and was attributed to the same factor.21
Concluding remarks
In conclusion, lithium doping significantly enhances the structural and photophysical properties of CsPbBr3 perovskite films. The doping improves crystallinity and reduces non-radiative recombination, leading to stronger photoluminescence intensity, particularly at lower temperatures. The surface passivation effect from the alkali cation doping, along with the weakening of electron–phonon coupling, contribute to these positive effects. These findings provide valuable insights into the non-radiative recombination mechanisms, which are crucial for improving the efficiency of perovskite solar cells and the performance of optoelectronic devices. The study suggests that Li-doped CsPbBr3 films hold promise for the development of high-stability, high-performance perovskite thin-film solar cells and optoelectronic devices.
Conflicts of interest
There are no conflicts to declare.
Acknowledgements
The authors would like to thank the LEAP RE initiative, founded by the Ministry of Higher Education, Scientific Research, and Innovation (MESRSI) for the financial support through the project “Environmentally friendly colloidal quantum dots for high performance solar cells”/(QDSOC). M. Bernabé and U. Shaffi would like to thank the Generalitat Valenciana for funding through program PROMETEUS Ref: CIPROM/2022/03.
References
- P. Teng, X. Han, J. Li, Y. Xu, L. Kang, Y. Wang, Y. Yang and T. Yu, Elegant Face-Down Liquid-Space-Restricted Deposition of CsPbBr3 Films for Efficient Carbon-Based All-Inorganic Planar Perovskite Solar Cells, ACS Appl. Mater. Interfaces, 2018, 10(11), 9541–9546, DOI:10.1021/acsami.8b00358.
- X. Liu, X. Tan, Z. Liu, H. Ye, B. Sun, T. Shi, Z. Tang and G. Liao, Boosting the Efficiency of Carbon-Based Planar CsPbBr3 Perovskite Solar Cells by a Modified Multistep Spin-Coating Technique and Interface Engineering, Nano Energy, 2019, 56, 184–195, DOI:10.1016/j.nanoen.2018.11.053.
- L. Atourki, M. Bernabé, M. Makha, K. Bouabid, M. Regragui, A. Ihlal, M. Abd-lefdil and M. Mollar, Effect of doping on the phase stability and photophysical properties of CsPbI2Br perovskite thin films, RSC Adv., 2021, 11, 1440–1449, 10.1039/D0RA08912E.
- Y. Jiaoxian, L. Guangxia, C. Chengmin, L. Yan, X. Meirong, W. Tailin, Z. Gang and Z. Lei, Perovskite CsPbBr3 Crystal: Growth and Applications, J. Mater. Chem. C, 2020, 8, 6326–6341, 10.1039/X0xx00000x.
- S. Sanchez, N. Christoph, B. Grobety, N. Phung, U. Steiner, M. Saliba and A. Abate, Efficient and Stable Inorganic Perovskite Solar Cells Manufactured by Pulsed Flash Infrared Annealing, Adv. Energy Mater., 2018, 8(30), 1802060, DOI:10.1002/aenm.201802060.
- F. Zhang, C. Xiao, Y. Li, X. Zhang, J. Tang, S. Chang, Q. Pei and H. Zhong, Gram-Scale Synthesis of Blue-Emitting CH3NH3PbBr3 Quantum Dots Through Phase Transfer Strategy, Front. Chem., 2018, 6, 444, DOI:10.3389/fchem.2018.00444.
- P. G. Papagiorgis, A. Manoli, A. Alexiou, P. Karacosta, X. Karagiorgis, G. Papaparaskeva, C. Bernasconi, M. I. Bodnarchuk, M. V. Kovalenko, T. Krasia-Christoforou and G. Itskos, Robust Hydrophobic and Hydrophilic Polymer Fibers Sensitized by Inorganic and Hybrid Lead Halide Perovskite Nanocrystal Emitters, Front. Chem., 2019, 7, 87, DOI:10.3389/fchem.2019.00087.
- S. S.-Y. Juang, P.-Y. Lin, Y.-C. Lin, Y.-S. Chen, P.-S. Shen, Y.-L. Guo, Y.-C. Wu and P. Chen, Energy Harvesting Under Dim-Light Condition With Dye-Sensitized and Perovskite Solar Cells, Front. Chem., 2019, 7, 209, DOI:10.3389/fchem.2019.00209.
- L. Atourki, M. Bernabé, M. Makha, K. Bouabid, M. Regragui, A. Ihlal, M. Abd-lefdil and M. Mollar, Effect of Doping on the Phase Stability and Photophysical Properties of CsPbI2 Br Perovskite Thin Films, RSC Adv., 2021, 11(3), 1440–1449, 10.1039/D0RA08912E.
- Y. Gao, Y. Wu, Y. Liu, C. Chen, X. Bai, L. Yang, Z. Shi, W. W. Yu, Q. Dai and Y. Zhang, Dual Functions of Crystallization Control and Defect Passivation Enabled by an Ionic Compensation Strategy for Stable and High-Efficient Perovskite Solar Cells, ACS Appl. Mater. Interfaces, 2020, 12(3), 3631–3641, DOI:10.1021/acsami.9b19538.
- X. Chang, W. Li, L. Zhu, H. Liu, H. Geng, S. Xiang, J. Liu and H. Chen, Carbon-Based CsPbBr3 Perovskite Solar Cells: All-Ambient Processes and High Thermal Stability, ACS Appl. Mater. Interfaces, 2016, 8(49), 33649–33655, DOI:10.1021/acsami.6b11393.
- W. Zhai, J. Lin, C. Li, S. Hu, Y. Huang, C. Yu, Z. Wen, Z. Liu, Y. Fang and C. Tang, Solvothermal Synthesis of Cesium Lead Halide Perovskite Nanowires with Ultra-High Aspect Ratios for High-Performance Photodetectors, Nanoscale, 2018, 10(45), 21451–21458, 10.1039/C8NR05683H.
- Y. H. Khattak, F. Baig, A. Shuja, L. Atourki, K. Riaz and B. M. Soucase, Device Optimization of PIN Structured Perovskite Solar Cells: Impact of Design Variants, ACS Appl. Electron. Mater., 2021, 3509–3520, DOI:10.1021/acsaelm.1c00460.
- Y. Zhang, W. Zhang, Y. Ye, K. Li, X. Gong and C. Liu, CsPbBr3 Nanocrystal-Embedded Glasses for Luminescent Solar Concentrators, Sol. Energy Mater. Sol. Cells, 2022, 238, 111619, DOI:10.1016/j.solmat.2022.111619.
- J. Kang and L.-W. Wang, High Defect Tolerance in Lead Halide Perovskite CsPbBr3, J. Phys. Chem. Lett., 2017, 8(2), 489–493, DOI:10.1021/acs.jpclett.6b02800.
- M. Saliba, T. Matsui, K. Domanski, J.-Y. Seo, A. Ummadisingu, S. M. Zakeeruddin, J.-P. Correa-Baena, W. R. Tress, A. Abate, A. Hagfeldt and M. Grätzel, Incorporation of Rubidium Cations into Perovskite Solar Cells Improves Photovoltaic Performance, Science, 2016, 354(6309), 206–209, DOI:10.1126/science.aah5557.
- C. Momblona, L. Gil-Escrig, E. Bandiello, E. M. Hutter, M. Sessolo, K. Lederer, J. Blochwitz-Nimoth and H. J. Bolink, Efficient Vacuum Deposited P-i-n and n-i-p Perovskite Solar Cells Employing Doped Charge Transport Layers, Energy Environ. Sci., 2016, 9(11), 3456–3463, 10.1039/C6EE02100J.
- S. D. Stranks, Nonradiative Losses in Metal Halide Perovskites, ACS Energy Lett., 2017, 2(7), 1515–1525, DOI:10.1021/acsenergylett.7b00239.
- H. Tan, A. Jain, O. Voznyy, X. Lan, F. P. García De Arquer, J. Z. Fan, R. Quintero-Bermudez, M. Yuan, B. Zhang, Y. Zhao, F. Fan, P. Li, L. N. Quan, Y. Zhao, Z.-H. Lu, Z. Yang, S. Hoogland and E. H. Sargent, Efficient and Stable Solution-Processed Planar Perovskite Solar Cells via Contact Passivation, Science, 2017, 355(6326), 722–726, DOI:10.1126/science.aai9081.
- Z. Fang, H. He, L. Gan, J. Li and Z. Ye, Understanding the Role of Lithium Doping in Reducing Nonradiative Loss in Lead Halide Perovskites, Adv. Sci., 2018, 5(12), 1800736, DOI:10.1002/advs.201800736.
- W. Zhao, Z. Yao, F. Yu, D. Yang and S. F. Liu, Alkali Metal Doping for Improved CH3 NH3 PbI3 Perovskite Solar Cells, Adv. Sci., 2018, 5(2), 1700131, DOI:10.1002/advs.201700131.
- M. Abdi-Jalebi, Z. Andaji-Garmaroudi, S. Cacovich, C. Stavrakas, B. Philippe, J. M. Richter, M. Alsari, E. P. Booker, E. M. Hutter, A. J. Pearson, S. Lilliu, T. J. Savenije, H. Rensmo, G. Divitini, C. Ducati, R. H. Friend and S. D. Stranks, Maximizing and Stabilizing Luminescence from Halide Perovskites with Potassium Passivation, Nature, 2018, 555(7697), 497–501, DOI:10.1038/nature25989.
- J. Cao, S. X. Tao, P. A. Bobbert, C. Wong and N. Zhao, Interstitial Occupancy by Extrinsic Alkali Cations in Perovskites and Its Impact on Ion Migration, Adv. Mater., 2018, 30(26), 1707350, DOI:10.1002/adma.201707350.
- J. A. Dawson, A. J. Naylor, C. Eames, M. Roberts, W. Zhang, H. J. Snaith, P. G. Bruce and M. S. Islam, Mechanisms of Lithium Intercalation and Conversion Processes in Organic–Inorganic Halide Perovskites, ACS Energy Lett., 2017, 2(8), 1818–1824, DOI:10.1021/acsenergylett.7b00437.
- Q. Jiang, M. Chen, J. Li, M. Wang, X. Zeng, T. Besara, J. Lu, Y. Xin, X. Shan, B. Pan, C. Wang, S. Lin, T. Siegrist, Q. Xiao and Z. Yu, Electrochemical Doping of Halide Perovskites with Ion Intercalation, ACS Nano, 2017, 11(1), 1073–1079, DOI:10.1021/acsnano.6b08004.
- M. Abdi-Jalebi, Z. Andaji-Garmaroudi, A. J. Pearson, G. Divitini, S. Cacovich, B. Philippe, H. Rensmo, C. Ducati, R. H. Friend and S. D. Stranks, Potassium- and Rubidium-Passivated Alloyed Perovskite Films: Optoelectronic Properties and Moisture Stability, ACS Energy Lett., 2018, 3(11), 2671–2678, DOI:10.1021/acsenergylett.8b01504.
- F. Zhang and K. Zhu, Additive Engineering for Efficient and Stable Perovskite Solar Cells, Adv. Energy Mater., 2020, 10(13), 1902579, DOI:10.1002/aenm.201902579.
- L. Kuai, Y. Wang, Z. Zhang, Y. Yang, Y. Qin, T. Wu, Y. Li, Y. Li, T. Song, X. Gao, L. Wang and B. Sun, Passivating Crystal Boundaries with Potassium-Rich Phase in Organic Halide Perovskite, Sol. RRL, 2019, 3(5), 1900053, DOI:10.1002/solr.201900053.
- Y. Shi, J. Xi, T. Lei, F. Yuan, J. Dai, C. Ran, H. Dong, B. Jiao, X. Hou and Z. Wu, Rubidium Doping for Enhanced Performance of Highly Efficient Formamidinium-Based Perovskite Light Emitting Diodes, ACS Appl. Mater. Interfaces, 2018, 10(11), 9849–9857, DOI:10.1021/acsami.8b00079.
- J. Jeong, M. Kim, J. Seo, H. Lu, P. Ahlawat, A. Mishra, Y. Yang, M. A. Hope, F. T. Eickemeyer, M. Kim, Y. J. Yoon, I. W. Choi, B. P. Darwich, S. J. Choi, Y. Jo, J. H. Lee, B. Walker, S. M. Zakeeruddin, L. Emsley, U. Rothlisberger, A. Hagfeldt, D. S. Kim, M. Grätzel and J. Y. Kim, Pseudo-Halide Anion Engineering for α-FAPbI3 Perovskite Solar Cells, Nature, 2021, 592(7854), 381–385, DOI:10.1038/s41586-021-03406-5.
- S. Ullah, J. Wang, P. Yang, L. Liu, S.-E. Yang, T. Xia, H. Guo and Y. Chen, All-Inorganic CsPbBr3 Perovskite: A Promising Choice for Photovoltaics, Mater. Adv., 2021, 2(2), 646–683, 10.1039/D0MA00866D.
- S. Aharon and L. Etgar, Two Dimensional Organometal Halide Perovskite Nanorods with Tunable Optical Properties, Nano Lett., 2016, 16(5), 3230–3235, DOI:10.1021/acs.nanolett.6b00665.
- Y. Bekenstein, B. A. Koscher, S. W. Eaton, P. Yang and A. P. Alivisatos, Highly Luminescent Colloidal Nanoplates of Perovskite Cesium Lead Halide and Their Oriented Assemblies, J. Am. Chem. Soc., 2015, 137(51), 16008–16011, DOI:10.1021/jacs.5b11199.
- N. Kumar, J. Rani and R. Kurchania, Advancement in CsPbBr3 Inorganic Perovskite Solar Cells: Fabrication, Efficiency and Stability, Sol. Energy, 2021, 221, 197–205, DOI:10.1016/j.solener.2021.04.042.
- J. Duan, Y. Zhao, B. He and Q. Tang, Simplified Perovskite Solar Cell with 4.1% Efficiency Employing Inorganic CsPbBr3 as Light Absorber, Small, 2018, 14(20), 1704443, DOI:10.1002/smll.201704443.
- J. Liang, C. Wang, Y. Wang, Z. Xu, Z. Lu, Y. Ma, H. Zhu, Y. Hu, C. Xiao, X. Yi, G. Zhu, H. Lv, L. Ma, T. Chen, Z. Tie, Z. Jin and J. Liu, All-Inorganic Perovskite Solar Cells, J. Am. Chem. Soc., 2016, 138(49), 15829–15832, DOI:10.1021/jacs.6b10227.
- Y. Pei, H. Guo, Z. Hu, J. Zhang and Y. Zhu, BiBr3 as an Additive in CsPbBr3 for Carbon-Based All-Inorganic Perovskite Solar Cell, J. Alloys Compd., 2020, 835, 155283, DOI:10.1016/j.jallcom.2020.155283.
- G. Wang, W. Dong, A. Gurung, K. Chen, F. Wu, Q. He, R. Pathak and Q. Qiao, Improving Photovoltaic Performance of Carbon-Based CsPbBr3 Perovskite Solar Cells by Interfacial Engineering Using P3HT Interlayer, J. Power Sources, 2019, 432, 48–54, DOI:10.1016/j.jpowsour.2019.05.075.
- H. Wu, J. Qiu, J. Wang, Y. Wen, Q. Wang, Z. Long, D. Zhou, Y. Yang and D. Wang, The Dual-Defect Passivation Role of Lithium Bromide Doping in Reducing the Nonradiative Loss in CsPbX3 (X = Br and I) Quantum Dots, Inorg. Chem. Front., 2021, 8(3), 658–668, 10.1039/D0QI01262A.
- D. Wang, J. Qiu, D. Zhou, S. Hu, Y. Wen, K. Zhang, Q. Wang, Y. Yang, H. Wu, Z. Long, X. Li, J. Pi and E. Cao, Lithium Doping Induced Self-Crystallization of CsPbBr3 Nanocrystal Glass with Improved Quantum Yield and Stability, Chem. Eng. J., 2021, 421, 127777, DOI:10.1016/j.cej.2020.127777.
- A. Subramanian, Z. Pan, Z. Zhang, I. Ahmad, J. Chen, M. Liu, S. Cheng, Y. Xu, J. Wu, W. Lei, Q. Khan and Y. Zhang, Interfacial Energy-Level Alignment for High-Performance All-Inorganic Perovskite CsPbBr3 Quantum Dot-Based Inverted Light-Emitting Diodes, ACS Appl. Mater. Interfaces, 2018, 10(15), 13236–13243, DOI:10.1021/acsami.8b01684.
- M. C. Brennan, M. Kuno and S. Rouvimov, Crystal Structure of Individual CsPbBr3 Perovskite Nanocubes, Inorg. Chem., 2019, 58(2), 1555–1560, DOI:10.1021/acs.inorgchem.8b03078.
- Z. Tang, S. Uchida, T. Bessho, T. Kinoshita, H. Wang, F. Awai, R. Jono, M. M. Maitani, J. Nakazaki, T. Kubo and H. Segawa, Modulations of Various Alkali Metal Cations on Organometal Halide Perovskites and Their Influence on Photovoltaic Performance, Nano Energy, 2018, 45, 184–192, DOI:10.1016/j.nanoen.2017.12.047.
- M. Ouafi, L. Atourki, L. Laânab, E. Vega, B. Mari, M. Mollar and B. Jaber, Hot Airflow Deposition: Toward High Quality MAPbI3 Perovskite Films, J. Alloys Compd., 2019, 790, 1101–1107, DOI:10.1016/j.jallcom.2019.03.187.
- M. Kulbak, D. Cahen and G. Hodes, How Important Is the Organic Part of Lead Halide Perovskite Photovoltaic Cells? Efficient CsPbBr3 Cells, J. Phys. Chem. Lett., 2015, 6(13), 2452–2456, DOI:10.1021/acs.jpclett.5b00968.
- A. Dey, P. Rathod and D. Kabra, Role of Localized States in Photoluminescence Dynamics of High Optical Gain CsPbBr3 Nanocrystals, Adv. Opt. Mater., 2018, 6(11), 1800109, DOI:10.1002/adom.201800109.
- H. Min, M. Kim, S.-U. Lee, H. Kim, G. Kim, K. Choi, J. H. Lee and S. I. Seok, Efficient, Stable Solar Cells by Using Inherent Bandgap of a-Phase Formamidinium Lead Iodide, Science, 2019, 366, 749–753 CrossRef CAS PubMed.
- X. B. Zhang, T. Taliercio, S. Kolliakos and P. Lefebvre, Influence of Electron-Phonon Interaction on the Optical Properties of III Nitride Semiconductors, J. Phys.: Condens. Matter, 2001, 13(32), 7053–7074, DOI:10.1088/0953-8984/13/32/312.
- B. Ai, C. Liu, Z. Deng, J. Wang, J. Han and X. Zhaoa, Low Temperature Photoluminescence Properties of CsPbBr3 Quantum Dots Embedded in Glasses, Phys. Chem. Chem. Phys., 2016, 18(1), 21–46, 10.1039/C5CP05142H.
- A. Shinde, R. Gahlaut and S. Mahamuni, Low-Temperature Photoluminescence Studies of CsPbBr3 Quantum Dots, J. Phys. Chem. C, 2017, 121(27), 14872–14878, DOI:10.1021/acs.jpcc.7b02982.
- M. Leroux, N. Grandjean, B. Beaumont, G. Nataf, F. Semond, J. Massies and P. Gibart, Temperature Quenching of Photoluminescence Intensities in Undoped and Doped GaN, J. Appl. Phys., 1999, 86(7), 3721–3728, DOI:10.1063/1.371242.
- H. He, Q. Yu, H. Li, J. Li, J. Si, Y. Jin, N. Wang, J. Wang, J. He, X. Wang, Y. Zhang and Z. Ye, Exciton Localization in Solution-Processed Organolead Trihalide Perovskites, Nat. Commun., 2016, 7(1), 10896, DOI:10.1038/ncomms10896.
- H. Cho, C. Wolf, J. S. Kim, H. J. Yun, J. S. Bae, H. Kim, J. Heo, S. Ahn and T. Lee, High-Efficiency Solution-Processed Inorganic Metal Halide Perovskite Light-Emitting Diodes, Adv. Mater., 2017, 29(31), 1700579, DOI:10.1002/adma.201700579.
- A. Shinde, R. Gahlaut and S. Mahamuni, Low-Temperature Photoluminescence Studies of CsPbBr3 Quantum Dots, J. Phys. Chem. C, 2017, 121(27), 14872–14878, DOI:10.1021/acs.jpcc.7b02982.
- K. Wu, A. Bera, C. Ma, Y. Du, Y. Yang, L. Li and T. Wu, Temperature-Dependent Excitonic Photoluminescence of Hybrid Organometal Halide Perovskite Films, Phys. Chem. Chem. Phys., 2014, 16(41), 22476–22481, 10.1039/C4CP03573A.
- Z. Liu, Q. Shang, C. Li, L. Zhao, Y. Gao, Q. Li, J. Chen, S. Zhang, X. Liu, Y. Fu and Q. Zhang, Temperature-Dependent Photoluminescence and Lasing Properties of CsPbBr3 Nanowires, Appl. Phys. Lett., 2019, 114(10), 101902, DOI:10.1063/1.5082759.
- M. Sebastian, J. A. Peters, C. C. Stoumpos, J. Im, S. S. Kostina, Z. Liu, M. G. Kanatzidis, A. J. Freeman and B. W. Wessels, Excitonic Emissions and Above-Band-Gap Luminescence in the Single-Crystal Perovskite Semiconductors CsPbB r 3 and CsPbC l 3, Phys. Rev. B, 2015, 92(23), 235210, DOI:10.1103/PhysRevB.92.235210.
- Y. Wang, M. Zhi, Y.-Q. Chang, J.-P. Zhang and Y. Chan, Stable, Ultralow Threshold Amplified Spontaneous Emission from CsPbBr3 Nanoparticles Exhibiting Trion Gain, Nano Lett., 2018, 18(8), 4976–4984, DOI:10.1021/acs.nanolett.8b01817.
|
This journal is © The Royal Society of Chemistry 2024 |