DOI:
10.1039/D4RA00686K
(Paper)
RSC Adv., 2024,
14, 21269-21276
Recyclable LaF3·Pd nanocatalyst in Suzuki coupling: green synthesis of biaryls from haloarenes and phenylboronic acids†‡
Received
26th January 2024
, Accepted 13th June 2024
First published on 5th July 2024
Abstract
Herein we prepared the novel LaF3·Pd nanocatalyst characterized by XRD and TEM analysis. The nanocatalyst was applied in Suzuki coupling reaction for the synthesis of biaryls in aqueous medium from readily available aryl halides (bromides and iodides) and substituted phenylboronic acids in the presence of K2CO3 as the base at 70 °C. The present method is capable of giving the C–C coupled product in good to excellent yields (up to 97%). The reactions were conducted under green conditions in aqueous medium and the nanocatalyst used in this study was recyclable. The recyclability and reusability of the catalyst was checked for seven consecutive cycles without significant loss in reactivity.
Introduction
C(sp2)–C(sp2) bond forming reactions1 are very important strategies to construct complex scaffolds in natural products,2 drug discovery, medicinal chemistry,2b functional materials, etc. Various transition metals have been used extensively in many reactions i.e., C–H activations,3 cross-coupling reactions, etc. to construct C–C bonds having moderate to complex motifs in many usual organic transformations. Palladium-catalyzed coupling reactions play a very important role in organic synthesis. The 2010 Nobel prize in chemistry supports the popularity, success, and importance of this field.4 Among all, the Suzuki coupling reaction is one of the most celebrated and frequently encountered reactions in the laboratory as well as industry for the construction of C–C bonds to synthesize biaryls.5 Biaryl-containing scaffolds as a backbone constitute a subunit in many organic frameworks, and are widely distributed in many natural products,6 functional materials, catalysts-ligands,7 pharmaceuticals and drugs,8 agrochemicals,9 etc. (Fig. 1). The biaryl-based scaffolds have been documented to exhibit a broad range of biological activities.10
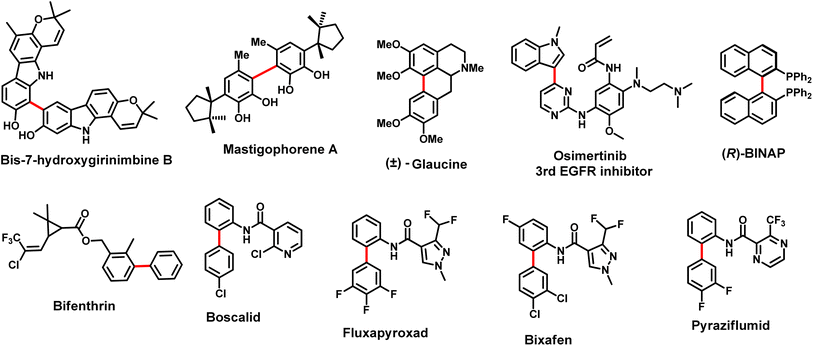 |
| Fig. 1 Representative examples of biaryls in naturally occurring molecules, catalysts, drugs, and agrochemicals with biaryl scaffolds. | |
Discovering greener approaches in organic synthesis is always a challenge to organic chemists. The demand for developing green and sustainable methods has been increasing in recent years. In this context, the use of green energy, solvents, one-pot processes with minimal waste production with high atom and step-economy, and the use of recyclable nanocatalysts are considered to be the better alternatives.5e In the literature the Suzuki coupling is well documented with the combination of various homogeneous catalysts and ligands, which also comes with some limitations such as expensive ligands, additional steps for ligand synthesis, metal contamination in the products, metal leaching.5d In recent years, transition metal-derived nanocatalysts have received a great deal of attention from synthetic chemists because of their high activity, and sustainable aspects as compared to homogeneous catalysts. They are frequently used in organic synthesis.11 In this backdrop, the Pd-based nanoparticles proved to be an excellent candidate in the coupling reaction for the formation of C–C bonds. Several coupling reactions such as Suzuki–Miyaura, Heck and Sonogashira reactions have been successfully conducted in the presence of Pd-nanocatalysts.12
The lanthanide-based nanoparticles (LNPs)13–15 are widely known for their optical properties and they find applications in bioimaging, optical sensing, image-guided therapy, biosensing, and optogenetics. Apart from biological applications, the LNPs have been utilized as efficient catalysts in organic synthesis.16–18 Therefore, in continuation of our research interest in lanthanide-based nanoparticles and C–C bond forming methodology development,19 we are interested in utilizing the LNP (LaF3·Pd)3 as a catalyst to carry out Suzuki coupling reactions and to our delight the LNP showed good catalytic activity and it was recovered from the reaction system and reused up to seven consecutive runs without significant loss of reactivity.
Results and discussion
Synthesis and characterization of LaF3·Pd nanocatalyst
The La–Pd bimetallic nanoparticles were prepared from the reaction of La2O3, Pd(OAC)2, and NH4F in the presence of 50% NH4OH at 120 °C in autoclave as displayed in Scheme 1.
 |
| Scheme 1 Preparation of the LaF3·Pd 5 at % nanoparticles. | |
XRD study
XRD patterns of synthesized LaF3·Pd is shown in Fig. 2. From the diffraction pattern it is obvious that the nanoparticles are highly crystalline and the diffraction peaks correspond to that of pure LaF3 hexagonal system (JCPDS No. 076-0510). The lattice parameters, cell volume and particle size calculated using Scherrer equation are given in the Table 1. The peaks from other phases were not detected in the as-prepared sample indicating that the as-prepared sample is pure. However the nanoparticles after used as catalyst some new peaks are appeared (marked with asterisk) indicating changes in the LaF3 nanoparticles. This change may be due to the formation of some LaF3 to LaFCO3 nanoparticle (JCPDS-074-0985) since K2CO3 is used in the reaction.
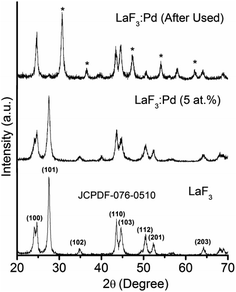 |
| Fig. 2 XRD pattern of LaF3·Pd. | |
Table 1 The lattice parameters, cell volume and particle size of LaF3·Pd
|
a (Å) |
b (Å) |
c (Å) |
Cell volume |
Particle size |
JCPDF-076-0510 |
4.148 |
4.148 |
7.354 |
109.58 |
|
LaF3 |
4.157 |
4.157 |
7.352 |
110.06 |
17.05 |
LaF3:Pd |
4.150 |
4.150 |
7.360 |
109.78 |
11.50 |
LaF3:Pd (after used) |
4.161 |
4.161 |
7.350 |
110.21 |
14.17 |
TEM image
The TEM image (Fig. 3b) of LaF3·Pd nanoparticles reveal that particles are spherical in shape with a diameter maximum about 8 nm (Fig. 3d). The high magnification TEM image (Fig. 3a) shows the lattice fringes of the nanoparticles corresponding to the lattice fringes (100) and (101) plane of hexagonal Lanthanum fluoride (JCPDS No. 076-0510). The lattice planes are also assigned in the SAED pattern Fig. 3c corresponding to the (100), (101), (110) and (112) plane. The lattice fringes in the HRTEM and SAED pattern indicated the well crystallinity of the particles.
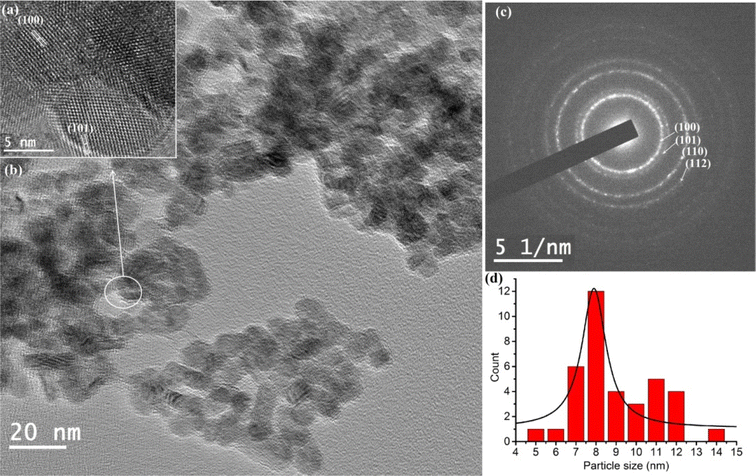 |
| Fig. 3 (a) HRTEM image (b) TEM image (c) SAED pattern (d) histogram of particle size of LaF3·Pd. | |
Palladium doped LaF3 nanoparticles for catalysis
From the X-ray photoelectron spectroscopy (XPS) spectrum in the Fig. 4a and b it clearly indicates the presence of La and Pd ions in the prepared nanoparticles. The La 3d binding energy around 853 and 837 eV of La 3d3/2 and La 3d5/2 respectively. From the Pd 3d scan the existence of metallic Pd(0) and Pd(II) is also confirmed where the binding energies of 3d5/2 are 336 eV, 337 eV and for that of 3d3/2 are 342 eV, 343 eV respectively. The PP at% are found to be La 3d5/2 = 0.37 at%, F 1s = 1.8 at%, Pd 3d3/2 = 0.09 and Pd 3d5/2 = 0.06 at% (Fig. 4).
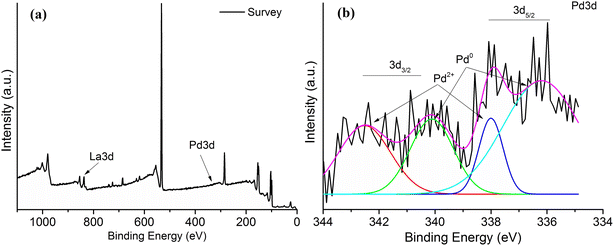 |
| Fig. 4 (a) X-ray photoelectron spectroscopy spectra (b) magnified XPS of LaF3·Pd. | |
Optimization of reaction condition using the LaF3·Pd nanocatalyst
With the LNP (LaF3·Pd) in hand, we were interested in exploring the catalytic efficiency of the LNP in Suzuki coupling. With this objective, we commenced the optimization study for the Suzuki coupling reaction by taking 4-bromophenol 1f (1.0 mmol), and phenylboronic acid 2a (1.5 mmol) as the standard substrates in the presence of 40 mg of the catalyst, in the presence of NaOH in aqueous medium under room temperature, pleasingly 3f was isolated in 81% yield (entry 1, Table 2). The structure of 3f was characterized by recording 1H and 13C NMR spectra. Then by keeping all the parameters same and varying the base such as KOH, K2CO3, Na2CO3, and Et3N the product 3f was isolated in 83%, 97%, 82%, and 84% yields respectively (entries 2–5, Table 2). From the screening of the bases the K2CO3 was found as the best choice. Next, to check the optimum amount of catalyst by keeping all the parameters the same and varying the catalyst loading, the amount of catalyst was decreased gradually from 30 mg to 15 mg and found that 15 mg is the optimum amount of catalyst needed for the current study (entries 6–8, Table 2). Then we turned our attention to check whether any other solvent can be employed to get enhanced yield and with less reaction time. So various solvents such as dioxane, DMSO, and DMF were screened by keeping the catalyst loading 15 mg and all other parameters constant (entries 9–11, Table 2), and found that no solvent gives better results than H2O. With this optimum condition for 3f (15 mg catalyst, H2O, K2CO3, RT for 1 hour), we turned our attention to study the substrate scope. Surprisingly, the optimum condition is not suitable for 3a (entry 1, Table 3).
Table 2 Optimization Table with 1f
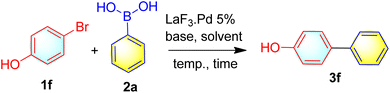
|
Entry |
Catalyst loading (mg) |
Base (equiv.) |
Solvent |
Temperature (°C) |
Time (hours) |
Yielda,b (%) |
Reaction conditions: 1f (1 mmol), 2a (1.5 mmol) in 3 mL of solvent. Isolated yield. |
1 |
40 |
NaOH (2) |
H2O |
RT |
1 |
81 |
2 |
40 |
KOH (2) |
H2O |
RT |
1 |
83 |
3 |
40 |
K2CO3 (2) |
H2O |
RT |
1 |
97 |
4 |
40 |
Na2CO3 (2) |
H2O |
RT |
1 |
82 |
5 |
40 |
Et3N (2) |
H2O |
RT |
1 |
84 |
6 |
30 |
K2CO3 (2) |
H2O |
RT |
1 |
97 |
7 |
20 |
K2CO3 (2) |
H2O |
RT |
1 |
97 |
8 |
15 |
K2CO3 (2) |
H2O |
RT |
1 |
97 |
9 |
15 |
K2CO3 (2) |
Dioxane |
RT |
1 |
90 |
10 |
15 |
K2CO3 (2) |
DMSO |
RT |
1 |
81 |
11 |
15 |
K2CO3 (2) |
DMF |
RT |
1 |
79 |
Therefore, optimum conditions were further investigated by taking bromobenzene 1a, and 2a. Under the study, the base, catalyst loading, and the solvent were kept constant, and increased the temperature from 50–100 °C (entries 1–5, Table 3). At 50 °C, the product 3a was isolated in 62% yield (entry 2, Table 3). Then with increase in temperature to 70 °C 3a was isolated in 85% yield (entry 3, Table 3) and further increase in temperature up to 100 °C improved the product yield further (entries 4 and 5, Table 3). Thus, 70 °C was found to be optimum temperature for the reaction (entry 3, Table 3). To our delight, this optimum condition worked well for all the substrates under the study.
Table 3 Optimization table with 1a
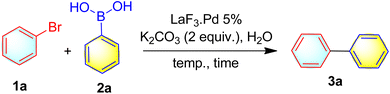
|
Entry |
Catalyst loading (mg) |
Base (equiv.) |
Solvent |
Temperature (°C) |
Time (hours) |
Yielda,b (%) |
Reaction conditions: 1a (1 mmol), 2a (1.5 mmol) in 3 mL of solvent. Isolated yield. |
1 |
15 |
K2CO3 (2) |
H2O |
RT |
40 |
8 |
2 |
15 |
K2CO3 (2) |
H2O |
50 |
4 |
62 |
3 |
15 |
K2CO3 (2) |
H2O |
70 |
4 |
85 |
4 |
15 |
K2CO3 (2) |
H2O |
80 |
4 |
85 |
5 |
15 |
K2CO3 (2) |
H2O |
100 |
4 |
85 |
Then, to check the electronic and steric effect, at first the bromobenzenes with electron donating substituents 1-bromo-4-methylbenzene 1b was reacted with 2a at 70 °C and 80% yield of the product was observed. Next, bromobenzene with 4-OMe and 3-OMe substituent were reacted with phenylboronic acid 2a and pleasingly the corresponding products 3c and 3d were isolated in 77% and 85% yields. Next, the 2-OH and 4-OH substituted bromobenzene were reacted with 2a. The respective biaryls 3e and 3f were isolated in 79% and 97% yields. Then the haloarenes with various electron withdrawing groups were tested. 4-CN substituted bromobenzene gave 75% yield of the corresponding biaryl 3g. Iodobenzene with 4-NO2 substitution resulted the corresponding biaryl 3h in 95% yield. Other electron withdrawing groups on the bromobenzene such as 2-CHO, 4-CHO, 3-CHO, 4-C(O)Me. 3-C(O)Me were also capable of resulting the corresponding biphenyls 3i, 3j, 3k, 3l, 3m in 82%, 93%, 75%, 85%, 88% yields respectively. Next, the fused biphenyls were also screened for synthesizing the biaryl systems. For this, 7-bromonaphthalen-2-ol and 1-bromonaphthalene were subjected to react with 2a. Pleasingle the corresponding fused aromatics based biaryls 3n and 3o were isolated in good yields (78% and 68% respectively). The heteroaromatic systems are important candidates in drug discovery, thus to check whether the present method can be applied for coupling with heteroaromatic halides, a reaction was performed between 2-bromo-4-methylpyridine and 2a. The corresponding coupled product 3p was isolated in 66% yield. After checking the substrate scope of haloarenes, we then turned our attention to check the substrate scope for the substituted phenylboronic acid. For that We have reacted 4-nitroiodobenzene with the phenylboronic acid with 4-Me and 4-OMe substitution and the expected coupled products 3q and 3r were obtained in 65% and 70% yield. Then, bromobenzene was reacted with the phenylboronic acid with 4-Me, 4-CF3 and 4-OMe substitutions and the respective coupled products 3s, 3t and 3u were isolated in 75%, 75% and 78% yields respectively. Next, 4-bromo-1,1′-biphenyl was reacted with 2a and pleasingly the coupled product 1,1′:4′,1′′-terphenyl 3v was isolated in 67% yield. Apart from the bromo and iodobenzene derivatives, we have also studied the scope of chloro aromatic and heteroaromatics. The 2-amino-5-chloropyridine and 4-chloroaniline were subjected to react with phenylboronic acid 2a in the developed reaction condition. But the coupled product 3w and 3x were not formed (Scheme 2).
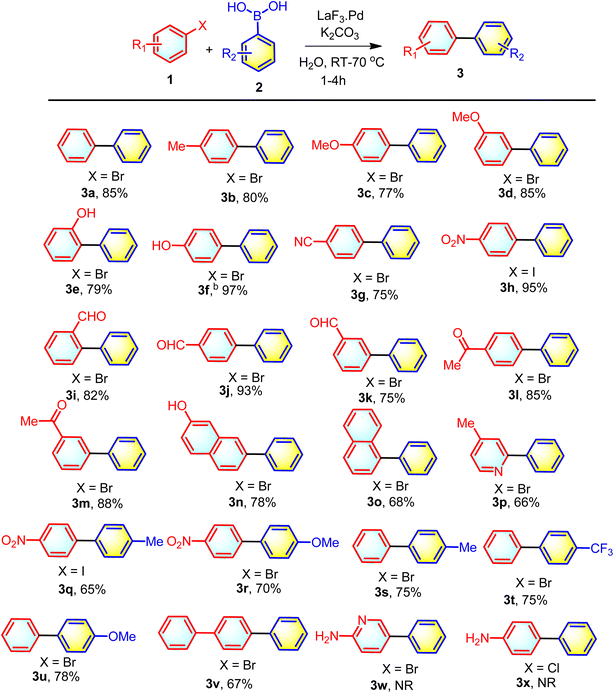 |
| Scheme 2 Substrate scope for the LaF3·Pd nanocatalyzed synthesis of biaryls. aReaction conditions: 1 (1.0 mmol), 2 (1.5 mmol), LaF3·Pd (15 mg), K2CO3 (2.0 mmol) and H2O (3.0 mL) were added in a 10 mL round-bottom flask and fitted with reflux condenser. The reaction mixture was stirred vigorously at 70 °C until the completion of the reaction (by TLC monitoring). bIn case of 3f the reaction was performed at room temperature. NR: no reaction. | |
Scale-up experiment
To further check, whether the present method can be applied to synthesize the biaryls in large scale, a reaction was performed by taking the 4-bromophenol 1f (10 mmol), phenylboronic acid 2a (15 mmol) at room temperature. To our delight, the corresponding biaryl 3f was isolated in 95% yield (1.6 g) (Scheme 3).
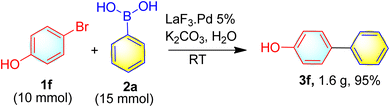 |
| Scheme 3 Gram scale synthesis of 3f. | |
Recyclability test
The nanocatalysts in recent years are becoming popular for their recyclability up to several cycles. Also owing to easy to handle, sustainable and green aspect, they are becoming popular in industries. Thus to check whether the present nanocatalyst LaF3·Pd can be recycled and reused, the reaction was performed by taking 1a (2.0 mmol) and 2a (3.0 mmol) in the presence of 30 mg of the catalyst under the optimized reaction condition. After completion of the reaction, the catalyst was recovered by centrifuge and dried and further utilized in the synthesis of 3a. Pleasingly, the catalyst was recovered and reused for seven consecutive cycles without significant loss in the catalytic activity and the product yield (Table 4).
Table 4 Recyclability of the catalyst and the isolated yields
No. of runs |
Catalyst used |
Catalyst recovered |
Yields (%) |
1st run |
30 mg |
28 mg |
84 |
2nd run |
28 mg |
27 mg |
85 |
3rd run |
27 mg |
25 mg |
83 |
4th run |
25 mg |
24 mg |
84 |
5th run |
24 mg |
22 mg |
83 |
6th run |
22 mg |
21 mg |
82 |
7th run |
21 mg |
19 mg |
82 |
Comparison
The nanocatalyzed Suzuki reaction is getting attention from synthetic chemists owing to its green aspects such as reusability. In this regard, there are many reports frequently published on the nanocatalyzed C–C bond formation by Suzuki coupling. Thus, to check the efficacy of our catalyst LaF3·Pd with the recent reported literature, we summarized the results of various nanocatalyzed Suzuki reactions with the present work (Table 5).20–34 It is clear from the table that the present catalyst is efficient enough for the synthesis of biaryls in terms of product yield and reaction time and also this can be recycled and reused.
Table 5 Comparison of recently reported works with the present work
Sl. no |
Catalyst |
Reaction condition |
Time |
Yield |
Ref. |
1 |
Nano-Fe3O4@CA-Pd |
K2CO3, EtOH : H2O, 75 °C |
25 min |
Trace-98% |
20 |
2 |
Fe3O4@SiO2–NHC–Pd(Π) NPs |
K2CO3, H2O, 60 °C |
1–16 h |
69–97% |
21 |
3 |
5%Pd/TiO2, anatase type |
Cs2CO3, DMA, 80 °C, under Ar, sealed, 1000 rpm |
24 h |
42–98% |
22 |
4 |
Fe3O4@SiO2-NMIM-Pd |
K2CO3, EtOH, 80 °C |
1.5–5 h |
27–97% |
23 |
5 |
Ni-TC@ASMNP |
K3PO4, dioxane, PPh3, 100 °C |
10–15 h |
75–97% |
24 |
8 |
Pd NPs@APC |
K2CO3, solvent-free, microwave |
5 min |
52–99% |
25 |
9 |
Fe3O4@SiO2–Pd |
CaO, EtOH : H2O (1 : 1), 85 °C |
20–70 min |
87–96% |
26 |
10 |
Fe3O4@NC/Pd |
KOH, H2O, 90 °C |
0.5 h |
53–99% |
27 |
11 |
ZrO2@AEPH2-PPh2-Pd(0) |
K2CO3, H2O, 80 °C |
20 min–24 h |
Trace-95% |
28 |
12 |
Fe3O4@boehmite-NH2-CoII NPs |
KOH, H2O, 80 °C |
25 min–9 h |
15–95% |
29 |
13 |
Cu–ninhydrin@GO–Ni MNPs |
Na2CO3, H2O, 80 °C |
50 min–7 h |
89–97% |
30 |
14 |
Pd/CuFe2O4 nanowires |
K2CO3, DMSO, 100 °C |
10 min–12 h |
27–99% |
31 |
15 |
Pd-SBT@MCM-41 |
PEG-400, 80 °C |
1–5 h |
87–98% |
32 |
16 |
Pd@CQD@Fe3O4 NPs |
t-BuOK, EtOH : H2O (1 : 1) 60–120 °C |
2–24 h |
88–100% |
33 |
17 |
Pd@4PVP |
K3PO4, EtOH/H2O (3 : 1) 80 °C |
3–18 h |
29–99% |
34 |
18 |
LaF3·Pd nanocatalyst |
K2CO3, H2O, RT-70 °C |
1–4 h |
66–97% |
This work |
Conclusion
To summarize, we synthesized the LaF3·Pd 5% nanocatalyst and characterized by XRD and TEM analysis. To check the catalytic efficiency of the synthesized nanocatalyst, we applied the nanocatalyst for the synthesis of biaryls via Suzuki coupling in the aqueous medium, a greener condition. To our delight, the catalyst is efficient enough to result the products in good to excellent yields within 1–4 hours. Also, the catalyst was recycled from the reaction medium and reused for seven consecutive runs without any significant decrease in the catalytic activity and product yield. This green and sustainable aspect of the present method meets the requirement of industrial applications. Thus, we believe the present report will be a valuable addition to the green and sustainable methodologies in synthetic organic transformation.
Data availability
The data that support the finding of the present study are available in ESI‡ of this article.
Conflicts of interest
There are no conflicts to declare.
Acknowledgements
B. B. P. is thankful to SERB (SRG/2019/002032), New Delhi, S&T Department Project-Odisha (ST-SCST-0061/2018/2724), UGC (F. 30-484/2019) (BSR) and G. P. is thankful to OURIIP-Odisha for funding. S. S. A. is thankful to CSIR India for junior research fellowship. The authors are grateful to Berhampur University for infrastructure. We acknowledge IISER Berhampur for NMR and sample characterization facilities. The authors are thankful to Alisha Rani Tripathy and Akash Bisoyi of IISER Thiruvananthapuram for their timely help.
References
-
(a) N. N. Rao, B. B. Parida and J. K. Cha, Org. Lett., 2014, 16, 6208–6211 CrossRef CAS PubMed;
(b) B. B. Parida, P. P. Das, M. Niocel and J. K. Cha, Org. Lett., 2013, 15, 1780–1783 CrossRef CAS PubMed;
(c) B. B. Parida, I. L. Lysenko and J. K. Cha, Org. Lett., 2012, 14, 6258–6261 CrossRef CAS PubMed.
-
(a) S. Chandrasekhar, B. B. Parida and C. Rambabu, J. Org. Chem., 2008, 73, 7826–7828 CrossRef CAS PubMed;
(b) B. P. Raiguru, J. Panda, S. Mohapatra and S. Nayak, J. Mol. Struct., 2023, 1294, 136282 CrossRef CAS.
-
(a) S. S. Acharya, S. Patra, R. Maharana, M. Dash, L. M. Barad and B. B. Parida, Org. Biomol. Chem., 2024, 22, 2916–2947 RSC;
(b) A. Saha, M. Shankar, S. Sau and A. K. Sahoo, Chem. Commun., 2022, 58, 4561–4587 RSC;
(c) S. S. Panda and N. K. Sharma, Org. Biomol. Chem., 2023, 21, 1468–1477 RSC.
- A. Biffis, P. Centomo, A. D. Zottom and M. Zecca, Chem. Rev., 2018, 118, 2249–2295 CrossRef CAS PubMed.
-
(a) T. Niwa, Y. Uetake, M. Isoda, T. Takimoto, M. Nakaoka, D. Hashizume, H. Sakurai and T. Hosoya, Nat. Catal., 2021, 4, 1080–1088 CrossRef CAS;
(b) I. Hussain, J. Capricho and M. A. Yawer, Adv. Synth. Catal., 2016, 358, 3320–3349 CrossRef CAS;
(c) S. E. Hooshmand, B. Heidari, R. Sedghi and R. S. Varma, Green Chem., 2019, 21, 381–405 RSC;
(d) A. Hassan, A. S. Baghel, A. Kumar and N. Das, Chem.–Asian J., 2023, e202300778 Search PubMed;
(e) S. S. Acharya and B. B. Parida, ChemistrySelect, 2024, 9, e202305233 CrossRef.
-
(a) S. Yuan, J. Chang and B. Yu, Top. Curr. Chem., 2020, 23, 378, DOI:10.1007/s41061-020-0285-9;
(b) S. S. Acharya, S. Patra, L. M. Barad, A. Roul and B. B. Parida, New J. Chem., 2024, 48, 7614–7638 RSC;
(c) M. C. Kozlowski, B. J. Morgan and E. C. Linton, Chem. Soc. Rev., 2009, 38, 3193–3207 RSC;
(d) G. Bringmann, T. Gulder, T. A. M. Gulder and M. Breuning, Chem. Rev., 2011, 111, 563–639 CrossRef CAS PubMed;
(e) G. Bringmann, T. Pabst, P. Henschel, J. Kraus, K. Peters, E.-M. Peters, D. S. Rycroft and J. D. Connolly, J. Am. Chem. Soc., 2000, 122, 9127–9133 CrossRef CAS.
-
(a) J. Wencel-Delord, A. Panossian, F. R. Leroux and F. Colobert, Chem. Soc. Rev., 2015, 44, 3418–3430 RSC;
(b) J. A. Ashenhurst, Chem. Soc. Rev., 2009, 39, 540–548 RSC.
- P. Wu, T. E. Nielsen and M. H. Clausen, Drug Discovery Today, 2016, 21, 5–10 CrossRef CAS PubMed.
-
(a) Y. Zhu, W. Dong and W. Tang, Adv. Agrochem, 2022, 1, 125–138 CrossRef;
(b) P. Devendar, R.-Y. Qu, W.-M. Kang, B. He and G.-F. Yang, J. Agric. Food Chem., 2018, 66, 8914–8934 CrossRef CAS PubMed.
-
(a) Z.-R. Li, F.-Z. Suo, B. Hu, Y.-J. Guo, D.-J. Fu, B. Yu, Y.-C. Zheng and H.-M. Liu, Bioorg. Chem., 2019, 84, 164–169 CrossRef CAS PubMed;
(b) C. N. Johnson, D. A. Erlanson, C. W. Murray and D. C. Rees, J. Med. Chem., 2017, 60, 89–99 CrossRef CAS PubMed;
(c) Y. Duan, Y. Guan, W. Qin, X. Zhai, B. Yu and H. Liu, MedChemComm, 2018, 9, 1779–1802 RSC;
(d) B. Yu, X.-J. Shi, Y.-F. Zheng, Y. Fang, E. Zhang, D.-Q. Yu and H.-M. Liu, Eur. J. Med. Chem., 2013, 69, 323–330 CrossRef CAS PubMed.
-
(a) R. S. Varma, ACS Sustainable Chem. Eng., 2016, 4, 5866–5878 CrossRef CAS PubMed;
(b) R. A. Sheldon, Chem. Soc. Rev., 2012, 41, 1437–1451 RSC;
(c) L. L. Chng, N. Erathodiyil and J. Y. Ying, Acc. Chem. Res., 2013, 46, 1825–1837 CrossRef CAS PubMed;
(d) H. Ahmad and M. K. Hossain, Mater. Adv., 2022, 3, 859–887 RSC.
-
(a) A. Balanta, C. Godard and C. Claver, Chem. Soc. Rev., 2011, 40, 4973–4985 RSC;
(b) A. Fihri, M. Bouhrara, B. Nekoueishahraki, J.-M. Basset and V. Polshettiwar, Chem. Soc. Rev., 2011, 40, 5181–5203 RSC;
(c) R. Narayanan, Molecules, 2010, 15, 2124–2138 CrossRef CAS PubMed;
(d) C. K. Pal, A. K. Jena, D. Das, S. Sahu and R. K. Singh, ChemistrySelect, 2021, 6, 7944–7949 CrossRef.
- P. Du, R. An, Y. Liang, P. Lei and H. Zhang, Coord. Chem. Rev., 2022, 471, 214745 CrossRef CAS.
- Z. Zhang, Q. Han, J. W. Lau and B. Xing, ACS Mater. Lett., 2020, 2, 1516–1531 CrossRef CAS.
- S. Y. Lee, M. Lin, A. Lee and Y. I. Park, Nanomaterials, 2017, 7, 411 CrossRef PubMed.
-
(a) S. Moradi, J. Safari and M. Moradian, ChemistrySelect, 2024, 9, e202302750 CrossRef CAS;
(b) M. Daraie, M. Mirzaei, M. Bazargan, V. S. Amiri, B. A. Sanati and M. M. Heravi, Sci. Rep., 2022, 12, 12004 CrossRef CAS PubMed.
- M. U. Khan and Z. A. Siddiqui, ACS Omega, 2018, 3, 10357–10364 CrossRef CAS PubMed.
-
(a) F. Ding, Y. Li, P. Yan, Y. Deng, D. Wang, Y. Zhang, I. Dragutan, V. Dragutan and K. Wang, Molecules, 2018, 23, 2435 CrossRef PubMed;
(b) A. B. Yousaf, M. Imran, M. Farooq and P. Kasak, Sci. Rep., 2018, 8, 4354 CrossRef PubMed.
-
(a) S. K. Swain, G. Phaomei, S. K. Tripathy, N. Yaiphaba, R. B. Devi, S. Nayak and B. B. Parida, J. Mol. Struct., 2022, 1247, 131330 CrossRef CAS;
(b) S. K. Choudhury, P. Rout, B. B. Parida, J.-C. Florent, L. Johannes, G. Phaomei, E. Bertounesque and L. Rout, Eur. J. Org Chem., 2017, 5275–5292 CrossRef CAS;
(c) S. K. Swain, A. Sahoo, S. K. Swain, S. K. Tripathy and G. Phaomei, Dalton Trans., 2020, 49, 14605–14612 RSC;
(d) L. Rout, B. B. Parida, J.-C. Florent, L. Johannes, S. K. Choudhury, G. Phaomei, J. Scanlon and E. Bertounesque, Chem.–Eur. J., 2016, 22, 14812–14815 CrossRef CAS PubMed.
- E. Ghonchepour, M. R. Islami and A. M. Tikadri, J. Organomet. Chem., 2019, 883, 1–10 CrossRef CAS.
- N. Y. Baran, T. Baran, M. Nasrollahzadeh and R. S. Varma, J. Organomet. Chem., 2019, 900, 120916 CrossRef CAS.
- T. Yamada, H. Masuda, K. Park, T. Tachikawa, N. Ito, T. Ichikawa, M. Yoshimura, Y. Takagi, Y. Sawama, Y. Ohya and H. Sajiki, Catalysts, 2019, 9, 461 CrossRef CAS.
- Y. Dong, F. Xue and Y. Wei, J. Phys. Chem. Solids, 2021, 153, 110007 CrossRef CAS.
- R. K. Sharma, M. Yadav, R. Gaur, Y. Monga and A. Adholeya, Catal. Sci. Technol., 2015, 5, 2728–2740 RSC.
- T. Baran, Carbohydr. Polym., 2018, 195, 45–52 CrossRef CAS PubMed.
- A. Khazaei, M. Khazaei and M. Nasrollahzadeh, Tetrahedron, 2017, 73, 5624–5633 CrossRef CAS.
- K. Zheng, C. Shen, J. Qiao, J. Tong, J. Jin and P. Zhang, Catalysts, 2018, 8, 443 CrossRef.
- N. Razavi, B. Akhlaghinia and R. Jahanshahi, Catal. Lett., 2017, 147, 360–373 CrossRef CAS.
- A. Mohhammadinezhad and B. Akhlaghinia, Green Chem., 2017, 19, 5625–5641 RSC.
- P. Moradi and M. Hajjami, RSC Adv., 2021, 11, 25867–25879 RSC.
- B. Lakshminarayana, J. Chakraborty, G. Satyanarayana and C. Subrahmanyam, RSC Adv., 2018, 8, 21030–21039 RSC.
- M. Nikoorazm, A. G. Choghamarani, M. Ghobadi and S. Massahi, Appl. Organomet. Chem., 2017, e3848 CrossRef.
- M. Gholinejad, F. Zareh and C. Najera, Appl. Organomet. Chem., 2018, 32, e3984 CrossRef.
- F. Masing, H. Nusse, J. Klingauf and A. Studer, Org. Lett., 2018, 20, 752–755 CrossRef PubMed.
Footnotes |
† The manuscript is dedicated to 70th Birth Anniversary of Prof. Satyaban Jena (Former Professor, Department of Chemistry, Utkal University, Odisha). |
‡ Electronic supplementary information (ESI) available. See DOI: https://doi.org/10.1039/d4ra00686k |
§ Equal contribution in the work. |
|
This journal is © The Royal Society of Chemistry 2024 |