DOI:
10.1039/D4RA00399C
(Paper)
RSC Adv., 2024,
14, 10516-10525
Preparation of a novel antibacterial magnesium carbonate coating on a titanium surface and its in vitro biocompatibility
Received
15th January 2024
, Accepted 25th March 2024
First published on 2nd April 2024
Abstract
Magnesium-based coatings have attracted great attention in surface modification of titanium implants due to their superior angiogenic and osteogenic properties. However, their biological effects as a carbonate-based constituent remain unrevealed. In this study, magnesium carbonate coatings were prepared on titanium surfaces under hydrothermal conditions and subsequently treated with hydrogen peroxide. Also, their antibacterial activity and in vitro cell biocompatibility were evaluated. The obtained coatings consisted of nanoparticles without cracks and exhibited excellent adhesion to the substrate. X-ray diffraction (XRD) results indicated pure magnesium carbonate coatings formed on the Ti surface after hydrothermal treatment. After hydrogen peroxide treatment, the phase composition of the coatings had no obvious change. Compared to the untreated coatings, the hydrogen peroxide-treated coatings showed increased surface roughness and hydrophilicity. Co-culture with Staphylococcus aureus (S. aureus) demonstrated that the obtained coatings had good antibacterial activity. In vitro cell culture results showed that the hydrogen peroxide-treated coatings enhanced the viability, proliferation, and osteogenic differentiation of bone marrow mesenchymal stem cells (BMSCs). These findings suggest that this MgCO3-based coating exhibits excellent antibacterial performance and osteogenic potential. Based on the above, this study provides a simple method for preparing titanium implants with dual antibacterial and osteogenic capabilities, holding great promise in clinical applications.
Introduction
According to a statistical survey, there are over 2 million bone transplantation surgeries performed globally each year,1 with a steady increase in the usage of bone repair-related implants. It has been reported that an estimated 2–5% of these implants may occur with infection in clinical application.2 The primary pathogen responsible for implant-related infections is often S. aureus.3–5 In cases of treatment failure, extreme measures such as amputation may be necessary, and in severe instances, it can even lead to fatal outcomes.6 To address this issue, numerous technologies have been developed to impart antimicrobial properties to implant surfaces.
Titanium has been widely used in bone repair and achieved general satisfactory effects in clinical application due to its superior physiochemical properties.7–9 However, in some compromised situations, including infections and poor osseointegration, there is a higher risk of implant failure.10,11 Therefore, the preparation of biofunctional titanium implants with antibacterial and osteogenic capabilities is highly desirable in the field of biomedical engineering. To enhance the antimicrobial activity, for instance, Schmidmaier et al. loaded gentamicin-poly(lactic-co-glycolic acid) coatings onto intramedullary nails made of titanium (Ti).12 This implant has received approval for use in Europe, and clinical studies have reported a significant reduction in infection rates compared with pure Ti nails.13 However, it is susceptible to inducing bacterial resistance.14 Studies have shown that inorganic metal ions such as silver (Ag), copper (Cu), and magnesium (Mg) exhibit potential antimicrobial properties without promoting antibiotic resistance.15–18 Especially for Mg, as the fourth most abundant metal element in the human body, it is considered safe and demonstrates excellent biocompatibility, biodegradability and antimicrobial activity.19–21 Research has demonstrated that Mg ions have the potential to upregulate the expression of integrins α2 and α3, thereby mediating the proliferation and osteogenic differentiation of human bone marrow mesenchymal stem cells (hBMSCs).22 Furthermore, magnesium ions can enhance the adhesion of osteoblasts onto biomaterials by promoting integrin expression.23,24 Moreover, Mg ions exhibit antibacterial activity probably due to their alkaline properties.25,26 Thus, many studies have focused on preparation of Mg ion-based coatings such as magnesium hydroxide and magnesium oxide to enhance the antimicrobial and osteogenic properties of the implants.27–29 In contrast, magnesium carbonate is predominantly utilized for gastrointestinal protection and mitigating stomach ailments,30,31 with limited exploration into its potential for bone formation and related aspects. Recent studies suggest that magnesium carbonate, owing to its alkaline nature, shows promising antibacterial properties.32 Additionally, its role as a magnesium ion-releasing agent has been proposed to promote bone formation.33
The decomposition of hydrogen peroxide generates reactive oxygen species (ROS) and other free radicals, which exhibit a broad-spectrum antibacterial activity.34–36 Some studies have also suggested that exogenous hydrogen peroxide stimulates the expression of VEGF in a dose-dependent manner in rat and murine in vitro models, thereby promoting vascular formation and wound healing.37 Ohlin et al. found that incubating titanium with 30% H2O2 at room temperature for 15 minutes significantly inhibited bacterial growth and reduced their viability.38 Furthermore, related research indicates that hydrogen peroxide-induced modification of the titanium surface leads to the formation of various micro/nanostructures and alters its wettability. These changes significantly affect the adhesion, proliferation, and osteogenic differentiation of osteoblasts, thereby enhancing bone formation.39,40 In addition, this modification simultaneously enhances osteogenesis while imparting antibacterial capabilities to the titanium surface.41
Therefore, the aim of this study is to develop a technique for coating titanium (Ti) with magnesium carbonate, and subsequently modify it with hydrogen peroxide to enhance its antibacterial activity. Also, the biological function of this coating was evaluated by co-culture with BMSCs.
Materials and methods
Coating preparation
Medical-grade pure titanium sheets (10 mm × 10 mm × 1.2 mm; Xingye Metal, Qinghe, China) were polished with silicon carbide paper up to a grit size of 1200 and etched in a mixture of 0.5 M nitric acid (Chengdu Kelon, China) and 0.3 M hydrofluoric acid (Chengdu Kelon, China) for 30 s, followed by rinsing in ultrapure water (18.2 MW cm). Then, the samples were subjected to ultrasonic treatment in a sequence of acetone (Chengdu Kelon, China), ethanol (Chengdu Kelon, China), and distilled water for 10 min, respectively. Subsequently, the samples were immersed into 60 mL of the solution containing of 0.05 M Mg(NO3)2·6H2O (Chengdu Kelon, China) and urea (2 g) (Chengdu Kelon, China). The mixture were transferred into a Teflon vessel and reacted at 180 °C for 12 hours. After cooling to room temperature, the samples were collected and thoroughly cleaned in ultrapure water under ultrasonic treatment for 10 min. Finally, the samples were dried at 60 °C for further use. The obtained samples were denoted as Ti–Mg, while the pristine Ti was denoted as Ti. For further treatment, the samples were subjected to 30% hydrogen peroxide treatment for 20 min, followed by drying at room temperature. These treated samples were denoted as Ti–H2O2 and Ti–Mg–H2O2, respectively.
Coating characterizations
The surface structure and elemental composition were studied using a scanning electron microscope (FEI-Quanta FEG 250, USA) and energy-dispersive X-ray spectroscopy (EDS). Crystal structure was investigated using XRD (FEI-Quanta FEG 250, USA). Surface hydrophilicity was measured using a contact angle analyzer (OCA40micro, Germany). Surface roughness was characterized using an atomic force microscope (AFM) (BRUKER Dimension Icon, Germany). After immersing the samples in 1 mL of calcium- and magnesium-free phosphate-buffered saline (PBS) for 21 days, the supernatant was collected and analyzed using an atomic absorption spectrophotometer (model AA-7000, manufactured by Shimadzu). To evaluate the adhesion of the coatings to the titanium substrate, a tape test was conducted following the ASTM International standard D3359.42,43
Bacterial inhibition
The test samples were evaluated for their inhibition of S. aureus (BNCC186335; BeNa Culture Collection). In brief, 0.5 mL of bacterial suspension (BNBio, Beijing, China) was transferred to 5 mL of pre-sterilized (121 °C for 15 minutes) liquid culture medium (containing 10.0 g of peptone, 5.0 g of beef extract, 5.0 g of sodium chloride, and 1000 mL of water, all from SolarBio, Beijing, China), and thoroughly mixed. The resulting liquid was streaked onto agar culture medium (100 mL liquid culture medium and 14.0 g of agar) and incubated at 37 °C for 24 hours. Afterwards, a single bacterial colony was selected, transferred to 5 mL of sterile liquid culture medium, and cultured in a shaking incubator (37 °C, 150 rpm) for 10 hours. The bacterial suspension was then diluted to a concentration of 105–107 CFU mL−1 for further use.
Subsequently, the Ti-based samples were sterilized by UV irradiation for 1 hour and placed in a 24-well culture plate. Next, 100 μL of the bacterial suspension was added onto the surface of the samples and incubated at 37 °C for 1 hour, followed by the addition of 1 mL of sterile liquid culture medium to each well. After culture for 6 hours, 100 μL of the diluted suspension was spread on agar plates. After incubating at 37 °C for 12 hours, bacterial growth on the culture plates was visually inspected and counted.
To assess the bacterial status on the samples, 100 μL of bacterial suspension (at a concentration of 105–107 CFU mL−1) was added to the sample surfaces placed in a 24-well culture plate. After incubating for 20 minutes, 1 mL of sterile liquid culture medium was added to each well, and further incubation was carried out in a shaking incubator (37 °C, 150 rpm) for an additional 12 hours. Live and dead bacteria were observed using a dual staining kit (Shanghai Maokang, China) under a fluorescence inverted microscope (Leica, Germany).
After 12 hours of incubation, bacterial respiratory activity was assessed using the fluorescent oxidative-reduction dye 5-cyano-2,3-ditolyl tetrazolium chloride (CTC, Shanghai Maokang, China). Stained bacteria were observed under a fluorescence inverted microscope.
Cytocompatibility
The Ti-based samples were sterilized using UV irradiation for 1 h and placed in a 24-well culture plate The extraction of rat bone marrow mesenchymal stem cells (rBMSCs) was performed using Sprague-Dawley (SD) rats obtained from the Experimental Animal Center at North Sichuan Medical College (Nanchong, Sichuan, China).44 After three passages, rBMSCs (1 × 104 cells per well) were seeded onto different types of discs (Ti, Ti–H2O2, Ti–Mg, Ti–Mg–H2O2) and cultured in α-minimum essential medium (α-MEM, Gibco, USA) supplemented with 10% fetal bovine serum (FBS, Gibco, USA) and 1% penicillin–streptomycin (PS, HyClone, USA). The culture medium was refreshed every two days, and cells were maintained at 37 °C in a 5% CO2 incubator.
Cell viability were assessed after 1 and 4 days of culture using a dual staining kit for live and dead cells (Abbkine, China).45 Stained cells were observed using an inverted fluorescence microscope (Leica, Germany). Additionally, on days 1, 4, and 7, cell proliferation was quantified using the CCK-8 assay kit (Vazyme) by measuring absorbance at 450 nm with a microplate reader (Thermo Fisher Scientific, Waltham, USA).
On day 3, the culture medium was switched to osteogenic induction medium, which consisted of 10% FBS, 50 μg mL−1 ascorbic acid (Sigma, USA), 10 mM β-glycerophosphate (Sigma), and 10 nM dexamethasone (Sigma, USA). The culture medium was renewed every two days during the cell culture period. After 14 days of culture, alkaline phosphatase (ALP) activity was measured using the ALP microplate assay kit (Beyotime, China) and normalized to total protein content determined with the BCA protein assay kit (Beyotime, China). ALP staining was carried out using the ALP staining kit (Solarbio, China) and observed under a stereomicroscope.
Following 21 days of culture, samples were stained with Alizarin Red S (OriCell, China) and examined under a stereomicroscope. Subsequently, 10% hexadecylpyridinium chloride was added to each well to dissolve the calcium nodules. Once the calcium nodules were fully dissolved, 100 μL of the solution was extracted, and absorbance was measured at a wavelength of 562 nm using a microplate reader.46
After 14 days of culture, the expression levels of four bone-related proteins [ALP, osteopontin (OPN), osteocalcin (OCN), runt-related transcription factor 2 (Runx2)] were quantified using the SYBR Green Q-PCR kit and following standard protocols (Vazyme, Nanjing, China).47 The relative gene expression levels were calculated using the 2-ΔΔCt method,47 with glyceraldehyde-3-phosphate dehydrogenase (GAPDH) as the reference gene (Table 1).
Table 1 Sequences of primers for bone-related genes
Gene |
Forward primer (5\-3\) |
Reverse primer (5\-3\) |
ALP |
GCAGGCAAGACACAGACT |
TGGAGG AGAGAAGGTCAG AT |
OPN |
CTTGAGCATTCCAAAGAGAGC |
CTTGTGGCTGTGAAACTT GTG |
OCN |
ACCGCCTACAAACGCATCTA |
AGAGGACAGGGAGGATCAAGT |
Runx-2 |
GCAGCACGCTATTAA ATCCAA |
GCCAAACAGACT CAT CCA TTC |
GAPDH |
CAGTGGCAAAGTGGAGATTGTTG |
TCGCTCCTGGAAGATGGTGAT |
Statistical analysis
The data is presented as the mean ± standard deviation and analyzed using one-way analysis of variance (ANOVA) followed by Tukey's post hoc multiple comparison test. Values with p < 0.05 are considered to have statistical significance.
Results
The coating morphology and phase composition
SEM images demonstrated that after undergoing sandblasting and acid-etching treatments, all samples exhibited some scratches and pits on their surfaces at low magnification (as shown in Fig. 1A), with no significant differences among them. However, at higher magnification (Fig. 1B), the surfaces of the original Ti and Ti–Mg–H2O2 samples appeared relatively smooth, exhibiting only slight traces of polishing. In contrast, the surfaces of Ti–Mg and Ti–Mg–H2O2 displayed uniformly distributed nanoparticles without any cracks. EDS analysis confirmed the presence of Ti, O, C, and Mg on the surfaces of both Ti–Mg and Ti–Mg–H2O2 (Fig. 1C and D). In the XRD study presented in Fig. 2, distinct diffraction peaks at 32.5°, 42.9°, and 53.9° were attributed to the (104), (113), and (116) crystal planes of magnesium carbonate (PDF #08-0479). Notably, the peaks of Ti–Mg and Ti–Mg–H2O2 are consistent with the diffraction pattern of standard magnesium carbonate, which were in agreement with the XRD results of magnesium carbonate powder reported by Liang et al.48 To examine the cross-section of the coatings, Ti foil was utilized as a substrate, followed by the same treatments and subsequent fracturing. SEM images (Fig. 1E) revealed two distinct layers vertically along the cross-sections: the bottom layer corresponding to the Ti substrate and the top layer to the Mg-based coating. The Ti–Mg–H2O2 coating was found to be dense and tightly adhered to the titanium substrate, with no evidence of separation or delamination (Fig. 1E). The EDS spectra (Fig. 1E) indicated an increase in the distribution of Mg, O, and C in the upward vertical direction and a decrease in Ti distribution, suggesting in situ growth of the Mg-based coating on the Ti matrix.
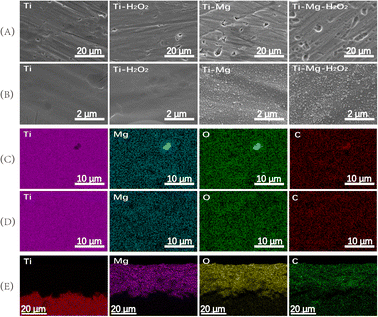 |
| Fig. 1 SEM images of different Ti-based samples: (A) ×5000, (B) ×50 000; the elements distribution on the surface of (C) Ti–Mg sample and (D) Ti–Mg–H2O2 sample; the elements distribution on the cross-section of (E) Ti–Mg–H2O2 sample. | |
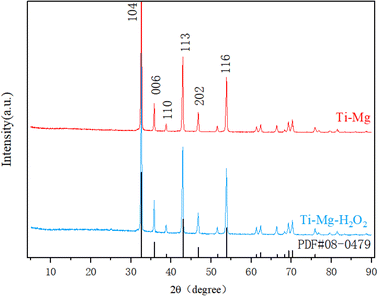 |
| Fig. 2 XRD spectra were obtained from powders derived from solutions of Ti–Mg and Ti–Mg–H2O2. | |
The coating thickness of the Ti–Mg–H2O2 sample was measured to be approximately 18 μm, indicating a relatively thin coating layer.
Surface wettability and roughness
Wettability analysis (Fig. 3A) revealed that the average water and contact angle on the Ti surface was 94.3 ± 3.6°, while on the Ti–H2O2 surface, it was significantly reduced to 82.2 ± 1.7°. This notable difference between the two samples (p < 0.05) suggests that H2O2 treatment enhances the hydrophilicity of the Ti surface to a certain extent. After the deposition of the coating, the water contact angle decreased significantly, with the Ti–Mg sample showing an angle of 65.9 ± 4.9° and the Ti–Mg–H2O2 sample having an angle of 42.1 ± 4.1° (p < 0.05) (Fig. 3B). These results indicate that the coating deposition improved hydrophilicity, and H2O2 treatment further enhanced this property.
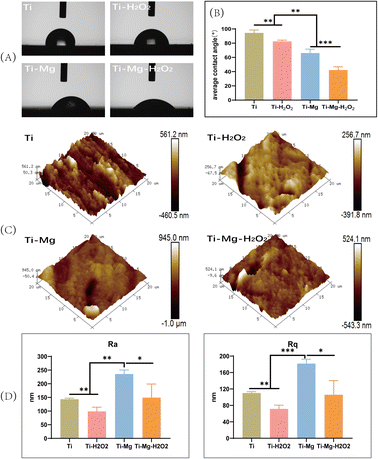 |
| Fig. 3 (A) Experimental photographs of water contact angles for different samples; (B) quantitative calculation of water contact angles for different samples; (C) AFM of different samples; (D) Ra Rq values for different samples (* indicates p < 0.05; ** indicates p < 0.01,*** indicates p < 0.001). | |
Surface roughness was characterized using Atomic Force Microscopy (AFM) (Fig. 3C and D). All materials exhibited similar topography with various pits and protrusions. Arithmetic mean surface roughness (Ra) and root mean square roughness (Rq) measurements showed that Ra values for Ti, Ti–H2O2, Ti–Mg, and Ti–Mg–H2O2 were 143.3 ± 3.2 nm, 98.7 ± 12.5 nm, 235.6 ± 12.5 nm, and 149.0 ± 40.7 nm, respectively, while Rq values were 110.0 ± 2.8 nm, 71.3 ± 7.5 nm, 181.6 ± 8.8 nm, and 105.8 ± 28.1 nm, respectively. Statistical analysis indicated that the Ra and Rq values of the Ti–Mg–H2O2 group were significantly different (p < 0.05) from the other groups, suggesting a rougher surface for the Ti–Mg–H2O2 group. Additionally, from a more microscopic perspective, the surfaces of the Ti and Ti–H2O2 groups appeared relatively smooth, while the Ti–Mg and Ti–Mg–H2O2 groups exhibited a higher density of nanoscale particles.
Coating adhesion
To evaluate the adhesion of the coatings on the titanium (Ti) substrates, a tape test was conducted according to the ASTM International Standard D3359. As shown in Fig. 4A, visual inspection indicated no visible residues on the tape surface. Based on the ASTM D3359 rating scale, both Ti–Mg and Ti–Mg–H2O2 group coatings were graded as 5B, indicating no visible peeling. These results demonstrate that the coatings on the Ti–Mg and Ti–Mg–H2O2 samples exhibit excellent adhesion to the titanium substrates.
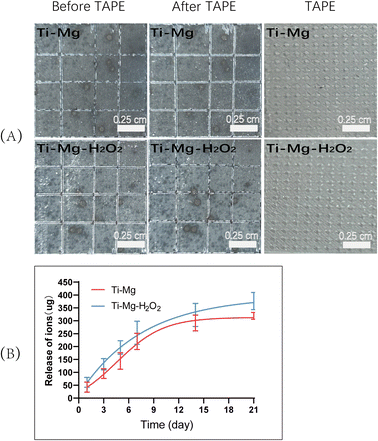 |
| Fig. 4 (A) Adhesion strength of the coatings on Ti–Mg and Ti–Mg–H2O2; (B) Mg2+ release curves for Ti–Mg and Ti–Mg–H2O2. | |
Magnesium ion release detection
To assess the release of ions from the coatings, the Ti–Mg and Ti–Mg–H2O2 groups were immersed in a calcium- and magnesium-free PBS solution. Over 21 days, the release trends of magnesium ions in both groups were similar, with no significant differences observed. In the initial 7 days, they exhibited consistent and controlled release, without any significant burst release (Fig. 4B). Subsequently, the release of magnesium ions slowed down, reaching about 82.73% after 14 days. Based on the release curve, it can be inferred that compared to the Ti–Mg group, the Ti–Mg–H2O2 group exhibited a faster release of Mg ions.
In vitro antibacterial performance
After incubating the samples with S. aureus on agar medium for 12 hours (Fig. 5A), a large number of bacterial colonies were observed in the Ti and Ti–Mg groups, while almost no visible colonies were seen in the Ti–H2O2 and Ti–Mg–H2O2 groups. Compared to the Ti group, the bacterial survival rate on the Ti–Mg surface decreased to approximately 78%, while in the Ti–H2O2 and Ti–Mg–H2O2 groups, bacteria were almost completely eliminated (Fig. 5B). Following a 12 hours incubation with S. aureus in liquid medium, the bacteria were stained with a dual-color staining reagent for live and dead cells (Fig. 5C). Both the Ti–H2O2 and Ti–Mg–H2O2 samples showed a significant number of red-stained dead bacteria, substantially more than in the Ti and Ti–Mg groups. Correspondingly, the number of green-stained live bacteria in the Ti–H2O2 and Ti–Mg–H2O2 groups was notably lower than in the Ti and Ti–Mg groups.
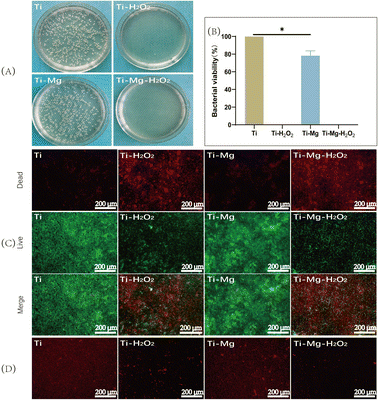 |
| Fig. 5 (A) Co-cultivation of various materials with S. aureus; (B) bacterial survival rates when co-cultivated with the materials; (C) staining of live/dead bacteria on the different surfaces (red represents dead bacteria, green represents live bacteria); (D) CTC bacterial staining (live bacteria labeled with red fluorescence) (* indicates p < 0.05). | |
Additionally, to investigate potential mechanisms of the antibacterial action, CTC redox dye staining was used to quantify active respiratory bacteria on different surfaces (Fig. 5D). The Ti and Ti–Mg groups displayed a large number of red-stained active bacteria. Compared to the Ti group, the number in the Ti–Mg group was reduced, indicating some antibacterial effect of the magnesium coating. Compared to the Ti and Ti–Mg groups, only a few red-stained active bacteria were visible in the Ti–H2O2 and Ti–Mg–H2O2 groups. The difference between these groups suggests that H2O2 treated Ti surfaces exhibit superior antibacterial properties. This may be achieved through the inhibition of bacterial respiratory chain formation.49
Cell adhesion and proliferation
After seeding cells on various materials for 72 hours, live/dead cell staining was performed to assess cell viability. As shown in Fig. 6A, the surfaces of all materials displayed a large number of green-stained live cells with only a few red-stained dead cells visible. Image analysis revealed that the live cell count followed the order Ti–Mg–H2O2 > Ti–Mg > Ti–H2O2 > Ti, and the differences among them were statistically significant (p < 0.05). CCK-8 assays (Fig. 6B) revealed a gradual increase in the number of cells on the surfaces of all samples over time. After 1 day of culture, there was no statistically significant difference in the proliferation of rBMSCs among different materials (P > 0.05). However, after 4 days of culture, cell proliferation showed a trend of Ti–Mg–H2O2 group > Ti–Mg group > Ti–H2O2 group > Ti group. The differences between each pair were statistically significant (P < 0.05). After 7 days of culture, the trend in cell proliferation was similar to that on day 4. All results indicate that the deposition of the MgCO3 coating, especially in combination with H2O2 treatment, can effectively enhance the proliferation of rBMSCs.
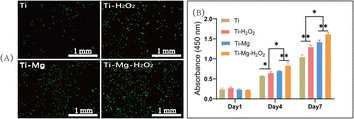 |
| Fig. 6 (A) Live/dead rBMSCs on different material surfaces, with live cells labeled with green fluorescence and dead cells labeled with red fluorescence; (B) the proliferation of rBMSCs inoculated on different material surfaces on days 1, 4, and 7 (* indicates p < 0.05; ** indicates p < 0.01). | |
ALP expression and in vitro calcium nodule deposition
After 14 days of culture, all surfaces were stained blue, with more stained cells observed on the surfaces of Ti–Mg and Ti–Mg–H2O2 (Fig. 7A), indicating these materials' capability to induce osteogenic differentiation of rBMSCs. Quantitative analysis (Fig. 7B) showed that the expression levels of ALP increased over time on all surfaces. On day 7, the Ti–Mg–H2O2 group exhibited the highest ALP content, followed by the Ti–Mg group, while the Ti group had the lowest ALP concentration. There were statistically significant differences in ALP content between the materials (p < 0.05). After 14 days of culture, a similar trend was observed, consistent with the results from day 7. These findings suggest that MgCO3 deposition combined with H2O2 treatment effectively promotes ALP expression in rBMSCs.
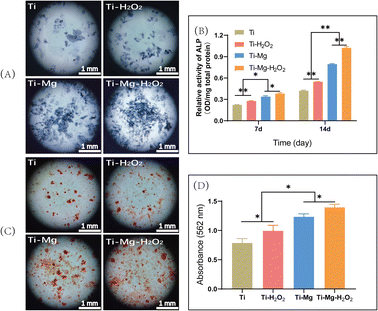 |
| Fig. 7 (A) Staining of alkaline phosphatase in rBMSCs on different material surface; (B) quantitative measurement of alkaline phosphatase content; (C) staining of calcium deposits with Alizarin Red S; (D) semi-quantitative measurement of calcium deposits (* indicates p < 0.05; ** indicates p < 0.01). | |
After 21 days of culture, Alizarin Red S staining was used to assess the formation of calcium nodule deposits (Fig. 7C). The results indicated the presence of orange-red calcium deposits on all surfaces, especially larger orange-red areas on the Ti–Mg and Ti–Mg–H2O2 samples. Semi-quantitative analysis of calcium nodule deposition (Fig. 7D) showed that the quantity of calcium nodules followed the trend of Ti–Mg–H2O2 > Ti–Mg > Ti–H2O2 > Ti, with significant differences between the groups (all p < 0.05). Based on these results, it is confirmed that the combination of MgCO3 deposition and H2O2 treatment effectively promotes in vitro mineralization.
Evaluation of bone-related gene expressions on various surfaces
Quantitative PCR (q-PCR) was utilized to measure the expression of osteogenesis-related genes, including OCN, Runx2, ALP, and OPN (Fig. 8). The expression of all these genes followed the trend of Ti–Mg–H2O2 > Ti–Mg > Ti–H2O2 > Ti, with significant differences observed between the groups (all p < 0.05). For OCN, the gene expression level in the Ti–Mg–H2O2 group was 128.8% higher than in the Ti–Mg group (p = 0.101), while the expression on Ti–Mg was 128.8% higher than on Ti–H2O2 (p = 0.0259), and the expression on Ti–H2O2 was 214.5% higher than on Ti (p = 0.0010). For OPN, the gene expression level in the Ti–Mg–H2O2 group was 116.8% higher than in the Ti–Mg group (p = 0.0046), the expression on Ti–Mg was 128.8% higher than on Ti–H2O2 (p = 0.0008), and the expression on Ti–H2O2 was 164.3% higher than on Ti (p = 0.0003). These results indicate that the coatings, especially when combined with H2O2 treatment, can significantly enhance the expression of osteogenic genes in rBMSCs.
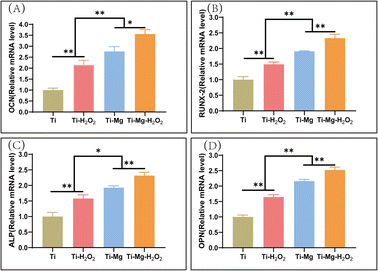 |
| Fig. 8 The gene expression levels normalized to GAPDH in rBMSCs including: (A) OCN, (B) Runx2, (C) ALP, (D) OPN after culture on different surfaces for 14 days (* indicates p < 0.05; ** indicates p < 0.01). | |
Discussion
In this study, a nanostructured magnesium carbonate coating was successfully prepared on Ti substrates using a hydrothermal reaction method which resulted in enhanced surface roughness and wettability compared with the pristine Ti surface. Additionally, the coating was further modified using H2O2 treatment, and the H2O2 treated coating exhibited a superior antibacteria activity. Moreover, compared to pristine Ti and hydrogen peroxide-treated Ti surfaces, the coated substrates exhibited improved proliferation of rBMSCs and promoted osteogenic differentiation through improving the ALP activity, bone matrix mineralization and the expressions of osteogenic-related genes.
Magnesium carbonate is an antacid medication known for its role in neutralizing stomach acid and protecting the gastric mucosa when used in the human body.50–52 Additionally, clinical trial research has shown that magnesium carbonate can be utilized for phosphate control in hemodialysis patients.53,54 In recent years, some studies have explored the regulation of Mg2+ release and promotion of bone formation through hydrogen-loaded MgO/MgCO3 particles.33 However, there are limited researches focused on depositing magnesium carbonate on the surface of titanium implants and evaluation of its capabilities in bone defect repair. In earlier studies, successful preparation of coatings containing magnesium on titanium (Ti) surfaces was achieved using techniques like sandblasting and electrophoretic deposition (EPD).55,56 In comparison to these methods, the hydrothermal reaction used in this study requires neither specialized equipment nor complex procedures. Moreover, in this reaction system, only Mg(NO3)2 and urea were used and reacted as following:
(NH2)2CO + H2O → 2NH3 + CO2, 2NH3 + H2O → 2NH4+ + OH− |
CO2 + H2O → CO32− + 2H−, Mg2+ + CO32− → MgCO3 |
At 298 K, the solubility product constants (Ksp) for Mg(OH)2 and MgCO3 are known to be 5.6 × 10−12 and 6.8 × 10−6,57,58 respectively. The nucleation driving force decreases with increasing Ksp,59 indicating that magnesium carbonate (MgCO3) is formed first rather than magnesium hydroxide (Mg(OH)2) under these conditions. However, if the alkaline environment is sufficiently strong, a transformation into magnesium hydroxide can occur.60 Magnesium hydroxide is less soluble compared to magnesium carbonate. When carbon dioxide is introduced into an alkaline solution, the situation changes: insufficient carbon dioxide leads to the formation of carbonate ions, while excess carbon dioxide results in the formation of bicarbonate ions. This process alters the pH of the solution and subsequently affects the dissolution and precipitation behavior of magnesium compounds.
Thus, the method herein is easy-simple and friendly environment, without waste product generation. Research reports have indicated that magnesium ions facilitate the proliferation and differentiation of osteoblasts.61 A substantial body of research has indicated that MgO and MgOH possess osteogenic, angiogenic, and antibacterial properties.62–64 However, their clinical utilization remains limited, possibly due to the concentration-dependent cytotoxicity induced by nanoscale magnesium oxide and magnesium hydroxide.65,66 In contrast, related studies have shown that consuming MgCO3 can lower serum phosphate levels in patients. The average daily dose is 1552 mg of MgCO3, which is equivalent to about 441 mg of elemental magnesium.53 Its safety in clinical use has been confirmed.50,51,53,54 Nonetheless, there has been limited exploration of depositing magnesium carbonate on the surface of titanium implants for bone repair, possibly because of its relatively modest functionality and weaker antibacterial capabilities.32 Hydrogen peroxide can introduce ROS (reactive oxygen species) onto the titanium surface, such as superoxide anion radicals (O2−), hydroxide ions (OH−), hydroxyl radicals (–OH), and other functional groups.67 Additionally, it can create micro-nanostructures and enhance hydrophilicity on the titanium surface, thus influencing the adhesion, proliferation, and osteogenic differentiation of osteoblasts, ultimately strengthening bone formation capacity.40 Therefore, in this study, after depositing magnesium carbonate, hydrogen peroxide was employed for further treatment.
In this study, a nanostructured magnesium carbonate coating was successfully synthesized on a titanium substrate using a hydrothermal reaction method, as illustrated in Fig. 1B, where both Ti and Ti–H2O2 samples exhibited smooth surfaces. However, as depicted in Fig. 3D, the surface of the magnesium coating demonstrated increased roughness, indicated by the Ra and Rq values. After modification with hydrogen peroxide, both Ra and Rq values decreased, which could be attributed to the oxidative effect of hydrogen peroxide, leading to the formation of an oxide film on the surface.68 Additionally, its cleaning and etching capabilities contributed to a smoother surface texture.69–71 Compared to the untreated magnesium coating, the Ra and Rq values were lower. A similar observation was made on the titanium substrate surfaces (Ti vs. Ti–H2O2), where the surface wettability of the coating were enhanced relative to the original Ti surface.
In this study, in vitro cell culture results indicate that, compared to pure titanium (Ti), magnesium-enriched titanium (Ti–Mg) significantly enhances the proliferation and differentiation of osteoblasts, with further enhancement observed in hydrogen peroxide-modified magnesium-enriched titanium (Ti–Mg–H2O2) compared to Ti–Mg. Additionally, after immersion in PBS for 21 days, detectable concentrations of magnesium ions were observed for both Ti–Mg and Ti–Mg–H2O2 (as shown in Fig. 4). These findings can be attributed to several key factors. Firstly, the magnesium carbonate coatings were composed of nanoparticles and exhibited increased roughness, which benefited for the adhesion and proliferation of osteoblasts on their surfaces.15,72,73 Also, these coatings deposition increased the surface wettability, which is crucial for the proteins adsorption, cell adhesion, proliferation, and differentiation of osteoblasts and related cells on implant surfaces.74 Secondly, magnesium ions released from magnesium carbonate may promote osteoblasts proliferation and differentiation through integrin-mediated pathways, strengthening the interaction between osteoblasts and biomaterials.23,24,75 These magnesium ions also serve as nucleation sites for hydroxyapatite formation, facilitating bone matrix mineralization.76 Finally, hydrogen peroxide introduces osteogenesis-related active groups, such as active hydroxyl (Ti–OH) and ROS (reactive oxygen species), onto the surface of titanium implants. Active hydroxyl groups can serve as deposition sites for calcium and phosphate ions, thereby promoting the formation of hydroxyapatite and enhancing the potential for new bone integration, further strengthening its osteogenic capacity.77 Additionally, a certain concentration of ROS can mediate the PI3K-AKT signaling pathway, thereby regulating cell adhesion, spreading, osteogenic differentiation, and bone growth.78,79
In this study, when co-cultured with bacteria, Ti + H2O2 and Ti–Mg–H2O2 exhibited excellent antibacterial properties compared to Ti. However, Ti–Mg showed 78.3% bacterial activity, and through CTC staining, it was observed that Ti–Mg had fewer live bacteria compared to Ti, while Ti + H2O2 and Ti–Mg–H2O2 both demonstrated a sparser presence of live bacteria, consistent with earlier research findings,32 as shown in Fig. 5.
In preliminary studies, magnesium carbonate coatings were immersed into 3% and 30% hydrogen peroxide for 20 min, respectively. In vitro antibacterial experiments and cell culture demonstrated that after reacting with 30% hydrogen peroxide, the magnesium carbonate coating exhibited superior antibacterial effect and no significant cytotoxicity compared with 3% hydrogen peroxide treatment. Thus, 30% hydrogen peroxide treatment was selected.
Compared to MgO and Mg(OH)2, both of which exhibit excellent antibacterial properties, magnesium carbonate is commonly used in clinical practice as a gastrointestinal protective drug,50,51 while hydrogen peroxide is frequently employed as an antibacterial agent.80 Both substances have established safety records. Therefore, it is reasonable to speculate that the hydrogen peroxide-modified magnesium carbonate coatings utilized in this study may possess favorable biocompatibility and hold promise for potential clinical applications. The antibacterial performance can be attributed to three mechanisms: firstly, the ability of hydrogen peroxide to generate reactive oxygen species, contributing to its antibacterial properties.34,81,82 Secondly, magnesium carbonate is alkaline and can alter the pH of bacterial survival in conjunction with magnesium ions.26 Finally, the direct interaction between nanoparticles and bacteria.83,84
In our research, the utilization of magnesium carbonate coatings following hydrogen peroxide treatment not only demonstrated exceptional antibacterial properties but also markedly amplified the osteogenic capabilities of BMSCs. However, the precise mechanisms underlying these effects remain incompletely elucidated and warrant further investigation for clarification. Subsequently, we intend to conduct in vivo animal assessments to delve deeper into this study.
Conclusion
In this study, magnesium carbonate-based coatings on titanium implants was successfully prepared via a hydrothermal reaction method, along with the development of magnesium-containing antibacterial coatings through hydrogen peroxide modification. The coatings exhibited enhanced wettability and surface roughness, which could improve the viability, proliferation, and osteogenic differentiation of BMSCs. Moreover, the hydrogen peroxide-modified coatings demonstrated superior antimicrobial properties. Collectively, these findings suggest this modification provides an effective strategy for design of functional orthopedic implants with antibacterial activity as well as potential osseointegration, which are promising in clinical application.
Author contributions
Shougang Xiang: conceptualization, methodology, investigation, writing – original draft. Chengdong Zhang: conceptualization, methodology, investigation, writing – original draft. Zhenju Guan: methodology, investigation. Xingping Li: methodology, investigation. Yumei Liu: validation, investigation; Gang Feng: validation, investigation. Xuwei Luo: conceptualization, supervision. Bo Zhang: conceptualization, methodology, investigation, writing – review & editing. Jie Weng: conceptualization, supervision. Dongqin Xiao: conceptualization, methodology, funding acquisition.
Conflicts of interest
The authors declare that they have no known competing financial interests or personal relationships that could have appeared to influence the work reported in this paper.
Acknowledgements
This study was funded by National Natural Science Foundation of China (82002289), Chunhui Project of Education Ministry of China (HZKY20220558), National Natural Science Foundation of Sichuan Province (2023NSFSC1740, 2022NSFSC0685, 2022NSFSC0609), the Medical Research Project Plan of Sichuan Province (Q22061, Q22034), the College-City Cooperation Project of Nanchong City (20SXQT0335, 22SXJCQN0002, 22SXQT0310, 22SXQT0385), Research Fund Project of Genertec Medical (TYYLKYJJ-2022-051).
References
- P. Khairallah and T. L. Nickolas, Clin. J. Am. Soc. Nephrol., 2022, 1, 121–130 CrossRef PubMed.
- M. Wang and T. Tang, J. Orthop. Translat., 2019, 17, 42–54 CrossRef PubMed.
- M. Ribeiro, F. J. Monteiro and M. P. Ferraz, Biomatter, 2012, 2, 176–194 CrossRef PubMed.
- K. J. Lauderdale, C. L. Malone, B. R. Boles, J. Morcuende and A. R. Horswill, J. Orthop. Res., 2010, 28, 55–61 CrossRef CAS PubMed.
- J. M. Schierholz and J. Beuth, J. Hosp. Infect., 2001, 49, 87–93 CrossRef CAS PubMed.
- C. Cyteval and A. Bourdon, Diagn. Interventional Imaging, 2012, 93, 547–557 CrossRef PubMed.
- M. Long and H. J. Rack, Biomaterials, 1998, 19, 1621–1639 CrossRef CAS PubMed.
- H. J. Rack and J. I. Qazi, Mater. Sci. Eng., C, 2006, 26, 1269–1277 CrossRef CAS.
- A. Yazdanian, A. Jahandideh and S. Hesaraki, Vet. Med. Sci., 2023, 9, 2342–2351 CrossRef CAS PubMed.
- S. Sakka, K. Baroudi and M. Z. Nassani, J. Invest. Clin. Dent., 2012, 3, 258–261 CrossRef PubMed.
- B. R. Chrcanovic, T. Albrektsson and A. Wennerberg, J. Oral Rehabil., 2014, 41, 443–476 CrossRef CAS PubMed.
- M. Lucke, G. Schmidmaier, S. Sadoni, B. Wildemann, R. Schiller, N. P. Haas and M. Raschke, Bone, 2003, 32, 521–531 CrossRef CAS PubMed.
- G. Schmidmaier, M. Kerstan, P. Schwabe, N. Südkamp and M. Raschke, Injury, 2017, 48, 2235–2241 CrossRef CAS PubMed.
- J. Esteban, M. Vallet-Regí and J. J. Aguilera-Correa, Antibiotics, 2021, 10, 1270 CrossRef CAS.
- R. A. Gittens, R. Olivares-Navarrete, S. L. Hyzy, K. H. Sandhage, Z. Schwartz and B. D. Boyan, Connect. Tissue Res., 2014, 55, 164–168 CrossRef CAS PubMed.
- D. A. Robinson, R. W. Griffith, D. Shechtman, R. B. Evans and M. G. Conzemius, Acta Biomater., 2010, 6, 1869–1877 CrossRef CAS PubMed.
- Y. Li, G. Liu, Z. Zhai, L. Liu, H. Li, K. Yang, L. Tan, P. Wan, X. Liu, Z. Ouyang, Z. Yu, T. Tang, Z. Zhu, X. Qu and K. Dai, Antimicrob. Agents Chemother., 2014, 58, 7586–7591 CrossRef PubMed.
- X. Zhang, Z. Zhang, Q. Shu, C. Xu, Q. Zheng, Z. Guo, C. Wang, Z. Hao, X. Liu, G. Wang, W. Yan, H. Chen and C. Lu, Adv. Funct. Mater., 2021, 31, 2008720 CrossRef CAS.
- X. Li, P. Gao, P. Wan, Y. Pei, L. Shi, B. Fan, C. Shen, X. Xiao, K. Yang and Z. Guo, Sci. Rep., 2017, 7, 40755 CrossRef CAS.
- Y. Yu, H. Lu and J. Sun, Acta Biomater., 2018, 71, 215–224 CrossRef CAS PubMed.
- D. Zhao, S. Huang, F. Lu, B. Wang, L. Yang, L. Qin, K. Yang, Y. Li, W. Li, W. Wang, S. Tian, X. Zhang, W. Gao, Z. Wang, Y. Zhang, X. Xie, J. Wang and J. Li, Biomaterials, 2016, 81, 84–92 CrossRef CAS.
- Y. H. Leem, K. S. Lee, J. H. Kim, H. K. Seok, J. S. Chang and D. H. Lee, J. Tissue Eng. Regener. Med., 2016, 10, E527–E536 CrossRef CAS.
- X. Zhang, Q. Chen and X. Mao, BioMed Res. Int., 2019, 2019, 1–13 CAS.
- D. Predoi, S. L. Iconaru, M. V. Predoi, G. E. Stan and N. Buton, Nanomaterials, 2019, 9, 1295 CrossRef CAS.
- L. M. Stabryla, K. A. Johnston, N. A. Diemler, V. S. Cooper, J. E. Millstone, S.-J. Haig and L. M. Gilbertson, Nat. Nanotechnol., 2021, 16, 996–1003 CrossRef CAS PubMed.
- H. Feng, G. Wang, W. Jin, X. Zhang, Y. Huang, A. Gao, H. Wu, G. Wu and P. K. Chu, ACS Appl. Mater. Interfaces, 2016, 8, 9662–9673 CrossRef CAS.
- C. Janning, E. Willbold, C. Vogt, J. Nellesen, A. Meyer-Lindenberg, H. Windhagen, F. Thorey and F. Witte, Acta Biomater., 2010, 6, 1861–1868 CrossRef CAS PubMed.
- Y. Nakamura, K. Okita, D. Kudo, D. N. D. Phuong, Y. Iwamoto, Y. Yoshioka, W. Ariyoshi and R. Yamasaki, Nanomaterials, 2021, 11, 1584 CrossRef CAS PubMed.
- C. C. Coelho, T. Padrão, L. Costa, M. T. Pinto, P. C. Costa, V. F. Domingues, P. A. Quadros, F. J. Monteiro and S. R. Sousa, Sci. Rep., 2020, 10, 19098 CrossRef CAS.
- M. Voropaiev and D. Nock, BMC Gastroenterol., 2021, 21, 112 CrossRef CAS PubMed.
- A. Tarnawski, A. Ahluwalia and M. K. Jones, Curr. Pharm. Des., 2013, 19, 126–132 CAS.
- K. Welch, M. A. Latifzada, S. Frykstrand and M. Strømme, ACS Omega, 2016, 1, 907–914 CrossRef CAS PubMed.
- H. Zhou, K. Yu, H. Jiang, R. Deng, L. Chu, Y. Cao, Y. Zheng, W. Lu, Z. Deng and B. Liang, Biomacromolecules, 2021, 22, 4552–4568 CrossRef CAS PubMed.
- M. V. Urban, T. Rath and C. Radtke, Wien. Med. Wochenschr., 2019, 169, 222–225 Search PubMed.
- A. A. Mohammadi, S. M. Seyed Jafari, M. Kiasat, M. R. Pakyari and I. Ahrari, Burns, 2013, 39, 1131–1136 CrossRef PubMed.
- N. Urao, V. Sudhahar, S. J. Kim, G. F. Chen, R. D. McKinney, G. Kojda, T. Fukai and M. Ushio-Fukai, PLoS One, 2013, 8, e57618 CrossRef CAS PubMed.
- M. Cho, T. K. Hunt and M. Z. Hussain, Am. J. Physiol.: Heart Circ. Physiol., 2001, 280, H2357–H2363 Search PubMed.
- A. Ohlin, E. Mattsson, M. Mörgelin, J. R. Davies, G. Svensäter, S. Corvec, P. Tengvall and K. Riesbeck, Eur. Spine J., 2018, 27, 2463–2468 CrossRef PubMed.
- M. Khodaei and S. Hossein Kelishadi, Surf. Coat. Technol., 2018, 353, 158–162 Search PubMed.
- H. Li, J. Huang, Y. Wang, Z. Chen, X. Li, Q. Wei, X. Liu, Z. Wang, B. Wen, Y. Zhao, J. Liu and J. Zuo, Oxid. Med. Cell. Longevity, 2022, 2022, 13 Search PubMed.
- A. E. Daw, H. A. Kazi, J. S. Colombo, W. G. Rowe, D. W. Williams, R. J. Waddington, D. W. Thomas and R. Moseley, J. Biomater. Appl., 2013, 28, 144–160 Search PubMed.
- L. Shi, W. Ning and L. Luo, Shanghai Coat., 2017, 55, 49–52 CAS.
- C. Magdaleno-López and J. de Jesús Pérez-Bueno, Int. J. Adhes. Adhes., 2020, 98, 102551 CrossRef.
- Y. Cao, P. Cheng, S. Sang, C. Xiang, Y. An, X. Wei, Z. Shen, Y. Zhang and P. Li, Regener. Biomater., 2021, 8, rbab019 CrossRef PubMed.
- L. Zhou, H. Wu, X. Gao, X. Zheng, H. Chen, H. Li, J. Peng, W. Liang, W. Wang, Z. Qiu, A. Udduttula, K. Wu, L. Li, Y. Liu and Y. Liu, Drug Des., Dev. Ther., 2020, 14, 3519–3533 CrossRef CAS PubMed.
- S. Chen, C. Zhang, D. Xiao, F. Shi, K. Liu, Y. Wan, K. Duan, J. Weng, G. Feng and Y. Yin, Surf. Coat. Technol., 2021, 426, 127769 CrossRef CAS.
- C. Zhang, X. Li, D. Xiao, Q. Zhao, S. Chen, F. Yang, J. Liu and K. Duan, J. Funct. Biomater., 2022, 13, 78 CrossRef CAS PubMed.
- W. Liang, Z. M. Li, L. Y. Wang, L. Chen and H. P. Li, Acta Phys. Sin., 2017, 03, 129–135 Search PubMed.
- F. Wang, Q. Wu, G. Jia, L. Kong, R. Zuo, K. Feng, M. Hou, Y. Chai, J. Xu, C. Zhang and Q. Kang, Adv. Sci., 2023, 30, 2303911 CrossRef PubMed.
- D. M. Schaefer, L. J. Wheeler, C. H. Noller, R. B. Keyser and J. L. White, J. Dairy Sci., 1982, 65, 732–739 CrossRef CAS PubMed.
- M. C. Sulz, M. Manz, P. Grob, R. Meier, J. Drewe and C. Beglinger, Digestion, 2007, 75, 69–73 CAS.
- J. Labenz, M. Anschütz, J. Walstab, R. S. Wedemeyer, H. Wolters and B. Schug, BMJ Open Gastroenterol., 2023, 1, e001048 CrossRef PubMed.
- I. P. Tzanakis, A. N. Papadaki, M. Wei, S. Kagia, V. V. Spadidakis, N. E. Kallivretakis and D. G. Oreopoulos, Int. Urol. Nephrol., 2008, 40, 193–201 CrossRef CAS PubMed.
- D. M. Spiegel, B. Farmer, G. Smits and M. Chonchol, J. Renal Nutr., 2007, 17, 416–422 Search PubMed.
- R. Sirello, D. Frattini, L. Banci, A. Baracchino, E. Canciani and C. Dellavia, Int. J. Oral Health Med. Res., 2018, 4, 1–6 Search PubMed.
- X. Cai, J. Cai, K. Ma, P. Huang, L. Gong, D. Huang, T. Jiang and Y. Wang, J. Biomater. Sci., Polym. Ed., 2016, 27, 954–971 CrossRef CAS PubMed.
- C. Dong, G. He, W. Zheng, T. Bian, M. Li and D. Zhang, Mater. Lett., 2014, 134, 286–289 CrossRef CAS.
- C. Y. Cao, C. H. Liang, Y. Yin and L. Y. Du, J. Hazard. Mater., 2017, 329, 222–229 CrossRef CAS PubMed.
- M. Guo, X. Sun, J. Chen and T. Cai, Acta Pharm. Sin. B, 2021, 8, 2537–2564 CrossRef PubMed.
- O. R. Cámara, Chem. Educ., 2000, 5, 120–132 CrossRef.
- H. Qin, J. Weng, B. Zhou, W. F. Zhang, G. Q. Li, Y. Q. Chen, T. T. Qi, Y. C. Zhu, F. Yu and H. Zeng, Biol. Trace Elem. Res., 2023, 201, 2823–2842 CrossRef CAS PubMed.
- Q. Zhao, L. Yi, L. Jiang, Y. Ma, H. Lin and J. Dong, Nanomedicine, 2019, 14, 1109–1133 CrossRef PubMed.
- C. L. Wetteland, N.-Y. T. Nguyen and H. Liu, Acta Biomater., 2016, 35, 341–356 CrossRef CAS PubMed.
- T. M. Bedair, C. K. Lee, D.-S. Kim, S.-W. Baek, H. M. Bedair, H. P. Joshi, U. Y. Choi, K.-H. Park, W. Park, I. Han and D. K. Han, J. Tissue Eng., 2020, 11, 204173142096759 CrossRef.
- J. Sun, S. Wang, D. Zhao, F. H. Hun, L. Weng and H. Liu, Cell Biol. Toxicol., 2011, 27, 333–342 CrossRef CAS PubMed.
- M. Pallavi, J. Waterman, Y. Koo, J. Sankar and Y. Yun, Appl. Sci., 2019, 9, 4304 CrossRef CAS.
- P. Tengvall, H. Elwing, L. Sjöqvist, I. Lundström and L. M. Bjursten, Biomaterials, 1989, 10, 118–120 CrossRef CAS PubMed.
- L. Benea and N. Simionescu-Bogatu, Materials, 2021, 23, 7404 CrossRef PubMed.
- G. Wang, H. Xia, W. Huang, J. Yang, B. Liu and L. Yuan, Micromachines, 2022, 12, 2204 CrossRef PubMed.
- Y. Wang, L. Jiang and L. M. Qian, Light Ind. Mach., 2019, 05, 1–5 CAS.
- Z. Wang, J. Li, G. Zhang, Y. Zhi, D. Yang, X. Lai and T. Ren, Materials, 2020, 10, 2270 CrossRef PubMed.
- P. A. Tran, K. Fox and N. Tran, J. Colloid Interface Sci., 2017, 485, 106–115 CrossRef CAS PubMed.
- A. E. Medvedev, A. Neumann, H. P. Ng, R. Lapovok, C. Kasper, T. C. Lowe, V. N. Anumalasetty and Y. Estrin, Mater. Sci. Eng., C, 2017, 71, 483–497 CrossRef CAS PubMed.
- N. Tsukimura, T. Ueno, F. Iwasa, H. Minamikawa, Y. Sugita, K. Ishizaki, T. Ikeda, K. Nakagawa, M. Yamada and T. Ogawa, Acta Biomater., 2011, 7, 4267–4277 CrossRef CAS PubMed.
- Y. H. Leem, K. S. Lee, J. H. Kim, H. K. Seok, J. S. Chang and D. H. Lee, J. Tissue Eng. Regener. Med., 2016, 10, E527–E536 CrossRef CAS PubMed.
- L. F. Zubieta-Otero, O. M. Gomez-Vazquez, B. A. Correa-Piña and M. E. Rodriguez-Garcia, MedComm: Biomater. Appl., 2023, 4, e64 Search PubMed.
- C. Jin, Oral Med., 2014, 34, 52–55 CAS.
- Q. Zhang, X. Z. Tang, X. X. Ding, Y. C. Yao, K. Song, Y. G. Cao and Q. Shi, Clin. Oral Med. J., 2019, 35, 136–140 Search PubMed.
- X. Li, X. Y. Ma, Y. F. Feng, Z. S. Ma, J. Wang, T. C. Ma, W. Qi, W. Lei and L. Wang, Biomaterials, 2015, 36, 44–54 CrossRef CAS PubMed.
- V. Patel, M. Kelleher and M. McGurk, Br. Dent. J., 2010, 208, 61–64 CrossRef CAS PubMed.
- Y. Yang, K. Chen, G. Wang, H. Liu, L. Shao, X. Zhou, L. Liu and S. Yang, Int. J. Mol. Sci., 2023, 13, 10566 Search PubMed.
- M. Y. Alkawareek, A. Bahlool, S. R. Abulateefeh and A. M. Alkilany, PLoS One, 2019, 14, e0220575 CrossRef CAS PubMed.
- N. Aničić, M. Vukomanović, T. Koklič and D. Suvorov, Small, 2018, 14, 1800205 CrossRef PubMed.
- C. Bankier, R. K. Matharu, Y. K. Cheong, G. G. Ren, E. Cloutman-Green and L. Ciric, Sci. Rep., 2019, 9, 16074 CrossRef CAS PubMed.
Footnote |
† Authors contributed equally to this work. |
|
This journal is © The Royal Society of Chemistry 2024 |