DOI:
10.1039/D3RA08288A
(Paper)
RSC Adv., 2024,
14, 4556-4567
A signal “switch-on” photoelectrochemical sensor based on a 3D-FM/BiOI heterostructure for the sensitive detection of L-ascorbic acid†
Received
5th December 2023
, Accepted 17th January 2024
First published on 2nd February 2024
Abstract
A highly efficient 3D flower MoS2 (3D-FM)-based heterostructure photocatalyst (3D-FM/BiOI) was successfully obtained via a simple hydrothermal synthesis strategy. 3D-FM/BiOI showed prominent photoelectrochemical performance, distinguished stability and good selectivity. The introduction of 3D-FM, by promoting the photoelectric property attributed to it, facilitated the separation of photogenerated electron–hole pairs. Since the redox process of L-ascorbic acid (L-AA) resulted in an increasing photocurrent of 3D-FM/BiOI, a signal “switch-on” photoelectrochemical sensor (PECS) was designed to sensitively determine L-AA for the first time. Under optimized conditions, the 3D-FM/BiOI PECS worked over a wide range from 1 μM to 0.8 mM with a low detection limit of 0.05 μM (S/N = 3). The PECS was successfully exploited for L-AA sensing in human urine with excellent accuracy and applicability, demonstrating its practical precision and superb serviceability. Furthermore, the 3D-FM/BiOI PECS exhibited satisfactory selectivity and stability, providing a great potential platform for the construction of an L-AA sensor in various practical samples and complicated environments.
1 Introduction
As a crucial component in biological metabolism, L-ascorbic acid (L-AA) is present in many bodily fluids and participates in several biological processes of great relevance.1 Furthermore, it is significant to the immune response and prevents free-radicals, such as those found in cancer and Parkinson's disease.2 Many fruits and vegetables contain L-AA, especially beverages derived from fruit juice. In the presence of heat, light and iron, the air-oxidation rate of L-AA is sharply accelerated.3 However, humans cannot self-synthesize it. Therefore, the development of a facile and rapid analytical method for the sensing of L-AA with high selectivity and sensitivity is particularly crucial in diagnostic applications and the pharmaceutical field.4,5
To date, several traditional methods, such as liquid chromatography,6 fluorescence,7 chemiluminescence and electrochemical methods,8 have been exploited for the determination of L-AA. Among these analytical methods, photoelectrochemical (PEC) detection9 is a brand-new approach to a sensing platform for biochemical molecules, which is attributed to electron transfer between target, photocatalyst and electrode with photo-irradiation.10 It integrates electrochemical and optical techniques to analyze the concentration of target analytes. Due to their electrochemical detection in conjunction with photo-irradiation, PEC11 sensors present some excellent properties, such as low background noise, and simple sample processing and operation.12 Inorganic semiconductors possess exceptional photoelectric conversion efficiency and are widely adopted photoelectric materials for constructing PEC sensors.
BiOX (X = Cl, Br, I) are p-type semiconductors and eminent photocatalyst materials, attributed to their pertinent band gaps and unique tetragonal crystal structure with a strong internal electric field, which can increase the effective separation of electron–hole pairs.13 Bi oxides with a band gap ranging from 1.8 to 3.0 eV yield high photocatalytic efficiency under visible light irradiation.14 Among Bi oxides, bismuth oxyhalides (BiOX, X = Br, Cl, I) with their great electrochemical and optical properties have attracted growing attention in modern applications such as photocatalysis15 and optoelectronic devices.16 Bi oxyhalides are characterized by a unique layered structure which makes them the dominant family of photoactive materials under visible light irradiation. The electrical field between [Bi2O2]2+ sheets and X slabs inhibits electron–hole recombination, which can increase the photocatalytic performance. Among BiOX catalysts, bismuth oxyiodide (BiOI) has been the subject of growing attention due to its narrow band gap (1.7–1.8 eV) and effective visible-light activity.
Recently, many important studies have been reported into BiOX, such as photocurrent-polarity switching between methylene-blue-loaded liposome and iodine-doped BiOCl for in situ amplified immunoassay17 and ultrathin mesoporous BiOCl nanosheet-mediated liposomes for photoelectrochemical immunoassay with in situ signal amplification.18 BiOI with its layered structure and strong internal electric field shows the best visible light response with a relatively narrow band gap compared with other BiOX (Cl or Br).19 Unfortunately, BiOI still exhibits some disadvantages of slow separation of photogenerated electron–hole pairs and the limitation of its band structure.20 To overcome these shortcomings, many approaches have been employed to modify BiOI to extend the lifetime of charge carriers, including morphological modulation21 and combination with other semiconductors.22 Therefore, various attempts, including co-catalysts, constructing heterostructures and doping, have been exploited to enhance its property. Constructing heterogeneous structures has been reported to be an effective approach, such as constructing g-C3N4/BiOI,23 BiOI/Bi2MoO6 nanocomposite24 and BiOI/graphene hydrogel.25
A 3D-FM construction composed of 2D nanosheets is supposed to be a particularly good catalyst for practical applications,26 which not only increases its structural stability but also maximizes the exposure of its active sites. It possesses high charge mobility, a large specific surface area and excellent photo-electron conversion with wide application in photocatalysis,27 dry lubrication,28 lithium batteries,29 and hydrogen production.30 Furthermore, 3D-FM plays a crucial role in photocatalysis and photoelectrochemistry. It is reported that 3D-FM can form a heterojunction with semiconductors such as Cu2O/3D-FM nanohybrid,31 In2O3/3D-FM nanofilm32 and Nafion/hemoglobin/3D-FM.33 Nevertheless, to the best of our knowledge, there have been few reports exploring the application of MoS2/semiconductor heterostructures for PEC sensing.
In this work, 3D-FM/BiOI composites were successfully synthesized through a facile and convenient one-step solvent thermal method, which exhibited much stronger photoactivity than BiOI. The 3D-FM/BiOI photocatalysts displayed greatly improved photocatalytic performance under visible light. The intimate interfacial contact between 3D-FM and BiOI nanosheets promoted charge separation efficiency. A 3D-FM/BiOI heterojunction-based PEC sensing system can promote the transfer of interfacial charges and improve the separation of photo-generated electron–hole pairs. A signal “switch-on” PECS was designed to sensitively determine L-AA, based on the redox process of L-AA. The enhanced activity was ascribed primarily to the formed heterostructure, which can promote the transfer of interfacial charges and improve the separation of photo-generated electron–hole pairs. The results showed that 3D-FM/BiOI exhibited excellent sensitivity, selectivity and stability, and the performance of the 3D-FM/BiOI sensor was superior to that of most reported sensors. To further verify its practical applicability, the established sensor was applied for L-AA detection in human urine samples. This work will provide inspiration to scientific researchers to construct photoelectrochemical sensors using heterostructure materials that will have potential application value.
2 Experimental section
2.1. Synthesis of the photocatalysts
All reagents were chemical analysis grade and there was no further purification for the experiments.
2.1.1 Synthesis of 3D-FM. All 3D-FM hemispheres were synthesized by a facile hydrothermal method.34 First, 7 mmol of thiourea (H2CSNH2), 0.25 mmol of ammonium molybdate (NH4)6Mo7O24·4H2O, and 1 mmol of hexadecyltrimethylammonium bromide (CTAB) were all diffused in 10 mL of ultrapure water to form a well-distributed solution. Then, the well-distributed solution was kept in a state of intense agitation until the addition of ammonia solution (3 M) adjusted the pH to the appropriate value. Subsequently, the obtained solution was transferred into a 25 mL Teflon-lined autoclave and heated at 200 °C for 24 h. The powdered product was obtained after naturally cooling down to room temperature. It was washed several times with analysis-grade ethanol and ultrapure water. Finally, the product was dried at 60 °C for 12 h to obtain the pure product.
2.1.2 Preparation of 3D-FM/BiOI. 3D-FM/BiOI was fabricated according to a previous report35 with some modification. First, 0.1 g of the obtained 3D-FM was added into a solution of Bi(NO3)3·5H2O in ethylene glycol (60 mL, 0.10 M). After the mixture had formed a homogeneous suspension under ultrasound, KI ethylene glycol solution (60 mL, 0.20 M) was added drop by drop into the above mixture with vigorous stirring. The above mixture solution was kept under stirring for 1 h and then transferred to a 100 mL Teflon-lined autoclave. It was heated to 160 °C for 12 h. Subsequently, the product was washing alternately with ethanol and ultrapure water. Finally, the solid was dried at 60 °C for 4 h to obtain 3D-FM/BiOI pure product.For comparison, pure BiOI was synthesized by the above method without adding 3D-FM.
2.2. Preparation of 3D-FM/BiOI/ITO electrodes
ITO electrodes were cleaned with acetone, isopropanol, ethanol, and deionized water in sequence for 30 min each under ultrasonic conditions, then dried at 60 °C for 2 h. Then, 10 mg of the prepared 3D-FM/BiOI and 20 μL of Nafion (5 wt%) were added to 1 mL of ultrapure water, and then subjected to ultrasonic waves for 1 h to obtain a 10 mg mL−1 3D-FM/BiOI suspension. Next, 50 μL of suspension was dropped onto the clean ITO, and dried at room temperature to obtain a 3D-FM/BiOI electrode. For comparison, individual suspensions of pure BiOI and 3D-FM were dropped onto ITO to give 3D-FM/ITO and BiOI/ITO by the above method.
2.3. PEC measurements
The PEC properties were measured with an electrochemical workstation (CHI 760E, Shanghai Chenhua Instrument Corporation, China) in a standard three-electrode system. An Ag/AgCl electrode in 3 M KCl, a Pt plate and 3D-FM/BiOI/ITO were exploited as the reference electrode, counter electrode and working electrode, respectively. The electrochemical behavior of 3D-FM/BiOI/ITO was investigated at different scanning speeds. A 20 W xenon lamp provided simulated sunlight (Beijing Newbet Technology Co., Ltd, China) and 0.1 M phosphate buffer solution (PBS, pH = 7.4) was used as the electrolyte. Cyclic voltammetry (CV) curves were performed from −0.2 V to 0.6 V vs. Ag/AgCl at a scan rate of 50 mV s−1. Electrochemical impedance spectroscopy (EIS) was conducted in 0.1 M KCl solution containing 0.5 mM of K3Fe(CN)6/K4Fe(CN)6 at −0.2 V vs. a reversible hydrogen electrode (RHE) with a small-amplitude alternating current of 10 mV in the frequency range from 0.1 Hz to 100 kHz. The PEC tests for L-AA were performed at a bias potential of −0.228 V (vs. saturated Ag/AgCl).
3 Results and discussion
3.1. Characterization of the synthesized materials
The morphology and elemental distribution of BiOI, 3D-FM and 3D-FM/BiOI were observed by scanning electron microscopy (SEM) and energy-dispersive X-ray spectroscopy (EDS). The microstructure of BiOI showed a regular spherical structure and a diameter of approximately 2–3 μm (Fig. 1a). The high-magnification image of the 3D-FM hemispheres showed that the uniform 2D nanosheet petals are arranged together in a disorganized and staggered manner, which could effectively inhibit the aggregation of a single 3D-FM nanosheet (Fig. 1b). The micrograph of BiOI showed it was attached closely to the surface of 3D-FM (Fig. 1c), indicating the form of the 3D-FM/BiOI heterostructure. Fig. 1d shows the EDS region of the 3D-FM/BiOI composite and its corresponding EDS mappings (Fig. 1e–i), which demonstrate that there are only Bi, O, I, Mo and S elements in the 3D-FM/BiOI composite and the BiOI are evenly distributed. To further investigate the morphological and structural information for the 3D-FM/BiOI composite, the TEM of BiOI and the 3D-FM/BiOI composite were analyzed, as shown in Fig. 2a and b, and the elemental distributions were observed with energy-dispersive X-ray spectroscopy (EDS) (c–g). The HRTEM of 3D-FM/BiOI was analyzed, and the results are shown in Fig. S1a and b,† and different lattice fringes are found.
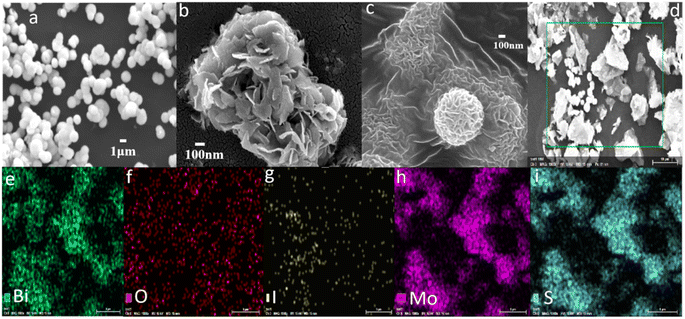 |
| Fig. 1 The SEM morphology of (a) BiOI, (b) 3D-FM and (c) 3D-FM/BiOI. (d) The energy-dispersive X-ray spectroscopy (EDS) region of the 3D-FM/BiOI composite and its corresponding EDS mappings (e–i). | |
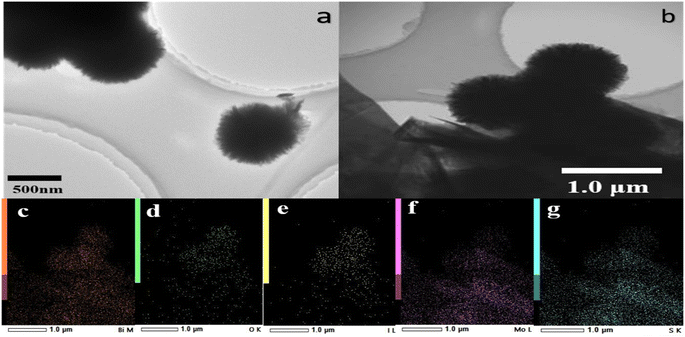 |
| Fig. 2 The TEM morphology of BiOI (a) and (b) 3D-FM/BiOI. (c–g) The elemental distribution observed with energy-dispersive X-ray spectroscopy (EDS). | |
The crystalline structures of BiOI, 3D-FM and 3D-FM/BiOI were identified by X-ray diffraction (XRD). The XRD pattern of 3D-FM was consistent with that reported in the literature.36 As shown in Fig. 3, the diffraction peaks of the tetragonal phase of BiOI were consistent with the diffraction peaks of (012), (110), (014) and (212) in the composites. The XRD pattern of the 3D-FM/BiOI heterostructure showed predominantly the main peaks of BiOI and one weak peak (004) of 3D-FM, due to the better crystallinity and stronger peak intensity of BiOI and the small content of 3D-FM.
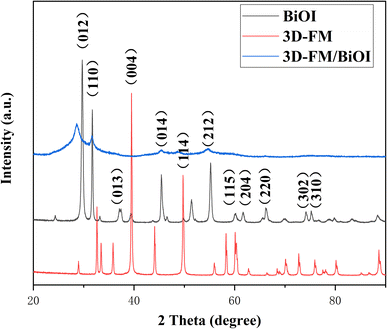 |
| Fig. 3 The X-ray diffraction patterns of BiOI, 3D-FM and 3D-FM/BiOI. | |
X-ray photoelectron spectroscopy (XPS) was undertaken to investigate the elemental composition and valence states of the 3D-FM/BiOI composites. Fig. 4 shows the typical survey scan and high-resolution spectra of the Bi (4f, 4d and 4p), Mo 3p, S 2p, O 1s, and I 3d peaks for the 3D-FM/BiOI composites, which are in accordance with the above EDS result. Bi, O, and I elements related to BiOI and the Mo, O, and S elements related to 3D-FM were observed in the survey XPS of 3D-FM/BiOI, illustrating that BiOI was successfully attached to 3D-FM. Fig. 5a clearly shows the binding energy of Bi 4f, in which the two peaks located at 165 eV and 156.5 eV are the characteristic peaks of Bi 4f5/2 and Bi 4f7/2 in 3D-FM/BiOI, suggesting that the chemical valence state of Bi element in the 3D-FM/BiOI composites is +3.32 The binding energies of I 3d3/2 and I 3d5/2 in 3D-FM/BiOI are located at 616 eV and 627.5 eV, respectively (Fig. 5d). This is the characteristic peak of I− in the 3D-FM/BiOI samples. For the O 1s spectrum (Fig. 5b), the peaks for the Bi–O bond (527.1 eV) and I–O bond (529.5 eV) were ascribed to BiOI. The peak of S 2P was exhibited in the spectrum of Bi + S (Fig. 5a). However, the existence of Mo indicates that 3D-FM had been successfully introduced into the composite (Fig. 5c). The above XPS analysis confirmed the successful fabrication of a 3D-FM/BiOI heterojunction.
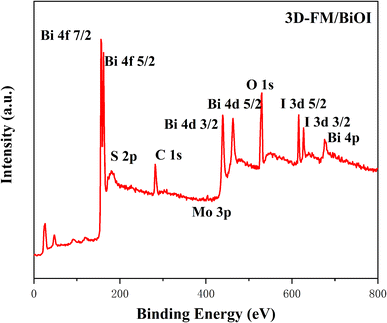 |
| Fig. 4 XPS spectra of 3D-FM/BiOI. | |
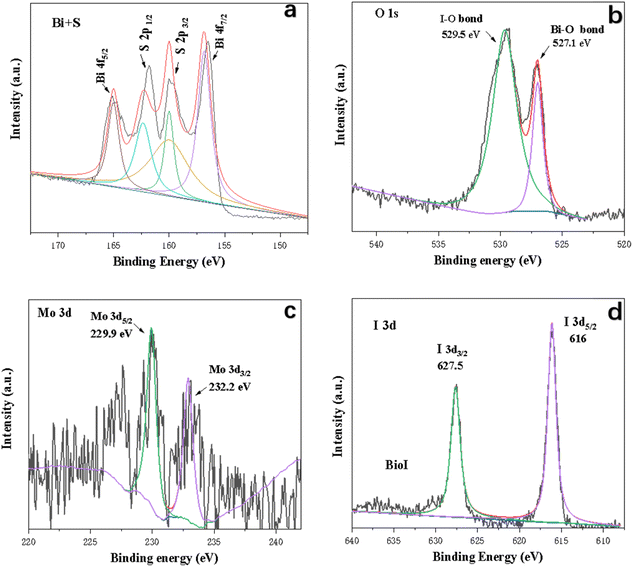 |
| Fig. 5 Survey spectra and high-resolution core spectra for (a) Bi + S, (b) O 1s, (c) Mo and (d) I 3d. | |
The bonding and chemical composition of BiOI and the 3D-FM/BiOI composites were investigated using FT-IR spectra. From Fig. 6, the band at about 3425 cm−1 is the stretching vibration of O–H, which is the deformation vibration of absorbed water molecules.35 The band at 498 cm−1 corresponds to the stretching mode of Bi–O, which results from BiOI. With increasing 3D-FM content, the peak of the 3D-FM/BiOI composites at 498 cm−1 is shifted slightly compared with pure BiOI. This proves the interaction of 3D-FM nanosheets and BiOI nanosheets, which formed an excellent heterojunction structure. The FT-IR results are in accordance with the XRD analysis, and indicate that the 3D-FM has been successfully coupled with BiOI.
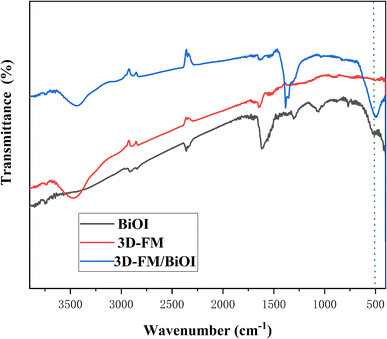 |
| Fig. 6 The FT-IR spectra of BiOI and 3D-FM/BiOI. | |
3.2. Sensing mechanism of the 3D-FM/BiOI sensing system
Hydroxyl radicals (˙OH) and photogenerated holes (h+) are the major active factors in the process of photocatalytic oxidation. Similar research has been reported, such as a contactless photoelectrochemical biosensor based on the ultraviolet-assisted gas sensing interface of 3D-SnS2 nanosheets37 and the photoelectrochemical bioanalysis of microRNA on yolk-in-shell Au@CdS based on a catalytic hairpin-assembly-mediated CRISPR-Cas12a system,38 etc.39,40 According to the properties of semiconductors, p-type semiconductors enter the solution with holes under light, and electrons enter the counter electrode.41,42 The electron donor consumes the holes, and the electron donor undergoes an oxidation reaction. In contrast, n-type semiconductors enter the solution under light, and holes enter the counter electrode. The electron acceptor consumes electrons in the solution, and the electron acceptor undergoes a reduction reaction. Above all, L-AA is easily oxidized and generally serves as an electron donor, and so serves as a hole scavenger. The probable mechanism of 3D-FM/BiOI as a photocatalyst was explored for an increase in photocatalytic capabilities and detecting the content of L-AA (Fig. 8). The minimum position of the conduction band (CB) and the maximum position of the valence band (VB) of BiOI and 3D-FM are estimated by employing the empirical formulae:where X is the absolute electronegativity, which is 5.94 eV. Ee is a constant of the free electrons energy with respect to the hydrogen scale (≈4.5 eV), and Eg is the forbidden bandwidth of a semiconductor. On the basis of the UV-vis spectra, the band gap energy of BiOI nanosheets is 1.38 eV (Fig. 7a). Therefore, the calculated EVB and ECB of BiOI are 2.13 and 0.75 eV, respectively. According to the Nernst equation (eqn (3)), the EVB (vs. NHE, pH = 7.4) and ECB (vs. NHE, pH = 7.4) of BiOI were calculated to be 1.69 V and 0.31 V, respectively. |
E(NHE, pH = 7.4) = E(NHE, pH = 0) − 0.059 pH
| (3) |
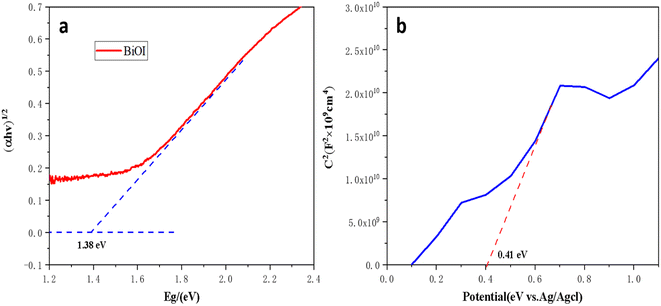 |
| Fig. 7 (a) The photon energy (hν) for BiOI and (b) the Fermi level (EF) of 3D-FM nanosheets. | |
Furthermore, the Fermi level (EF) of the 3D-FM nanosheets (0.41 eV) (Fig. 7b) is more positive than the CB potential of BiOI, which favors the transfer of photoelectrons on the VB of BiOI to 3D-FM through the heterojunction interface, thereby promoting the separation of photocarriers.
Under visible light irradiation (λ > 400 nm), the photogenerated electrons on the VB are excited and transferred to the corresponding CB, and the holes are left on the valence band of BiOI and 3D-FM nanosheets. Because the CB potential of 3D-FM is more negative than that of BiOI, the photo-induced electrons on the CB of 3D-FM can easily transfer to the CB of BiOI through the interface. At the same time, the VB position of 3D-FM is more negative than that of BiOI, so the holes can migrate from BiOI to the 3D-FM nanosheets; thus the efficient separation of photogenerated carriers occurs.
L-AA is an effective hole capture agent in photoelectric detection. The 3D-FM/BiOI PEC sensor is a kind of target-analyzing device based on a photoelectrically active material. When the light is on, the electrons in the 3D-FM/BiOI material are excited, and the identifying probe on the material captures L-AA, causing a change in photocurrent or photovoltage. The photoelectric signal changes with the concentration of L-AA, and there is a functional relationship between the two. Therefore, the change in the photoelectric signal can be used to quantitatively determine the L-AA. The valence band potential of the photocatalysts of the 3D-FM/BiOI composite is much higher than the oxidation potential of L-AA (+0.153 eV). When 3D-FM/BiOI/ITO is stimulated by light, L-AA can easily be oxidized by holes to dehydroascorbic acid (DHA), and the hydrogen ions gain electrons and will be reduced to H2 at the opposite electrode. In this process, L-AA acts as a hole scavenger by providing electrons to the photocatalysts of the 3D-FM/BiOI composite, reducing the recombination of the electron–hole pair and enhancing the photocurrent signal. The PEC sensing mechanism on L-AA may follow eqn (4)–(6):
|
BiOI/3D-FM + hν → BiOI/3D-FM + h+ + e−
| (4) |
The separation rate of electrons and holes determines the effect of the photocatalytic activity. Weak PL peaks represented low recombination rates of photo-excited electrons and holes. PL spectra of BiOI, 3D-FM and BiOI/3D-FM with an excitation wavelength of 350 nm (Fig. 9a) showed that the peaks of BiOI/3D-FM samples are lower than those of BiOI and 3D-FM, which suggested that the recombination of electrons and holes is restrained due to the formation of a heterojunction between BiOI and 3D-FM. The sample of BiOI/3D-FM had the lowest PL intensity among all the samples, which indicated the heterojunctions and surface-active oxygen between BiOI and 3D-FM. The cooperative effect of the above two factors caused it to have higher migration efficiency of photoelectron and hole pairs, which improved the ability for photocatalytic performance.
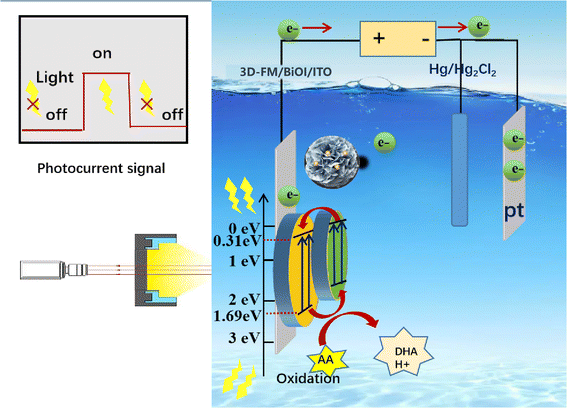 |
| Fig. 8 The probable mechanisms of 3D-FM/BiOI as photocatalysts under visible light. | |
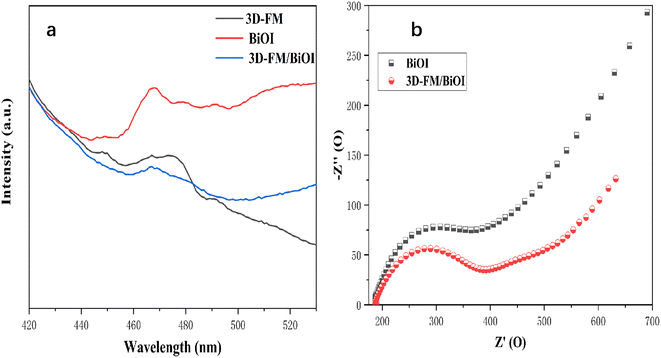 |
| Fig. 9 (a) Photoluminescence emission spectra of (a) 3D-FM, BiOI and 3D-FM/BiOI. (b) EIS plots of BiOI and 3D-FM/BiOI/ITO. | |
3.3. Characteristics of PEC sensing
The electrochemical property of the 3D-FM/BiOI electrode was further explored with the CV curves (Fig. 10a) in 1.0 M KCl solution containing 5.0 mM [Fe(CN)6]3−/4−. 3D-FM/BiOI exhibited almost no significant variation in redox peak current compared to that in pure BiOI, which may be attributed to the addition of a small amount of 3D-FM.
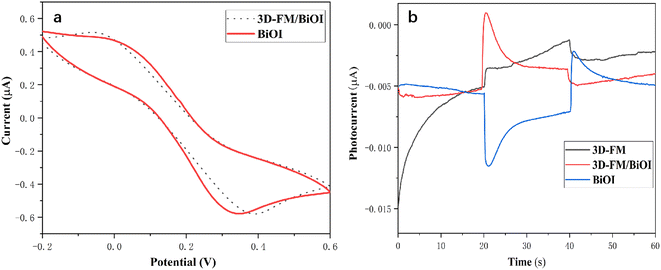 |
| Fig. 10 (a) CV curves of 3D-FM/BiOI and pure BiOI. (b) Photocurrent of 3D-FM/BiOI, BiOI and 3D-FM under visible light illumination. | |
The relationship between the enhanced photocurrent and charge transfer was investigated by EIS. The semicircle in an EIS plot can reveal the charge transfer resistance. As depicted in Fig. 9b, 3D-FM/BiOI/ITO exhibited a smaller arc size than BiOI and the calculated charge transfer resistance value of 3D-FM/BiOI (200.5 Ω) was also smaller than that of BiOI (275 Ω). These results further confirmed that the introduction of 3D-FM could promote charge transfer and reduce overall charge transfer resistance, which plays an important role in enhancing the photocurrent.
Under visible light illumination, the PEC properties of 3D-FM, BiOI and 3D-FM/BiOI were explored to evaluate the transient photocurrent response by estimating 60 s photocurrent curves. As shown in Fig. 10b, 3D-FM exhibited a weak anode photocurrent, while BiOI as a p-type semiconductor showed an extremely weak cathode photocurrent owing to its relatively narrow band gap (1.95 eV), which makes the electrons and holes easy to recombine. After introducing 3D-FM into BiOI, a remarkably improved photocurrent response was observed for the 3D-FM/BiOI electrode, which was several times larger than that of BiOI. The sensitive photocurrent response is attributed to the effective separation and transmission of photocarriers in 3D-FM/BiOI, which is due to the excellent conductivity of multilayer 3D-FM and the strong interface connection between 3D-FM and BiOI.
3.4. Optimization of experimental conditions
All optimization of experimental conditions was conducted in 0.1 M PBS (pH 7.4) containing 1 μM of L-AA. To achieve optimal performance of the PEC sensor, a study of the influence of the ratio of BiOI to 3D-FM was conducted. When the ratio of BiOI to 3D-FM was significantly increased from 10
:
1 to 10
:
3, the photocurrent difference also significantly increased. However, when the ratio was further increased to 10
:
4, the photocurrent difference increased only slowly (Fig. 11a). This is because the introduction of 3D-FM can enhance the photoelectric activity, but it is not conducive to the oxidation of L-AA. Therefore, the optimal ratio of 3D-FM to BiOI was 10
:
3 and this was selected for the sensing experiments.
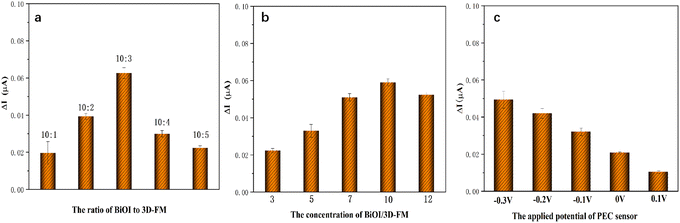 |
| Fig. 11 The effects of (a) the ratio of BiOI to 3D-FM; (b) concentration of BiOI/3D-FM; (c) applied potential. Conditions: (a) concentration: 10 mg mL−1; applied potential, −0.2 V; (b) the ratio of BiOI to 3D-FM, 10 : 3; applied potential, −0.2 V; (c) the ratio of BiOI to 3D-FM, 10 : 3; concentration, 10 mg L−1 (All experiments were conducted in 0.1 M PBS (pH 7.4) containing 1 μM L-AA.). | |
The influence of the concentration of 3D-FM/BiOI was then explored. When the concentration of BiOI/3D-FM was varied from 3 to 12 mg mL−1, the photocurrent difference was greatly enhanced (Fig. 11b). When the concentration was further increased from 10 to 12 mg mL−1, a significant decrease in photocurrent difference was exhibited. The decline was due to the thicker coating on the surface of ITO hindering electron transfer, thereby affecting the oxidation of L-AA and leading to a decrease in the photocurrent difference. Thus, 10 mg mL−1 was adopted as the appropriate concentration of 3D-FM/BiOI for the subsequent experiments.
The applied potential of a PEC sensor can affect the amplitude of the photocurrent. A more negative application potential leads to a stronger cathode photocurrent and electron drift caused by larger measurement bias. Furthermore, the effect of an applied potential ranging from −0.3 V to 0 V was investigated. As illustrated in Fig. 11c, the photocurrent difference was greatly enhanced, resulting in the negative shift of the potential. However, a larger applied voltage will lead to a stronger background noise signal, which will interfere with the detection of a low content L-AA. Thus, a bias voltage of −0.2 V was ultimately used in the following experiments.
3.5. Analytical performance of the 3D-FM/BiOI PEC sensing system
The sensing property of the 3D-FM/BiOI sensing platform for accurate detection of L-AA was explored under optimal conditions. As shown in Fig. 12a, the concentration of L-AA increased in the range from 1 μM to 0.8 mM, corresponding to an increase in the photocurrent difference of the 3D-FM/BiOI sensor. Fig. 12b demonstrates that there is a good linear relationship between the concentration of L-AA and photocurrent difference. The linear equation can be described as ΔI = 6.7750[C] + 0.0576 and the coefficient is 0.9941, where ΔI represents the photocurrent difference of L-AA at different concentrations and [C] is the concentration of L-AA. The limit of detection (LOD) calculated from the equation LOD = 3σ/k was 0.05 μM, where σ is the standard deviation of the blank solution (n = 10) and k is the slope of the linear equation.
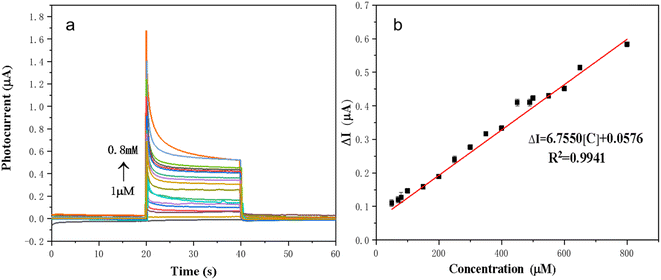 |
| Fig. 12 (a) PEC responses of the BiOI/3D-FM sensor at different L-AA concentrations. (b) The corresponding calibration curve for the determination of L-AA. | |
3.6. Applications in real sample analysis
To confirm the practicability and feasibility of the developed method, and also to evaluate the applicability of this fabricated photoelectrode for L-AA detection in real human urine samples (as shown in Table 1), in general the standard addition method was used to detect L-AA in a real sample and three repeated experiments were conducted to ensure the authenticity of the data. The urine samples were collected from one male who was 25 years old. Typically, 20 μL of human urine sample was added to 20 mL of 0.1 M PBS (pH 7.4) solution, and then it was measured by the proposed method. To evaluate the accuracy of the analytical result, a recovery test was conducted at three spiked levels (5, 20, 100 μM). The recoveries of L-AA detection in human urine were 96.40–106.50% with a relative standard deviation below 4.32%, indicating the feasibility and good reproducibility of the 3D-FM/BiOI sensor in the detection of human urine samples.
Table 1 Recovery of L-AA in human urine by 3D-FM/BiOI/ITO photoelectric detectiona
Sample |
Determined (μM) |
Spiked (μM) |
Found (μM) |
Recovery (%) |
RSD (%) |
RSD (%) = (standard deviation/the average of the data obtained by repeating 3 times) × 100%. |
1 |
0.45 ± 0.01 |
5 |
4.82 |
96.40 |
4.32 |
2 |
0.43 ± 0.01 |
20 |
21.3 |
106.50 |
3.05 |
3 |
0.47 ± 0.03 |
100 |
101.5 |
101.5 |
2.63 |
3.7. Selectivity, reproducibility and stability of the 3D-FM/BiOI sensor
The selectivity of 3D-FM/BiOI was explored and investigated in the presence of potential interferents. Sensing solutions including L-AA, dopamine, L-threonine, uric acid, urea, L-arginine and sucrose D-AA were added to the electrolyte to investigate their potential effects on L-AA detection (Fig. 13a). Compared with the photocurrent of 1 μM L-AA, the change in photocurrent was less than 3% after adding 50 μM of interferents to the electrolyte with 1 μM of L-AA, which proved that the 3D-FM/BiOI sensor possesses excellent selectivity and anti-interference capability. The good selectivity is attributed to the structure of the 3D-FM/BiOI PEC sensor and the charge generation/transfer mechanism of 3D-FM/BiOI.
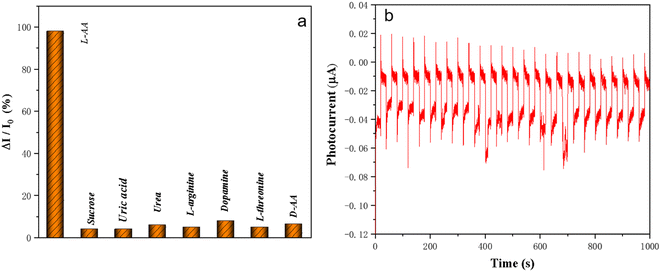 |
| Fig. 13 (a) Evaluation of selectivity of the BiOI/3D-FM sensor to interfering substances. (b) Photocurrent stability of BiOI/3D-FM. | |
The serviceability of 3D-FM/BiOI was estimated according to reproducibility and stability. The experiments were conducted in 0.1 M PBS (pH 7.4) containing 1 μM of L-AA. Different batches of 3D-FM/BiOI were explored, which showed similar PEC performance for the determination of L-AA, demonstrating the good reproducibility of 3D-FM/BiOI. The stability of the 3D-FM/BiOI sensor was investigated by repeating on/off cycles within 1000 s under intermittent visible light irradiation. Fig. 13b shows that the photocurrent can still maintain its initial intensity and there is almost no change after 1000 s. Furthermore, the PEC properties of 3D-FM/BiOI remained unchanged after it was stored at 4 °C in the dark for 7 days. The stability of 3D-FM/BiOI for L-AA was proved by all the results.
3.8. Comparison with other sensors toward L-AA
The analytical performance of the 3D-FM/BiOI PEC sensor toward L-AA was compared with other reported PEC sensors (Table 2). Compared with other reported PEC sensors,43–48 the established PEC sensor exhibited a wider linear range and much lower LOD for detection of L-AA due to the separated light source and electric detection signal of the PEC sensor, which can effectively reduce the background noise.
Table 2 Comparison of linear range and detection limit of enzyme-free photoelectrochemical sensors for the determination of L-AAa
Modified material |
Linear range |
Detection LOD |
Reference |
Dy6-SCN monocrystal: [Dy6L2(μ3-OH)4(μ2-OH)2(SCN)8(H2O)4]·6CH3CN·2CH3OH·H2O PDI: perylenediimide CoHCF: cobalt hexacyanoferrate g-C3N4 NSs: graphitic carbon nitride nanosheets. |
BiVO4/FTO |
5–300 μM |
1 μM |
43 |
Au/ZnSe/ZnO |
0.2–1.8 mM |
— |
44 |
Dy-OSCN/FTO |
7.94 μM–1.113 × 10−2 M |
3.35 μM |
45 |
CoOOH-modified PD |
0.1–10 μM |
33.4 nM |
46 |
CoHCF-modified Pt |
1.0 × 10−6–1.0 × 10−3 M |
1 μM |
47 |
g-C3N4NSs |
0.25–100 μM |
— |
48 |
This work |
1 μM–0.8 mM |
0.05 μM |
|
4 Conclusions
In summary, a PECS of 3D-FM/BiOI was successfully prepared and applied in sensing L-AA in human urine with excellent accuracy and applicability, demonstrating its practical precision and superb serviceability. Furthermore, the 3D-FM/BiOI PECS exhibited satisfactory selectivity and stability, providing a great potential platform for the construction of an L-AA sensor in various practical samples and complicated environments.
Author contributions
H. G. planned the experiments, conducted the experiments, analyzed the data, and wrote the paper draft. W. X. and S. W. supported and discussed the manuscript. J. L. and H. M. analyzed the data.
Conflicts of interest
The authors declare no conflict of interest.
Acknowledgements
The authors gratefully acknowledge financial support from Jilin Provincial Department of Science and Technology Project [2021020142GX].
References
- Y. Li, F. Pan and S. Yin, et al., Nafion assisted preparation of prussian blue nanoparticles and its application in electrochemical analysis of l-ascorbic acid, Microchem. J., 2022, 177, 107278 CrossRef CAS.
- L. Shen, M. A. Khan and X. Wu, et al., Fe–N–C single-atom nanozymes based sensor array for dual signal selective determination of antioxidants, Biosens. Bioelectron., 2022, 205, 114097 CrossRef CAS.
- H. Alhazimeh, M. G. Al-Fandi and L. M. K. Al-Ebbini, Development of an inkjet-printed electrochemical nanosensor for ascorbic acid detection, Sens. Rev., 2022, 42(3), 342–353 CrossRef.
- Y. Liu, L. Liang and X. Zhu, et al., N-Doped Carbon Dots as Fluorescent "Turn-Off" Nanosensors for Ascorbic Acid and Fe3+ Detection, ACS Appl. Nano Mater., 2022, 5(5), 7268–7277 CrossRef.
- A. Singh, K. Kulathuraan and K. Pakiyaraj, et al., Green Synthesized Carbon Quantum Dots from Curcuma Longa for Ascorbic Acid Detection, Adv. Sci. Technol., 2022, 117, 97–101 Search PubMed.
- L. A. Pachla and P. T. Kissinger, Determination of ascorbic acid in foodstuffs, Pharmaceuticals, and body fluids by liquid chromatography with electrochemical detection, Anal. Chem., 1976, 48(2), 364–367 CrossRef CAS PubMed.
- Y. N. Wang, S. D. Wang and Y. Fan, et al., A dual-chemosensor based on Ni-CP: Fluorescence turn-on sensing toward ascorbic acid and turn-off sensing toward acetylacetone, J. Lumin., 2022, 243, 118680–118685 CrossRef CAS.
- W. K. Anan, A. Olarnwanich and C. Sriprachuabwong, et al., Disposable paper-based electrochemical sensor utilizing inkjet-printed Polyaniline modified screen-printed carbon electrode for Ascorbic acid detection, J. Electroanal. Chem., 2012, 685, 72–78 CrossRef.
- Y. Hou, L. Zhu and H. Hao, et al., A novel photoelectrochemical aptamer sensor based on rare-earth doped Bi2WO6 and Ag2S for the rapid detection of Vibrio parahaemolyticus, Microchem. J., 2021, 165, 106132 CrossRef CAS.
- L. P. Tu and G. W. Xu, QDs Based Photoelectrochemical Sensors for Detection of Ascorbic Acid[J], . Mater Sci Forum, 2021, 1033, 77–81 Search PubMed.
- C. T. Hou, J. Y. Peng and Q. Xu, et al., Elaborate fabrication of MOF-5 thin films on a glassy carbon electrode (GCE) for photoelectrochemical sensors, RSC Adv., 2012, 2, 12696–12698 RSC.
- J. X. Chen and G. C. Zhao, Nano-encapsulant of ascorbic acid-loaded apoferritin-assisted photoelectrochemical sensor for protease detection, Talanta, 2017, 168, 62–66 CrossRef CAS.
- L. Zhao, X. Zhang and C. Fan, et al., First-principles study on the structural, electronic and optical properties of BiOX (X=Cl, Br, I) crystals, Physica B, 2012, 407(17), 3364–3370 CrossRef CAS.
- F. Zhang, S. Zou and T. Wang, et al., CeO2/Bi2WO6 Heterostructured Microsphere with Excellent Visible-light-driven Photocatalytic Performance for Degradation of Tetracycline Hydrochloride, Photochem. Photobiol., 2017, 93(5), 1154–1164 CrossRef CAS.
- X. Chang, J. Huang and C. Cheng, et al., BiOX (X= Cl, Br, I) photocatalysts prepared using NaBiO 3 as the Bi source: characterization and catalytic performance, Catal. Commun., 2010, 11, 460–464 CrossRef CAS.
- A. M. Ganose, M. Cuff and K. T. Butler, et al., Scanlon Interplay of orbital and relativistic effects in bismuth oxyhalides: BiOF, BiOCl, BiOBr, and BiOI, Chem. Mater., 2016, 28, 1980–1984 CrossRef CAS.
- H. Y. Wang, X. Y. Wan and X. Wang, et al., Ultrathin mesoporous BiOCl nanosheets-mediated liposomes for photoelectrochemical immunoassay with in situ signal amplification, Biosens. Bioelectron., 2023, 239, 115628 CrossRef CAS.
- R. J. Zeng, J. H. Xu and T. K. Liang, et al., Photocurrent-polarity-switching photoelectrochemical biosensor for switching spatial distance electroactive tags, ACS Sens., 2023, 8, 317–325 CrossRef CAS PubMed.
- W. L. Huang and Q. Zhu, DFT calculations on the electronic structures of BiOX (X = F, Cl, Br, I) photocatalysts with and without semicore Bi 5d states, J. Comput. Chem., 2009, 30(2), 183–190 CrossRef CAS.
- G. Li, F. Qin and R. Wang, et al., BiOX (X = Cl, Br, I) nanostructures: Mannitol-mediated microwave synthesis, visible light photocatalytic performance, and Cr(VI)
removal capacity, J. Colloid Interface Sci., 2013, 409, 43–51 CrossRef CAS PubMed.
- J. Q. Liu and W. Y. Cheng, Recent Advances in the High Performance BiOX(X=Cl, Br, I) Based Photo-catalysts, J. Inorg. Mater., 2015, 30(10), 1009 CrossRef CAS.
- X. Zhang, Z. Ai and F. Jia, etc. Generalized One-Pot Synthesis, Characterization, and Photocatalytic Activity of Hierarchical BiOX (X = Cl, Br, I) Nanoplate Microspheres, J. Phys. Chem. C, 2008, 112(3), 747–753 CrossRef CAS.
- B. K. Liu, X. L. Han and Y. Wang, et al., Synthesis of g-C3N4/BiOI/BiOBr heterostructures for efficient visible-light-induced photocatalytic and antibacterial activity, J. Mater. Sci.: Mater. Electron., 2018, 29, 14300–14310 CrossRef CAS.
- W. T. Li, Y. F. Zheng and H. Y. Yin, et al., Heterojunction BiOI/Bi2MoO6 nanocomposite with much enhanced photocatalytic activity, J. Nanopart. Res., 2015, 17(6), 271 CrossRef.
- D. M. Chen, J. J. Yang and Y. Zhu, et al., Fabrication of bioi/graphene hydrogel/fto photoelectrode with 3d porous architecture for the enhanced photoelectrocatalytic performance, Appl. Catal., B, 2018, 233, 202–212 CrossRef CAS.
- H. S. Yang, J. Zhou and J. Bao, et al., A simple hydrothermal one-step synthesis of 3d-mos2/rgo for the construction of sensitive enzyme-free hydrogen peroxide sensor, Microchem. J., 2020, 162, 105746 CrossRef.
- P. F. Wang, P. H. Shi and Y. C. Hong, et al., Facile deposition of ag3po4 on graphene-like mos2 nanosheets for highly efficient photocatalysis, Mater. Res. Bull., 2015, 62, 24–29 CrossRef CAS.
- P. Serles, K. Gaber and S. Pajovic, High temperature microtribological studies of mos2 lubrication for low earth orbit, Lubricants, 2020, 8(4), 49 CrossRef.
- X. S. Zhou, L. J. Wan and Y. G. Guo, Facile synthesis of MoS2@CMK-3 nanocomposite as an improved anode material for lithium-ion batteries, Nanoscale, 2012, 19, 5868–5871 RSC.
- X. H. Zhang, X. H. Huang and M. Q. Xue, et al., Hydrothermal synthesis and characterization of 3D flower-like MoS2 microspheres, Mater. Lett., 2015, 148, 67–70 CrossRef CAS.
- Y. Liu, X. Xu and J. Zhang, et al., Flower-like MoS2 on Graphitic Carbon Nitride for Enhanced Photocatalytic and Electrochemical Hydrogen Evolutions, Appl. Catal., B, 2018, 239, 334–344 CrossRef CAS.
- S. Wang, Y. Guan and L. Wang, et al., Fabrication of a novel bifunctional material of BiOI/Ag3VO4 with high adsorption-photocatalysis for efficient treatment of dye wastewater, Appl. Catal., B, 2015, 168, 448–457 CrossRef.
- T. Yang, Y. Chen and B. Qu, et al., Construction of 3D flower-like MoS2 spheres with nanosheets as anode materials for high-performance lithium ion batteries, Electrochim. Acta, 2014, 115, 165–169 CrossRef CAS.
- X. Zhang, H. Suo and R. Zhang, et al., Photocatalytic Activity of 3D Flower-like MoS2 Hemispheres, Mater. Res. Bull., 2017, 100, 249–253 CrossRef.
- Q. Wang, L. Guo and W. Gao, et al., Facile synthesis of bioi/mxene heterostructure as a superior photoelectrochemical sensor for sensitive detection of glucose, Anal. Chim. Acta, 2022, 1233, 340511 CrossRef CAS PubMed.
- W. H. Zhu, Y. S. Xia and J. X. Xia, et al., Facile synthesis of few-layered mos2 modified bioi with enhanced visible-light photocatalytic activity, Colloids Surf., A, 2016, 511, 1–7 CrossRef.
- L. T. Huang, G. N. Cai and R. J. Zeng, et al., Contactless photoelectrochemical biosensor based on ultraviolet-assisted gas sensing interface of 3D-SnS2nanosheets: From mechanism reveal to practical application, Anal. Chem., 2022, 94, 9487–9495 CrossRef CAS PubMed.
- R. J. Zeng, J. H. Xu and L. L. Lu, et al., Photoelectrochemical bioanalysis of microRNA on yolk-in-shell Au@CdS based on catalytic hairpin assembly-mediated CRISPR-Cas12a system, Chem. Commun., 2022, 58, 7562–7565 RSC.
- Y. Gao, Y. Y. Zeng and X. L. Liu, et al., Liposome-mediated in situ formation of type-I heterojunction for amplified photoelectrochemical immunoassay, Anal. Chem., 2022, 94, 4859–4865 CrossRef CAS.
- Z. C. Yu, H. X. Gong and J. H. Xu, et al., Exploiting photoelectric activities and piezoelectric properties of NaNbO3 semiconductors for point-of-care immunoassay, Anal. Chem., 2022, 94, 3418–3426 CrossRef CAS PubMed.
- Y. Gao, Y. Y. Zeng and X. L. Liu, et al., Liposome-mediated in situ formation of type-I heterojunction for amplified photoelectrochemical immunoassay, Anal. Chem., 2022, 94, 4859–4865 CrossRef CAS PubMed.
- Z. C. Yu, H. X. Gong and J. H. Xu, et al., Exploiting photoelectric activities and piezoelectric properties of NaNbO3 semiconductors for point-of-care immunoassay, Anal. Chem., 2022, 94, 3418–3426 CrossRef CAS PubMed.
- L. Li, M. Q. Li and H. Liu, et al., A portable non-enzyme photoelectrochemical ascorbic acid sensor based on BiVO4 electrode under 20 W LED light, J. Electroanal. Chem., 2019, 855, 113573 CrossRef CAS.
- X. Wang, G. Li and J. Peng, et al., The Au/ZnSe/ZnO Heterojunction Improves the Electron Transfer Behavior to Enhance the Detection Performance of Ascorbic Acid, J. Alloys Compd., 2021, 873, 159721 CrossRef CAS.
- Z. Zhao, D. Han and R. Xiao, et al., An enzyme-free photoelectrochemical sensor platform for ascorbic acid detection in human urine[J], Chemosensors, 2022, 10(7), 268 CrossRef CAS.
- Y. H. Chi, An ultrasensitive photoelectrochemistry sensor for ascorbic acid based on etching perylenediimide modified CoOOH nanosheets, Mater. Express, 2019, 9(7), 741–748 CrossRef.
- H. Li, Q. Gao and L. Chen, et al., Photocurrent determination ascorbic acid using an n-silicon electrode modified by platinum and cobalt hexacyanoferrate films, Sens. Actuators, B, 2012, 173(10), 540–546 CrossRef CAS.
- R. M. Motaghed, L. Ge and H. Jiang, et al., A facile photoelectrochemical sensor for high sensitive ROS and AA detection based on graphitic carbon nitride nanosheets, Biosens. Bioelectron., 2018, 107, 54 CrossRef.
|
This journal is © The Royal Society of Chemistry 2024 |
Click here to see how this site uses Cookies. View our privacy policy here.