DOI:
10.1039/D3RA07934A
(Paper)
RSC Adv., 2024,
14, 6006-6015
MgFe2O4@Tris magnetic nanoparticles: an effective and powerful catalyst for one-pot synthesis of pyrazolopyranopyrimidine and tetrahydrodipyrazolopyridine derivatives†
Received
19th November 2023
, Accepted 31st January 2024
First published on 15th February 2024
Abstract
Magnesium (Mg) as a metal has wide applications, but its use in chemical reactions is rarely reported. Currently, magnesium catalytic processes are being developed to synthesize basic chemical compounds. Therefore, an effective and recyclable nano-catalyst was synthesized using MgFe2O4@Tris in this study. The structure of MgFe2O4@Tris was characterized by various techniques including Fourier-transform infrared (FT-IR), scanning electron microscopy (SEM), transmission electron microscopy (TEM), energy dispersive X-ray (EDX), thermogravimetric analysis (TGA), X-ray diffraction (XRD), and vibrating sample magnetometer (VSM) techniques. Finally, the catalytic activity of this nano-catalyst was evaluated for the synthesis of pyrazolopyranopyrimidine and tetrahydrodipyrazolopyridine derivatives. Among the advantages of this catalyst are its high catalytic activity, high yields, use of environmentally friendly solvents, easy magnetic separation, and the possibility of reusing the catalyst.
1. Introduction
Nanoparticles are an important class of nanometer-scale materials that exhibit unrivaled physicochemical attributes for widespread applications, such as in biomedicine,1 biofuels,2 sensors,3 batteries,4 and catalysts.5 The increase in the amount of scientific discussion on the topic of magnetic materials shows the increasing interest in this field of science.6 Spinel ferrites with the generic formula MFe2O4 (M = Mg, Mn, Ni, Co, or Zn) are important magnetic substances due to their high stability, excellent electrical and magnetic properties, catalytic properties and biocompatibility.7 Magnesium ferrite (MgFe2O4) is a magnetic nanomaterial that has good magnetic properties and electrical and thermal resistance.8 Recently, MgFe2O4 nanoparticles have attracted increasing amounts of interest because they have various applications in Li-ion batteries,9 gas sensors,10 catalysts,11 and adsorbents.12
Multicomponent reactions (MCRs) are reactions between more than three compounds in one step and are important organic chemistry reactions for the synthesis of intricate molecules. They are widely used in all fields of organic synthesis.13 In the process of multicomponent reactions, magnetic nanocatalysts are the best option as heterogeneous catalysts due to their easy product separation technique, easy recovery, and environmentally friendly properties.
Heterocyclic compounds14 are one of the largest families of organic compounds. They are compounds with a cyclic structure that have at least one carbon atom and at least one heteroatom such as O, N, or S in their structure. Pyrazolopyranopyrimidines15 and tetrahydrodipyrazolopyridines16 are two important classes of nitrogen-containing heterocyclic compounds with diverse applications. Pyrazolopyranopyrimidines are a group of polycyclic-fused heterocyclic compounds, consisting of rings containing pyrimidine, pyran, and pyrazole. Pyrazolopyranopyrimidine derivatives are of great interest because of their broad pharmacological and biological properties and activities as antimicrobial,17 antituberculosis,18 anti-inflammatory,19 analgesic,20 and anticancer agents.21 Tetrahydrodipyrazolopyridines are among the most important nitrogen-containing heterocyclic compounds, and they consist of two parts, pyrazole and 1,4-dihydropyridine. Due to anticancer,22 antiviral,23 and anti-leishmanial24 properties, they are of particular importance in organic chemistry. Nanocatalysis has found numerous applications in the synthesis of heterocyclic compounds due to the development of nanotechnology.25 A majority of currently available and commercially approved drugs contain heterocycles containing nitrogen and oxygen. Due to the versatile applications of heterocycle synthesis catalyzed by metal nanoparticles, its significance should not be overlooked.26
In this research, we report tris(hydroxymethyl) aminomethane-functionalized MgFe2O4 magnetic nanoparticles (MgFe2O4@Tris) as a new, efficient and recyclable catalyst for the synthesis of pyrazolopyranopyrimidine and tetrahydrodipyrazolopyridine derivatives. These heterocyclic compounds were prepared under mild conditions in a green solvent and at room temperature (Scheme 1).
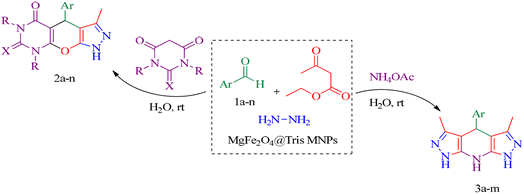 |
| Scheme 1 Synthesis of pyrazolopyranopyrimidine and tetrahydrodipyrazolopyridine compounds using MgFe2O4@Tris MNPs. | |
2. Experimental
2.1. General
All reactants used in this research were purchased from Merck, Fluka, or Sigma-Aldrich chemical companies. The melting points were defined using a Barnstead Electrothermal 9100 in capillary tubes. The infrared (IR) spectra of samples were recorded in KBr pellets using a VRTEX 70 spectrophotometer (Bruker, Germany). 13C and 1H NMR spectra (in Hertz) were obtained using a Bruker DRX-250 AVANCE instrument in DMSO-d6 as the solvent and TMS as the internal standard. Energy-dispersive X-ray spectroscopy (EDX) and scanning electron microscopy (SEM) were carried out and utilized a Czech TESCAN instrument. Thermogravimetric analysis (TGA) was performed using a thermogravimetric analyzer (PerkinElmer-STA6000, USA), and magnetic measurements of the nanocatalyst were obtained using a vibrating sample magnetometer (VSM; MDKB). X-ray diffraction (XRD) was carried out using a Holland Philips PW1730 and TEM of the magnetic nanoparticles (MNPs) was recorded with a Philips-EM 208S TEM.
2.2. Synthesis of MgFe2O4@Tris MNPs
2.2.1. General method for the synthesis of MgFe2O4 nanoparticles.
A mixture of FeCl3 (8 mmol = 1.3 g) and MgCl2·6H2O (4 mmol = 0.81 g) was dissolved in 40 mL of deionized water in a round-bottomed flask (250 mL). Then, it was stirred for 30 minutes at 500 rpm (revolutions per minute) by a mechanical stirrer. Then, 10 mL of sodium hydroxide solution (0.1 M) was added to the reaction mixture and heated for 2 h at 80 °C until brown precipitates were obtained. The product was separated by an external magnet and washed with deionized water, then washed twice with ethanol and dried in an oven at 80 °C for 24 h and calcined at 700 °C for 2 h.11
2.2.2. General method for the synthesis of MgFe2O4@Tris nanoparticles.
First, 1 g of MgFe2O4 produced in the previous step was dispersed in a mixture of 20 mL of H2O and 30 mL of EtOH for 20 min. Then, 2.0 g of tris(hydroxymethyl)aminomethane was added and refluxed for 24 h. Finally, the obtained MgFe2O4@Tris MNPs were separated by an external magnet and washed many times with water and dried at 80 °C.27
2.3. General method for the synthesis of pyrazolopyranopyrimidine derivatives
Initially, 1 mmol of hydrazine hydrate, 1 mmol of ethyl acetoacetate, and 2 mL of H2O were added to the reaction vessel. Then, 1 mmol of aldehyde, 1 mmol of barbituric acid and 10 mg of MgFe2O4@Tris were added to the reaction mixture, and the resulting mixture was stirred at 300 rpm at room temperature. The progress of the reaction was monitored by thin-layer chromatography (TLC). After completion of the reaction, 10 mL of hot ethanol at a temperature of about 60 °C was added to the reaction mixture to dissolve the solid product. The catalyst was separated using an external magnet. The solvent was evaporated, and the crude product was obtained. The pure product was prepared through recrystallization from hot ethanol. The obtained pure products were characterized using melting points, FT-IR spectra, 1H NMR spectra and 13C NMR spectra.
2.4. General method for the synthesis of tetrahydrodipyrazolopyridine derivatives
In a 5 mL round-bottomed flask, a mixture of ethyl acetoacetate (2 mmol), hydrazine hydrate (2 mmol), aldehyde (1 mmol), ammonium acetate (3 mmol), and MgFe2O4@Tris (15 mg) in water (2 mL) was stirred at 300 rpm at room temperature for an appropriate amount of time. The reaction progress was followed using thin-layer chromatography. After completion of the reaction, 10 mL of hot ethanol (at about 60 °C) was added to the reaction mixture to dissolve the solid product. Then, the catalyst was separated by a magnet. The solvent was evaporated, and the crude product was obtained. The pure product was prepared through recrystallization from hot ethanol. The obtained pure products were characterized using melting points, FT-IR spectra, 1H NMR spectra and 13C NMR spectra.
3. Results and discussion
3.1. Synthesis and characterization of MgFe2O4@Tris
MgFe2O4@Tris nanoparticles were successfully prepared by the method outlined in Scheme 2. First, magnesium ferrite MNPs were synthesized using the co-precipitation technique.11 Next, MgFe2O4@Tris was prepared by reacting MgFe2O4 with tris(hydroxymethyl)aminomethane in refluxed EtOH/H2O under nitrogen for 24 h.27 Next, the prepared catalyst was fully identified by various characterization methods such as FT-IR, VSM, EDX, EDX mapping, TEM, SEM, XRD, and TGA analysis.
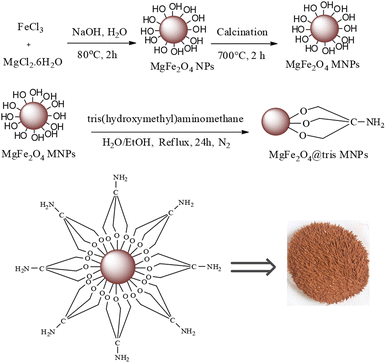 |
| Scheme 2 Stepwise synthesis of MgFe2O4@Tris MNPs. | |
3.1.1. FT-IR analysis.
The FT-IR spectra of MgFe2O4 and MgFe2O4@Tris MNPs are displayed in Fig. 1. The FT-IR spectrum of MgFe2O4 displayed bands at 3412 and 1627 cm−1 corresponding to the stretching and bending vibrations of the O–H bond. In addition, two peaks at 575 and 438 cm−1 correspond to Mg–O stretches (Fig. 1A). Peaks at 2855, 2923, and 1383 were observed in the FT-IR spectra of MgFe2O4@Tris due to stretching and bending vibrations in C–H aliphatic groups. The absorption peaks at 3430 and 1631 cm−1 are attributed to the stretching and bending vibrations of N–H groups. The vibrations at 1081 and 1150 cm−1 are related to C–C and C–N bonds (Fig. 1B).
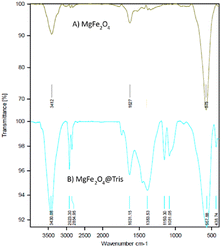 |
| Fig. 1 FT-IR spectra of (A) MgFe2O4 and (B) MgFe2O4@Tris MNPs. | |
3.1.2. XRD analysis.
To recognize the formation of the magnetite crystal phase in the nanocatalyst, the synthesized samples were analyzed by X-ray diffraction (XRD). The XRD analyses of MgFe2O4 (green) and MgFe2O4@Tris (red) nanoparticles are shown in Fig. 2a. The X-ray diffraction planes at (111), (220), (311), (400), (422), (511), (440), (533), (622), and (444) confirm the formation of a cubic spinel structure. The locations of all peaks in the XRD analysis of MgFe2O4 were matched to the standard XRD analysis of MgFe2O4.28 These peaks showed that the structure of MgFe2O4 MNPs corresponds to the standard pattern with reference card numbers ICSD: 96-100-6064, ICSD: 96-901-3215 and ICSD: 96-901-5066 (Fig. 2b). The particle size determined from the XRD data was 59.09 nm, which was calculated using the Debye–Scherrer formula (D = Kλ/β
cos
θ).
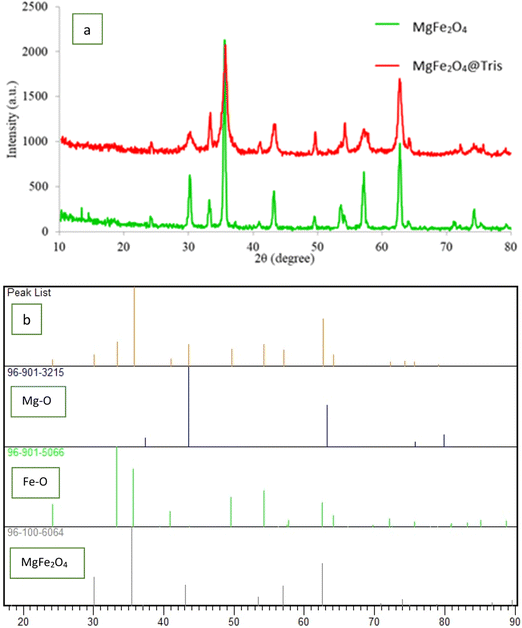 |
| Fig. 2 (a) XRD patterns of MgFe2O4 (green line) and MgFe2O4@Tris MNPs (red line). (b) Standard XRD patterns. | |
3.1.3. SEM analysis.
The morphology and particle size of the nano-solid magnesium ferrite were analyzed using scanning electron microscopy (SEM). As shown in Fig. 3, the images show a spherical structure with an average particle diameter of 65–90 nm and a uniform distribution and size.
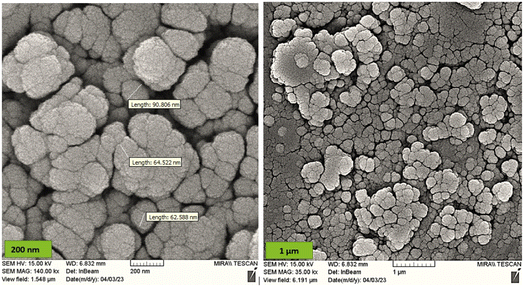 |
| Fig. 3 SEM images of MgFe2O4@Tris MNPs at different magnifications. | |
3.1.4. TEM analysis.
Transmission electron microscopy (TEM) analysis was used to examine the shape and size of the magnetic nanoparticles (Fig. 4). The images obtained confirm the formation of particles with a spherical morphology. Additionally, the particle size distribution histogram obtained from the TEM images revealed that the MgFe2O4@Tris nanoparticles have an average size of 46 nm.
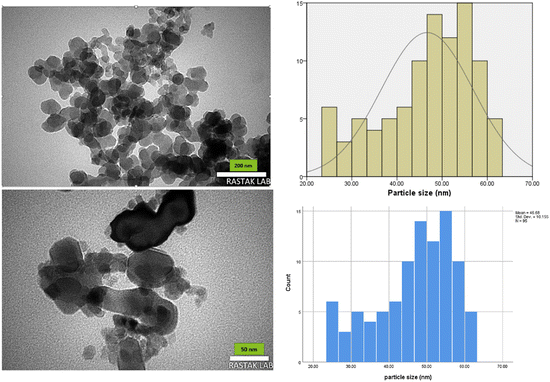 |
| Fig. 4 TEM images and histograms of MgFe2O4@Tris nanoparticles at different magnifications. | |
It is shown from SEM, TEM, and XRD analyses that the catalyst has a nanometer-sized structure.
3.1.5. EDX analysis.
Energy dispersive X-ray spectroscopy (EDX) is a powerful analytical tool for characterizing and determining elements present in catalyst components and the purity of the nanoparticles. The EDX spectrum of MgFe2O4@Tris is shown in Fig. 5, which confirms the elements Mg, Fe, O, N, and C in the nano-catalyst structure. Additionally, there are no peaks related to any impurities. It can be concluded that the nano-catalyst has been successfully produced. EDX mapping spectra of MgFe2O4@Tris confirm the synthesis of the catalyst. The elements were shown to be uniformly distributed in the nano-catalyst (Fig. 6).
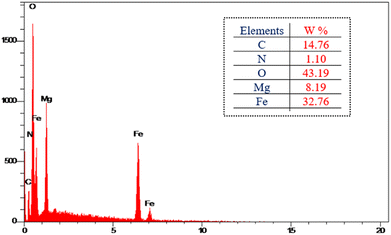 |
| Fig. 5 EDX spectrum of MgFe2O4@Tris MNPs. | |
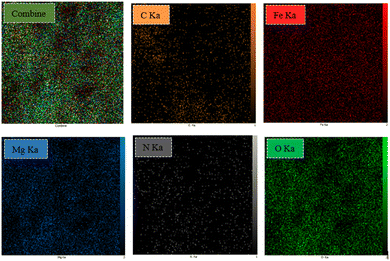 |
| Fig. 6 EDX mapping spectra of MgFe2O4@Tris MNPs. | |
3.1.6. TGA analysis.
The thermogravimetric analysis (TGA) diagram of the MgFe2O4@Tris nanoparticles is represented in Fig. 7. The TGA diagram of the MgFe2O4@Tris nanoparticles shows a small weight loss below 200 °C, corresponding to the evaporation of physically adsorbed solvents and OH groups on the nano-catalyst surface, and the next weight loss at temperatures above 200 °C is related to the removal of organic moieties on the MgFe2O4@Tris nanoparticles.
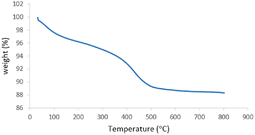 |
| Fig. 7 TGA thermogram of MgFe2O4@Tris MNPs. | |
3.1.7. VSM analysis.
The magnetization characteristics of the MgFe2O4 and MgFe2O4@Tris nanoparticles were analyzed using a vibrating sample magnetometer (VSM). The magnetization diagrams of MgFe2O4 (red line) and MgFe2O4@Tris (blue line) are shown in Fig. 8. The values of the saturation magnetization of the MgFe2O4 and MgFe2O4@Tris nanoparticles are 18 and 10 emu/g, respectively. The observed decrease in magnetic moment (MS) results from the addition of diamagnetic organic species to the surface of the MNPs, which confirms the presence of Tris on the surface of the MgFe2O4 nanoparticles. Meanwhile, the catalyst can be easily recycled from the reaction mixture using a simple magnetic field.
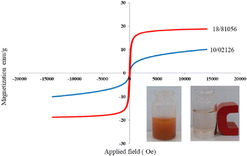 |
| Fig. 8 VSM diagram of MgFe2O4 (red line) and MgFe2O4@Tris MNPs (blue line). | |
3.2. Catalytic studies
Following the successful synthesis and full characterization of MgFe2O4@Tris MNPs, we investigated the efficiency and activity of this nano-catalyst in the synthesis of pyrazolopyranopyrimidine and tetrahydrodipyrazolopyridine derivatives.
3.2.1. Pyrazolopyranopyrimidine.
A four-component reaction between para-chlorobenzaldehyde, ethyl acetoacetate, hydrazine hydrate and barbituric acid was used as the sample reaction, and the effect of diverse conditions involving different temperatures, catalyst amounts, and solvents was examined. The results are summarised in Table 1. The best efficiency of product 2a is obtained when conducting the reaction in water at room temperature and in the presence of 10 mg of MgFe2O4@Tris MNPs (Table 1, entry 3). To verify the catalytic activity, we expanded the reaction to a range of aromatic aldehydes and barbituric acid under optimal reaction conditions (Scheme 3), and the results are reported in Table 2.
Table 1 Optimization of reaction conditions in the sample reactiona
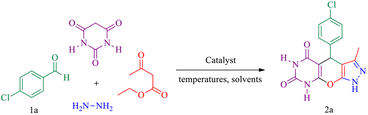
|
Entry |
Amount of catalyst (mg) |
Solvent |
Temp (°C) |
Time (min) |
Yieldb (%) |
Reaction conditions: hydrazine hydrate (1 mmol), ethyl acetoacetate (1 mmol), para-chlorobenzaldehyde (1 mmol) and barbituric acid (1 mmol) in the presence of catalyst and solvent (2 mL).
Isolated yield.
|
1 |
30 |
H2O |
rt |
15 |
98 |
2 |
20 |
H2O |
rt |
10 |
98 |
3
|
10
|
H
2
O
|
rt
|
10
|
98
|
4 |
5 |
H2O |
rt |
10 |
90 |
5 |
10 |
H2O/EtOH |
rt |
10 |
97 |
6 |
10 |
EtOH |
rt |
20 |
85 |
7 |
10 |
DMF |
rt |
30 |
75 |
8 |
10 |
CH3CN |
rt |
50 |
65 |
9 |
10 |
DCM |
rt |
120 |
20 |
10 |
10 |
EtOAc |
rt |
60 |
75 |
11 |
10 |
n-Hexane |
rt |
120 |
Trace |
12 |
10 |
H2O |
40 |
10 |
98 |
13 |
10 |
H2O |
60 |
10 |
98 |
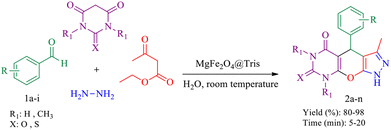 |
| Scheme 3 Synthesis of pyrazolopyranopyrimidine derivatives catalysed by MgFe2O4@Tris MNPs. | |
Table 2 Synthesis of pyrazolopyranopyrimidine derivatives catalysed by MgFe2O4@Tris MNPs
Entry |
Aldehyde |
R1 |
X |
Product |
Time (min) |
Yielda (%) |
M.p. (°C) (ref.) |
Isolated yield.
|
1 |
4-Cl C6H5 |
H |
O |
2a
|
10 |
98 |
218–220 (ref. 15) |
2 |
C6H5 |
H |
O |
2b
|
7 |
98 |
215–218 (ref. 15) |
3 |
4-OMe C6H5 |
H |
O |
2c
|
15 |
97 |
226–228 (ref. 15) |
4 |
2-OH C6H5 |
H |
O |
2d
|
10 |
97 |
264–268 (ref. 17) |
5 |
2-OMe C6H5 |
H |
O |
2e
|
15 |
92 |
228–230 (ref. 15) |
6 |
3-OH C6H5 |
H |
O |
2f
|
10 |
94 |
278–280 (ref. 30) |
7 |
3-NO2 C6H5 |
H |
O |
2g
|
20 |
80 |
266–268 (ref. 17) |
8 |
4-Br C6H5 |
H |
O |
2h
|
20 |
95 |
210–212 (ref. 30) |
9 |
4-Cl C6H5 |
H |
S |
2i
|
5 |
98 |
224–226 (ref. 35) |
10 |
4-OMe C6H5 |
H |
S |
2j
|
10 |
92 |
224–225 (ref. 15) |
11 |
C6H5 |
H |
S |
2k
|
5 |
94 |
219–220 (ref. 15) |
12 |
2-OH C6H5 |
H |
S |
2l
|
5 |
95 |
267–270 (ref. 35) |
13 |
2-OMe C6H5 |
H |
S |
2m
|
5 |
97 |
222–225 (ref. 15) |
14 |
4-Cl C6H5 |
CH3 |
O |
2n
|
10 |
98 |
200–202 (ref. 15) |
A proposed mechanism for the synthesis of pyrazolopyranopyrimidines using MgFe2O4@Tris MNPs as the catalyst is shown in Scheme 4.15
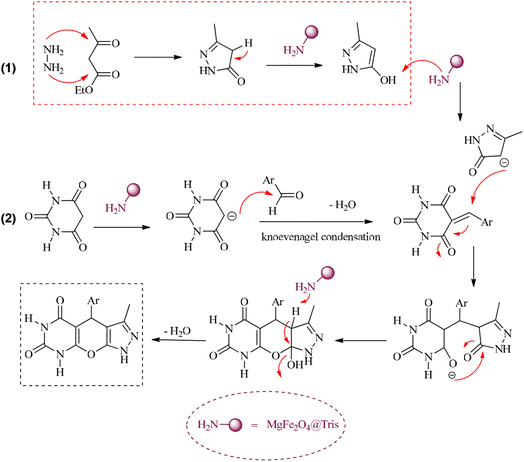 |
| Scheme 4 Proposed mechanism for the synthesis of pyrazolopyranopyrimidines using MgFe2O4@Tris MNPs. | |
3.2.2. Tetrahydrodipyrazolopyridine.
We used MgFe2O4@Tris MNPs to prepare tetrahydrodipyrazolopyridine derivatives (Scheme 5). To optimize the amount of catalyst, solvent, and reaction temperature, the reaction of ethyl acetoacetate, hydrazine hydrate, para-chlorobenzaldehyde, and ammonium acetate was selected as the model reaction (Table 3). The best efficiency of product 3a is achieved when the reaction is carried out in H2O as the solvent at room temperature and in the presence of 15 mg of MgFe2O4@Tris MNPs (Table 3, entry 3). Subsequently, a vast range of tetrahydrodipyrazolopyridine derivatives was synthesized with success under optimal conditions with excellent yields and short reaction times, and the results of these reactions are presented in Table 4. The suggested mechanism for the production of tetrahydrodipyrazolopyridine using MgFe2O4@Tris MNPs as a catalyst is shown in Scheme 6.38
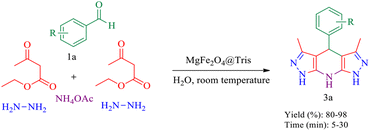 |
| Scheme 5 Synthesis of tetrahydrodipyrazolopyridine derivatives catalysed by MgFe2O4@Tris MNPs. | |
Table 3 Optimization of reaction conditions in the sample reactiona
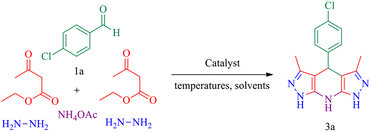
|
Entry |
Amount of catalyst (mg) |
Solvent |
Temp (°C) |
Time (min) |
Yieldb (%) |
Reaction conditions: hydrazine hydrate (2 mmol), ethyl acetoacetate (2 mmol), para-chlorobenzaldehyde (1 mmol) and ammonium acetate (1.5 mmol) in the presence of catalyst and solvent (2 mL).
Isolated yield.
|
1 |
30 |
H2O |
rt |
10 |
98 |
2 |
20 |
H2O |
rt |
10 |
98 |
3
|
15 |
H
2
O
|
rt
|
10
|
98
|
4 |
10 |
H2O |
rt |
15 |
90 |
5 |
15 |
H2O/EtOH |
rt |
10 |
95 |
6 |
15 |
EtOH |
rt |
45 |
50 |
7 |
15 |
DMF |
rt |
30 |
95 |
8 |
15 |
CH3CN |
rt |
120 |
20 |
9 |
15 |
EtOAc |
rt |
120 |
Trace |
10 |
15 |
n-Hexane |
rt |
120 |
Trace |
11 |
15 |
H2O |
40 |
10 |
98 |
12 |
15 |
H2O |
60 |
10 |
98 |
Table 4 Synthesis of tetrahydrodipyrazolopyridine derivatives catalysed by MgFe2O4@Tris MNPs
Entry |
Aldehyde |
Product |
Time (min) |
Yielda (%) |
M.p. (°C) (ref.) |
Isolated yield.
|
1 |
4-Cl C6H5 |
3a
|
10 |
98 |
252–254 (ref. 16) |
2 |
C6H5 |
3b
|
5 |
98 |
241–243 (ref. 16) |
3 |
4-OMe C6H5 |
3c
|
20 |
90 |
187–190 (ref. 16) |
4 |
2-OMe C6H5 |
3d
|
15 |
93 |
180–182 (ref. 37) |
5 |
2-NO2 C6H5 |
3e
|
20 |
88 |
189–190 (ref. 16) |
6 |
3-NO2 C6H5 |
3f
|
20 |
85 |
284–286 (ref. 16) |
7 |
4-Br C6H5 |
3g
|
25 |
91 |
169–170 (ref. 16) |
8 |
4-OH C6H5 |
3h
|
20 |
92 |
269–270 (ref. 36) |
9 |
2-Cl C6H5 |
3i
|
15 |
90 |
165–167 (ref. 16) |
10 |
4-NO2 C6H5 |
3j
|
20 |
80 |
298–300 (ref. 16) |
11 |
3-Br C6H5 |
3k
|
30 |
91 |
247–248 (ref. 16) |
12 |
(CH3)2 N C6H5 |
3l
|
30 |
90 |
240–243 (ref. 16) |
13 |
Terephthalaldehyde |
3m
|
10 |
96 |
>300 (ref. 37) |
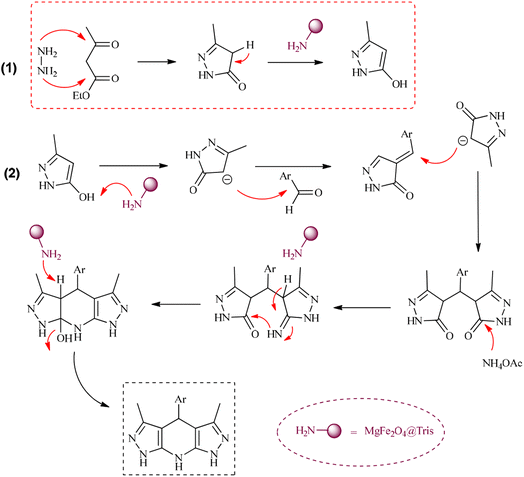 |
| Scheme 6 Proposed mechanism for the synthesis of tetrahydrodipyrazolopyridine using MgFe2O4@Tris MNPs. | |
3.2.3. Comparison with other catalysts.
To demonstrate the superiority and high efficiency of the nano-catalyst synthesized in this research, the results obtained for the synthesis of pyrazolopyranopyrimidines and tetrahydrodipyrazolopyridines using MgFe2O4@Tris MNPs were compared with the catalysts reported in previous studies. As shown in Table 5, this nano-catalyst has advantages such as high catalytic activity, low reaction time and excellent product yields.
Table 5 Comparison of the catalytic activity of MgFe2O4@Tris MNPs with other catalysts in the synthesis of products 2a (entries 1–5) and 3a (entries 6–10)
Entry |
Catalyst |
Conditions |
Time (min) |
Yield (%) |
Ref. |
1 |
[BNPs-Caff]HSO4 |
H2O, 50 °C |
45 |
92 |
29
|
2 |
β-Cyclodextrin |
H2O, 40 kHz, 50 °C |
30 |
90 |
30
|
3 |
[MerDABCO-SO3H]Cl |
H2O, 80 °C |
15 |
94 |
17
|
4 |
H3[PMo7W5O40]·24H2O |
Solvent free, 80 °C |
40 |
94 |
31
|
5
|
MgFe
2
O
4
@Tris
|
H
2
O, rt
|
10
|
98
|
This work
|
6 |
Pseudopolymeric magnetic nanoparticles |
EtOH, rt |
60 |
63 |
16
|
7 |
HANCD@urease |
H2O, 70 °C |
70 |
95 |
32
|
8 |
Nano-CdZr4(PO4)6 |
EtOH, reflux |
40 |
92 |
33
|
9 |
Nano-ovalbumin |
H2O, 55 °C |
35 |
94 |
34
|
10
|
MgFe
2
O
4
@Tris
|
H
2
O, rt
|
10
|
98
|
This work
|
3.2.4. Catalyst recovery and reuse.
One of the important features of the catalyst synthesized in this study is its magnetism. This feature simplifies catalyst recovery and separation. The possibility of reusing the MgFe2O4@Tris nano-catalyst in the synthesis of products 2a and 3a (as model reactions) was considered. The results are presented in Fig. 9. In summary, the catalytic activity was tested over 5 cycles, and similar activities were displayed, without any notable loss of the original catalytic activity. Additionally, the FT-IR spectra and SEM images of MgFe2O4@Tris before and after recovery (Fig. 10 and 11) exhibited good similarity due to the stability of the catalyst.
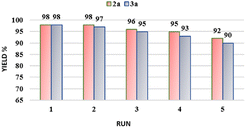 |
| Fig. 9 Possibility of reusing MgFe2O4@Tris MNPs in the synthesis of products 2a and 3a. | |
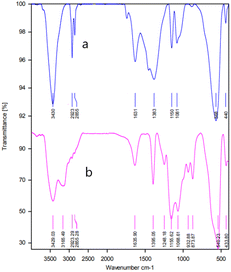 |
| Fig. 10 FT-IR spectrum of fresh MgFe2O4@Tris (a) and MgFe2O4@Tris after recovery (b). | |
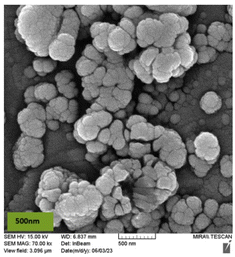 |
| Fig. 11 SEM images of MgFe2O4@Tris MNPs after recovery. | |
4. Conclusions
We introduced an innovative magnetic heterogeneous nano-catalyst, made from MgFe2O4 nanoparticles and tris(hydroxymethyl)aminomethane using a co-precipitation method. Various techniques were used for the full characterization of the synthesized nanocatalysts. The catalytic activity of this nanocatalyst for the synthesis of pyrazolopyranopyrimidine and tetrahydrodipyrazolopyridine derivatives was evaluated. A key feature of this protocol was a high product yield, and this catalyst can simply be separated from the reaction mixture using an external magnet and reused for multiple cycles without appreciable loss of its original catalytic activity. To summarize this study, this method can serve as a powerful strategy for the synthesis of important active molecules.
Author contributions
All authors contributed to data analysis, supervised the project, drafted and revised the paper and agreed to be responsible for all aspects of this work.
Conflicts of interest
The authors declare no conflicts of interest.
Acknowledgements
The authors are grateful to Ilam University for supporting this work.
Notes and references
-
(a) M. I. Anik, M. K. Hossain, I. Hossain, A. M. U. B. Mahfuz, M. T. Rahman and I. Ahmed, Nano Sel., 2021, 2, 1146–1186 CrossRef CAS;
(b) M. P. Nikolova and M. S. Chavali, Biomimetics, 2020, 5, 27 CrossRef CAS.
- P. T. Sekoai, C. N. M. Ouma, S. P. du Preez, P. Modisha, N. Engelbrecht, D. G. Bessarabov and A. Ghimire, Fuel, 2019, 237, 380–397 CrossRef CAS.
-
(a) L. Gloag, M. Mehdipour, D. Chen, R. D. Tilley and J. J. Gooding, Adv. Mater., 2019, 31(48), 1904385 CrossRef CAS;
(b) E. Asadian, M. Ghalkhani and S. Shahrokhian, Sens. Actuators, B, 2019, 293, 183–209 CrossRef CAS.
- M. R. Asghar, M. T. Anwar, A. Naveed and J. Zhang, Membranes, 2019, 9, 78 CrossRef CAS PubMed.
-
(a) C. Wang and D. Astruc, Prog. Mater. Sci., 2018, 94, 306–383 CrossRef CAS;
(b) N. Wang, Q. Sun and J. Yu, Adv. Mater., 2019, 31(1), 1803966 CrossRef.
- E. Katz, Magnetochemistry, 2020, 6, 6 CrossRef.
- H. Qin, Y. He, P. Xu, D. Huang, Z. Wang, H. Wang, Z. Wang, Y. Zhao, Q. Tian and C. Wang, Adv. Colloid Interface Sci., 2021, 294, 102486 Search PubMed.
- Q. Chen, A. J. Rondinone, B. C. Chakoumakos and Z. John Zhang, J. Magn. Mater., 1999, 194, 1–7 CrossRef CAS.
- D. Narsimulu, B. N. Rao, N. Satyanarayana and E. S. Srinadhu, ChemistrySelect, 2018, 3, 8010–8017 CrossRef CAS.
-
(a) T. P Sumangala, I. Pasquet, L. Presmanes, Y. Thimont, C. Bonningue, N. Venkataramani, S. Prasad, V. Baco-Carles, P. Tailhades and A. Barnabé, Ceram. Int., 2018, 44, 18578–18584 CrossRef;
(b) J. Patil, D. Nadargi, I. S. Mulla and S. S. Suryavanshi, Mater. Lett., 2018, 213, 27–30 CrossRef CAS.
-
(a) R. Eisavi and K. Naseri, RSC Adv., 2021, 11, 13061–13076 RSC;
(b) N. G. Salunkhe, C. A. Ladole, N. V. Thakare and A. S. Aswar, Res. Chem. Intermed., 2018, 44, 355–372 CrossRef CAS.
- M. Adel, M. A. Ahmed and A. A. Mohamed, J. Phys. Chem. Solids, 2021, 149, 109760 CrossRef CAS.
- R. C. Cioc, E. Ruijter and R. V. A. Orru, Green Chem., 2014, 16, 2958–2975 RSC.
-
Modern Heterocyclic Chemistry, ed. J. Alvarez-Builla, J. J. Vaquero and J. Barluenga, Wiley, 2011 Search PubMed.
- H. Rostami and L. Shiri, ChemistrySelect, 2019, 4, 8410–8415 CrossRef CAS.
- M. Dashteh, M. Yarie, M. A. Zolfigol, A. Khazaei and S. Makhdoomi, Appl. Organomet. Chem., 2021, 35(6), e6222 CrossRef CAS.
- P. Patil, A. Yadav, L. Bavkar, N. B N, N. D. Satyanarayan, A. Mane, A. Gurav, S. Hangirgekar and S. Sankpal, J. Mol. Struct., 2021, 1242, 130672 CrossRef CAS.
- K. T. Patil, D. K. Jamale, N. J. Valekar, P. T. Patil, P. P. Warekar, G. B. Kolekar and P. V. Anbhule, Synth. Commun., 2017, 47, 111–120 CrossRef CAS.
- M. E. A. Zaki, H. A. Soliman, O. A. Hiekal and A. E. Rashad, Z. Naturforsch., C, 2006, 61, 1–5 CrossRef CAS PubMed.
- S. C. Kuo, L. J. Huang and H. Nakamura, J. Med. Chem., 1984, 27, 539–544 CrossRef CAS.
- R. O. Saleh, H. Achmad, B. T. Daminov, H. H. Kzar, A. B. Mahdi, A. T. Hammid, M. K. Abid, M. J. C. Opulencia, Y. F. Mustafa and H. Sharma, Front. Chem., 2022, 10, 928047 CrossRef CAS.
- R. R. Chinthaparthi, V. L. Chittiboena, S. Jorepalli and C. S. R. Gangireddy, J. Heterocycl. Chem., 2021, 58, 1104–1116 CrossRef CAS.
- K. S. Gudmundsson, B. A. Johns and S. H. Allen, Bioorg. Med. Chem. Lett., 2008, 18, 1157–1161 CrossRef CAS PubMed.
- H. de Mello, A. Echevarria, A. M. Bernardino, M. Canto-Cavalheiro and L. L. Leon, J. Med. Chem., 2004, 47, 5427–5432 CrossRef CAS PubMed.
- T. M. Dhameliya, H. A. Donga, P. V. Vaghela, B. G. Panchal, D. K. Sureja, K. B. Bodiwala and M. T. Chhabria, RSC Adv., 2020, 10, 32740–32820 RSC.
-
(a) T. M. Dhameliya, R. J. Patel, R. H. Amin, D. K. Sureja and K. B. Bodiwala, Mini-Rev. Org. Chem., 2023, 20, 800–817 CrossRef CAS;
(b)
Advanced Nanocatalysis for Organic Synthesis and Electroanalysis, ed. T. M. Dhameliya, R. J. Patel, N. D. Gajjar, R. H. Amin, K. B. Bodiwala and D. K. Sureja, Bentham Science Publishers, 2022, pp. 114–157 Search PubMed.
- M. Rezaie Kahkhaie, N. Hazeri, M. T. Maghsoodlou and A. Yazdani-Elah-Abadi, Res. Chem. Intermed., 2021, 47, 5007–5025 CrossRef CAS.
- F. Naaz, H. K. Dubey, C. Kumari and P. Lahiri, SN Appl. Sci., 2020, 2, 808 CrossRef CAS.
- M. Bakherad, R. Doosti, M. Mirzaee and K. Jadidi, Iran. J. Catal., 2017, 7, 27–35 CAS.
- S. V. Akolkar, N. D. Kharat, A. A. Nagargoje, D. D. Subhedar and B. B. Shingate, Catal. Lett., 2020, 150, 450–460 CrossRef CAS.
- D. S. Aher, K. R. Khillare, L. D. Chavan and S. G. Shankarwar, Monatsh. fur Chem., 2022, 153, 79–85 CrossRef CAS.
- F. Tamaddon and D. Arab, RSC Adv., 2019, 9, 41893–41902 RSC.
- J. Safaei-Ghomi, H. Shahbazi-Alavi, R. Sadeghzadeh and A. Ziarati, Res. Chem. Intermed., 2016, 42, 8143–8156 CrossRef CAS.
- N. Salehi and B. B. F. Mirjalili, Res. Chem. Intermed., 2018, 44, 7065–7077 CrossRef CAS.
- A. Patil, S. Gajare, G. Rashinkar and R. Salunkhe, Catal. Lett., 2020, 150, 127–137 CrossRef CAS.
- G. Mohammadi Ziarani, M. Khademi, F. Mohajer, M. Anafcheh, A. Badiei and J. B. Ghasemi, Res. Chem. Intermed., 2022, 48, 2111–2133 CrossRef CAS.
- M. Lashanizadegan, K. Nikoofar, A. Aghaei, F. Mehrikaram and H. Mirzazadeh, Solid State Sci., 2019, 95, 105937 CrossRef.
- F. Tamaddon, E. Ahmadi-AhmadAbadi and H. Kargar, Appl. Organomet. Chem., 2021, 35(11), e6386 CrossRef CAS.
|
This journal is © The Royal Society of Chemistry 2024 |
Click here to see how this site uses Cookies. View our privacy policy here.