DOI:
10.1039/D3RA07506K
(Paper)
RSC Adv., 2024,
14, 7290-7302
Surfactant modified coconut husk fiber as a green alternative sorbent for micro-solid phase extraction of triazole fungicides at trace level in environmental water, soybean milk, fruit juice and alcoholic beverage samples
Received
3rd November 2023
, Accepted 26th February 2024
First published on 1st March 2024
Abstract
In this work, micro-solid phase extraction using surfactant modified biosorbent was investigated for trace level determination of triazole fungicides prior to their analysis by high performance liquid chromatography. Coconut husk fiber (CHF) was selected as an effective biosorbent in the extraction process. Fourier transform infrared spectrometry, scanning electron microscopy and transmission electron microscopy methods were used to characterize the modified biosorbent. Various factors affecting the extraction efficiency of the proposed method were studied including the amount of coconut husk fiber biosorbent (0.1 g), kind and concentration of surfactant as a modifier (sodium dodecyl sulfate, 10 mmol L−1), kind and volume of desorption solvent (methanol, 150 μL), and extraction period (including vortex adsorption time, centrifugation adsorption time, vortex desorption time and centrifugation adsorption time approximately 10 min). Under the selected conditions, the calibration plot was found to be linear in the range of 9–300 μg L−1 with a coefficient for determination of greater than 0.99. The limits of detection and limits of quantification for the studied triazole fungicides were 3.00 and 9.00 μg L−1, respectively. Finally, the proposed method was successfully applied to determine triazole fungicides in environmental water, soybean milk, fruit juice and alcoholic beverage samples with acceptable recoveries obtained in the range of 67.0% to 105.0%.
Introduction
The use of pesticides in agriculture is essential to manage a variety of pests that could damage crops, and also to improve the quality of the food.1 The triazole class is one of the new generations of pesticides recently presented in the agricultural field. Triazole fungicides are extensively used in agriculture because of their antifungal activity against a wide variety of crop diseases.2 Triazole fungicides inhibit one specific enzyme, C14-demethylase, which plays a role in sterol production. Sterols such as ergosterol, are needed for membrane structure and function, making them essential for the development of functional cell walls. Therefore, these fungicides result in abnormal fungal growth and eventually death.3 The class of triazoles, such as propiconazole, triticonazole, tebuconazole, hexaconazole, and diniconazole are particularly used to prevent and control some diseases, for instance, sheath blight and false smut, blast, and fruits spots.4 Because of their characteristics such as high chemical and photochemical stability, low biodegradability and easy transport in the environment, make them persist in soil and water. It poses a potential threat to human health and environmental protection.5,6 In order to minimize the impact of pesticide residues to humans and the environment, the acceptable level in food and biological samples is usually defined by the maximum residue limit (MRL). The MRLs of hexaconazole and diniconazole in fruits and vegetables for human consumption were set at 0.01–0.05 mg kg−1 by Codex Alimentarius Commission (CAC). For example, the MRL of hexaconazole, triadimefon and bitertanol is 0.01–0.02 mg kg−1; the MRL of tebuconazole is 0.02–5.0 mg kg−1; and the MRL of myclobutanil is 0.05–3.0 mg kg−1. Due to their extensive uses and the hazards associated with them, the determination of these emerging contaminants requires the innovative development of effective and robust analytical approaches to control human exposure.
The analytical techniques for quantification of triazole fungicides in food and beverages are mostly based on chromatographic techniques i.e. high-performance liquid chromatography (HPLC),7 liquid chromatography with mass spectrometry (LC-MS),8 gas chromatography (GC) and gas chromatography with mass spectrometry (GC-MS).6 Sample clean-up and enrichment methods are recommended prior to instrumental analysis to enhance the sensitivity and obtain accurate results. In this respect, preconcentration method are usually required, such as liquid–liquid extraction (LLE),9 liquid phase microextraction (LPME),10 dispersive liquid–liquid microextraction (DLLME),11 air-agitated liquid–liquid microextraction (AALLME),1 solid phase extraction (SPE),12 solid phase micoextraction (SPME)13 and micro-solid-phase extraction (μ-SPE).14
Micro-solid-phase extraction (μ-SPE) or adsorbent-based microextraction represents one of versatile sample preparation methods, which has many advantages, such as simplicity, low sample, and organic solvent consumption, rapidity, and high reusability.15 These approaches are more advantages in comparison with solvent-based extraction because of their less need for amount of sorbent and toxic solvents.16 The adsorbents used in μ-SPE have a significant influence on the efficient extraction of analytes. Currently, many types of research are focused on improving the enrichment and extraction capacity of adsorbents for target analytes. Various sorbents have been used including molecularly imprinted polymers (MIPs),17,18 molecularly imprinted nanospheres (MINs)19 and biosorbents.20 Biosorbents, which is one of sorbents, have recently been successfully employed in adsorption processes.20 Coconut palm (Cocos nucifera) is one of the essential crops in Southeast Asia. However, the current utilization ratio of coconut is less than 50% of their annual output and substantial amounts of waste coconut husk have been generated after processing of its edible components such as coconut milk and coconut meat.21 Porous materials have drawn our attention owing to their inherent advantages, such as high surface area, satisfactory adsorption capacity and good chemical stability.22 Compared to the background, incorporating the advantages of modification on coconut husk fiber porous materials to construct an alternative adsorbent used in μ-SPE with improved extraction performance and adsorption capacity is enormously significant. On the other hand, the high surface area and large pore volume of coconut husk fiber porous materials play a key role in enhancing extraction efficiency, which not only provides for enhancing the contact area but also can increase active sites.21,22 Therefore, the coconut husk fiber powder was found to be a green sorbent, biodegradable, reduces the use of solvent, and simple, rapid, and inexpensive adsorbent in μ-SPE. These potential advantages of coconut husk fiber offer a perspective as adsorbents used in μ-SPE to extract trace triazole fungicides from complex samples. Coconut husk fiber (CHF) having high surface areas, are hydrophilic in nature, and show limited adsorption capacities for hydrophobic compounds. However, they can be modified by the coating surfactant to enhance their adsorptive tendency towards organic compounds.23 The surfactants are adsorbed to the surface of mineral oxides through attractive electrostatic interactions between the charged mineral oxide surface and the oppositely charged surfactant head group resulting in the formation of monolayers of adsorbed surfactant termed hemimicelles.
The present work was intended to assess the use of surfactant modified coconut husk fiber (CHF) powder for the extraction of triazole fungicides in environmental water, soybean milk, fruit juice, and alcoholic beverage samples. The parameters affecting the extraction efficiency were investigated and the proposed method was applied to analyze complex sample matrices. The proposed method was compared with other extraction methods previously reported.
Experimental
Chemicals and reagents
All chromatography-grade solvents including acetonitrile, methanol, ethanol and propanol were purchased from Merck KGaA, Germany. Sodium dodecyl sulfate (SDS) was obtained from Merck Schuchardt OHG, Germany. Cetyltrimethylammonium bromide (CTAB) and sodium dodecylbenzene sulfonate (SDBS) were obtained from Sigma-Aldrich, Germany. The analytical standards of triazole including myclobutanil, triadimefon, tebuconazole, hexaconazole and diniconazole were purchased from Dr Ehren-storfer GmbH, Germany. Stock standard solution of each triazole fungicide (1000 μg mL−1) was prepared by dissolving an appropriate amount in methanol and stored at 4 °C. Working standard solutions was prepared by diluting the stock standard solution with water. Deionized water (Millipore Waters, USA) with the resistivity of 18.2 MΩ cm was used throughout the experiments.
Instruments
The morphology of surfactant modified coconut husk fiber (CHF) powder were characterized by transmission electron microscope (TEM; Model FEI, Technai G2 F20, USA) and scanning electron microscope (SEM; Model JEOL JSM-6460LV, Canada). Extention process of the functional group after surface modification was performed with a Bruker INVENIO-S Fourier transformed infrared spectrometer (Bruker corp, Massachusetts, USA) at 4000–400 cm−1 using diamond lens attenuated total resistance (ATR). Phase separation were completed by centrifugation agitator (Centurion, England). A vortex mixer (Fisher Scientific, USA) was also used.
A Waters 1525 Binary HPLC pump system (Water, Massachusetts, USA) equipped with Waters 2489 UV visible detection (Water, Massachusetts, USA) was operated for separation and detection of triazole fungicides. The separation was carried out using a Purospher® STAR RP-18 endcapped (150 × 4.6 mm, 5 μm) column (Merck, Germany) with isocratic elution of acetonitrile and water (50
:
50 v/v) as mobile phase, at a flow rate of 1.0 mL min−1. The injection volume was 20 μL. The detection wavelength was set at 220 nm. The results were acquired using Empower 3 software to utilize and control the system as well as for data processing.
Preparation of biosorbent
Coconut husk fiber (CHF) were obtained from Roi-Et province, Northeast, Thailand. The fibers were divided from the husks and, then washed by distillation water and dried at 65 °C until CHF material was dry up. After that, they were sifted through sieves with pore sizes of 180 μm. The powder was kept in desiccator before used.
Micro solid phase extraction (μ-SPE) procedure
Schematic diagram of the μ-SPE method using coconut husk fiber powder prior to HPLC analysis is showed in Fig. 1. Coconut husk fiber (CHF) powder (0.1) g was added into 15 mL conical centrifuge tube which containing standard or sample solution (10.00 mL). After that, 150 μL of 10 mmol per L sodium dodecyl sulphate was added. The solution was then vortexed for 20 s and centrifuged at 3500 rpm for 5 min to enhance the sorption of analytes into sorbent. After that, the supernatant was removed. Then, 150 μL of methanol was added to elute the target analytes. The mixture was vortexed for desorption process at 1500 rpm for 10 s and centrifuged at 3500 rpm for 5 min. The clear supernatant was collected and then filtered through a 0.45 μm membrane filter before analysis by HPLC.
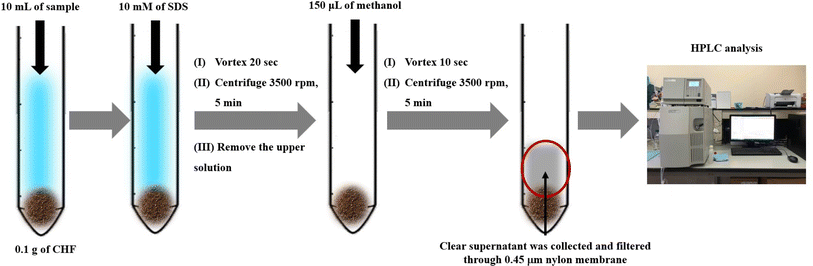 |
| Fig. 1 Schematic diagram of the proposed micro solid phase extraction followed by HPLC-UV analysis. | |
Sample preparation
In this study, the environmental waters were obtained from the different natural located near agricultural in Maha Sarakham province, Northeast, Thailand. These samples were filtered through a Whatman (no. 1) filter paper and passed through 0.45 μm nylon membrane filter before extract using the proposed method.
Two kinds of commercial fruit juice samples including, orange, and pomegranate were purchased from the local supermarket in Maha Sarakham province, Northeast, Thailand. Each fruit juice 10 g was centrifuged at 4000 rpm for 10 min and the supernatant was collected. The supernatant was diluted with ultrapure water at ratio (1
:
2).24
Two kinds of soybean milk samples were purchased from the local supermarket in Maha Sarakham province, Northeast, Thailand. Sample (1.0 mL) was added into 15 mL centrifuge tube. After that, 5 mL of acetonitrile and 1 mL of 0.1 mol per L trifluoroacetic acid were added before applying to vortex for 3 min. The solution was then centrifuged at 4500 rpm for 10 min. These samples were filtered through a Whatman (no. 1) filter paper and passed through 0.45 μm nylon membrane filter before extract using the proposed method.
Alcoholic beverage samples including beers and wines (alcohol content as 5%) were purchased from different supermarkets in Maha Sarakham province, Northeast, Thailand. At initial, each alcoholic sample (10 mL) were degassed for 15 min in ultrasonic bath, and then stored at room temperature. After degassing, the samples were filtered through 0.45 μm nylon membrane filter before analysis.
Results and discussion
Characterization of the coconut husk fiber (CHF)
Fourier transformed infrared (FTIR) spectra was used to characterize the structure of cellulose, hemicellulose, and lignin of coconut husk fiber after the pretreatment method. The FT-IR spectra of CHF, SDS modified CHF-adsorption and SDS modified CHF-desorption are illustrated in Fig. 2(a–c), respectively. The similarities of cellulose fiber (as shown in Fig. 2) indicated that there were no significant changes in the structure of the coconut husk fiber before and after pretreatment.
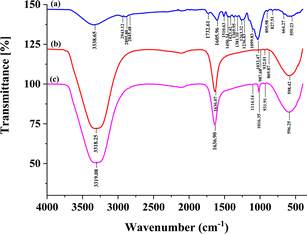 |
| Fig. 2 FTIR spectra of (a) coconut husk fiber (CHF), (b) SDS modified CHF after adsorption of triazole fungicides (100 μg L−1 each) and (c) SDS modified CHF after desorption process. | |
The absorption bands are observed in two wavenumber regions of 3660–2900 cm−1 and 1630–900 cm−1. The presence of peaks on the spectra of cellulose samples coming from CHF corresponds to bands of microcrystalline cellulose (as shown in Fig. 2(a)). In contrast, differences in absorption band intensities and the appearance of new peaks in spectra of CHF-adsorption are observed. Identification of the absorption bands is ensuring. The attended peaks in the wave number range of 3660–2900 cm−1 are characteristic of the stretching vibration of O–H and C–H bonds in polysaccharides. The broad peak at 3331 cm−1 is characteristic of the stretching vibration of the hydroxyl group in polysaccharides.25 This peak also includes inter- and intra-molecular hydrogen bond vibrations in cellulose.26 The band at 2895 cm−1 is referred to CH stretching vibration of all hydrocarbon ingredients in polysaccharides.27 But the peak of –CH stretching of hydrocarbon in polysaccharides does not appear in CHF-adsorption and CHF-desorption spectra because a coconut husk fiber modified by surfactant when passing through the adsorption and desorption process interaction between functional group on CHF surface with target analytes have changed.
Typical bands entrusted to cellulose were inspected in the region of 1630–900 cm−1. The peaks located at 1636.07 cm−1 correspond to vibration of water molecules absorbed in cellulose.25 The absorption bands at 1428, 1367, 1334, 1027 and 896 cm−1 belong to stretching and bending vibrations of –CH2 and –CH, –OH and C–O bonds in cellulose.28 Moreover, CHF-adsorption and CHF-desorption in this procedure show that the adsorption bands shifted at 987.68, 932.01, and 598.42 cm−1, and desorption bands shifted at 1114.14, 1016.35, 931.91, and 596.25 cm−1 belong to stretching and bending vibrations of –CH2 and –CH, –OH, and C–O bonds, respectively in cellulose due to the pretreatment, adsorption, and desorption process. The band at around 1420–1430 cm−1 is associated with the amount of the crystalline structure of the cellulose, while the band at 897 cm−1 is assigned to the amorphous region in cellulose.29 The aforementioned peak appeared in the corresponding FTIR results of CHF, CHF-adsorption, and CHF-desorption.
SEM image of coconut husk fiber powder are shown in Fig. 3. The SEM result presents the morphological properties of cellulose in CHF (Fig. 3(a)) and after adsorption process (Fig. 3(b)). It exhibited that porosity can be observed along composite structure due to the μ-SPE procedure; the porosity can be formed by modification of CHF-adsorption process. It was remarkable to note that the porous structure was affected by surfactant coated and adsorption of target analytes in micro extraction procedure. The irregular size/shape and interconnected structure as channels were observed. It was important to note that the amount of pore and pore radius was reduced when they were replaced by triazoles in the adsorption procedure. In desorption process as shown in Fig. 3(c), it was found that the size, shape, and interconnected structure of CHF pores decreased.
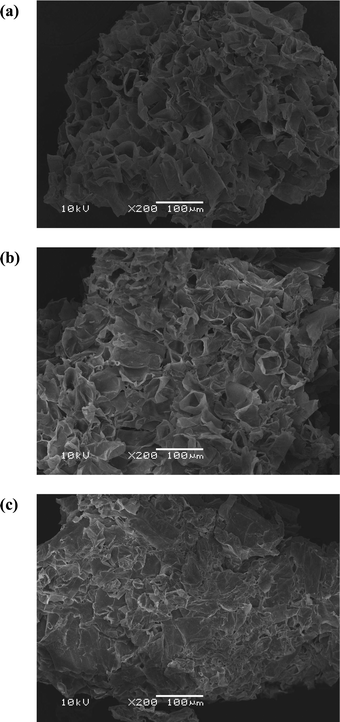 |
| Fig. 3 SEM images of (a) coconut husk fiber, (b) SDS modified CHF after adsorption with triazole fungicides (100 μg L−1 each) and (c) SDS modified CHF after desorption process. | |
Transmission Electron Microscopy (TEM) are also studied (as shown in Fig. 4). The TEM image for coconut husk fiber powder without adsorption procedure (as shown in Fig. 4(a)) showed the microparticles of coconut husk fiber powder as sheet-shaped particles. The images of the TEM for surfactant modified coconut husk fiber powder after the adsorption procedure (Fig. 4(b)) with triazole fungicides (100 μg L−1 each) are seen as tiny spherical particles clinging to the sheet-shaped particles of fiber structure in CHF. In the desorption procedure, some parts of CHF surface powder, and triazoles particles disappeared due to the desorption effect as shown in Fig. 4(c).
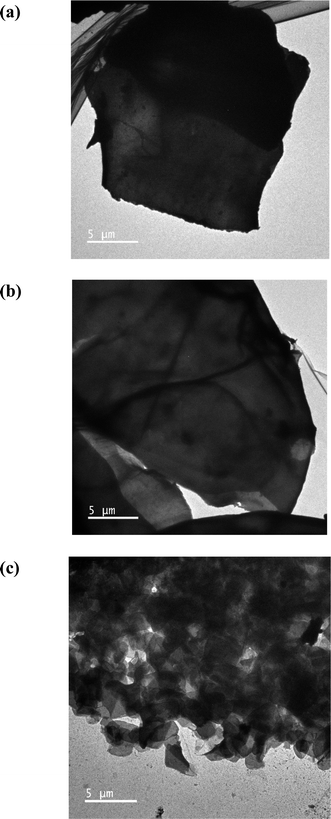 |
| Fig. 4 TEM images of (a) coconut husk fiber, (b) SDS modified CHF after adsorption with triazole fungicides (100 μg L−1 each) and (c) SDS modified CHF after desorption process. A single projector lens may provide a range of magnification of 5 : 1, for all images. | |
Optimization of μ-SPE conditions
Some important parameters were investigated including the amount of coconut husk fiber (CHF), kind and concentration of surfactant, kind and volume of desorption solvent and extraction period. Thus, the μ-SPE procedure required to optimize and obtain the optimal extraction conditions. In this section, the optimization was carried out on an aqueous solution containing 100 μg L−1 of each analyte and the parameters were performed by modifying one at a time while keeping the remaining constant.
Effect of amount of coconut husk fiber (CHF)
One of the most important parameters of the μ-SPE procedure is to determine the effective amount of the coconut husk fiber (CHF). In order to obtain a high extraction efficiency, amounts of CHF were investigated in the range of 0.03–0.3 g. Other parameters were held constant as follows: sample volume 10 mL; 150 μL of 10 mmol per L sodium dodecyl sulphate; vortexed 20 s; centrifuged at 3500 rpm 5 min and methanol (150 μL) as desorption solvent. As illustrated in Fig. 5(a), the extraction efficient in term of peak area of all triazoles increased with increasing CHF amount and reached to the maximum at 0.1 g. And then, peak area of triazoles decreased. This might be that increase in the amount of CHF could be the absorbent trend to aggregation, which led to a negative effect on the binding site between the CHF and the target analytes. Therefore, 0.1 g of CHF was employed in the further experiment.
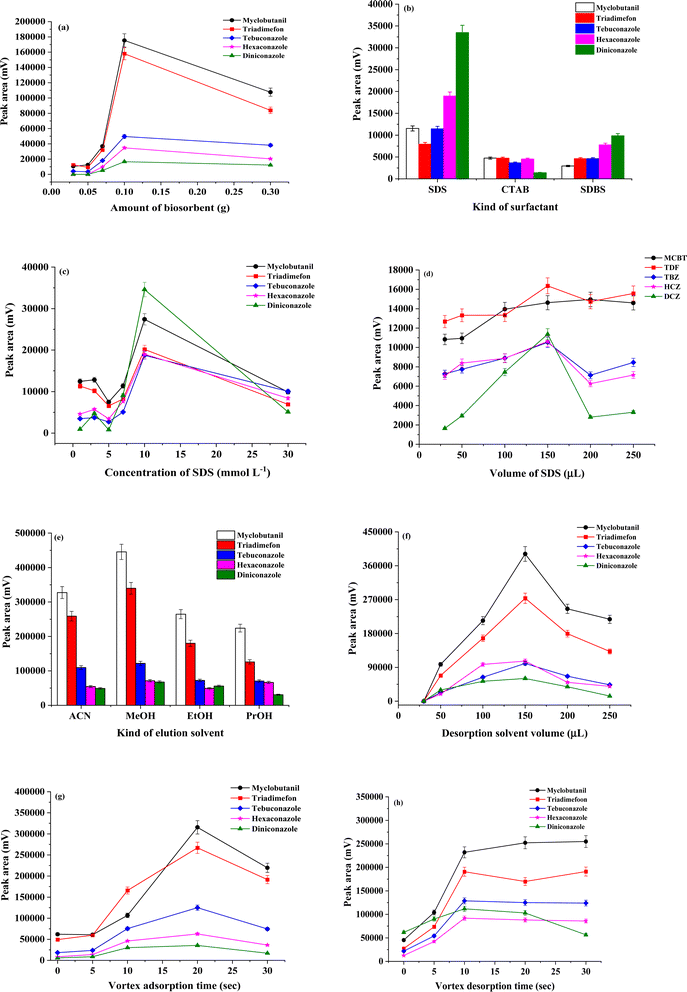 |
| Fig. 5 Effect of experimental condition on the extraction efficiency: (a) amount of biosorbent (coconut husk fiber powder), (b) kind of surfactant, (c) concentration of SDS, (d) volume of SDS, (e) kind of desorption solvent, (f) desorption solvent volume, (g) vortex time adsorption, and (h) vortex time desorption. | |
Effect of surfactant as modifier and pH on adsorption
Surfactant molecules have a polar and non-polar moiety, so they can dissolve in water as monomers or be incorporated with other molecules to form a micelle.30 The surfactants could be adsorbed to the surface of CHF through attractive electrostatic interactions resulting in the formation of monolayers of adsorbed surfactant termed hemimicelles. After that, hydrophobic interactions between hydrocarbon chains of surfactant molecules result in the formation of admicelles.31 In term of hemimicelles, the hydrophobic tails are exposed to aqueous solution, so the surfactant-modified CHF surface will become hydrophobic, which favors the adsorption of non-ionic organic compounds onto the sorbents. Since possessing larger special areas and more ion-exchangeable OH groups on surface, modified CHF are expected to have obvious advantage for the sorption of ionic surfactant, and behave remarkable adsorption capability to organic compounds correspondingly.32 Various surfactants, the cationic CTAB, and non-ionic Triton X-114, apart from the anionic SDBS and SDS, were evaluated. The percentage of each surfactant used was calculated by considering their respective critical micelle concentrations (CMC). The CMC refers to the concentration above which a surfactant forms micelle,33 depends on various factors. In this case, the concentration chosen of SDS, CTAB, SDBS, and Triton X-114 as additives were slightly below their corresponding CMC values (8.2 mmol L−1, 1.0 mmol L−1, 0.61, and 0.24 mmol L−1, respectively). Bad chromatographic signal was obtained when using Triton X-114. The results in Fig. 5(b) shows that SDS was the most effective in enhancing the extraction performance of CHF. SDS coated on CHF, with its polar group protruding towards the aqueous phase,34 assisted to reduce the surface tension between the hydrophobic CHF and the solution surface. Therefore, triazole molecules dissolved in the solution were effectively closer to the sorbent. The pH directly affects the adsorption process of different class of molecules in water. Additionally, the zero point of charge (zpc) of CHF occurs at about pH 5–6. In other words, CHF surface is positively charged at acidic conditions (pH < 6), whereas it is negatively charged in a more alkaline media (pH > 6). CHF surface must be positively charged to adsorb any anionic surfactant through ionic interaction.35 Which, all the cycle of the triazole is positively charged and the positive poles are the atoms of carbon and hydrogen causing a desirable electrostatic attraction between the SDS on the CHF surface and protonated analytes resulting in improved extraction efficiency of the analytes. The other three surfactants did not share this advantage provided by SDS.
The concentration of SDS functionalized on CHF was explored at concentrations below and above the CMC level (between 0.5 and 30 mmol L−1, while keeping other parameter constant: CHF amount 0.3 g, sample volume 10 mL; vortexed 20 s; centrifuged at 3500 rpm 5 min and methanol (150 μL) as desorption solvent) to determine the optimum interaction with CHF for the most suitable extraction performance. As illustrated in Fig. 5(c), the extraction efficiencies of triazole fungicides generally increased when 1–10 mmol L−1 of SDS were used and decreased when the concentration of SDS was higher than 10 mmol L−1. Thus, 10 mmol L−1 of SDS was the optimum concentration of surfactant required to functionalize the CHF biosorbent. The reason for such trends to be observed was largely due to the change of interaction between SDS and the studied triazoles, as the concentration of SDS was increased. Below the CMC level (at 0.5 mmol L−1 of SDS), the extraction efficiency in terms of peak area decreased, mainly due to the slight electrostatic attraction. It was therefore estimated that 0.10 mmol L−1 of SDS was the most suitable concentration.
The effect of SDS volume was studied in the range of 30–250 μL using a concentration 0.10 mmol L−1 of SDS. Other parameters were held constant as follows: CHF amount 0.3 g; sample volume 10 mL; vortexed 20 s; centrifuged at 3500 rpm 5 min and methanol (150 μL) as desorption solvent. It was found that the highest peak area was obtained for all triazoles using an SDS volume of 150 μL (Fig. 5(d)). At higher volumes than 150 μL, the peak areas of all the analytes slightly decreased. Therefore, 150 μL of SDS was selected for further experiments.
Effect of sample volume
Sample volume is one of the most important factors to be investigated because it determines the extraction efficiency of the method. The effect of sample volume on the extraction efficiency was varied in the range of 5.00–15.00 mL (data not shown). Other parameters were held constant as follows: CHF amount 0.3 g; vortexed 20 s; centrifuged at 3500 rpm 5 min and methanol (150 μL) as desorption solvent. The peak areas of the analytes increased with increase of the sample volume from 3.00 to 10.00 mL, and then decreased with further increase of sample volume. Therefore, the sample volume of 10.00 mL was utilized in the subsequent experiments.
Effect of kind and volume of desorption solvent
Another important factor in extraction procedure is the desorption solvent. Reasonable desorption solvent is helpful to absolutely desorb the target TFs from the extraction bio-material in a short time. So as to improve the accuracy of the experiment, four typical organic solvents were compared, such as acetonitrile, methanol, ethanol, and isopropanol (as shown in Fig. 6(e)). Other parameters were held constant as follows: CHF amount 0.3 g; sample volume 10 mL; vortexed 20 s and centrifuged at 3500 rpm 5 min. The results in Fig. 5(e) indicated that methanol achieved the higher desorption ability than other desorption solvents for the target triazole fungicides. Therefore, methanol was used as the desorption solvent for further experiments.
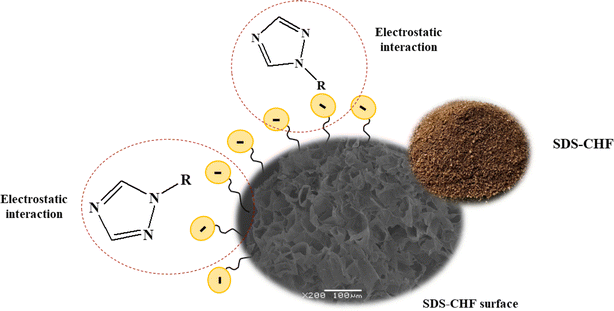 |
| Fig. 6 The modification process of SDS modified CHF involved proposed reactions mechanism. | |
The volume of desorption solvent should be attentively optimized as it can have a significant effect on the results of sensitivity and reproducibly in microextraction method. The desorption volume (30, 50, 100, 150, 200 and 250 μL) was also optimized (as shown in Fig. 5(f)) to ensure high desorption efficiency of studied fungicides. Other parameters were held constant as follows: CHF amount 0.3 g; sample volume 10 mL; vortexed 20 s; and centrifuged at 3500 rpm 5 min. It was found that, low volume of methanol 30 μL wasn't sufficient for desorb of target fungicides. After that the peak area constantly increased with increasing the volume of desorption solvent, the full desorption of all target fungicides was achieved by 150 μL. When the volume of methanol greather than 150 μL, the peak area of fungicides decreased due to the dilution effect. As a result, methanol 150 μL was selected.
Effect of adsorption time
In μ-SPE procedure is a stable-based technique, and the institution of equilibrium invest the rate of mass transfer along with the extraction time. Contributing an adequate contact time between the target analytes and CHF to reach an adsorption equilibrium is an important. The rate of mass transfer can be increased by vortex, and equilibrium can be obtained in an approximately shorter time. Vortex and centrifuge were another important parameter that could affect the separation of TFs from CHF powder. The vortex adsorption time was investigated between 0 and 30 s. Other parameters were held constant as follows: CHF amount 0.3 g; sample volume 10 mL; centrifuged at 3500 rpm 5 min; and methanol (150 μL) as desorption solvent. The results exhibited that when the vortex time was up to 20 s, extraction efficiency in terms of peak area was improved after which it gradually decreased. As can be seen in Fig. 5(g), 20 s was selected as the optimal vortex adsorption time.
The centrifugation rate and time were varied in the ranged of 1500–4500 rpm and 3–10 min, respectively. It was found that these parameters were effective (data not shown) and so 3500 rpm and 5 min were selected as centrifugation rate and time, respectively.
Effect of desorption time
The effect of desorption period on extraction efficiency was more evaluated by varying the vortex time from 0 to 30 s as shown Fig. 5(h). It was found that the extraction efficiency increases in term of peak area with the increase of time from 0 to 10 s. There was a slight change when vortex desorption time was over 10 s. The centrifugation rate and time of desorption were varied in the ranged of 1500–4500 rpm and 3–10 min, respectively. It was found that these parameters were effective and so 3500 rpm and 5 min were selected as centrifugation rate and time, respectively. Therefore, an optimum vortex time (10 s), centrifugation rate (3500 rpm) and centrifugation time (5 min) of desorption apparently was selected.
Stability and reusability
To assess the stability of SDS on CHF which evaluated in term of reproducibility of the production procedures for eleven triazoles across six different batches. The percentage of RSD were calculated (data not shown), the findings show high accuracy, with RSDs less than 8.1%. These favorable results indicate that the suggested SDS on CHF sorbent can be used to extract all the triazoles under investigation.
The reusability of the prepared surfactant modified coconut husk fiber was studied by using aqueous solution containing mixed TFs standard solution (each TF of 100 μg L−1). The sorbent was washed by methanol for 5 times between the cycles and then dried for the next SPE cycle (data not shown). It was found that the peak area of TFs had significant decrease after 3 SPE cycles, indicating that the prepared sorbent could be reused at least 3 times (data do not show). Extraction efficiency in term of peak area decreased thereafter because the sorbent was destroyed. This indicates that sorbent possesses excellent reusability as an efficient adsorbent.
Adsorption mechanism of CHF sorbent
The adsorption mechanism (as shown in Fig. 6) was used to estimate the capability of the proposed method. Mechanisms appear as a function of the physicochemical properties of the lignocellulosic in CHF sorbent and the interaction in a sample aqueous solution. These properties can be estimated as a function of surface charge, solubility, hydrophobicity, chemical components, reactivity, and molecular size.36 Individual properties specified and the sorption mechanism of interaction between CHF sorbent or while the appearance of struggle between the mechanisms target TFs have different closeness with active sites of sorbents, thus it is an essential to examine the entail mechanism. Important factors that influence the adsorption mechanism include (1) types and amount of lignocellulosic sorbent material, (2) the chemistry of target analytes solution, and (3) environmental conditions.37 Functional groups on CHF surfaces of lignocellulosic materials can cooperate with functional groups of TFs in several modes. The adsorption capacity of CHF can be ascribed to carboxylic, hydroxyl, and phenolic functional groups exhibit in hemicellulose and lignin.25 The adsorption procedure is mostly completed through ion exchange, electrostatic interaction, surface complexation, physical and chemical adsorption, diffusion, and/or precipitation, so existing different types of interaction with the surface of the material.
Surfactants are amphiphilic compounds, which contain a nonpolar hydrocarbon-chain bonded to a polar group (anionic, cationic, neutral or zwitterionic).38 They are used in extraction method including, liquid–liquid extraction and solid-phase extraction. In solid-phase extraction procedure, surfactants are used to increase the dispersion of sorbent particles in the bulk solution or to enhance sorption ability the surface of the solid material to increase the extraction properties of the sorbent. Overall, surfactants in extraction processes significantly improve the efficiency and selectivity of the procedures, as well as the solubility of the compounds, resulting in higher extraction recovery of the analytes and cost-effective sample preparation. Several mechanisms are involved in surfactant adsorption on TFs which have a positive charge. The main forces driving the adsorption of TFs are electrostatic. Anionic surfactant treatment of CHF developed a negative charge on the surface due to the adsorption of SDS, mainly through their alkyl groups. In physisorption, the electrostatic static interaction (section effect of surfactant as modifier and pH on adsorption) is observed between an adsorbate (target TFs) and SDS modified CHF surface. However, chemisorption occurred by the formation of chemical bonds between the surfaces of solid (adsorbent) and functional groups of target analytes.39 Herein, it is difficult to discard TFs being adsorbed from the adsorbent; therefore, chemisorption is of irreversible nature. In contrast, the adsorption mechanism in this study is like the physisorption procedure as a reversible, i.e., desorption of the adsorbate arises by increasing the temperature and does not require any activation energy.
Moreover, the addition of the surfactant also greatly influenced the extraction efficiency it favors mass transfer of analytes into the organic phase.40 Nonionic and ionic surfactants with different hydrophilic–lipophilic balance (HLB) values, which measure the degree of their hydrophilicity or lipophilicity.41 The surface of modified CHF will become hydrophobic, which favors the adsorption of non-ionic organic compounds onto the sorbents. Then, the extraction efficiency increased.
Evaluation of analytical method
To validate the analytical performance of the proposed method, the concerning analytical aspects of the method consist of linearity, coefficient for determination, enrichment factor (EF), the limit of detection (LOD), and limit of quantification (LOQ) were evaluated under the optimum conditions. The results are summarized in Table 1. The proposed method provides a good linearity of the five triazole fungicides were observed in the range 9–300 μg L−1 for all analytes with a coefficient for determination of greater than 0.99. LODs and LOQs were calculated based on signal to noise (S/N) of 3, and 10, respectively. LODs (3.00 μg L−1) and LOQs (9.00 μg L−1) of five triazole fungicides were obtained. The precision of the proposed method was invented by testing inter-day and intra-day variations. The inter-day values were evaluated by five replicates at two concentrations (50 and 100 μg L−1) in the same day, and the obtained relative standard deviation (RSD) values were in the range from 1.96 to 4.38%. The intra-day precision values were measured on three ensuring days with RSD values in the range from 0.63 to 4.25%. Good precision with RSDs were less than 5.0%. The enrichment factor (EF), defined as the concentration ratio of the analytes in the settled phase (Cset) later the sedimented phase and in the aqueous sample (Co), ranged from 2.7–25.9 folds. Chromatograms of the studied triazole fungicides obtained from direct HPLC is shown in Fig. 7(a) and preconcentrated by the proposed microextraction method is shown in Fig. 7(b).
Table 1 Analytical characteristics of the standards triazole fungicides
Analyte |
Linear range (μg L−1) |
Linear equation |
R2 |
LOD (μg L−1) |
LOQ (μg L−1) |
Intra-day precisiona (n = 5), RSD (%) |
Inter-day precisiona (n = 5), RSD (%) |
EF (Cex/Co)b |
tR |
Peak area |
tR |
Peak area |
Precision were calculated at the concentration of 50 and 100 μg L−1 of each triazole. Ratio of the concentration of the target analytes in extraction phase to the initial concentration in the original sample solutions. |
Myclobutanil |
9–300 |
y = 389 553x + 1864.5 |
0.9974 |
3 |
9 |
0.22 |
0.63 |
0.26 |
1.96 |
25.9 |
Triadimefon |
9–300 |
y = 314 270x + 1665.8 |
0.9964 |
3 |
9 |
0.25 |
0.99 |
0.28 |
2.30 |
34.9 |
Tebuconazole |
9–300 |
y = 452 574x + 105.21 |
0.9973 |
3 |
9 |
0.02 |
2.74 |
0.04 |
3.41 |
23.4 |
Hexaconazole |
9–300 |
y = 3878 92x + 3273.1 |
0.9959 |
3 |
9 |
0.04 |
2.89 |
0.09 |
3.58 |
12.7 |
Diniconazole |
9–300 |
y = 297 186x + 6728.6 |
0.9973 |
3 |
9 |
0.16 |
4.25 |
0.20 |
4.38 |
2.7 |
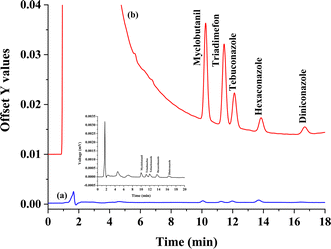 |
| Fig. 7 Chromatograms of standard triazole fungicides obtained by (a) without preconcentration and (b) with micro solid phase extraction using SDS modified CHF: concentration of all standards was 100 μg L−1. | |
Recovery studies and real sample analysis
The extraction of triazoles in different samples, including environmental water, fruit juice, soybean milk and alcoholic beverage samples, was accomplished according to the developed μ-SPE method. A matrix-matched calibration approach was used to evaluate the matrix effect in real samples. A set of matrix-matched calibration curves was prepared using representative samples spiked with 9–30 μg L−1 of each triazoles. The percentage of matrix effect (% ME) can be calculated by eqn (1):where Sm and Ss are the slopes of the calibration curve in the matrix and solvent, respectively. Typically, a ME between 80 and 120% suggests that there are no matrix effects, a ME between 50 and 80% or 120 and 150% corresponds to minor matrix effects, and a ME less than 50% or greater than 150% involves major matrix effects.38 It was found that minor MEs for environmental water and alcoholic beverage samples were observed, whereas major MEs were found in fruit juice, soybean milk (data not shown).
To test the accuracy, samples spiked at three different concentration levels (9, 15, and 30 μg L−1) were analyzed to determine the recoveries. The relative recoveries are summarized in Table 2. The acceptable relative recoveries (RR) were obtained in the range of 67.0–105% with RSD in the range from 0.18 to 4.59%. Fig. 8 show the overlaid chromatograms of sample and spike sample, as in Fig. 8(a) beer sample and Fig. 8(b) soybean milk sample. The results demonstrated that the proposed method based on CHF-μ-SPE coupled with HPLC performances accurate and dependable for microextraction method and determination triazole fungicides in real samples.
Table 2 Recovery obtained from the determination of studied triazoles in studied samples (n = 3)
Sample |
Spiked (μg L−1) |
% recovery at different spiked levels (% RSD, n = 3) |
MCBT |
TDF |
TBZ |
HCZ |
DCZ |
Agricultural water I |
9 |
91.6 (3.1) |
71.5 (1.3) |
86.9 (4.6) |
73.1 (2.2) |
73.2 (2.5) |
15 |
82.2 (1.5) |
87.2 (2.5) |
92.3 (1.4) |
97.8 (1.0) |
82.5 (1.3) |
30 |
86.2 (0.8) |
90.9 (2.5) |
80.1 (1.2) |
76.1 (0.5) |
72.1 (1.8) |
Agricultural water II |
9 |
72.5 (3.8) |
81.9 (1.5) |
83.2 (2.7) |
73.2 (2.9) |
97.1 (1.0) |
15 |
78.2 (2.8) |
86.4 (2.4) |
79.4 (3.7) |
88.3 (1.9) |
94.5 (1.6) |
30 |
96.3 (0.8) |
94.2 (3.2) |
92.5 (1.7) |
94.6 (0.3) |
97.5 (0.8) |
Soybean milk I |
9 |
76.9 (2.6) |
97.5 (1.8) |
78.7 (2.5) |
72.6 (4.2) |
79.9 (1.0) |
15 |
94.0 (2.6) |
105.0 (1.3) |
77.9 (0.2) |
91.6 (2.2) |
89.2 (2.0) |
30 |
100.2 (1.7) |
105.7 (0.6) |
86.1 (1.5) |
80.9 (1.0) |
87.7 (1.4) |
Soybean milk II |
9 |
71.7 (2.9) |
66.6 (3.7) |
67.0 (2.8) |
76.7 (1.8) |
81.8 (0.7) |
15 |
82.9 (2.0) |
75.7 (3.1) |
76.9 (0.8) |
82.2 (1.6) |
76.3 (0.9) |
30 |
84.4 (1.2) |
83.9 (0.9) |
89.4 (2.0) |
92.8 (1.4) |
91.4 (2.5) |
Soybean milk III |
9 |
70.1 (2.2) |
68.9 (2.6) |
67.8 (3.0) |
70.5 (1.8) |
95.8 (3.8) |
15 |
81.1 (2.8) |
74.1 (3.1) |
74.1 (3.4) |
73.2 (2.4) |
90.8 (1.1) |
30 |
89.4 (2.0) |
92.8 (1.4) |
91.4 (2.5) |
93.2 (1.7) |
80.9 (0.8) |
Orange juice |
9 |
81.9 (2.6) |
80.4 (2.6) |
90.9 (3.6) |
81.5 (2.7) |
90.2 (1.1) |
15 |
88.6 (2.9) |
98.8 (2.8) |
99.5 (1.5) |
99.6 (1.9) |
92.1 (0.6) |
30 |
91.2 (1.2) |
101.7 (1.3) |
99.8 (1.7) |
92.2 (1.8) |
99.2 (2.0) |
Pomegranate juice |
9 |
79.7 (4.1) |
67.5 (3.1) |
75.7 (4.0) |
67.7 (1.6) |
74.3 (3.7) |
15 |
84.8 (2.7) |
86.4 (1.7) |
88.9 (2.2) |
83.9 (1.9) |
83.9 (1.4) |
30 |
86.4 (0.5) |
93.1 (1.9) |
91.2 (1.6) |
87.2 (0.8) |
102.4 (1.7) |
Beer I |
9 |
82.3 (3.4) |
70.8 (4.1) |
76.0 (3.7) |
73.1 (3.2) |
74.4 (1.9) |
15 |
83.7 (2.0) |
95.2 (2.2) |
99.2 (2.7) |
99.0 (1.0) |
82.1 (0.3) |
30 |
91.9 (2.0) |
96.2 (2.5) |
95.1 (1.3) |
97.5 (1.3) |
102.9 (1.4) |
Beer II |
9 |
73.7 (1.8) |
68.1 (2.2) |
84.1 (3.8) |
67.4 (0.9) |
100.2 (2.3) |
15 |
99.5 (3.0) |
86.2 (3.8) |
92.7 (3.1) |
101.2 (1.2) |
98.8 (1.1) |
30 |
81.6 (2.5) |
93.2 (1.0) |
92.9 (0.6) |
97.8 (1.4) |
103.6 (1.3) |
Red wine |
9 |
68.9 (0.9) |
68.1 (2.3) |
71.8 (3.5) |
68.9 (1.6) |
73.2 (2.5) |
15 |
79.3 (2.2) |
75.6 (3.6) |
74.9 (4.5) |
73.4 (2.7) |
80.7 (0.8) |
30 |
81.3 (1.9) |
78.2 (1.2) |
75.9 (1.7) |
71.4 (0.8) |
102.4 (0.8) |
White wine |
9 |
68.6 (2.8) |
68.9 (2.2) |
67.8 (4.2) |
67.8 (3.0) |
72.5 (1.2) |
15 |
81.3 (2.0) |
80.9 (3.1) |
77.2 (3.6) |
80.2 (2.3) |
81.4 (1.5) |
30 |
88.0 (0.9) |
90.1 (0.7) |
90.2 (0.7) |
76.9 (1.0) |
101.7 (1.8) |
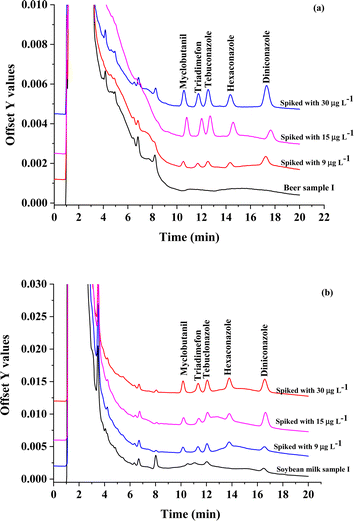 |
| Fig. 8 The Overlaid chromatograms of sample and spike sample, as in (a) beer sample and (b) soybean milk sample. | |
Assessment of method greenness
To estimate the green standpoint of the proposed method, analytical eco-scale evaluation tool was applied.42 Briefly, the analytical eco-scale approach provides quantitative information regarding the greenness of the analytical method in terms of the type and amount of reagents and solvents, instrument energy, occupational hazards, and waste generation. To determine the final eco-scale score, penalty points are allocated to each parameter, and the total penalty points are subtracted from 100. A set of penalty points (PPs) are used to assign the departure from ideal greenness. The PPs for hazardous chemicals can be determined by multiplying the sub-total PPs for a given quantity and the hazard. The ultimate AEC value can be calculated from “AEC = 100 − total PPs”. The scores higher than >75 illustrate an ideal green, the scores between 50 and 75 are acceptable green, and the scores <50 are insufficient green method.43 The final score for this method is 74 and it can be acceptable as a green method. The obtained results can be found in Table 3.
Table 3 The penalty points (PPs) for microextraction method for the determination of triazole fungicides in environmental water, soybean milk, fruit juice and alcoholic beverage samples
Reagent |
Penalty points (PPs) |
Instrument |
PPs |
Amount PP |
Hazard PP |
Total PPs (amount PP × hazard PP) |
ACN (<10 mL) |
1 |
4 |
4 |
HPLC-UV |
1 |
MeOH (<10 mL) |
1 |
6 |
6 |
Occupational hazard |
0 |
SDS (<10 mL) |
1 |
6 |
6 |
Waste |
5 |
TFA (<10 mL) |
1 |
2 |
2 |
Centrifuge |
1 |
|
|
|
|
Vortex |
1 |
Subtotal PP |
|
|
18 |
|
8 |
Total PPs |
|
|
26 |
|
|
Analytical eco-sale score (AEC) |
|
|
74 |
|
|
Comparison of the proposed method to other related methods
Table 4 exhibits the analytical performance of the established μ-SPE using surfactant modified coconut husk fiber compared with other microextraction methods6,7,37,44–48 used to determine triazole fungicides in various samples when comparing the type of sorbent, amount of sorbent, kind and volume of desorption solvent, extraction time, instrument, limit of detection (LOD), and relative recovery (RR) values achieved. Another advantage of this preparation of CHF biosorbent in the proposed method is simple and rapid, there was no specific and expensive reagent involved in the reactions, while the previous study used some expensive, specific reagents which were difficult to synthesize. Some studies have used commercial sorbent that is easy to use but expensive. It is a good choice for the development of adsorbents in accordance with the principle of green analytical chemistry. In addition, the method provided favorable LOD and RR values. Consequently, the proposed μ-SPE using surfactant modified coconut husk fiber as a biosorbent was a simple, rapid, and efficient pretreatment method for the determination of triazole fungicides via HPLC.
Table 4 Comparisons of the proposed method with other methods for the quantitation of triazole fungicidesa
Extraction method |
Sorbent |
Amount of sorbent (g) |
Desorption solvent |
Extraction time (min) |
Linear range (μg L−1) |
Recovery (%) |
LOD |
EF |
% RSD |
Ref. |
*NR: not reported. ** SPME-HPLC-DAD; solid phase micro extraction-high performance liquid chromatography-diode array detector, MSPE-GC-FID; magnetic solid-phase extraction-gas chromatography-flame ionization detector, SBSE-HPLC-DAD; stir bar sorption extraction-high performance liquid chromatography-diode array detector, QuECHERS-LC-MS/MS; quick, easy, cheap, effective, rugged, and safe-liquid chromatography tandem mass spectrometry, SPE-HPLC-MS/MS; solid phase extraction-high performance liquid chromatography tandem mass spectrometry, PT-SPE-GC-FID; pipette-tip solid-phase extraction-gas chromatography-flame ionization detector, UA-dSPME-GC-FID; ultrasonic assisted dispersive solid phase microextraction-gas chromatography-flame ionization detector. *** GO-poly-3-aminophenol; graphene oxide-poly 3-aminophenol, MOPs; magnetic porous organic polymers, HC-POF; hydroxyl-containing porous organic framework, BCDP; bridged bis(β-cyclodextrin)-bonded chiral stationary phase, Fe3O4-MWCNT@MOF-199; magnetic-multiwalled carbon nanotubes @metal organic framework-199, CAs; carbon aerogels, CMC/Zn (BDC)/GO; carboxymethylcellulose/Zn-based metal–organic framework/graphene oxide. |
SPME-HPLC-DAD |
GO-poly-3-aminophenol |
0.03 |
Methanol (5 mL) |
15 |
0.5–100 |
95.2–98.0 |
LOQ: 0.2–0.4 μg L−1 |
190–196 |
2.8–4.2 |
37 |
MSPE-GC-FID |
MOPs |
5.00 |
Ethyl acetate (400 μL) |
30 |
0.5–200 |
84.7–117 |
0.12–0.19 μg L−1 |
845–903 |
2.6–6.8 |
6 |
SBSE-HPLC-DAD |
HC-POF |
0.05 |
Acetonitrile (200 μL) |
50 |
0.1–500 |
80.7–111 |
0.022–0.071 μg L−1 |
49–57 |
6.4–12.4 |
7 |
QuECHERS-LC-MS/MS |
BCDP |
NR |
Acetonitrile (20 mL) |
7 |
50–20000 |
76.1–103.4 |
0.05–0.1 μg kg−1 |
0.43–0.57 |
4.8–11.9 |
44 |
SPE-HPLC-MS/MS |
Fe3O4-MWCNT@ MOF-199 |
0.01 |
Acetonitrile: ammonium water (7 : 3 v/v, 4 mL) |
20 |
5–500 |
63.1–90.3 |
0.25–1.83 μg L−1 |
NR |
0.06–6.1 |
45 |
PT-SPE-GC-FID |
CAs |
0.005 |
Acetonitrile (500 μL) |
NR |
0.24–200 mg kg−1 |
81–119 |
0.08–16 mg kg−1 |
NR |
0.4–8.5 |
46 |
UA-dSPME-GC-FID |
CMC/Zn (BDC)/GO |
0.015 |
Ethanol (100 μL) |
10 |
1–1000 |
83–86 |
0.3–1.5 ng mL−1 |
419–426 |
3.4–7.3 |
47 |
μSPE- HPLC-DAD |
Coconut husk fiber |
0.1 |
Methanol (150 μL) |
10 |
9–300 |
67–105 |
3 μg L−1 |
2.7–25.9 |
<5 |
This work |
Conclusion
In this work, surfactant modified coconut husk fiber as a green sorbent for trace determination of triazole fungicides was developed. The surface of modified CHF will become hydrophobic, which favors the adsorption of non-ionic organic compounds onto the sorbents. CHF modification also enhanced the mechanical properties of cellulose and lignocellulosic while enhancing the extraction performance of the CHF materials. Meanwhile, the CHF as the biosorbent in μ-SPE method not only reduced the agricultural waste device but also resolved the use of expensive commercial adsorbents in the extraction process. The CHF-μ-SPE assessment was established for analyzing some trace triazole fungicides in various samples followed by HPLC. This method showed good relative recoveries, high sensitivity, and satisfied repeatability. Furthermore, this method indicated several benefits including simplicity, the use of a small amount of sample and organic solvent, relatively low cost, easy elution of analytes, and short time analysis. Ultimately, the analytical method can be applied to the determination of trace triazole fungicides from other complex samples. The green analytical way of μ-SPE using CHF is demonstrated to be more tested and employed in the advancement of CHF materials in different fields.
Conflicts of interest
There are no conflicts to declare.
Acknowledgements
The authors gratefully acknowledge financial supports for this research from Thailand Science Research and Innovation (TSRI), Mahasarakham University, and Center of Excellence for Innovation in Chemistry (PERCH-CIC), Ministry of Higher Education, Research and Innovation. R. Kachangoon and J. Vichapong would like to thank the National Research Council of Thailand (NRCT): (N41A640218).
References
- M. A. Farajzadeh, M. R. A. Mogaddam and A. A. Aghdam, J. Chromatogr. A, 2013, 1300, 70–78 CrossRef CAS PubMed.
- M. Kahle, I. J. Buerge, A. Hauser, M. D. Müller and T. Poiger, Environ. Sci. Technol., 2008, 42(19), 7193–7200 CrossRef CAS PubMed.
- H. Zhang, M. Qian, X. Wang, X. Wang, H. Xu, Q. Wang and M. Wang, J. Sep. Sci., 2012, 35(7), 773–777 CrossRef CAS PubMed.
- J. Hafizovic, M. Bjørgen, U. Olsbye, P. D. C. Dietzel, S. Bordiga, C. Prestipino, C. Lamberti and K. P. Lillerud, J. Am. Chem. Soc., 2007, 129(12), 3612–3620 CrossRef CAS PubMed.
- K. A. Lewis, J. Tzilivakis and D. J. Warner, Hum. Ecol. Risk Assess., 2016, 22(4), 1050–1064 CrossRef CAS.
- D. Li, M. He, B. Chen and B. Hu, J. Chromatogr. A, 2019, 1601, 1–8 CrossRef CAS PubMed.
- Y. Wang, M. He, B. Chen and B. Hu, J. Chromatogr. A, 2020, 1633, 461628 CrossRef CAS PubMed.
- M. E. Hergueta-Castillo, E. López-Rodríguez, R. López-Ruiz, R. Romero-González and A. Garrido Frenich, Food Chem., 2022, 368, 130860 CrossRef CAS PubMed.
- Y. Wen, J. Li, F. Yang, W. Zhang, W. Li, C. Liao and L. Chen, Talanta, 2013, 106, 119–126 CrossRef CAS PubMed.
- F. Barahona, A. Gjelstad, S. Pedersen-Bjergaard and K. E. Rasmussen, J. Chromatogr. A, 2010, 1217(13), 1989–1994 CrossRef CAS PubMed.
- M. Pastor-Belda, I. Garrido, N. Campillo, P. Viñas, P. Hellín, P. Flores and J. Fenoll, Food Chem., 2017, 233, 69–76 CrossRef CAS PubMed.
- A. J. A. Charlton and A. Jones, J. Chromatogr. A, 2007, 1141(1), 117–122 CrossRef CAS PubMed.
- A. Menezes Filho, F. N. dos Santos and P. A. de Paula Pereira, Talanta, 2010, 81, 346–354 CrossRef CAS PubMed.
- Y. Wang, S. Jin, Q. Wang, G. Lu, J. Jiang and D. Zhu, J. Chromatogr. A, 2013, 1291, 27–32 CrossRef CAS PubMed.
- C. Wu, L. Wang, H. Li and S. Yu, Food Chem., 2019, 292(5), 260–266 CrossRef CAS PubMed.
- F. Augusto, E. Carasek, R. G. C. Silva, S. R. Rivellino, A. D. Batista and E. Martendal, J. Chromatogr. A, 2010, 1217(16), 2533–2542 CrossRef CAS PubMed.
- M. Arabi, A. Ostovan, J. Li, X. Wang, Z. Zhang, J. Choo and L. Chen, Adv. Mater., 2021, 33, 2100543 CrossRef CAS PubMed.
- M. Arabi, A. Ostovan, A. R. Bagheri, X. Guo, L. Wang, J. Li, X. Wang, B. Li and L. Chen, TrAC, Trends Anal. Chem., 2020, 128, 115923 CrossRef CAS.
- M. Arabi, A. Ostovan, A. R. Bagheri, X. Guo, J. Li, J. Ma and L. Chen, Talanta, 2020, 215, 120933 CrossRef CAS PubMed.
- C. S. T. Araújo, V. N. Alves, H. C. Rezende and N. M. M. Coelho, Microchem. J., 2010, 96(1), 82–85 CrossRef.
- M. A. S. Azizi Samir, F. Alloin and A. Dufresne, Biomacromolecules, 2005, 6(2), 612–626 CrossRef PubMed.
- Y. B. Jiang, L. H. Wei, Y. Z. Yu and T. Zhao, eXPRESS Polym. Lett., 2007, 1(5), 292–298 CrossRef CAS.
- S. Rubio and D. P. Bendito, TrAC, Trends Anal. Chem., 2003, 22, 470 CrossRef CAS.
- I. A. Senosy, H. M. Guo, M. N. Ouyang, Z. H. Lu, Z. H. Yang and J. H. Li, Food Chem., 2020, 325, 126944 CrossRef CAS PubMed.
- M. Poletto, V. Pistor, M. Zeni and A. J. Zattera, Polym. Degrad. Stab., 2011, 96(4), 679–685 CrossRef CAS.
- M. C. Popescu, C. M. Popescu, G. Lisa and Y. Sakata, J. Mol. Struct., 2011, 988, 65–72 CrossRef CAS.
- M. F. Rosa, E. S. Medeiros, J. A. Malmonge, K. S. Gregorski, D. F. Wood, L. H. C. Mattoso, G. Glenn, W. J. Orts and S. H. Imam, Carbohydr. Polym., 2010, 81(1), 83–92 CrossRef CAS.
- F. Xu, J. Yu, T. Tesso, F. Dowell and D. Wang, Appl. Energy, 2013, 104, 801–809 CrossRef CAS.
- M. Poletto, H. L. Ornaghi Júnior and A. J. Zattera, Materials, 2014, 7(9), 6105–6119 CrossRef PubMed.
- Y. Huang, Q. Zhou and G. Xie, J. Hazard. Mater., 2011, 193, 82–89 CrossRef CAS PubMed.
- A. Moral, M. D. Sicilia, S. Rubio and D. Perez-Bendito, Anal. Chim. Acta, 2006, 569, 132–138 CrossRef CAS.
- H. Y. Niu, Y. Q. Cai, Y. L. Shi, F. S. Wei, S. F. Mou and G. B. Jiang, J. Chromatogr. A, 2007, 1172, 113–120 CrossRef CAS PubMed.
- H. R. Sobhi, M. Ghambarian, M. Behbahani and A. Esrafili, J. Chromatogr. A, 2017, 1518, 25–33 CrossRef CAS PubMed.
- C. Richard, F. Balavoine, P. Schultz, T. W. Ebbesen and C. Mioskowski, Science, 2003, 300(5620), 775–778 CrossRef CAS PubMed.
- K. Press-Kristensen, E. Lindblom, J. E. Schmidt and M. Henze, Water Sci. Technol., 2008, 57(8), 1253–1256 CrossRef CAS PubMed.
- F. de S. Dias, L. A. Meira, C. N. Carneiro, L. F. M. dos Santos, L. B. Guimarães, N. M. M. Coelho, L. M. Coelho and V. N. Alves, TrAC, Trends Anal. Chem., 2023, 158, 116891 CrossRef CAS.
- S. Iftekhar, D. L. Ramasamy, V. Srivastava, M. B. Asif and M. Sillanpää, Chemosphere, 2018, 204, 413–430 CrossRef CAS PubMed.
- M. Chen, Q. Yi, J. Hong, L. Zhang, K. Lin and D. Yuan, Anal. Methods, 2015, 7, 1896–1905 RSC.
- M. N. Sahmoune, Environ. Chem. Lett., 2019, 17, 697–704 CrossRef CAS.
- K. Molaei, A. A. Asgharinezhad, H. Ebrahimzadeh, N. Shekari, N. Jalilian and Z. Dehghani, J. Sep. Sci., 2015, 38, 3905–3913 CrossRef CAS PubMed.
- K. Cherkashina, M. Voznesenskiy, O. Osmolovskaya, C. Vakh and A. Bulatov, Talanta, 2020, 214, 120861 CrossRef CAS PubMed.
- A. Gałuszka, Z. M. Migaszewski, P. Konieczka and J. Namieśnik, TrAC, Trends Anal. Chem., 2012, 37, 61–72 CrossRef.
- H. Sereshti, M. Zarei-Hosseinabadi, S. Soltani and M. Taghizadeh, Food Chem., 2022, 396, 133743 CrossRef CAS PubMed.
- M. Kadivar and A. Aliakbar, Food Chem., 2019, 299, 125127 CrossRef CAS PubMed.
- Y. Shuang, T. Zhang, H. Zhong and L. Li, Food Chem., 2021, 345, 128842 CrossRef CAS PubMed.
- G. Liu, M. Tian, M. Lu, W. Shi, L. Li, Y. Gao, T. Li and D. Xu, J. Chromatogr. B, 2021, 1166, 122500 CrossRef CAS PubMed.
- H. Sun, J. Feng, J. Feng, M. Sun, Y. Feng and M. Sun, Food Chem., 2022, 395, 133633 CrossRef CAS PubMed.
- Z. Aladaghlo, S. Javanbakht, A. Sahragard, A. Reza Fakhari and A. Shaabani, Food Chem., 2023, 403, 134273 CrossRef CAS PubMed.
|
This journal is © The Royal Society of Chemistry 2024 |