DOI:
10.1039/D3RA07417J
(Paper)
RSC Adv., 2024,
14, 2453-2465
Experimental and in silico insights: interaction of dimethyl sulphoxide with 1-hexyl-2-methyl imidazolium bromide/1-octyl-2-methyl imidazolium bromide at different temperatures
Received
31st October 2023
, Accepted 3rd January 2024
First published on 12th January 2024
Abstract
Ionic liquids have gained attention as ‘designer solvents’ since they offer a broad spectrum of properties that can be tuned by altering the constituent ions. In this work, 1-alkyl-2-methyl imidazolium-based ionic liquids with two different alkyl chains (alkyl = hexyl and octyl) have been synthesized and characterized. Since the binary mixture of ionic liquids with molecular solvents can give rise to striking physicochemical properties, the interaction of the synthesized room temperature ionic liquids, 1-hexyl-2-methyl imidazolium bromide [HMIM][Br]/1-octyl-2-methyl imidazolium bromide [OMIM][Br] with DMSO has been examined through density and specific conductance at T = (303.15, 308.15, 313.15 and 318.15) K under atmospheric pressure. The obtained molar volume and excess molar volume are fitted to the Redlich–Kister polynomial equation, and the standard deviation is noted. The positive excess molar volume at elevated temperatures indicates volume expansion due to the mutual loss of dipolar association and differences in the sizes and shapes of the constituent molecules. To have a better understanding of the reactivity and efficacy of 1-hexyl-2-methyl imidazolium bromide and 1-octyl-2-methyl imidazolium bromide with DMSO, the Becke, 3-parameter, Lee–Yang–Parr (B3LYP) correlation function of density functional theory (DFT) has been used. The ORCA Program version 4.0 calculates the highest occupied molecular orbital (HOMO) and lowest unoccupied molecular orbital (LUMO) energy. The effective reactivities of both the compounds that showed an energy band gap (ΔE), i.e., the difference between ELUMO and EHOMO, are 7.147 and 8.037 kcal mol−1.
Introduction
Solvents are a broad category of chemicals that can be diluted, dissolved, or dispersed by other substances.1 To produce homogeneous conditions, they facilitate the diffusion of reactants and catalysts. They also store and transmit the thermal energy required for chemical transformations, stabilize transition states, and stop undesired side reactions through dilution. However, common solvents used historically have undetected limitations. Green solvents have been introduced to help reduce the harmful effects of toxic solvents on the environment.2 High vapor pressure, flammability, toxicity, and air pollution are disadvantages of traditional solvents.3,4 In several applications, green solvents developed by scientists have outperformed traditional ones. Supercritical fluids, supercritical water, and ionic liquids are alternatives that fall under the “green solvents’’ category.5
Ionic liquids (ILs), often called ionic melt, liquid electrolytes, ionic fluids, or fused salts, are a class of substances entirely made of ions and capable of liquidity at temperatures below 100 °C.6,7 Unlike partially ionizable liquids like water/salt solutions and molecular liquids like dichloromethane or hexane, which contain molecules, ILs only contain cations and anions. The generally recognized definition states that these salts have a low vapor pressure and stay liquid below 100 °C.8,9 ILs are non-flammable and non-volatile substances, making them deemed environmentally beneficial substances. They provide improved reaction speeds, enabling a decrease in solvent amounts during different technological processes, resulting in cost savings and reducing waste risk. ILs can dissolve various organic, inorganic, and polymeric materials over a broad and wide temperature range. They are very viscous, non-flammable, moderately non-ionizing, and non-explosive compounds. ILs are also excellent solvents and have biocatalytic potential. They are also becoming more popular as substitute lubricants. Ionic liquids have attracted the attention of scientists due to their wide range of applications in various fields.10,11 Xu et al. have examined the efficiency of 1-octyl-3-methylimidazolium bromide as shale inhibitor. The hydration inhibition ability was investigated through soaking test and hot-rolling dispersion experiment. The inhibition ability of the ionic liquid was analyzed by FT-IR, XRD, TGA and surface tension measurements and the results exhibit the hydration inhibition ability was superior in comparison to other commonly used inhibitors. This was possible because 1-octyl-3-methylimidazolium bromide could easily be adsorbed onto sodium montmorillonite through electrostatic interaction and due to intercalation, water molecules present inside was ejected.12 This finding was supported by the observations of Luo et al. and Yang et al.13,14 The role of imidazolium based ILs in the electrochemical CO2 reduction reaction has also been explored. The ILs were diluted in acetonitrile to reduce the viscosity and it was observed that the anionic part of the imidazolium salts influenced the solubility of CO2 and the conductivity of the solution.15 Likewise, Li et al. have investigated on the carboxylate functionalized zeolitic imidazolate framework that enables the catalytic N-formylation utilizing ambient CO2. The presence of imidazole carboxylate species activates hydrosilane and CO2 and stimulates amine to take part in the formylation reaction. This indeed augments the catalyst capture and exchange capacity toward ambient CO2.16
Imidazolium-based ILs have received much attention and reporting even though different cation and counter-anion combinations can be used in ILs. The nitrogen-rich heteroatom compound-based ILs have a low melting point, good chemical stability, and excellent ionization performance.17 Because of the stable positive charge in the aromatic ring, which serves as an adaptable cation framework for the ILs, using imidazolium as a cation structure is a fascinating molecular strategy. The imidazolium ring typically includes two N atoms connected by the methylene group. As a result, the imidazole ring's two nitrogen atoms are classified as N1 (amino nitrogen) donors and N3 (imino nitrogen) acceptors, respectively.18 The imidazole ring is quaternized with organic substituents, particularly alkyl, aryl, and tertiary nitrogen (N3), to produce imidazolium-based ILs with a permanent positive charge. By doing so, the imidazole ring can take and donate protons during substitution processes. Additionally, secondary amine (N1) undergoes various reactions that support its amphoteric behaviors (accepts and donates protons) and adjustable imidazolium characteristics. As a result, imidazolium-based ILs have a wide range of practical uses, including as catalysts,19 sensors,20 adsorption,21,22 and biomass pre-treatment.23 Due to their almost nonexistent vapor pressure and ionic makeup, ILs can be helpful in various applications.24,25 They have significantly impacted the chemical industry due to their perceived status as “designer” or alternative “green” solvents. The ILs can be solvated to a different extent by the solvents due to the presence of various types of interactions such as ionic, hydrogen bonds, π–π, and van der Waals forces. Specifically in non-aqueous solutions, ionic interactions are more dominant due to the partial association of oppositely charged ions to form ion pairs. So, solvation is one of the critical factors in determining the rate of physicochemical processes in solution. Chu and coworkers have demonstrated 2-substituted imidazolium IL as a solvent for the Baylis–Hillman reaction (2-methyl imidazole). It has been shown that 2-methyl-substituted positions are rationally acidic and mainly synthesized by alkylation reactions.26 Shekaari et al. have reported on density, speed of sound and electrical conductance of 1-hexyl-3-methyl-imidazolium bromide in aqueous medium at different temperatures. The derived properties like apparent molar volume and isentropic compressibility have been calculated for different temperatures using the density and speed of sound. The calculated thermophysical properties reflect that interaction between IL and water decreases with increase in temperature.27 On the other hand, Dash et al. have examined the thermophysical measurements of aqueous solution of 1-hexyl-3-methyl-imidazolium bromide at 298.15 K and 0.1 MPa. The negative excess molar volume at this temperature indicates the compactness of the solution resulting from attractive interaction between the unlike components due to hydrogen bonding.28 The density, viscosity, and conductivity of 1-butyl-3-methylimidazolium bromide in water, methanol, ethanol, and acetonitrile have been measured at room temperature. The density decreases with rise in temperature and conductivity showed increasing trend.29 Recently, the solubility of ILs in polar solvents like dimethyl sulphoxide (DMSO), dimethylacetamide (DMA), dimethylformamide (DMF) have gained adequate attention due to their increasing dissolving rates and low costs at different temperatures compared to pure ILs.30,31 It has been reported that the solution of 1-butyl-3-methylimidazolium acetate in DMSO, DMF and DMA can efficiently dissolve cellulose at room temperature. DMSO has been considered as the most appropriate co-solvent for ILs for dissolving cellulose.32 Therefore binary mixtures of ionic liquids with DMSO are increasingly used for varied sectors.33–35 Amphiphilic molecule like DMSO contains a hydrophilic sulfoxide group with two hydrophobic methyl groups. It is endowed with two vital properties; it is soluble in water and possesses the potential to dissolve varied lipophilic compounds. The hydrophilic groups mainly form hydrogen bonds, and hydrophobic moieties tend to self-aggregate. To understand the usefulness of the solvent, the knowledge of the molecular interaction mechanisms, particularly the structure-making and breaking associations and interstitial accommodation, is essential to understand the ion–ion and ion–solvent interactions that exist in the binary mixtures.
In this perspective, in the first section, we initially present the thermophysical properties of the two synthesized 2-methylimidazolium-based ionic liquids and the mixture of ILs with DMSO.36 At atmospheric pressure, density and specific conductance were recorded for both systems in the range of 303.15 to 318.15 K. Using the density measurements, the molar volumes of the binary mixtures at different temperatures were calculated. The excess values were calculated and fitted to the Redlich–Kister polynomial. To add novelty to this work and gain more insight into the orientation of the molecules after the interaction, quantum chemical calculations applied in modeling simulation studies have been performed.
Experimental
Materials
The chemicals used in the study are listed in Table 1. All the chemicals were used as such without further purification.
Table 1 Lists of chemicals used in the study
Chemicals |
Purity |
Molecular formula |
Molecular mass (g mol−1) |
Density (g cm−3) |
CAS no. |
Source |
2-Methylimidazole |
99% |
C4H6N2 |
82.10 |
1.09 |
693-98-1 |
Sigma-Aldrich |
1-Bromohexane |
99% |
CH3(CH2)5Br |
165.08 |
1.18 |
111-25-1 |
Loba Chemie |
1-Bromooctane |
98% |
CH3(CH2)7Br |
193.12 |
1.112 |
111-83-1 |
Loba Chemie |
Chloroform |
≥99% |
CHCl3 |
119.38 |
1.474 |
67-66-3 |
Merck |
Acetone |
≥99% |
CH3COCH3 |
58.08 |
0.784 |
67-64-1 |
Merck |
DMSO |
≥99% |
(CH3)2SO |
78.13 |
1.101 |
67-68-5 |
Merck |
Methods
Synthesis. In a round bottom flask fitted, 0.01 mol of 2-methylimidazole was taken, and to it 0.02 mol of bromo alkanes (bromo hexane and bromo octane) were added with chloroform. The round bottom flask was fitted with a condenser and stirred for 6 h at 70 °C. The temperature was maintained to get the best result. The solution was left undisturbed overnight, and the obtained liquid was brownish-yellow viscous37 (Fig. 1). The IR studies were taken for the obtained IL.
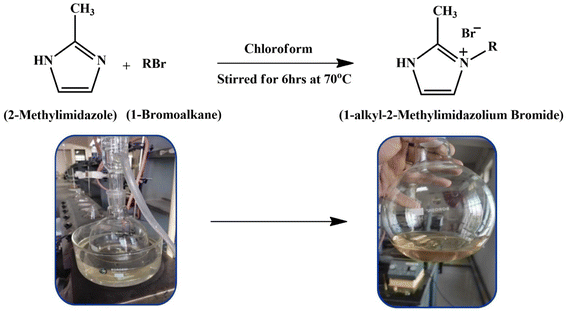 |
| Fig. 1 Synthesis of 1-alkyl-2-methyl imidazolium bromide (where R = hexyl and octyl). | |
Characterisation and thermophysical measurements
The FTIR spectra were recorded using Shimadzu IR-Spectrometer (FTIR 8201) within the 4000–400 cm−1. For thermophysical measurements, the required quantity of the synthesized ILs; 1-hexyl-2-methyl imidazolium bromide [HMIM][Br] and 1-octyl-2-methyl imidazolium bromide [OMIM][Br] were mixed with DMSO based on volume with a calculated precision of ±1 × 10−4 in mole fraction. The binary mixtures' mole fraction was calculated using the density and volume values. The density was recorded at T = 303.15, 308.15, 313.15 and 318.15 K with the help of a pycnometer and Citizen digital balance (CY 320C). It was calibrated using double distilled water. A Japanese conductivity meter made by Horiba Laqua called the F74 was used to evaluate the conductance of the binary mixtures and pure components. The uncertainties were estimated to be 0.1% for concentration and 1% for conductivity, respectively.
Data analysis
The molar volumes (V12) of the binary mixture of 1-hexyl-2methylimidazolium bromide [HMIM][Br]/1-octyl-2methylimidazolium bromide [OMIM][Br] with DMSO have been calculated using the density (ρ12) data recorded at T = (303.15, 308.15, 313.15 and 318.15) K, given in eqn (1) |
V12 = (X1M1 + X2M2)/ρ12
| (1) |
where X1, X2, M1, and M2 represent the mole fraction and molecular mass of DMSO and [HMIM][Br]/[OMIM][Br] respectively and ρ12 is the density of the binary mixture of ILs and DMSO.
The excess molar volume VE12 is calculated using eqn (2)
|
VE12 = V12 − (V1X1 + V2X2)
| (2) |
where
V1 and
V2 are the molar volumes of the DMSO and [HMIM][Br]/[OMIM][Br] respectively.
The values of the excess properties are correlated by using the Redlich–Kister polynomial using eqn (3)
|
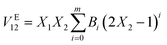 | (3) |
where,
Bi is the fitting coefficient and
m represents the order of the polynomial equation.
The standard deviations, σ(VE12) have been reported using the following expression (4)
|
σ(VE12) = [∑(VE12,exp − VE12,cal)2/(n − m)]1/2
| (4) |
where,
n symbolizes the experimental data points and
m is the number of coefficients respectively.
In silico analysis
Retrieval of compounds. The structures of (a) 1-hexyl-2-methyl imidazolium bromide (b) 1-octyl-2-methyl imidazolium bromide, and (c) dimethyl sulfoxide was drawn using ChemDraw20.0 software. The structures were converted to.pdb format, using Chen3D 20.0 software, the preferred format for various in silico analyses.
In silico combinatorial chemistry
Combinatorial chemistry is the sum of repetitive and covalent coupling of different “building blocks” to represent a spectrum of structurally different molecules called as chemical library. In the current arena, computational tools play a pivotal role in Combinatorial chemistry through combinatorial drugs, which is the preferred aegis of the pharmaceutical sector. It is well known that solvent and thermal effect play a crucial role in understanding the properties of a drug metabolism. Taking together the above concerns, the compounds [HMIM][Br] and [OMIM][Br] interacted with the solvent dimethyl sulfoxide through an In silico approach (Schrödinger Release 2022-4: Maestro, Schrödinger, LLC, New York, NY, 2022).
Density functional theory (DFT) for quantum chemical calculation
Quantum computation studies were performed with the help of density functional theory (DFT) to investigate the reactivity and efficiency of the compounds. The reactivity of [HMIM][Br] and [OMIM][Br] were analyzed using the Becke, 3-parameter, Lee–Yang–Parr (B3LYP) correlation function of DFT. The DFT analysis was performed using the highest occupied molecular orbital (HOMO) and lowest unoccupied molecular orbital (LUMO) energy. The ORCA Program version 4.0 was used for calculation of energy of orbitals. The electronic energy, frontier HOMOs, LUMOs, gap energy, and dipole moment were measured for the potential drugs. The following eqn (5) was used for calculation of DFT. |
 | (5) |
where, {Vnuclei + nr}n ≡ trial density and F ≡ universal functional.
Molecular dynamics (MD) simulations
Molecular dynamics (MD) is a sophisticated computational tool for envisaging and analyzing the physical movements of atoms and molecules in macromolecular structure-to-functions.36 The mode of binding, the specificity of the substrate, and the dynamic behaviour can be clearly explained. The atoms and molecules are allowed to interact in a set time. This clearly illustrates the system's dynamic “evolution”.38 The MD protocol includes minimization, heating, equilibration, and production.39 The OPLS4 force field was used for minimization, and topology and atomic coordinates were obtained automatically, followed by the addition of ILs to the DMSO solvent model orthorhombic box (15 × 15 × 10 Å).40 The Particle Mesh Ewald (PME) boundary condition has been used to ensure that no solute atoms occurred within 10 Å distance of the border. The entire system was simulated at 303.15 and 318.15 K for 100 ns using the NPT ensemble, and the structural alterations and dynamic behaviour were investigated using root mean square deviation (RMSD) and root mean square fluctuation (RMSF) graphs. The RMSF method is used to find the flexible region.41
Results and discussion
FTIR analysis
To validate the synthesis of [HMIM][Br] and [OMIM][Br], an active group investigation of ILs using FT-IR data was used (Fig. 2). Several discernible peaks provided evidence for an imidazolium ring, including N–H stretching at 3397.96 cm−1, C
C at 1601.51 cm−1, and C
N at 1617.98 cm−1. Peaks obtained at 1378.85 cm−1, 3063.37 cm−1, and 1117.55 cm−1 supported the existence of an imidazolium ring. Aliphatic C–H stretch signals were also discovered at 2928.35 cm−1, pointing to the presence of alkyl chain groups.42–44 Numerous earlier studies revealed that the absence of the C–Br stretching absorbance band in the IR spectra for the absorption of single-element anions Br was caused by the synthesis of the imidazolium salt and the lack of bond vibration.45–47
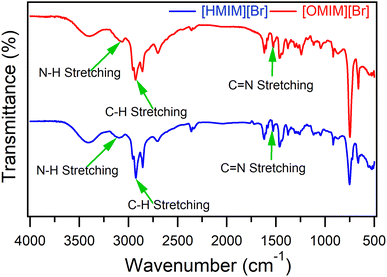 |
| Fig. 2 FTIR spectrum of pure [HMIM][Br] (in blue) and [OMIM][Br] (in red). | |
Density measurements
The density of pure [HMIM][Br] and [OMIM][Br] has been measured at T = 303.15, 308.15, 313.15, and 318.15 K and presented in Table 2.
Table 2 Density of pure [HMIM][Br] and [OMIM][Br]a
Temperature (K) |
Density (g cm−3) |
[HMIM][Br] |
[OMIM][Br] |
Standard uncertainties (0.68 level of confidence) for T = 0.001 K, U(c)ρ = 0.0001 g cm−3. |
303.15 |
1.2621 |
1.2074 |
308.15 |
1.2602 |
1.2072 |
313.15 |
1.2591 |
1.2068 |
318.15 |
1.2583 |
1.2064 |
The densities of the binary mixture of the ILs with DMSO recorded in the temperature range of 303.15–318.15 K at 5 K intervals have been represented in (Fig. 3). From the plots, it is evident that the density of the binary mixture of ILs with DMSO increases with the increase in mole fractions of [HMIM][Br] and [OMIM][Br]. This is because the densities of ILs are more than the solvent DMSO. But with the temperature rise, it is observed that the density of the binary mixture decreases with an increase in the mole fraction of the ILs.
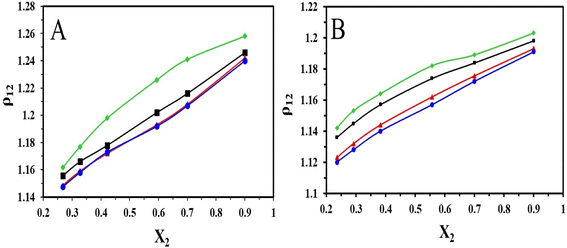 |
| Fig. 3 Plot of variation of density with different mole fractions of (A) [HMIM][Br] and (B) [OMIM][Br] at T = (♦303.15, ■308.15, ▲313.15 and ●318.15) K. | |
Molar volume
The derived properties, such as molar volumes, were calculated using density values (ρ12) of the binary mixtures of [HMIM][Br]/[OMIM][Br] in DMSO at different temperatures. As the density decreases with an increase in the mole fraction of [HMIM][Br] and [OMIM][Br], the molar volume of the binary mixture increases. This behavior is justified because density and volume are inversely related.48 With the temperature rise, the molar volume displays an increasing trend due to the mutual loss of dipolar association and the constituent molecules' differences in shape and size (Table 3).
Table 3 Representation of molar volumes (V12) of binary mixture of [HMIM][Br]/[OMIM][Br] in DMSO at different temperaturesa
X2 |
V12 (cm3 mol−1) |
303.15 K |
308.15 K |
313.15 K |
318.15 K |
Standard uncertainties (0.68 level of confidence) for T = 0.001 K, U(c)ρ = 0.0001 g cm−3. |
[HMIM][Br] |
0.267 |
106.041 |
106.601 |
107.269 |
107.587 |
0.327 |
113.280 |
114.215 |
115.049 |
115.438 |
0.422 |
124.765 |
126.883 |
127.533 |
127.860 |
0.594 |
145.568 |
148.475 |
149.595 |
149.972 |
0.700 |
158.248 |
161.502 |
162.571 |
162.841 |
0.900 |
182.986 |
184.748 |
185.343 |
185.642 |
![[thin space (1/6-em)]](https://www.rsc.org/images/entities/char_2009.gif) |
[OMIM][Br] |
0.236 |
109.078 |
109.655 |
110.924 |
111.221 |
0.292 |
117.611 |
118.442 |
119.793 |
120.217 |
0.383 |
131.908 |
132.706 |
134.214 |
134.685 |
0.557 |
158.914 |
160.024 |
161.649 |
162.348 |
0.700 |
181.684 |
182.482 |
183.770 |
184.319 |
0.900 |
212.337 |
213.223 |
214.117 |
214.477 |
Excess properties
The mixture's molecular environment is altered by adding more of a component, dramatically changing the forces between different molecules, and affecting the thermodynamic characteristics of the binary liquid system.49 The easiest way to describe the departure from ideality in a liquid mixture is through the excess thermodynamic properties. The experimental density data of the binary mixtures were used to compute the excess molar volume values (VE12). The standard deviation values and coefficients that were obtained after the experimental data were fitted into the Redlich–Kister polynomial are displayed in Table 4. The excess characteristics are estimated using the Redlich–Kister fitting coefficients. Fig. 4 represents the excess molar volume for the binary mixture of [HMIM][Br] and DMSO at T = 303.15, 308.15 K, 313.15 K, and 318.15 K. At 303.15 K, the excess tends to be positive, and after that, a negative trend follows. But at higher temperatures, there is a positive trend at all mole fractions of [HMIM][Br]. Two competing causes have led to this. Volume expansion is carried on by mutual loss of dipolar association and differences in the sizes and shapes of the constituent molecules. Dipole-induced interactions between polar and dissimilar molecules bring on volume contraction. The experiment results indicate that the latter effect predominated in the binary mixture, supporting the idea that structural factors cause mixtures to expand relative to pure components. The variation in VE12 for binary combinations is shown in (Fig. 5) based on the mole fraction of [OMIM][Br]. In comparison to [HMIM][Br], the VE12 values are negative at all mole fractions of [OMIM][Br], indicating the indirect influence of the H-bond formed by the neighboring functional group S
O with hydrogen present in the alkyl chain. This observation was in coherence with the findings of Dash et al. where negative excess volume was noted at 298.15 K for solution of 1-hexyl-3-methylimidazolium bromide in water due to hydrogen bonding that resulted in compactness of the system.28
Table 4 List of coefficients and standard deviation values of [HMIM][Br] and [OMIM][Br] at T = 303.15, 308.15, 313.15 and 318.15 K
Temp. (K) |
B0 |
B1 |
B2 |
B3 |
σ |
[HMIM][Br] |
303.15 |
0.889 |
−7.150 |
0.541 |
0.018 |
0.017 |
308.15 |
10.007 |
6.444 |
−5.45 |
0.011 |
0.002 |
313.15 |
12.728 |
−9.492 |
−4.320 |
−0.051 |
0.094 |
318.15 |
12.186 |
9.997 |
−5.183 |
0.095 |
0.116 |
![[thin space (1/6-em)]](https://www.rsc.org/images/entities/char_2009.gif) |
[OMIM][Br] |
303.15 |
−11.543 |
6.886 |
−2.925 |
0.001 |
0.181 |
308.15 |
3.632 |
3.483 |
4.752 |
9.807 |
0.466 |
313.15 |
8.893 |
4.207 |
7.121 |
−0.047 |
0.490 |
318.15 |
10.624 |
5.902 |
5.968 |
−0.064 |
0.401 |
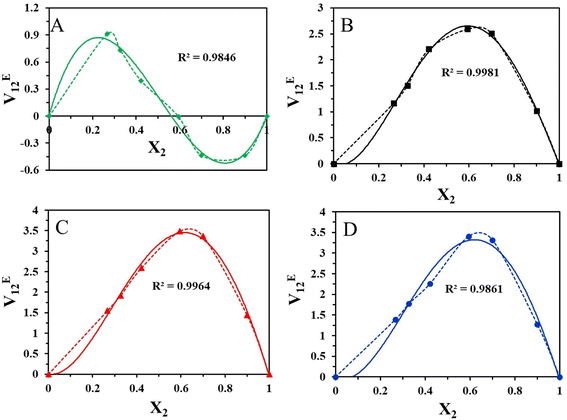 |
| Fig. 4 Representation of VE12 of [HMIM][Br] in DMSO at T = (♦303.15 (A), ■308.15 (B), ▲313.15 (C) and ●318.15 (D)) K. The solid lines represent the Redlich–Kister correlations and dotted lines denote the experimental values. | |
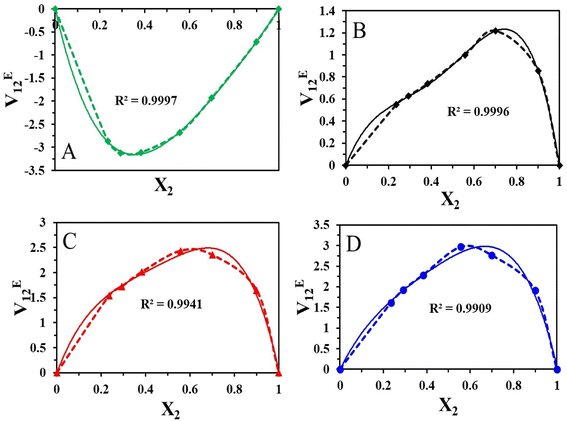 |
| Fig. 5 Representation of VE12 of [OMIM][Br] in DMSO at T = (♦303.15 (A), ■308.15 (B), ▲313.15 (C) and ●318.15 (D)) K. The solid lines represent the Redlich–Kister correlations and dotted lines denote the experimental values. | |
In this study, the VE12 values are affected by several variables, including geometric, chemical, and physical aspects. It is clear from the detailed examination that the molecular interaction between the [HMIM][Br]/[OMIM][Br] + DMSO is more dominant in case [OMIM][Br] at 303.15 K due to greater chain length of the alkyl group. Due to the absence of a dipolar connection at high temperatures, VE12 values suggest that the interactions between the binary mixture's constituents are not selective.
Conductivity measurements
The measurement of the conductivity of ILs gives information about the ionic interactions in the liquid mixtures. Due to the viscous nature of ionic liquids, the conductivity tends to remain low. The conductivity of pure IL is influenced by the presence of cosolvent. The increase or decrease of conductivity relies on the nature of the solvent and the extent of dissociation of IL into ions.50 The conductivity of neat [HMIM][Br] and [OMIM][Br] at T = 303.15, 308.15, 313.15, and 318.15 K have been recorded and presented in Table 5.
Table 5 Representation of specific conductivity of pure [HMIM][Br] and [OMIM][Br] at different temperatures
Temperatures in K |
Specific conductivity (κ), S cm−1 |
[HMIM][Br] |
[OMIM][Br] |
303.15 K |
1.013 |
1.141 |
308.15 K |
1.159 |
1.205 |
313.15 K |
1.216 |
1.425 |
318.15 K |
1.330 |
1.625 |
Fig. 6 represent the variation of specific conductivity of the binary mixtures of [HMIM][Br]/[OMIM][Br] and DMSO. It is observed that at low mole fraction of ILs, the specific conductivity value is high but gradually decreases as the concentration increases. This clearly indicates that smaller ions can move more easily than the larger ions. On the other hand, it is detected that as the temperature increases the conductivity of [HMIM]/[OMIM] in DMSO increases. This observation is well supported by the expansion in volume and positive excess molar volumes. This finding showed similar trend as reported for 1-butyl-3-methylimidazolium bromide in water where the conductivity increased with rise in temperature.29
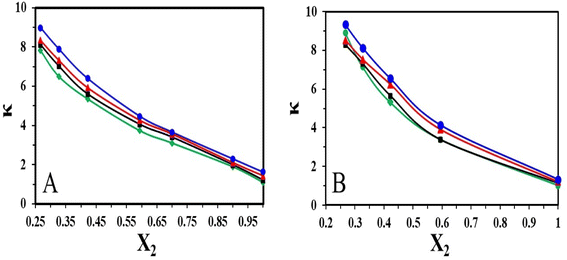 |
| Fig. 6 Plot of specific conductivity of (A) [HMIM][Br] and (B) [OMIM][Br] in DMSO at T = (♦303.15, ■308.15, ▲313.15 and ●318.15) K. | |
Quantum chemical calculation
Quantum chemical calculations applied in modelling simulation studies can be used for investigating frontier molecular characteristics. Considering the importance of the structure and reactivity of compounds, we carried out DFT simulations for [HMIM][Br] and [OMIM][Br] using ORCA Programme (version 4.0) to understand its geometry and orientation (Table 6).
Table 6 Electronic energy, energy in atomic unit of highest occupied molecular orbital (HOMO), lowest unoccupied molecular orbital (LUMO), gap energy and dipole moment of the compounds DFT
Pubchem compound ID |
Compound |
Electronic energy (eV) |
ELUMO (kcal mol−1) |
EHOMO (kcal mol−1) |
GAP energy (ΔE) (kcal mol−1) |
Dipole moment (Debye) |
ChemDraw |
[HMIM][Br] |
−83547.243 |
1.349 |
−5.798 |
7.147 |
25.60293 |
ChemDraw |
[OMIM][Br] |
−85670.505 |
1.778 |
−6.259 |
8.037 |
21.97936 |
The effective reactivity for both the compounds which showed band energy gap (ΔE), i.e. the difference between ELUMO and EHOMO, was 7.147 and 8.037 kcal mol−1 (Fig. 7A–D).
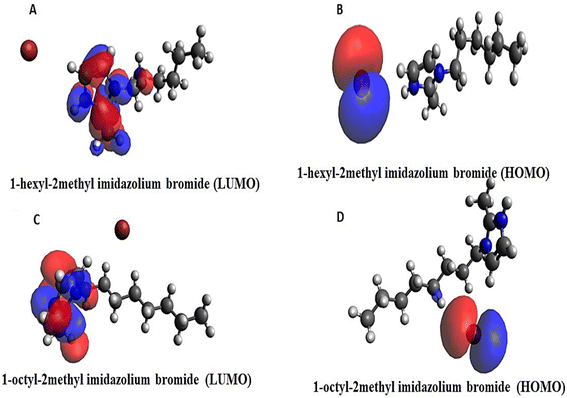 |
| Fig. 7 LUMO and HOMO plots of [HMIM][Br] (A and B) and [OMIM][Br] (C and D) which exhibited higher reactivity. Red colour indicates the positive electron density and the negative electron density in represented in blue colour. | |
The lower the gap energy, the higher the reactivity. [HMIM][Br] displayed the most remarkable reactivity compared to [OMIM][Br] based on its lowest band energy gap, which was calculated to be 7.147 kcal mol−1.
Trajectory analysis of MD simulations
In the current investigation, we employed the MD simulations for two compounds immersed in DMSO under different temperatures. The interaction studies were Holo1: [HMIM][Br] interacting with DMSO at 303.15 K, Holo2: [HMIM][Br] interacting at 318.15 K, Holo3: [OMIM][Br] interacting with DMSO at 303.15 K and Holo4: [OMIM][Br] interacting with DMSO at 318.15 K using Desmond suit (Schrödinger Release 2022-4: Maestro, Schrödinger, LLC, New York, NY, 2022) in order to understand the dynamic behaviour, mode of binding and inhibitor specificity in all the four systems. A 100 ns MD simulation was used to assess structural rearrangements as well as the stability of such combinations. The RMSD profile of the backbone atoms, at 100 ns, was used to determine the dynamic stability of all the systems (Holo1–Holo4) (Fig. 8A–D).
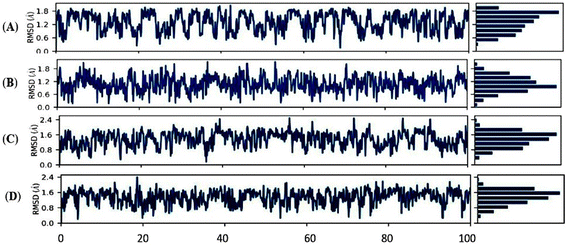 |
| Fig. 8 Conformational RMSD stability of Holo states throughout 100 nanoseconds (ns) time period of MDS. (A) Backbone-RMSD of [HMIM][Br]–DMSO at 303.15 K. (B) Backbone-RMSD of [HMIM][Br]–DMSO at 318.15 K. (C) Backbone-RMSD of [OMIM][Br]–DMSO at 303.15 K. (D) Backbone-RMSD of [OMIM][Br]–DMSO at 318.15 K. | |
The backbone RMSD graph of Holo1: [HMIM][Br] interacting with DMSO at 303.15 K, depicted a consistent instability throughout the MD simulation time compared to Holo2: [HMIM][Br] interacting with DMSO at 318.15 K, which revealed a comparative stable trajectory after 70 ns of MD simulation, during 100 ns of simulation time frame. In Holo1 state the RMSD reflected value between ∼0.1 to ∼1.8 Å, from 0 to 100 ns of MD simulations whereas Holo2 state depicted the RMSD value between ∼0.3 to ∼1.8 Å. The RMSD graph of Holo1 revealed slightly higher deviations compared to Holo2. This depicts that the temperature may play a vital role in the interaction of compound [HMIM][Br] with DMSO solvent and can assist in stabilizing and destabilizing through changing its conformation compared to Holo1. Similarly, RMSD of Holo3: [OMIM][Br] interacting with DMSO at 303.15 K revealed a stable trajectory after 90 ns of MD simulation, during 100 ns of simulation time frame compared to Holo4. In the Holo3 state, the RMSD reflected a value between ∼0.4 to ∼2.0 Å, from 90 to 100 ns of MD simulations, whereas the Holo4 state depicted the RMSD value between ∼0.8 to ∼1.6 Å from 30 to 50 ns but after 50 ns of MDS there were deviations. The RMSD graph of Holo3 revealed slightly higher deviations compared to Holo4. This depicts that the temperature may play a key role in interaction of compound [OMIM][Br] interacting with DMSO solvent at 318.15 K can assist in stabilizing and destabilizing through changing its conformation when compared to Holo3.
The RMSD finding was further corroborated by RMSF to monitor the fluctuation of atoms. The mobility of different atoms was observed in both the states through RMSF plots (Fig. 9A–D).
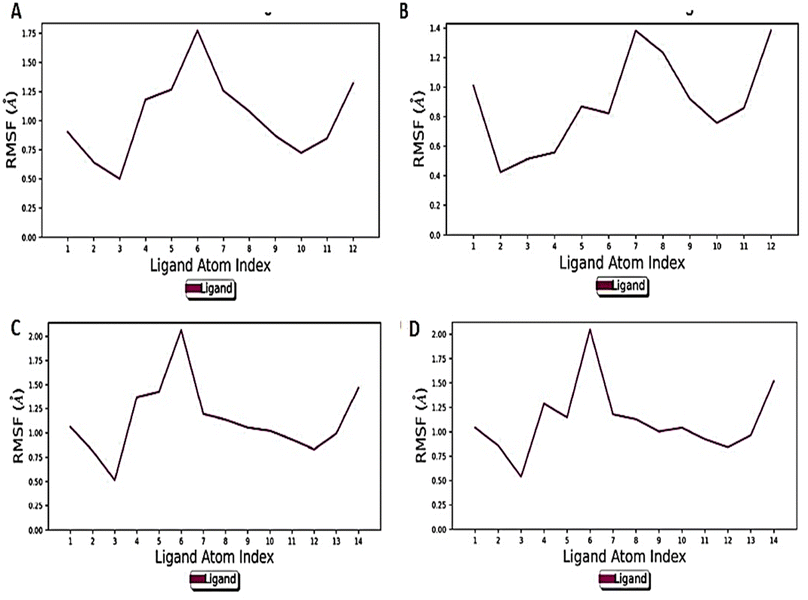 |
| Fig. 9 Conformational stability of Apo and Holo states recorded throughout 100 nanoseconds of MDS. Cα-RMSF profile of (A) [HMIM][Br]–DMSO at 303.15 K, (B) [HMIM]–DMSO at 318.15 K, (C) [OMIM][Br]–DMSO at 303.15 K, (D) [OMIM][Br]–DMSO at 318.15 K. | |
Overall, the Holo3 and Holo4 state showed more fluctuations than the Holo1 and Holo2 state which are depicted in peaks of the RMSF graph, demonstrating the simulation's constrained motions. The overall compactness for all the states during the simulation of 100 ns was explained using properties such as radius of gyration (rGyr), as shown in (Fig. 10A–D).
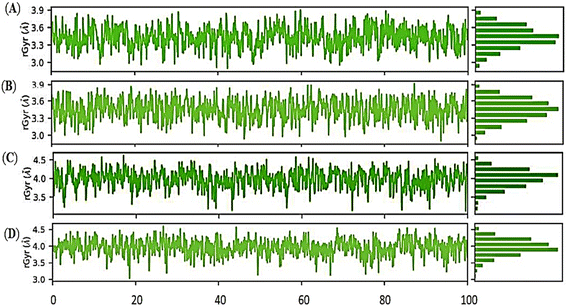 |
| Fig. 10 MD simulations of 100 nanoseconds for solvent accessible surface analysis (SASA); (A) [HMIM][Br]–DMSO at 303.15 K, (B) [HMIM][Br]–DMSO at 318.15 K, (C) [OMIM][Br]–DMSO at 303.15 K, (D) [OMIM][Br]–DMSO at 318.15 K. | |
The radius of gyration (rGyr) equals its principal moment of inertia. The fluctuation graphs of rGyr vs. simulation duration show that Holo1 showed decrease in gyration during 90 to 100 ns of MD simulations. In case of Holo2 the rGyr remains constantly fluctuated over the simulation procedure. The 1-hexyl-2-methyl imidazolium bromide interacting with DMSO at 303.15 K rGyr variation ranging from ∼3.0 to ∼3.7 Å during 90 to 100 ns time frame, and ∼2.9 to ∼3.7 during 90 to 100 ns time frame in Holo2. Holo3 depicted rGyr values ∼3.1 to ∼4.4 Å throughout the MD simulation time frame. Holo4 depicted rGyr values ∼3.2 to ∼4.4 Å during 90 to 100 ns time frame. Taking to gather all the rGyr values Holo1 indicated more compact than Holo2 state and other states, representing that the value of rGyr is inversely proportional to compactness and vice versa.51 These consequences of rGyr are well supported by RMSF analysis. The frequency of the interactions between ILs and DMSO is directly proportional to the visible surface area. SASA's illustration (Fig. 11A–D).
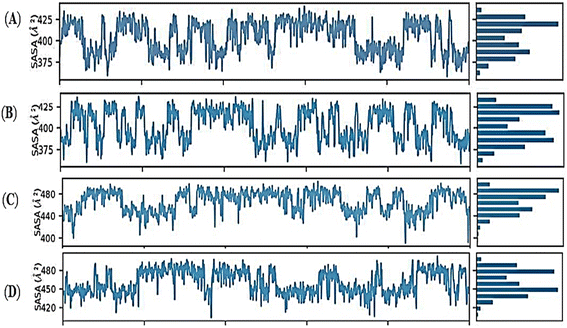 |
| Fig. 11 MD simulations of 100 nanoseconds for solvent accessible surface analysis (SASA); (A) [HMIM][Br]–DMSO at 303.15 K, (B) [HMIM][Br]–DMSO at 318.15 K, (C) [OMIM][Br]–DMSO at 303.15 K, (D) [OMIM][Br]–DMSO at 318.15 K. | |
Mainly in the Holo states, the available solvent surface was reduced. The findings of SASA revealed that the Holo1: [HMIM][Br] interacting with DMSO at 303.15 K, can alter the hydrophilic and hydrophobic interaction areas, which might potentially affect the orientations. During 55 ns to 75 ns MD simulation, the SASA graph of Holo1 reflected a buried stat represented SASA with ∼380 to ∼430 Å2. The Holo2 state represented SASA with ∼400 to ∼430 Å2, during 30 to 50 ns of MD simulation time frame. In case of Holo3 and Holo4 the SASA value was ∼440 Å2 to ∼480 Å2 during 50 to 70 ns and ∼425 Å2 to ∼480 Å2 during 60 to 80 ns of time frame. The results of SASA depict that temperature plays a key role in orientation change during the interaction of compound and solvent. This may be the reason for getting different time frames for buried regions in other Holo states. This suggests that the atoms of Holo states may shift from the accessible to the buried region may produce an orientational change.
Post MDS analysis
During MD simulations, the intra and inter molecular atomic bonds of the Holo states were plotted using Schrödinger Release 2022-4 (Fig. 12A–D). Simulation of all the Holo states revealed variable bonding during the simulation. The post MD simulation analysis of revealed change in the atomic arrangement in Holo states. Compared to Holo1, and Holo3, Holo2 and Holo4 were found to have comparatively more compactness.
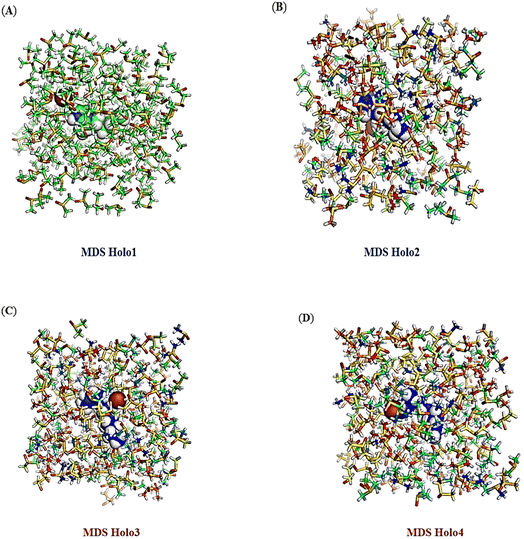 |
| Fig. 12 MDS interaction study. (A) [HMIM][Br]–DMSO at 303.15 K, (B) [HMIM][Br]–DMSO at 318.15 K, (C) [OMIM][Br]–DMSO at 303.15 K, (D) [OMIM][Br]–DMSO at 318.15 K. The images were generated by PyMol tool. | |
Conclusions
Density and conductivity measurements of [HMIM][Br] and [OMIM][Br] in DMSO have been reported at temperatures T = (303.15, 308.15, 313.15 and 318.15) K. The molar volume and excess molar volume evaluated using density data correlated well with the Redlich–Kister polynomial. The parameters indicate that the interaction between [HMIM][Br]/[OMIM][Br] and DMSO decrease with increase in temperature from 303.15 to 318.15 K. This observation was in coherence with similar systems reported in literature. The negative excess molar volume for the binary mixtures at 303.15 K for all mole fractions of [OMIM][Br] indicates the formation of hydrogen bonds between the S
O and alkyl chain. However, there is a loss of dipolar connection between the components as the temperature rises. The conductivity of the ILs increases with temperature indicating non-specific interaction between the ILs and DMSO. The energy difference between the LUMO and HOMO of the systems has been calculated using DFT. The MD simulation studies reveal that temperature play a vital role in orientation change during the interaction of ionic liquids with the solvent. This suggests that the atoms of Holo states may shift from accessible to buried region and may result in orientational change. Overall, both experimental and computational results will undeniably provide appreciated and exceptional knowledge on molecular interactions between ILs and DMSO which have been the spotlight of chemical research in the last decade.
Author contributions
Conceptualization: Sanghamitra Pradhan. Data Curation: Itishree Panda, Bikash Ranjan Behera, Debasmita Jena, Santosh Kumar Behera, Sangram Keshari Samal, Sanghamitra Pradhan. Funding acquisition: Sanghamitra Pradhan, Sangram Keshari Samal, Santosh Kumar Behera. Investigation: Itishree Panda, Bikash Ranjan Behera, Debasmita Jena, Santosh Kumar Behera, Sangram Keshari Samal, Sanghamitra Pradhan. Software: Sanghamitra Pradhan, Sangram Keshari Samal, Santosh Kumar Behera. Supervision: Sanghamitra Pradhan. Visualization, writing – original draft, writing – review & editing: Itishree Panda, Bikash Ranjan Behera, Debasmita Jena, Santosh Kumar Behera, Sangram Keshari Samal, Sanghamitra Pradhan.
Conflicts of interest
The authors have no conflicts of interest to declare.
Acknowledgements
Ms. Itishree Panda acknowledges the Siksha ‘O’ Anusandhan Deemed to be University for providing Ph.D. fellowship. Dr Sangram Keshari Samal highly acknowledges the Ramanujan fellowship (SB/S2/RJN-038/2016) of the Department of Science and Technology, and Ramalingaswami Re-entry fellowship (Ref: D.O. No. BT/HRD/35/02/2006) of Department of Biotechnology, Government of India. The authors also thank the Indian Council of Medical Research-Regional Medical Research Centre, Bhubaneswar for providing a scientific platform. The authors also express their deep sense of gratitude to Department of Biotechnology, National Institute of Pharmaceutical Education and Research, Ahmedabad, Gujarat for giving us the chance to use Schrödinger, LLC, New York, NY, 2022 (Schrödinger Release 2022-4: Maestro, Schrödinger, LLC, New York, NY, 2022).
References
- C. J. Clarke, W.-C. Tu, O. Levers, A. Brohl and J. P. Hallett, Green and sustainabke solvents in chemical processes, Chem. Rev., 2018, 118, 747–800 CrossRef CAS PubMed
. - M. Petkovic, K. R. Seddon, L. P. N. Rebelo and C. Silva Pereira, Ionic liquids: a pathway to environmental acceptability, Chem. Soc. Rev., 2011, 40, 1383–1403 RSC
. - M. Espino, M. de los Ángeles Fernández, F. J. V. Gomez and M. F. Silva, Natural designer solvents for greening analytical chemistry, TrAC, Trends Anal. Chem., 2016, 76, 126–136 CrossRef CAS
. - Z. Xue, L. Qin, J. Jiang, T. Mu and G. Gao, Thermal. electrochemical and radiolytic stabilities of ionic liquids, Phys. Chem. Chem. Phys., 2018, 20, 8382–8402 RSC
. - H. Machida, M. Takesue and R. L. Smith, Simultaneous and selective recovery of cellulse and hemicellulose fractions from wheat bran supercritical water hydrolysis, J. Supercrit. Fluids, 2011, 60, 2–15 CrossRef CAS
. - M. Galiński, A. Lewandowski and I. Stępniak, Ionic liquid as electrolytes, Electrochim. Acta, 2006, 51, 5567–5580 CrossRef
. - Ionic Liquids as Green Solvents, ed. R. D. Rogers and K. R. Seddon, American Chemical Society, Washington, DC, 2003, vol. 856 Search PubMed
. - Z. Lei, B. Chen, Y.-M. Koo and D. R. MacFarlane, Introduction: Ionic liquids, Chem. Rev., 2017, 117, 6633–6635 CrossRef PubMed
. - J. P. Hallett and T. Welton, Room temperature ionic liquids: solvents for synthesis and catalysis, Chem. Rev., 2011, 111, 3508–3576 CrossRef CAS PubMed
. - S. K. Singh, S. Banerjee, K. Vanka and P. L. Dhepe, Understanding interactions between lignin and ionic liquids with experimental and theoretical studies during catalytic depolymerization, Catal. Today, 2018, 309, 98–108 CrossRef CAS
. - P. G. Jessop, Fundamental properties and practical applications of ionic liquids: concluding remarks, Faraday Discuss., 2018, 206, 587–601 RSC
. - J. Xu, Z. Qiu, X. Zhao, H. Zhong and W. Huang, Study of 1-octyl-3-methylimidazolium bromide for inhibiting shale hydration and dispersion, J. Pet. Sci. Eng., 2019, 177, 208–214 CrossRef CAS
. - Z. Luo, L. Wang, P. Yu and Z. Chen, Experimental study on the application of an ionic liquid as shale inhibitor and inhibitive mechanism, Appl. Clay Sci., 2017, 150, 267–274 CrossRef CAS
. - L. Yang, G. Jiang, Y. Shi and X. Yang, Application of ionic liquid and polymeric ionic liquid as shale hydration inhibitors, Energy Fuels, 2017, 31, 4 CrossRef
. - A. Fortunati, F. Risplendi, M. R. Fiorentin, G. Cicero, E. Parisi, M. Castellino, E. Simone, B. Iliev, T. J. S. Schubert, N. Russo and S. Hernandez, Understanding the role of imidazolium-based ionic liquids in the electrochemical CO2 reduction reaction, Commun. Chem., 2023, 6, 84 CrossRef CAS PubMed
. - Z. Li, H. Li and S. Yang, Carboxylate-functionalized zeolitic imidazolate framework enables catalytic N-formylation using ambient CO2, Adv. Sustainable Syst., 2022, 6, 2100380 CrossRef CAS
. - S. Pal, A. Mukherjee and P. Ghosh, Imidazolium based ionic liquid-assisted processing of natural biopolymers containing amine/amide functionalities for sustainable fiber production, Mater. Today Sustain., 2021, 14, 100082 CrossRef
. - A. Verma, S. Joshi and D. Singh, Imidazole: Having versatile biological activities, J. Chem., 2013, 2013, 1–12 Search PubMed
. - H. Wan, Z. Wu, W. Chen, G. Guan, Y. Cai, C. Chen, Z. Li and X. Liu, Heterogenization of ionic liquid based on mesoporous material as magnetically recyclable catalyst for biodiesel production, J. Mol. Catal. A: Chem., 2015, 398, 127–132 CrossRef CAS
. - R. Gao, D. Wang, J. R. Heflin and T. E. Long, Imidazolium sulfonate containing pentablock copolymer-ionic liquid membranes for electroactive actuators, J. Mater. Chem., 2012, 22, 13473 RSC
. - S. Kassaye, K. K. Pant and S. Jain, Hydrolysis of cellulosic bamboo biomass into reducing sugars via a combined alkaline solution and ionic liquid pretreatment steps, Renewable Energy, 2017, 104, 177–184 CrossRef CAS
. - Z. Zhao, H. Dong and X. Zhang, The research progress of CO2 capture with ionic liquids, Chin. J. Chem. Eng., 2012, 20, 120–129 CrossRef CAS
. - S. K. Singh and P. L. Dhepe, Novel synthesis of immobilised Bronsted-acidic ionic liquid: application of lignin depolymerization, ChemistrySelect, 2018, 3, 5461–5470 CrossRef CAS
. - M. Filice, J. M. Guisan and J. M. Palomo, Effect of ionic liquids as additives in the catalytic properties of different immobilized preparations of Rhizomucor miehei lipase in the hydrolysis of peracetylated lactal, Green Chem., 2010, 12, 1365 RSC
. - K. R. Seddon, A. Stark and M.-J. Torres, Influence of chloride, water, and organic solvents on the physical properties of ionic liquids, Pure Appl. Chem., 2000, 72, 2275–2287 CrossRef CAS
. - J.-C. Hsu, Y.-H. Yen and Y.-H. Chu, Tetrahedron Lett., 2004, 45, 4673–4676 CrossRef CAS
. - H. Shekaari, Y. Mansoori and R. Sadeghi, Density, speed of sound, and electrical conductance of ionic liquid 1-hexyl-3-methyl-imidazolium bromide in water at different temperatures, J. Chem. Thermodyn., 2008, 40, 852–859 CrossRef CAS
. - D. Dash, S. Kumar, C. Mallika and U. K. Mudali, Thermophysical, Volumetric, and Excess Properties of Aqueous Solutions of 1-Hexyl-3-Methylimidazolium Bromide at 298.15 K and 0.1 MPa, Hindawi Publishing Corporation, 2013 Search PubMed
. - P. N. Tshibangu, S. N. Ndwandwe and E. D. Dikio, Density, viscosity and conductivity study of 1-butyl-3-methylimidazolium bromide, Int. J. Electrochem. Sci., 2011, 6, 2201–2213 CrossRef CAS
. - R. Rinaldi, Instantaneous dissolution of cellulose in organic electrolyte solutions, Chem. Commun., 2011, 47, 511–513 RSC
. - A. Xu and F. Wang, Carboxylate ionic liquid solvent systems from 2006 to 2020: thermal properties and application in cellulose processing, Green Chem., 2020, 22, 7622–76664 RSC
. - A. Xu, Y. Wang and R. Liu, Cellulose dissolution in diallylimidazolium methoxyacetate + N-methylpyrrolidinone mixture, Sci. Rep., 2019, 9, 11518 CrossRef PubMed
. - N.-N. Wang, Q.-G. Zhang, F.-G. Wu, Q.-Z. Li and Z.-W. Yu, Hydrogen bonding interactions between a representative pyridinium based ionic liquid [BuPy][BF4] and water/Dimethyl sufoxide, J. Phys. Chem. B, 2010, 114, 8689–8700 CrossRef CAS PubMed
. - S. Zhao, X. Tian, Y. Ren, J. Wang, J. Liu and Y. Ren, A theoretical investigation of the interactions between hydroxyl-functionalized ionic liquid and water/methanol/dimethyl sulfoxide, J. Mol. Model., 2016, 22, 195 CrossRef PubMed
. - G. Rai and A. Kumar, Probing thermal interactions of ionic liquids with dimethyl sulfoxide, ChemPhysChem, 2012, 13, 1927–1933 CrossRef CAS PubMed
. - K. Chen, B. Xu, L. Shen, D. Shen, M. Li and L.-H. Guo, Functions and performance of ionic liquids in enhancing electrocatalytic hydrogen evolution reactions: a comprehensive review, RSC Adv., 2022, 12, 19452–19469 RSC
. - W. Feng, Y. Lu, Y. Chen, Y. Lu and T. Yang, Thermal stability of imidazolium based ionic liquids investigated by TG and FTIR techniques, J. Therm. Anal. Calorim., 2016, 125, 143–154 CrossRef CAS
. - J. D. Durrant and J. A. McCammon, Molecular dynamics simulations and drug discovery, BMC Biol., 2011, 9, 71 CrossRef CAS PubMed
. - R. Raghu, V. Devaraji, K. Leena, S. D. Riyaz, P. Baby Rani, P. Kumar Naik, P. K. Dubey, D. Velmurugan and M. Vijayalakshmi, Virtual screening and discovery of novel aurora kinase inhibitors, Curr. Top. Med. Chem., 2014, 14, 2006–2019 CrossRef CAS PubMed
. - D. Shivakumar, J. Williams, Y. Wu, W. Damm, J. Shelley and W. Sherman, Prediction of absolute solvation free energies using molecular dynamics free energy perturbation and the OPLS force field, J. Chem. Theory Comput., 2010, 6, 1509–1519 CrossRef CAS PubMed
. - I. Aier, P. K. Varadwaj and U. Raj, Structural insights into conformational stability of both wild-type and mutant EZH2 receptor, Sci. Rep., 2016, 6, 34984 CrossRef CAS PubMed
. - Q. Ge, D. Lou, J. Zeng, C. Pan, S. Wang, W. Zhang, L. Zhang and X. Wang, Structural evolution of imidazolium-based poly(ionic liquid) assemblies during solvent evaporation, eXPRESS Polym. Lett., 2017, 11, 84–95 CrossRef CAS
. - B. B. Polesso, R. Duczinski, F. L. Bernard, H. Z. Ferrari, M. da Luz, F. D. Vecchia, S. M. C. de Menezes and S. Einloft, Enhancement of CO2/N2 selectivity and CO2 uptake by tuning concentration and chemical structure of imidazolium based ILs immobilized in mesoporous silica, Mater. Res., 2019, 22, 84–95 Search PubMed
. - D. Danielewicz, M. Kmiotek and B. Surma-Ślusarska, Properties and fibre characterisation of bleached hemp, birch and pine pulps : a comparison, Fibres Text. East. Eur., 2019, 27, 118–123 CrossRef CAS
. - D. F. Montaño, H. Casanova, W. I. Cardona and L. F. Giraldo, Functionalization of monomorillonite with ionic liquids based on 1-alkyl-3-methylimidazolium: Effect of anion and length chain, Mater. Chem. Phys., 2017, 198, 386–392 CrossRef
. - G. Ameta, A. K. Pathak, C. Ameta, R. Ameta and P. B. Punjabi, Sonochemical synthesis and characterization of imidazolium based ionic liquids: A green pathway, J. Mol. Liq., 2015, 211, 934–937 CrossRef CAS
. - A. Ahmed, Y. Chaker, E. H. Belarbi, O. Abbas, J. N. Chotard, H. B. Abassi, A. N. Van Nhien, M. El Hadri and S. Bresson, XRD and ATR/FTIR investigations of various montmorillonite clays modified by monocationic and dicationic imidazolium ionic liquids, J. Mol. Struct., 2018, 1173, 653–664 CrossRef CAS
. - S. Pradhan and S. Mishra, A thermodynamic investigation of solute-solvent interactions through volumetric, ultrasonic, dielectric, refractive and excess properties of binary mixtures of Tri-n-butyl phosphate with dichloro, trichloro and tetrachloromethane at 298.15 K, J. Mol. Liq., 2019, 279, 561–570 CrossRef CAS
. - S. Pradhan, S. K. Behera, S. K. Samal, I. Panda, P. K. Sahu and S. Priyadarshini, Interaction between 2-methylimidazole and 1-butanol/1-octanol: Thermophysical and computational studies, ChemistrySelect, 2023, 8(13), e202204931 CrossRef CAS
. - S. Thawarkar, N. D. Khupse and A. Kumar, Solvent-mediated molar conductivity of protic ionic liquids, Phys. Chem. Chem. Phys., 2015, 17, 475–482 RSC
. - S. Behera, N. Mahapatra, C. Tripathy and S. Pati, Drug repurposing for identification of potential inhibitors against SARS-CoV-2-spike receptor binding domain: An in silico approach, Indian J. Med. Res., 2021, 153, 132 CrossRef CAS PubMed
.
|
This journal is © The Royal Society of Chemistry 2024 |