DOI:
10.1039/D3RA07371H
(Review Article)
RSC Adv., 2024,
14, 2590-2601
Novel applications of photobiocatalysts in chemical transformations
Received
29th October 2023
, Accepted 28th December 2023
First published on 15th January 2024
Abstract
Photocatalysis has proven to be an effective approach for the production of reactive intermediates under moderate reaction conditions. The possibility for the green synthesis of high-value compounds using the synergy of photocatalysis and biocatalysis, benefiting from the selectivity of enzymes and the reactivity of photocatalysts, has drawn growing interest. Mechanistic investigations, substrate analyses, and photobiocatalytic chemical transformations will all be incorporated in this review. We seek to shed light on upcoming synthetic opportunities in the field by precisely describing mechanistically unique techniques in photobiocatalytic chemistry.
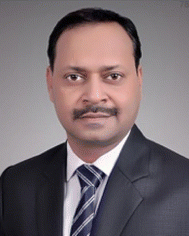 Praveen P. Singh | Praveen P. Singh is an Assistant Professor in the Department of Chemistry at the United College of Engineering and Research, Prayagraj, India. He obtained his BSc and MSc degrees in Organic Chemistry from T. D. P. G. College (V. B. S. Purvanchal University) Jaunpur and his DPhil. from the Department of Chemistry, University of Allahabad, India. His current research interests include the development of synthetic receptors for the recognition of biological target structures and the application of visible light chemical photocatalysis towards organic synthesis as well as nanophotocatalysis. |
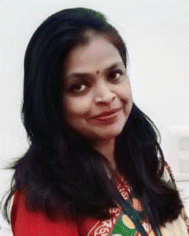 Surabhi Sinha | Surabhi Sinha is presently working as an Assistant Professor in the Department of Chemistry, United College of Engineering & Research, Naini, Prayagraj. She completed her graduate and post-graduate studies and DPhil. at the University of Allahabad, Prayagraj. Her research interests mainly include quaternary complexes of biochemical significance and organic synthetic transformations. She is a life member of several professional bodies. |
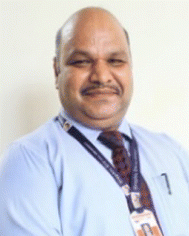 Pankaj Nainwal | Dr Pankaj Nainwal is a Professor in the School of Pharmacy, Graphic Era Hill University, Dehradun, Uttarakhand. He is an alumni of the JSS College of Pharmacy, Ootacmund, Tamil Nadu. During his academic journey he has taught in universities in various states. He specializes in pharmacognosy, phytochemistry, phytopharmacy, phytomedicines and photocatalysis. |
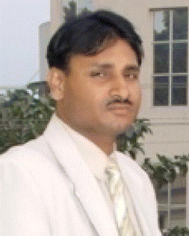 Pravin K. Singh | Pravin K. Singh is an Assistant Professor at the Department of Chemistry, C. M. P. College, Allahabad, India (Constituent P. G. College of Central University of Allahabad). Dr Singh is actively engaged in advanced research work for the development of environmentally benign, new synthetic routes for various bioactive heterocyclic compounds. He completed his BSc, MSc, doctorate (DPhil.) and post-doctorate (DSc) studies at the University of Allahabad, India. |
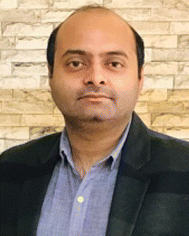 Vishal Srivastava | Vishal Srivastava is an Assistant Professor in the Department of Chemistry, C. M. P. College, (Constituent P. G. College of Central University of Allahabad) Prayagraj, India. He completed his graduate (BSc) and post-graduate (MSc) studies and his doctoral degree (DPhil.) in organic chemistry at the Department of Chemistry, University of Allahabad, India. His current research work involves the design of novel biologically active photoredox catalysed synthetic organic compounds. |
1. Introduction
Considering that it is secure to use and easily accessible, light is the ideal energy source for environmentally friendly chemical synthesis.1 The leading scientist Ciamician presented his ideas in the lecture “Photochemistry of the Future” in 1912, and predicted that sunlight, a plentiful and renewable source, could be used to create compounds in a manner similar to plants and that solar energy in organic synthesis had a lot of potential.2–4
Photocatalysis and biocatalysis (photobiocatalysis)5–10 have attracted a lot of attention in the field of catalysis due to features that make these chemical synthesis methods more effective and environmentally sustainable. As enzymes may facilitate complex processes with excellent stereoselectivity and efficiency in water-based media at room temperature, the pharmaceutical and chemical industries have adopted biocatalysts in growing numbers as tools for green chemistry.11–14 In contrast, photocatalysis has become a potent method that uses visible light excitation to reach particular reactivities, via the formation of open-shell intermediates, that are inaccessible via thermal activation modes. While in the past organic compounds were directly activated using ultraviolet (UV) light, modern photochemical activation approaches depend on the selective excitation of photocatalysts with visible light and can prevent the harmful destruction of organic molecules brought on by using high-energy UV light. Through the conversion of photonic energy into chemical energy, visible light-mediated photocatalysis offers a gentle, long-lasting, and clean technique for chemical activation and has been utilised to facilitate numerous challenging transformations.15–21
Replicability and reproducibility challenges are frequently raised by researchers in novel fields of research, including in photobiocatalysis. Studies are often conducted by a select group of laboratories utilising their own in-house solutions to run and investigate reactions. It is therefore vital to provide criteria for reporting the technical and chemical specifics of photobiocatalytic reactions as the subject advances to broader applicability. Since these guidelines have been shown to be significant for both biocatalysis and photocatalysis, we emphasise here the characteristics that are particularly relevant to photobiocatalysis (Fig. 1).22
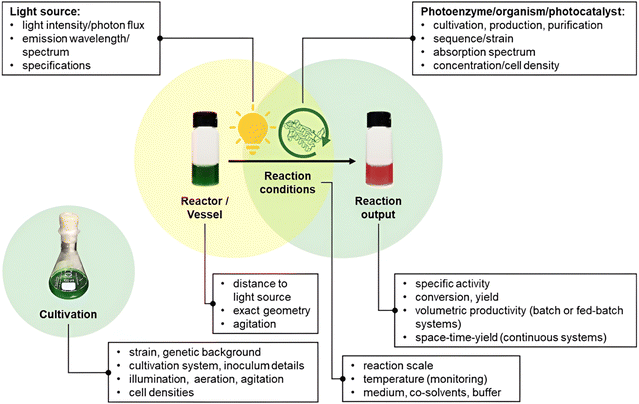 |
| Fig. 1 General criteria for reporting photobiocatalytic reactions. Reproduced with permission from ref. 22. Copyright © 2023 The Authors. ChemPhotoChem published by Wiley-VCH GmbH. | |
Photobiocatalysis makes use of excellent enzyme selectivity and the distinctive chemical changes that photocatalysis is capable of to carry out chemical transformations (Fig. 2).23–26 Because enzymes are highly evolved for specific biological functions, their limited synthetic potential can be addressed by providing them with additional reactivity by using photoactive cofactors like flavin or nicotinamide adenine dinucleotide (phosphate) (NAD(P)H) or photocatalysts like ruthenium and iridium organometallic complexes, organic dyes, etc.27,28 The protein framework of enzymes, in turn, can provide a regulated environment that provides possibilities to direct reactive intermediates toward the required stereo- and chemo-selective outcome, a current issue for conventional photocatalysts.29 The problems of compatibility between enzymes and photocatalysts which arise because they both work under different reaction conditions and the kinetics of the photogenerated reactive intermediates and enzyme catalysis are obstacles to merging the two types of catalysis. Furthermore, it is difficult to employ protein engineering techniques for the improvement of photoenzymatic systems due to the challenges in achieving uniform illumination and an inert atmosphere for a significant amount of samples.30,31
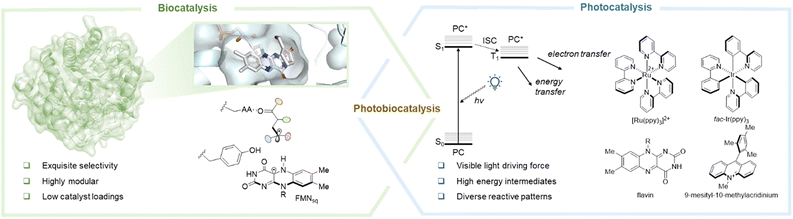 |
| Fig. 2 In the presence of light, the combination of photocatalysis and biocatalysis simplifies challenging reactions. Photocatalyst = PC; S0 = singlet ground state; S1 = first singlet excited state; T1 = first triplet excited state; FMNsq = semiquinoneflavin mononucleotide. Reproduced with permission from ref. 26. Copyright © 2022 American Chemical Society. | |
The rapidly expanding field of photobiocatalysis is devoted to the creation of novel light-dependent biocatalytic processes.32–37 The link between the photochemical process and the enzymatic transformation will be used to group reports in this evaluation. We will clarify crucial terms and concentrate on subtle mechanistic differences that set several techniques apart from one another. We aim to add to the understanding of how light can be employed in biocatalytic synthesis by describing the mechanistic differences between various techniques.
The first technique focuses mostly on photoenzymatic catalysis, where a cofactor inside a protein active site is photoexcited, it facilitates the electron or energy transfer necessary to change a starting material into a finished product (Fig. 3a). Synergistic photoenzymatic catalysis is addressed in the second approach (Fig. 3b). In these processes, an external cofactor is excited to enable a chemical change within a protein active site. The third procedure incorporates tandem photocatalyst/enzyme reactions (Fig. 3c). In these trans-formations, the photochemical reaction takes place in the presence of the enzyme but is not a part of the mechanism by which the enzyme transforms raw materials into the end products. This procedure is further divided into (i), processes that use light to regenerate cofactors and (ii), reactions where the enzyme substrate is altered by photoexcitation. The fourth method addresses natural photosynthesis and enzymatic processes. Through the utilisation of cyanobacteria, these systems generate NADPH, which enzymes can use to reduce substrates (Fig. 3d). This concise review deals with photobiocatalysis as an effective method in energy production and chemical manufacture and is a follow-up to our work on photocatalyzed organic synthesis,38,39 paying particular attention to the most recent and noteworthy developments in this field. Those already working in the field of photoredox catalysed synthesis and interested in the usage of photobiocatalysis-driven chemical and energy production may find this review to be particularly useful.
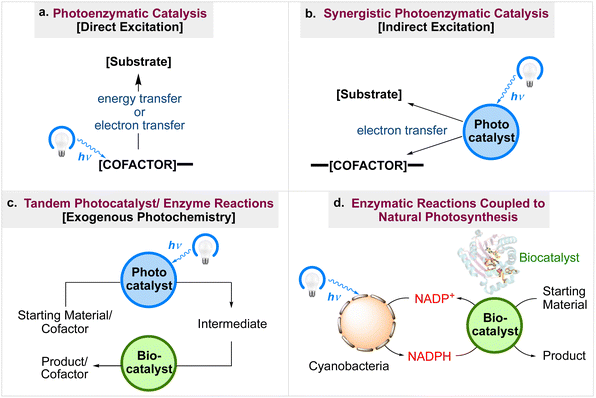 |
| Fig. 3 Types of photobiocatalysis used in chemical transformations.34 | |
2. Applications of photobiocatalysts in chemical transformations
2.1. Photobiocatalytic H2 production
The long-term goal of researchers and a potential remedy for energy problems, environmental degradation, and global warming is to transform renewable solar energy into fuels and value-added chemicals. In order to efficiently capture and store solar energy in chemical bonds, photosynthetic biohybrid systems (PBS) can utilise both artificial semiconductor materials with high solar conversion efficiency and living cells with high product selectivity.40
An intriguing approach for producing H2 has been established through photobiocatalysis. Kosem et al.41 have examined the natural properties of biocatalysts and the significance of each component in determining the effectiveness of the system. Tris (2-amino-2-hydroxymethyl-1,3-propanediol) was shown via photocatalytic research to be the optimal electron donor for the reduction of viologen by TiO2 (Scheme 1). A study of the biocatalytic reaction showed that the function of the whole-cell biocatalysts was strongly influenced by cell permeability, the redox potential of the electron mediators, and the cell envelope. Recombinant Escherichia coli, which has a turnover frequency of 39.43 3.77 s−1 based on [FeFe]-hydrogenase activity, was illustrated to be a more effective biocatalyst than Anabaena variabilis in a photobiocatalytic system. A thorough investigation revealed that Tris and MV2+ had less of an impact on H2 synthesis than TiO2, light, and the biocatalysts. With a solar-to-H2 conversion of 1.58 (0.10%), a maximum rate of 16.73 1.03 mol min−1 was achieved. The production of highly effective photobiocatalysts will be guided by an understanding of the functions of each component. Table 1 provides information on the capabilities of hydrogen evolution photobiocatalytic systems, including relative H2 yield (to bare cells) and endurance.
 |
| Scheme 1 In the presence of Tris as a sacrificial agent and MV2+ as an electron mediator, a TiO2 photobiocatalytic H2 generation mechanism was coupled to two distinct whole-cell biocatalysts: recombinant E. coli (A) or the cyanobacterium A. variabilis (B). Reproduced with permission from ref. 41. Copyright © 2023 Elsevier. | |
Table 1 Summary of the performance of hydrogen evolution photobiocatalytic systems, including relative H2 yield (to bare cells) and duration
Species |
Photosensitizer |
Efficiency/yielda |
Relative H2 yield to bare cells |
Duration |
Ref. |
The apparent quantum efficiency (AQE) of a light-driven H2 production system is the number of additional evolved H2 molecules multiplied by 2 and divided by the number of incident photons. |
Azotobactervinelandii |
CdS–ZnS quantum dots |
AQEa 13% |
NA |
2 h |
42 |
Desulfovibriodesulfuricans |
CdS, methyl viologen |
AQE 23% |
NA |
50 h |
43 |
Desulfovibriodesulfuricans |
CdS |
AQE 4% |
NA |
10 d |
43 |
Escherichia coli |
TiO2 |
AQE 0.31% |
NA |
15 h |
44 |
Escherichia coli |
CdS |
AQE 7.93% |
1.3 fold |
3 h |
45 |
Escherichia coli |
AgInS2/In2S3 |
AQE 3.3% |
1.3 fold |
3 h |
46 |
Escherichia coli |
CdS |
Yield 81 μmol/108 cells |
10 fold |
24 h |
47 |
Escherichia coli |
TiO2 |
Yield 3.6 mmol per mmol glucose |
2.8 fold |
15 h |
48 |
Escherichia coli |
Eosin Y |
AQE > 10% |
>10 fold |
24 h |
49 |
Escherichia coli |
Iodine-doped hydrothermally carbonized carbon |
AQE 9.11% |
1.57 fold |
3 h |
50 |
Klebsiella pneumonia |
Hydrothermal carbonation carbon |
Yield 1020 μmol |
1.35 fold |
3 h |
51 |
Rhodopseudomonaspalustris |
CdS nanoparticles |
Photosynthetic efficiency 6.73% |
2 fold |
120 h |
52 |
Rhodopseudomonaspalustris |
Oligofluorene, polythiophene |
Yield 1250 nmol |
∼1.67 fold |
2 h |
53 |
Shewanellaoneidensis |
Eosin Y |
AQE 0.6% |
>10 fold |
24 h |
54 |
Shewanellaoneidensis |
Cu2O, reduced graphene oxide |
Yield 322 μmol per g Cu2O |
>10 fold |
4 h |
55 |
Shewanellaoneidensis |
CuInS2/ZnS quantum dots |
AQE 15.02% |
10 fold |
45 h |
56 |
2.2. Photobiocatalytic CO2 reduction and conversion
A promising technique to address climate challenges and store solar energy is the conversion of CO2 into value-added compounds utilising renewable solar energy as the driving force. In addition, when compared to the conversion of solar energy to hydrogen, the conversion of solar energy to organic molecules has the advantage of the products being easy to transport and store.57–65 The descriptions of the capabilities of the photobiocatalytic CO2 conversion systems are given in Table 2. The mechanism66a,b by which the production of CO2RR by photocatalysis proceeds is given in Scheme 2.
Table 2 Summary of the performance of photobiocatalytic CO2 conversion systems
Species |
Materials |
Products |
Efficiency/yield |
Duration |
Ref. |
The apparent quantum efficiency (AQE) refers to the ratio of electrons used to convert the substrate to product and the incident photons. |
Azotobactervinelandii |
CdS/ZnS QDs |
Formic acid |
Turnover number 106 |
NA |
42 |
Cupriavidusnecator |
CdS/ZnS QDs |
C2H4, isopropanol, 2,3-butanediol, C11–C15 methyl ketones, polyhydroxybutyrate |
Turnover number 106–108 |
NA |
42 |
Escherichia coli |
CdS |
L-Malate and butyrate |
L-Malate yield = 1.48 mol per mol glucose |
4 days |
67 |
Butyrate yield = 0.79 mol per mol glucose |
Methanosarcinabarkeri |
CdS |
Methane |
AQEa = 0.34% |
5 days |
68 |
Methanosarcinabarkeri |
Ni:CdS |
Methane |
AQE = 2.08% |
6 days |
69 |
Moorellathermoacetica |
PDI/PFP |
Acetic acid |
AQE = 1.6% |
3 days |
70 |
Moorellathermoacetica |
CdS |
Acetic acid |
AQE = 2.44% |
4 days |
71 |
Moorellathermoacetica |
CdS NPs, TiO2–Mn phthalocyanine |
Acetic acid |
Yield ∼1.2 mM |
3.5 days |
72 |
Moorellathermoacetica |
Au NC |
Acetic acid |
AQE = 2.86% |
7 days |
73 |
Moorellathermoacetica |
Au NC, alginate |
Acetic acid |
Yield ∼ 1.4 mM |
3 days |
74 |
Rhodopseudomonaspalustris |
CdS QDs |
Methane |
Yield ∼171 nmol per mg total protein |
4 days |
75 |
Rhodopseudomonaspalustris |
CdS NPs |
Carotenoid, PHB |
Photosynthetic efficiency 5.98% |
8 days |
76 |
Sporomusaovata |
Silicon nanowire |
Acetate |
AQE = 3.6% |
7 days |
77 |
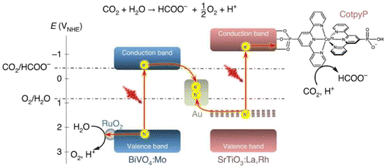 |
| Scheme 2 An energy diagram depicting photosynthetic CO2RR production coupled with water oxidation. The reduction potentials are given versus the NHE at pH 6.7. Reproduced with permission from ref. 66a. Copyright 2020 Springer Nature Publishing AG. | |
2.3. Coordination of electron transfer and enzyme protection
In order to coordinate electron transmission and enzyme protection for photo-enzymatic alcohol synthesis, Jiang and colleagues reported78 a metal hydride-embedded titania (MH/TiO2) coating that is engineered on graphitic carbon nitride (GCN). The MH/TiO2 coating serves two essential functions: (1) it prevents the GCN core and coating from deactivating alcohol dehydrogenase (ADH); and (2) it allows electron transfer from GCN to nicotinamide adenine dinucleotide (NAD+) and then to the alcohol dehydrogenase-catalyzed form of formaldehyde. The coordinated photo-enzymatic system was able to create methanol at a rate of 1.78 0.21 mol min−1 mg(ADH)−1, which is 420% more than the rate of the system made up of ADH and GCN without the coating. Additionally, the coordinated system is capable of producing methanol constantly for a minimum of three light–dark cycles, whereas the GCN and ADH system entirely shuts down after one light–dark cycle. By combining synthetic and biological modules for solar chemical-based conversion, this study reveals the potential of redox-active mineral coverings (Scheme 3).
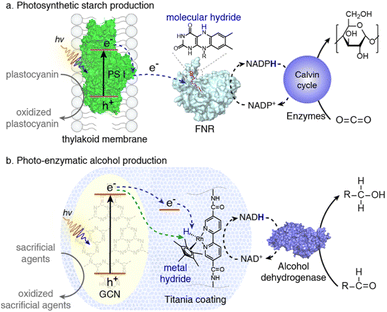 |
| Scheme 3 (a) Production of photosynthetic starch using a thylakoid membrane and FNR with molecular hydride integration. (b) MH-embedded TiO2 layer for photo-enzymatic alcohol synthesis. Reproduced with permission from ref. 78. Copyright © 2020 American Chemical Society. | |
2.4. Photobiocatalytic conversion of proteomembranes into artificial chloroplasts
By employing inverted E. coli vesicles and heterodinuclear tpphz-bridged Ru–Rh photocatalysts, Rau et al. presented79 a hybrid system that can concurrently produce the two physiologically active cofactors NADH and ATP while drawing energy from an external source, such as visible light. Thus, it mimics how natural chloroplasts work, which is to produce reduced nicotinamides and ATP for subsequent energy-demanding reductive cascade reactions. It was discovered by investigating the various ATP synthesis steps that the photocatalytically produced NADH actually acidifies the inside of the proteomembrane vesicles. The phosphorylation of glucose ultimately results from the usage of the resultant pmf, transforming ADP and Pi into ATP. In green plants, photochemically generated ATP is used in a biochemically comparable phosphorylation reaction to activate ribulose-5-phosphate for CO2 fixation and reduction. Thus, the final step of the studied reaction is also similar to the natural chloroplast system. Additionally, it was discovered that the overall charge and lipophilicity of the coordination compounds can influence how the examined Ru polypyridine complexes interact with the E. coli-generated vesicles. These findings pave the way for more applications, such as chain reactions using both the cofactors ATP and NADH. If appropriate enzymes were added to the cofactor-producing inorganic–biologic hybrid system indicated, energy-intensive reductive activations of N2, as well as CO2 fixation processes would be feasible (Scheme 4).
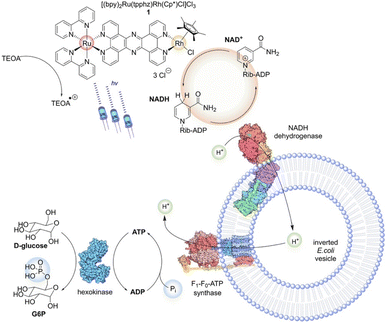 |
| Scheme 4 An illustration of the photobiocatalytic process that uses inverted E. coli vesicles as the main cofactor conversion machinery to connect the photocatalytic NADH generation by 1 with enzymatic ATP and G6P production. Reproduced with permission from ref. 79. Copyright © 2021 The Authors. Angewandte Chemie International Edition published by Wiley-VCH GmbH. | |
2.5. Morpholine-based buffers that activate aerobic photobiocatalysis
According to the findings of Gonçalves et al.,80 morpholine-based buffers, particularly 3-(N-morpholino)propanesulfonic acid (MOPS), encourage photoinduced flavoenzyme-catalyzed asymmetric redox transformations by regenerating the flavin cofactor through sacrificial electron donation and by raising the functional stability of flavin-dependent oxidoreductase. In order to solve the oxygen problem in aerobic conditions, which is harmful to delicate enzymes, the active forms of flavin are stabilised by MOPS by creating a spin correlated ion pair ensemble, 3[flavin-MOPS+] (Scheme 5).
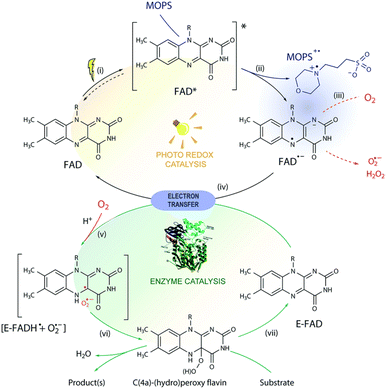 |
| Scheme 5 Mechanism that has been proposed for MOPS-mediated photobiocatalysis. Steps of the photoredox cycle: (i) the oxidized FAD is excited by light to FAD*, capable of (ii) oxidizing MOPS. The resulting FAD semiquinone (FAD˙−) can (iii) generate ROS in the presence of O2 via electron transfer or (iv) reduce the oxidized FAD bound to the enzyme (E-FAD), regenerating its oxidized form FAD. Steps of the biocatalytic cycle: (v) the resulting caged radical pair [E-FADH˙ + O2˙−] forms (vi) C(4a)-(hydro)peroxyflavin, responsible for the conversion of the substrate, and E-FAD is regenerated (vii). The ROS produced may induce enzyme deactivation, which is minimized in the presence of MOPS due to stabilization of the FAD semiquinone via formation of a [FAD˙−–MOPS˙+] ensemble (in purple). For simplicity, protonation equilibria are not shown. Reproduced with permission from ref. 80. Copyright © 2019 Royal Society of Chemistry. | |
2.6. Photobiocatalytic artificial dehalogenase for cross-coupling reactions
A light-harvesting metallo-enzyme platform for organometallic cross-coupling reactions under moderate conditions has been created by Wang et al.81 It is feasible to increase the synergism of dual catalysis by rationally combining an artificial photosensitizer (i.e., benzophenone) and a NiII(bpy) complex, two catalytic entities that are relatively incompatible in solution. The effective transformations of various aryl halides to phenols and a useful C–N bond formation were two examples of the catalytic utility. The use of the NiII cofactor, a wholly synthetic metal complex, also differs significantly from the most popular photobiocatalytic procedures that rely on natural redox enzymes. In addition, this synthetic enzyme is the first dehalogenase used for organic synthesis, which complements natural counterparts that are only known for bioremediation. As a result, this current study opens up new possibilities for combining synthetic photo and biocatalysts to push the limits of artificial enzyme catalysis for a variety of difficult bond configurations (Schemes 6 and 7).
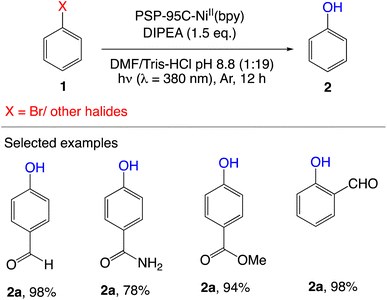 |
| Scheme 6 Artificial dehalogenase for cross-coupling.81 | |
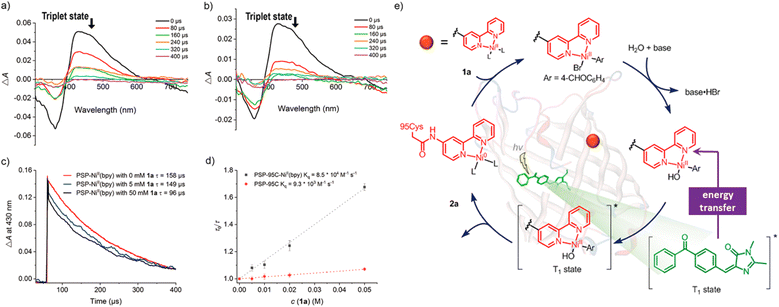 |
| Scheme 7 Transient absorption studies and proposed plausible reaction mechanism. (a) PSP-95C-bpy in Tris–HCl pH 8.8 buffer. (b) PSP-95C-NiII(bpy) in Tris–HCl pH 8.8 buffer with bromide 1a and DIPEA. (c) Kinetic traces of PSP-95C-NiII(bpy) with 1a recorded at 430 nm in the presence of DIPEA. (d) Stern–Volmer plots and extracted quenching rate constants. (e) Plausible reaction mechanism. Reproduced with permission from ref. 81. Copyright © 2021 American Chemical Society. | |
2.7. Aerobic photobiocatalysis
Due to the variety of enzymes available, their high catalytic activities and specificities, and the environmental friendliness of the processes, biocatalytic transformation has gained increasing attention in the field of green chemical synthesis. The majority of redox enzymes in nature rely on nicotinamide cofactors such as NAD+/reduced NAD+/nicotinamide adenine dinucleotide (NADH). An exceptional possibility to create fully integrated green processes is provided by the utilisation of solar energy, particularly visible light, in the creation of cofactors through the coupling of photocatalysis and biocatalysis. However, the quick decomposition and inactivation of the enzymatic material caused by photogenerated reactive oxygen species (ROS) has made the use of photocatalysts and enzymes difficult. Li et al. developed82 core–shell structured polymer micelles and vesicles with aggregation-induced emission (AIE) properties as visible-light mediated photocatalysts for extremely stable and recyclable photobiocatalysis under aerobic conditions. The photoactive hydrophobic core of the polymer micelles and the hydrophobic membrane of the polymer vesicles can effectively regenerate NAD+ from NADH, while the hydrophilic surface layer of the polymer colloids protects the enzymatic material (glucose 1-dehydrogenase) from the attack of photogenerated ROS. The enzyme maintains its active state after at least 10 regeneration cycles, and the polymer micelles and vesicles continue to function as photocatalysts. These polymer colloids could potentially help to establish commercially viable photobiocatalytic systems (Scheme 8).
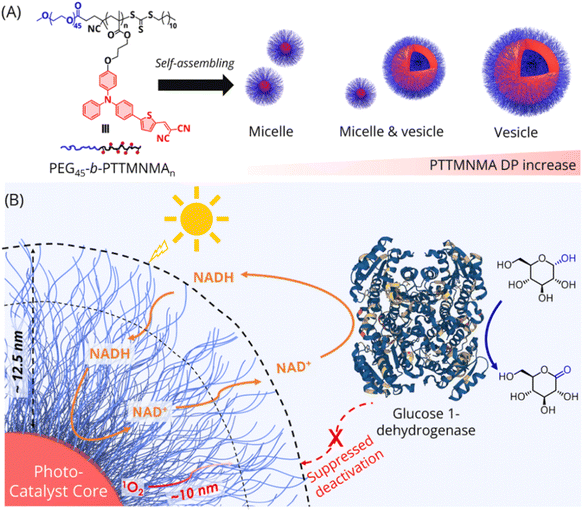 |
| Scheme 8 (A) The micelle and vesicle models of the chemical structure of the TTMN-based AIE block copolymers. (B) A demonstration of the aerobic photobiocatalysis system using glucose 1-dehydrogenase and a micelle/vesicle photocatalyst to carry out tandem photobiocatalytic reactions in aqueous solution. Reproduced with permission from ref. 82. Copyright © 2022 American Chemical Society. | |
2.8. Triplet–triplet annihilation-based photon-upconversion
The application of synthetic organic chemistry has been substantially expanded, particularly through the use of light-driven enzymatic catalysis. However, the restricted wavelength range of visible (sun)light can often only utilised by photoenzymes. Using triplet–triplet annihilation-based upconversion (TTA-UC), which transforms light with long wavelengths into light with shorter wavelengths, the wavelength range can be expanded. In their study on the viability of light upconversion, Park et al. developed83 TTA-UC poly(styrene) (PS) nanoparticles that were doped with a platinum(II) octaethylporphyrin (PtOEP) photosensitizer and a 9,10-diphenylanthracene (DPA) annihilator (PtOEP:DPA@PS) Using 550 nm light, PtOEP:DPA@PS nanoparticles were photoexcited, resulting in the upconverted emission of DPA at 418 nm. With a high energy transfer efficiency, the TTA-UC emission may photoactivate flavin-dependent photodecarboxylases. As a result, under green light irradiation (λ = 550 nm), the photodecarboxylase from Chlorella variabilis NC64A was able to catalyse the conversion of fatty acids into long chain secondary alcohols (Scheme 9).
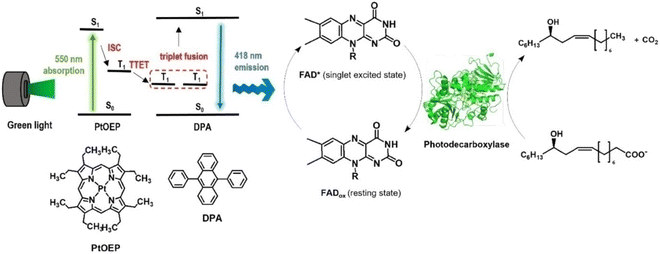 |
| Scheme 9 Overall concept of triplet–triplet annihilation-based photon-upconversion (TTA-UC) for light-driven enzymatic catalysis. The TTA-UC allows the conversion of long wavelength light (λ = 550 nm) to short wavelength light (λ = 418 nm), which activates FAD in the enzymes for catalysis. PtOEP: platinum(II) octaethylporphyrin, DPA: 9,10-diphenylanthracene, ISC: intersystem crossing, TTET: triplet–triplet energy transfer. Reproduced with permission from ref. 83. Copyright © 2022, The Author(s). | |
2.9. Photobiocatalytic synthesis of enantiopure 1-arylpropane-2-ols
Rodríguez-Fernández et al. have researched84 how light-driven and biocatalyzed processes can work together. First, a photocatalytic Meerwein arylation was investigated to produce a series of 1-arylpropan-2-ones in aqueous solution using [Acr-Mes]ClO4, an organic photosensitizer. Following the light-induced synthesis of the aryl radicals of the diazonium salt, an oxygenated radical intermediate was oxidised by the excited acridinium photocatalyst, followed by intramolecular electron transfer, producing the final products in a range of yields (15–95%). In order to reduce the ketone intermediates, stereo-complementary alcohol dehydrogenases were used, taking advantage of the mild conditions used in this stage. Numerous valuable 1-arylpropan-2-ols were produced with low to good overall yields (14–76%) and excellent stereoselectivity (90 to >99% ee) via the screening various ADHs for the enzymatic carbonyl reduction and the optimisation of the reaction conditions to facilitate the sequential photobiocatalytic linear approach (Scheme 10).
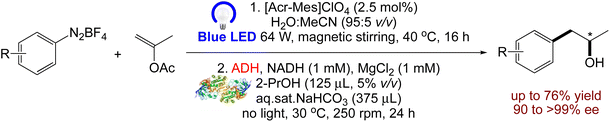 |
| Scheme 10 Synthesis of enantiopure 1-arylpropane-2-ols from aryl diazonium salts via a photobiocatalytic cascade.84 | |
2.10. Photobiocatalytic asymmetric synthesis
Hyster et al. reported that flavin-dependent “ene”-reductases can catalyze the asymmetric synthesis of tertiary alcohols via a photoenzymatic alkene carbohydroxylation (Scheme 11a).85 Mechanistic investigations indicate that the production of C–O bonds happens via a 5-endo-trig cyclization with the pendant ketone, resulting in an α-oxy radical that is subsequently hydrolyzed and oxidised to generate the product. Similarly, they also reported a highly chemo- and stereoselective C-alkylation of nitroalkanes with alkyl halides catalyzed by an engineered flavin-dependent “ene”-reductase (ERED) (Scheme 11b).86 According to a mechanistic investigation, radical initiation is triggered by the excitation of an enzyme-templated charge–transfer complex that develops between the substrates and cofactor. Furthermore, they also reported a highly chemoselective and enantioselective Csp3–Csp3 photoenzymatic cross-electrophilic coupling (XEC) between alkyl halides and nitroalkanes catalysed by flavin-dependent ‘ene’-reductases (EREDs) (Scheme 11c).87 This synthetic methodology demonstrates the unprecedented efficacy of biocatalysts in controlling stereoselectivity and differentiating Csp3 electrophile substrates. In continuation of their work on asymmetric synthesis, the Hyster group have also carried out several other organic chemical transformations88 using photobiocatalysis.
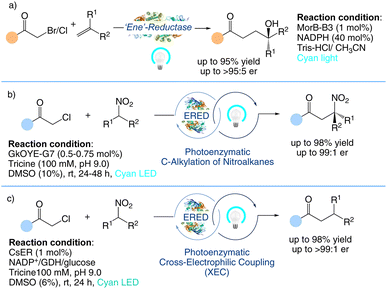 |
| Scheme 11 Photobiocatalytic asymmetric synthesis. (a) Asymmetric carbohydroxylation of alkenes.85 (b) Asymmetric C-alkylation of nitroalkanes.86 (c) Asymmetric sp3–sp3 cross-electrophile coupling.87 | |
3. Future prospects for photobiocatalysis
Since photobiocatalysis is still in its infancy, there are undoubtedly many obstacles as well as much potential in this emerging field. In this review, we have covered the most recent advances in the merging of photocatalysis with biocatalysis and its application in chemical transformations.31 This field has developed several new opportunities with various challenges. In fact, the substance used as the photocatalyst plays a role in the conversion of light energy. The catalytic performance of the cascade system will be further enhanced by using strong, superior photocatalysts and enzymes that are complementary to one another. It might be possible to accomplish difficult conversions with the combination of novel catalysts. The combination of controlled enzyme evolution and innovative biocatalytic reaction mechanisms holds enormous potential for addressing long-standing issues in chemical synthesis. The application of photobiocatalytic techniques will definitely enhance the range of chemical reactions that can be carried out by organic chemists. The use of photobiocatalysis will continue to be advanced further with new concepts and approaches.
4. Conclusions
In recent years, photobiocatalysis has grown at an exponential rate, revealing important variations in the biological processes involved, the most important of which is the way light drives the chemical transformation. Synthetic organic chemists have a growing awareness of the potential of photocatalysis and its application in synthetic problem-solving and are becoming more well-versed than ever. The potential to synthesise fine compounds in this rapidly developing area has been demonstrated by the tactics that have emerged in the last decade to combine photocatalysis with biocatalysis in an advantageous manner. Without the need for harsh chemicals or heat energy, visible light photoexcitation of naturally existing photoactive enzymatic cofactors might facilitate challenging reactions that are not achievable using ground-state catalysis. Although photocatalysis can facilitate a wider range of chemical transformations, biocatalysis benefits from the high selectivity that photochemistry at enzyme active sites provides, something made possible by the defined environment of the biocatalyst. However, for photobiocatalysis to be widely used, there must be defined guidelines for reporting photobiocatalytic experiments and access to reasonably priced, well-characterized illumination equipment. It is anticipated that light-dependent enzymatic reactions will become a common tool in biocatalytic laboratories as a result of all these discoveries.
Conflicts of interest
There are no conflicts to declare.
References
- T. P. Yoon, M. A. Ischay and J. Du, Nat. Chem., 2010, 2, 527–532 CrossRef CAS PubMed.
- G. Ciamician, Science, 1912, 36(926), 385–394 CrossRef CAS.
- A. Albini and M. Fagnoni, Green Chem., 2004, 6, 1–6 RSC.
- L. Candish, K. D. Collins, G. C. Cook, J. J. Douglas, A. Gómez-Suárez, A. Jolit and S. Keess, Chem. Rev., 2022, 122, 2907–2980 CrossRef CAS.
- Z. C. Litman, Y. Wang, H. Zhao and J. F. Hartwig, Nature, 2018, 560, 355–359 CrossRef CAS PubMed.
- Y. Wang, X. Huang, J. Hui, L. T. Vo and H. Zhao, ACS Catal., 2020, 10, 9431–9437 CrossRef CAS.
- X. Huang, B. Wang, Y. Wang, G. Jiang, J. Feng and H. Zhao, Nature, 2020, 584, 69–74 CrossRef CAS.
- X. Huang, J. Feng, J. Cui, G. Jiang, W. Harrison, X. Zang, J. Zhou, B. Wang and H. Zhao, Nat. Catal., 2022, 5, 586–593 CrossRef CAS.
- N. Yang, Y. Tian, M. Zhang, X. Peng, F. Li, J. Li, Y. Li, B. Fan, F. Wang and H. Song, Biotechnol. Adv., 2022, 54, 107808 CrossRef CAS.
- Y. Tan, J. Ma, F. Zhang, S. Wang, F. Lan, H. Liu and R. Li, ACS Sustainable Chem. Eng., 2022, 10, 12065–12071 CrossRef CAS.
- S. Wu, R. Snajdrova, J. C. Moore, K. Baldenius and U. T. Bornscheuer, Angew. Chem., Int. Ed., 2021, 60, 88–119 CrossRef CAS.
- L. Hilterhaus, A. Liese, U. Kettling and G. Antranikian, Applied Biocatalysis: From Fundamental Science to Industrial Applications, Wiley-VCH, Weinheim, 2016 Search PubMed.
- J. M. Choi, S. S. Han and H. S. Kim, Biotechnol. Adv., 2015, 33, 1443–1454 CrossRef CAS.
- R. A. Sheldon and J. M. Woodley, Chem. Rev., 2018, 118, 801–838 CrossRef CAS PubMed.
- C. R. J. Stephenson, T. P. Yoon and D. W. C. MacMillan, Visible Light Photocatalysis in Organic Chemistry, Wiley-VCH, Weinheim, 2017 Search PubMed.
- M. H. Shaw, J. Twilton and D. W. C. MacMillan, J. Org. Chem., 2016, 81, 6898–6926 CrossRef CAS PubMed.
- N. A. Romero and D. A. Nicewicz, Chem. Rev., 2016, 116, 10075–10166 CrossRef CAS PubMed.
- J. M. R. Narayanam and C. R. J. Stephenson, Chem. Soc. Rev., 2011, 40, 102–113 RSC.
- L. Marzo, S. K. Pagire, O. Reiser and B. König, Angew. Chem., Int. Ed., 2018, 57, 10034–10072 CrossRef CAS.
- C. K. Prier, D. A. Rankic and D. W. C. MacMillan, Chem. Rev., 2013, 113, 5322–5363 CrossRef CAS PubMed.
- F. Strieth-Kalthoff and F. Glorius, Chem, 2020, 6, 1888–1903 CAS.
- V. Alphand, J. H. V. B. Willem, V. Jurkaš, S. Kara, R. Kourist, W. Kroutil, F. Mascia, M. F. Nowaczyk, C. E. Paul, S. Schmidt, J. Spasic, P. Tamagnini and C. K. Winkler, ChemPhotoChem, 2023, 7, e202200325 CrossRef CAS.
- L. Schmermund, V. Jurkas, F. F. Özgen, G. D. Barone, H. C. Büchsenschütz, C. K. Winkler, S. Schmidt, R. Kourist and W. Kroutil, ACS Catal., 2019, 9, 4115–4144 CrossRef CAS.
- R. Amanaka and K. Nakamura, Photobiocatalysis, in Future Directions in Biocatalysis, ed. T. Matsuda, Elsevier, Amsterdam, 2017 Search PubMed.
- S. Zhang, S. Liu, Y. Sun, S. Li, J. Shi and Z. Jiang, Chem. Soc. Rev., 2021, 50, 13449–13466 RSC.
- W. Harrison, X. Huang and H. Zhao, Acc. Chem. Res., 2022, 55, 1087–1096 CrossRef CAS.
- K. Chen and F. H. Arnold, Nat. Catal., 2020, 3, 203–213 CrossRef CAS.
- C. K. Prier and F. H. Arnold, J. Am. Chem. Soc., 2015, 137, 13992–14006 CrossRef CAS.
- E. Meggers, Chem. Commun., 2015, 51, 3290–3301 RSC.
- B. T. Nicholls, D. G. Oblinsky, S. I. Kurtoic, D. Grosheva, Y. Ye, G. D. Scholes and T. K. Hyster, Angew. Chem., Int. Ed., 2022, 61, e202113842 CrossRef CAS PubMed.
- Y. Peng, Z. Chen, J. Xu and Q. Wu, Org. Process Res. Dev., 2022, 26, 1900–1913 CrossRef CAS.
- J. S. Catharina and T. Gulder, ChemBioChem, 2019, 20, 1871–1897 CrossRef.
- S. H. Lee, D. S. Choi, S. K. Kuk and C. B. Park, Angew. Chem., Int. Ed., 2018, 57, 7958–7985 CrossRef CAS.
- M. A. Emmanuel, S. G. Bender, C. Bilodeau, J. M. Carceller, J. S. DeHovitz, H. Fu, Y. Liu, B. T. Nicholls, Y. Ouyang, C. G. Page, T. Qiao, F. C. Raps, D. R. Sorigué, S.-Z. Sun, J. Turek-Herman, Y. Ye, A. Rivas-Souchet, J. Cao and T. K. Hyster, Chem. Rev., 2023, 123(9), 5459–5520 CrossRef CAS.
- J. A. Maciμ-Agulló, A. Corma and H. Garcia, Chem.–Eur. J., 2015, 21, 10940–10959 CrossRef.
-
(a) N. Kosem, M. Watanabe, J. T. Song, A. Takagaki and T. Ishihara, Appl. Catal., A, 2023, 651, 119019 CrossRef CAS;
(b) S. Shi, C. Zeng, T. Si, B. Wang and P. K. Wong, ACS ES&T Engg, 2022, 2(6), 989–1000 Search PubMed.
- C.-H. Yun, J. Kim, F. Hollmann and C. B. Park, Chem. Sci., 2022, 13, 12260 RSC.
-
(a) V. Srivastava, P. K. Singh and P. P. Singh, Croat. Chem. Acta, 2014, 87, 91–95 CrossRef;
(b) V. Srivastava, P. K. Singh and P. P. Singh, Chem. Heterocycl. Compd., 2014, 50, 573–578 CrossRef CAS;
(c) V. Srivastava, P. K. Singh and P. P. Singh, Croat. Chem. Acta, 2015, 88, 59–65 CrossRef CAS;
(d) V. Srivastava, P. K. Singh and P. P. Singh, Croat. Chem. Acta, 2015, 88, 227–233 CrossRef CAS;
(e) V. Srivastava, P. K. Singh and P. P. Singh, Asian J. Chem., 2016, 28, 2159–2163 CrossRef CAS;
(f) V. Srivastava, P. K. Singh and P. P. Singh, Rev. Roum. Chim., 2016, 61, 755–761 Search PubMed;
(g) V. Srivastava, P. K. Singh and P. P. Singh, Croat. Chem. Acta, 2017, 90, 435–441 CrossRef CAS;
(h) V. Srivastava, P. K. Singh, S. Kanaujia and P. P. Singh, New J. Chem., 2018, 42, 688–691 RSC;
(i) P. K. Singh, P. P. Singh and V. Srivastava, Croat. Chem. Acta, 2018, 91, 383–387 CrossRef;
(j) V. Srivastava, P. K. Singh and P. P. Singh, Tetrahedron Lett., 2019, 60, 40–43 CrossRef CAS;
(k) V. Srivastava, P. K. Singh and P. P. Singh, Tetrahedron Lett., 2019, 60, 1333–1336 CrossRef CAS;
(l) V. Srivastava, P. K. Singh and P. P. Singh, Tetrahedron Lett., 2019, 60, 151041 CrossRef CAS;
(m) P. P. Singh and V. Srivastava, ChemistrySelect, 2023, 8(44), e202302732 CrossRef CAS.
-
(a) V. Srivastava, P. K. Singh, A. Srivastava and P. P. Singh, RSC Adv., 2020, 10, 20046–20056 RSC;
(b) V. Srivastava, P. K. Singh and P. P. Singh, Rev. Roum. Chim., 2020, 65, 221–226 CrossRef;
(c) A. Srivastava, P. K. Singh, A. Ali, P. P. Singh and V. Srivastava, RSC Adv., 2020, 10, 39495–39508 RSC;
(d) V. Srivastava, P. K. Singh, A. Srivastava and P. P. Singh, RSC Adv., 2021, 11, 14251–14259 RSC;
(e) V. Srivastava and P. P. Singh, Org. Biomol. Chem., 2021, 19, 313–321 RSC;
(f) P. P. Singh, P. K. Singh, M. Z. Beg, A. Kashyap and V. Srivastava, Synth. Commun., 2021, 51, 3033–3058 CrossRef CAS;
(g) V. Srivastava, P. K. Singh, A. Srivastava, S. Sinha and P. P. Singh, Photochemistry, 2021, 1, 237–276 Search PubMed;
(h) V. Srivastava, P. K. Singh, S. Tivari and P. P. Singh, Org. Chem. Front., 2022, 9, 1485–1507 RSC;
(i) V. Srivastava, P. K. Singh and P. P. Singh, J. Photochem. Photobiol., C, 2022, 50, 100488 CrossRef CAS;
(j) P. P. Singh and V. Srivastava, RSC Adv., 2022, 12, 18245–18265 RSC;
(k) P. P. Singh, G. Pandey, S. Sinha and V. Srivastava, RSC Adv., 2022, 12, 29826 RSC;
(l) S. Tivari, P. K. Singh, P. P. Singh and V. Srivastava, RSC Adv., 2022, 12, 35221 RSC;
(m) M. Mishra, V. Srivastava, P. K. Singh and P. P. Singh, Croat. Chem. Acta, 2022, 95, 25–30 CrossRef CAS;
(n) S. P. Singh, V. Srivastava, P. K. Singh and P. P. Singh, Tetrahedron, 2023, 132, 133245 CrossRef CAS;
(o) M. Z. Beg, P. K. Singh, P. P. Singh, M. Srivastava and V. Srivastava, Mol. Diversity, 2023 DOI:10.1007/s11030-022-10595-2;
(p) V. Srivastava, S. Tivari, P. K. Singh and P. P. Singh, Catal. Lett., 2023 DOI:10.1007/s10562-023-04345-8;
(q) P. P. Singh, P. K. Singh and V. Srivastava, Org. Chem. Front., 2023, 10, 216–236 RSC;
(r) P. P. Singh, J. Singh and V. Srivastava, RSC Adv., 2023, 13, 10958–10986 RSC;
(s) V. P. Singh, A. K. Singh, V. Srivastava and P. P. Singh, Tetrahedron, 2023, 147, 133658 CrossRef CAS.
- S. Shi, C. Zeng, T. Si, B. Wang and P. K. Wong, ACS ES&T Engg, 2022, 2, 989–1000 Search PubMed.
- N. Kosem, M. Watanabe, J. T. Songa, A. Takagakia and T. Ishihara, Appl. Catal., A, 2023, 651, 119019 CrossRef CAS.
- Y. Ding, J. R. Bertram, C. Eckert, R. R. Bommareddy, R. Patel, A. Conradie, S. Bryan and P. Nagpal, J. Am. Chem. Soc., 2019, 141(26), 10272–10282 CrossRef CAS.
- M. Martins, C. Toste and I. A. C. Pereira, Angew. Chem., Int. Ed., 2021, 60(16), 9055–9062 CrossRef CAS PubMed.
- Y. Honda, H. Hagiwara, S. Ida and T. Ishihara, Angew. Chem., 2016, 128(28), 8177–8180 CrossRef.
- B. Wang, C. Zeng, K. H. Chu, D. Wu, H. Y. Yip, L. Ye and P. K. Wong, Adv. Energy Mater., 2017, 7(20), 1700611 CrossRef.
- Z. Jiang, B. Wang, J. C. Yu, J. Wang, T. An, H. Zhao, H. Li, S. Yuan and P. K. Wong, Nano Energy, 2018, 46, 234–240 CrossRef CAS.
- W. Wei, P. Sun, Z. Li, K. Song, W. Su, B. Wang, Y. Liu and J. Zhao, Sci. Adv., 2018, 4(2), eaap9253 CrossRef PubMed.
- B. Ramprakash and A. Incharoensakdi, Bioresour. Technol., 2020, 318, 124057 CrossRef CAS.
- Y. Honda, Y. Shinohara and H. Fujii, Catal. Sci. Technol., 2020, 10(17), 6006–6012 RSC.
- K. Xiao, T. H. Tsang, D. Sun, J. Liang, H. Zhao, Z. Jiang, B. Wang, J. C. Yu and P. K. Wong, Adv. Energy Mater., 2021, 11(21), 2100291 CrossRef CAS.
- H. S. Chan, K. Xiao, T. H. Tsang, C. Zeng, B. Wang, X. Peng and P. K. Wong, Front. Microbiol., 2021, 12, 654033 CrossRef.
- B. Wang, K. Xiao, Z. Jiang, J. Wang, J. C. Yu and P. K. Wong, Energy Environ. Sci., 2019, 12(7), 2185–2191 RSC.
- Z. Wang, D. Gao, H. Geng and C. Xing, J. Mater. Chem. A, 2021, 9(35), 19788–19795 RSC.
- S. F. Rowe, G. L. Gall, E. V. Ainsworth, J. A. Davies, C. W. J. Lockwood, L. Shi, A. Elliston, I. N. Roberts, K. W. Waldron, D. J. Richardson, T. A. Clarke, L. J. C. Jeuken, E. Reisner and J. N. Butt, ACS Catal., 2017, 7(11), 7558–7566 CrossRef CAS.
- H. Shen, Y. Z. Wang, G. Liu, L. Li, R. Xia, B. Luo, J. Wang, D. Suo, W. Shi and Y. C. Yong, ACS Catal., 2020, 10(22), 13290–13295 CrossRef CAS.
- B. Luo, Y. Z. Wang, D. Li, H. Shen, L. X. Xu, Z. Fang, Z. Xia, J. Ren, W. Shi and Y. C. Yong, Adv. Energy Mater., 2021, 11(19), 2100256 CrossRef CAS.
- C. Du, X. Wang, W. Chen, S. Feng, J. Wen and Y. Wu, Mater. Today Adv., 2020, 6, 100071 CrossRef.
- H. Xie, J. Wang, K. Ithisuphalap, G. Wu and Q. Li, J. Energy Chem., 2017, 26(6), 1039–1049 CrossRef.
- F. Li, Y. C. Li, Z. Wang, J. Li, D. H. Nam, Y. Lum, M. Luo, X. Wang, A. Ozden, S. F. Hung, B. Chen, Y. Wang, J. Wicks, Y. Xu, Y. Li, C. M. Gabardo, C. T. Dinh, Y. Wang, T. T. Zhuang, D. Sinton and E. H. Sargent, Nat. Catal., 2020, 3(1), 75–82 CrossRef CAS.
- X. Wang, C. Gao, J. Low, K. Mao, D. Duan, S. Chen, R. Ye, Y. Qiu, J. Ma, X. Zheng, R. Long, X. Wu, L. Song, J. Zhu and Y. Xiong, Sci. Bull., 2021, 66(13), 1296–1304 CrossRef CAS.
- M. B. Ross, P. D. Luna, Y. Li, C. T. Dinh, D. Kim, P. Yang and E. H. Sargent, Nat. Catal., 2019, 2(8), 648–658 CrossRef CAS.
- H. Salehizadeh, N. Yan and R. Farnood, Chem. Eng. J., 2020, 390(15), 124584 CrossRef CAS.
- C. Rudolf and H. Grammel, Enzyme Microb. Technol., 2012, 50(4–5), 238–246 CrossRef CAS PubMed.
- Y. Wang, J. Liu, Y. Wang, A. M. Al-Enizi and G. Zheng, Small, 2017, 13(43), 1701809 CrossRef PubMed.
- K. C. Christoforidis and P. Fornasiero, ChemCatChem, 2019, 11(1), 368–382 CrossRef CAS.
-
(a) Q. Wang, J. Warnan, S. Rodríguez-Jiménez, J. J. Leung, S. Kalathil, V. Andrei, K. Domen and E. Reisner, Nat. Energy, 2020, 5(9), 703–710 CrossRef CAS;
(b) Y. Wang, E. Chen and J. Tang, ACS Catal., 2022, 12, 7300–7316 CrossRef CAS.
- G. Hu, Z. Li, D. Ma, C. Ye, L. Zhang, C. Gao, L. Liu and X. Chen, Nat. Catal., 2021, 4(5), 395–406 CrossRef CAS.
- J. Ye, J. Yu, Y. Zhang, M. Chen, X. Liu, S. Zhou and Z. He, Appl. Catal., B, 2019, 257(15), 117916 CrossRef CAS.
- J. Ye, G. Ren, L. Kang, Y. Zhang, X. Liu, S. Zhou and Z. He, iScience, 2020, 23(7), 101287 CrossRef CAS.
- P. Gai, W. Yu, H. Zhao, R. Qi, F. Li, L. Liu, F. Lv and S. Wang, Angew. Chem., Int. Ed., 2020, 59(18), 7224–7229 CrossRef CAS PubMed.
- K. K. Sakimoto, A. B. Wong and P. Yang, Science, 2016, 351(6268), 74–77 CrossRef CAS PubMed.
- K. K. Sakimoto, S. J. Zhang and P. Yang, Nano Lett., 2016, 16(9), 5883–5887 CrossRef CAS PubMed.
- H. Zhang, H. Liu, Z. Tian, D. Lu and Y. Yu, Nat. Nanotechnol., 2018, 13(10), 900–905 CrossRef CAS.
- S. Cestellos-Blanco, H. Zhang and P. Yang, Faraday Discuss., 2019, 215, 54–65 RSC.
- M. Y. Chen, Z. Fang, L. X. Xu, D. Zhou, X. J. Yang, H. J. Zhu and Y. C. Yong, Bioresour. Bioprocess., 2021, 8, 30 CrossRef.
- B. Wang, Z. Jiang, J. C. Yu, J. Wang and P. K. Wong, Nanoscale, 2019, 11(19), 9296–9301 RSC.
- Y. Su, S. Cestellos-Blanco, J. M. Kim, Y.-x. Shen, Q. Kong, D. Lu, C. Liu, H. Zhang, Y. Cao and P. Yang, Joule, 2020, 4(4), 800–811 CrossRef CAS.
- S. Zhang, Y. Zhang, Y. Chen, D. Yang, S. Li, Y. Wu, Y. Sun, Y. Cheng, J. Shi and Z. Jiang, ACS Catal., 2021, 11, 476–483 CrossRef CAS.
- A. K. Mengele, D. Weixler, S. Amthor, B. J. Eikmanns, G. M. Seibold and S. Rau, Angew. Chem., Int. Ed., 2022, 61, e202114842 CrossRef CAS.
- L. C. P. Gonçalves, H. R. Mansouri, E. L. Bastos, M. Abdellah, B. S. Fadiga, J. S. Rudroff and M. D. Mihovilovic, Catal. Sci. Technol., 2019, 9, 1365 RSC.
- Y. Fu, J. Huang, Y. Wu, X. Liu, F. Zhong and J. Wang, J. Am. Chem. Soc., 2021, 143, 617–622 CrossRef CAS.
- N. Zhang, S. Trépout, H. Chen and M.-H. Li, J. Am. Chem. Soc., 2023, 145, 288–299 CrossRef CAS.
- S. -Y. Hwang, D. Song, E. -J. Seo, F. Hollmann, Y. You and J. -B. Park, Sci. Rep., 2022, 12, 9397 CrossRef CAS PubMed.
- L. Rodríguez-Fernández, J. Albarrán-Velo, I. Lavandera and V. Gotor-Fernández, Adv. Synth. Catal., 2023, 365, 1883–1892 CrossRef.
- Y. Ouyang, J. Turek-Herman, T. Qiao and T. K. Hyster, J. Am. Chem. Soc., 2023, 145, 17018–17022 CrossRef CAS.
- C. G. Page, J. Cao, D. G. Oblinsky, S. N. MacMillan, S. Dahagam, R. M. Lloyd, S. J. Charnock, G. D. Scholes and T. K. Hyster, J. Am. Chem. Soc., 2023, 145, 11866–11874 CrossRef CAS.
- H. Fu, J. Cao, T. Qiao, Y. Qi, S. J. Charnock, S. Garfinkle and T. K. Hyster, Nature, 2022, 610, 302–307 CrossRef CAS PubMed.
-
(a) S. G. Bender and T. K. Hyster, ACS Catal., 2023, 13, 14680–14684 CrossRef CAS;
(b) H. D. Clements, A. R. Flynn, B. T. Nicholls, D. Grosheva, S. J. Lefave, M. T. Merriman, T. K. Hyster and M. S. Sigman, J. Am. Chem. Soc., 2023, 145(32), 17656–17664 CrossRef CAS PubMed;
(c) Y. Ye, J. Cao, D. G. Oblinsky, D. Verma, C. K. Prier, G. D. Scholes and T. K. Hyster, Nat. Chem., 2023, 15, 206–212 CrossRef CAS.
|
This journal is © The Royal Society of Chemistry 2024 |
Click here to see how this site uses Cookies. View our privacy policy here.