DOI:
10.1039/D3RA07000J
(Paper)
RSC Adv., 2024,
14, 2429-2438
Enhancement of the photocatalytic activity of rGO/NiO/Ag nanocomposite for degradation of methylene blue dye
Received
14th October 2023
, Accepted 4th January 2024
First published on 12th January 2024
Abstract
The current study focuses on boosting the photocatalytic ability of reduced graphene oxide (rGO) by decorating the rGO nano-sheets with nickel oxide (NiOx) and silver (Ag) nanomaterials. The developed ternary nanomaterials were investigated using FTIR, XRD, FESEM, TEM, Raman, and UV-vis to evaluate the photo-degradation process. The rGO/NiOx/Ag ternary system showed promising photocatalytic dye degradation under simulated sunlight irradiance. The addition of NiOx and Ag nanomaterials widened the catalytic activity spectrum from the visible region to the UV-region. Besides, these materials hindered the electron–hole recombination, boosting the catalytic activity. The reusability results also clearly showed that the synthesized ternary nanomaterials have good reproducibility and stability for photocatalytic degradation of industrial wastewater.
Introduction
For our life on earth, we all rely on clean water. Regardless, the increasing industrial and residential contaminants that are regularly discharged into natural water supplies pollute the ecosystem. Several research studies have reported that 12% of dyes used in textile industries, including Rose Bengal, Rhodamine B, Methylene Blue (MB), Indigo Red, Caramine, Victoria blue, Red 120, Thymol blue, Eriochrome, Methylene Blue (MB), and Black-T (EBT),1–5 are lost throughout the production and processing actions and end up in wastewater.6 Such dye-polluted wastewater contains non-biodegradable, extremely toxic, and colored pigments which can be toxic and detrimental to living creatures.7,8 This has prompted scholars from all around the world to work together to tackle the problem by developing effective methods to clean or treat the water. Polluted water may be efficiently cleansed by decomposing organic contaminants in a safe and environmentally benign manner utilizing a photocatalytic degradation method.9–13
Due to their low cost, good stability, environmental friendliness, and non-toxicity, semiconductor photocatalysts have sparked a lot of interest in the degradation of organic pollutants over the last decade.14 Semiconductor metal oxide nanoparticles have been proposed to be efficient photocatalysts for photodegradation.15,16 Among the numerous semiconductor metal oxide nanoparticles, nickel oxide (NiOx) nanoparticles with a band gap of 3.6–4.0 eV were chosen as a photocatalyst.17,18 It is an important material in environmental research because of its multiple applications in the breakdown of inorganic contaminants, poisonous dyes, and the biological sector. However, its low light transmittance, coagulation proclivity, and poor dispersibility restrict its efficiency19 limiting its use in a variety of environmental issues. This disadvantage creates opportunities for progress in this field. Because of their outstanding photocatalytic activity, metal oxide, graphene/noble metal/metal oxide, and metal oxide nanohybrids have received a lot of interest. Carbon nanostructures like graphene, fullerenes, carbon nanotubes, and carbon ribbon-based nanocomposites are gaining popularity in photovoltaics, photocatalysis, and energy storage devices.20
The reduced graphene oxide (rGO) is used to serve as a catalyst for a variety of reasons, including its high surface area, strong light absorption, and good electron mobility.21–24 Various studies on connecting rGO to suitable semiconductors have additionally been conducted. The charge transport properties of carbon, as well as its electronic interaction with a semiconductor, enable some novel electrical and photocatalytic uses. The incorporation of well-known metals/metal sulfides/metal oxides catalytic nanomaterials with materials made from graphene results in higher efficiency over the materials in their naked state. In graphene/metal oxide photocatalytic composites, graphene is used as a charge acceptor and transporter, an enhanced photons absorber, and an extensive surface host and support.11,25–27 Because of graphene's electrical conductivity, charge carriers obtained during photoexcitation can be accepted quickly, resulting in less electron–hole pair recombination. The processes such as doping, composite creation, dye sensitization, and noble metal inclusion are favored techniques for activating photocatalytic materials in the visible region.28–33 Surface plasmon resonance (SPR) behaviour has been found to cause the aforementioned properties in Ag or Au anchored ZnO-based heterostructures.30,33
After taking into account all of these parameters, we propose combining all three components (NiOx, rGO, and Ag) to improve photocatalytic activity by lengthening electron–hole pair separation rate and improving visible light area functioning.32,34,35 As a result, a hydrothermal method was used to create a rGO/NiOx hybrid composite. The Ag nanoparticles were coated on rGO/NiOx as rGO/NiOx/Ag and the resulting materials were tested for photocatalytic activity. Through ultraviolet-visible diffuse reflectance (UV-vis) spectroscopy, the basic roles of as-synthesized rGO, rGO/NiOx, and rGO/NiOx/Ag nanocomposites in the photocatalytic process were examined in detail. Under UV light irradiation, the rGO/NiOx/Ag nanocomposite improved photocatalytic activity towards the destruction of methylene blue dye. As a result, the degradation efficiency of methylene blue dye in rGO/NiOx/Ag nanocomposite was 91%, much above that of rGO/NiOx. The higher photocatalytic activity of the rGO/NiOx/Ag composites was mostly owing to improved carrier separation efficiency, according to our findings.
Materials and method
Synthesis of rGO nano-sheets
500 mg of graphite powder (99%, Alfa Aesar) were placed in a beaker followed by addition of 25 mL sulfuric acid (98%, Merck) while stirring at temperature of 2 °C. Then, 1.5 g potassium permanganate (99%, Merck) was poured to the solution at temperature of 10 °C. Then, the graphite solution was stirred at RT for 45 min. After that, 200 mL distilled water (DW) was added into the beaker, followed by extra 2 h ultrasonic treatment. By adding sodium hydroxide (98%, Merck) solution (1 M), the pH of suspension was adjusted at 6, followed by sonication for 1 h. 5 g L-ascorbic acid (99%, Merck) was poured in 50 mL DW and was drop wised to the graphite oxide solution at RT. These solution was stirred at 95 °C for 120 min for reduction of graphite oxide. The obtained precipitates were filtered and washed with DW and a 1 M HCl solution (37%, Merck) to reduce pH to neutral. Finally, the washed precipitates were dried at temperature of 40 °C for 48 h to obtain rGO nano-sheets.
Synthesis of rGO/NiOx hybrid composite
713 mg of nickel nitrate hexahydrate (Ni(NO3)2·6H2O, 99%, Merck) and 360 mg urea (99%, Merck) were dissolved to 6 mL of DW (solution A) with assistance of sonication at RT for 30 min. 300 mg rGO powder was added in 150 mL DW in a beaker and sonicated for 1 h at temperature of 40 °C (solution B). Then, solution B put on a magnetic stirrer and whole of solution A was drop wised to it. The final solution was kept on constant stirring for 2 h at RT. The obtained solution was transferred into a Teflon autoclave, and maintained at 150 °C for 8 h. After that, the resultant was washed with ethanol and DW and dried in an oven at 60 °C overnight. Finally, the obtained powders were annealed at 300 °C for 3 h to product rGO/NiOx composite.
Synthesis of Ag nanoparticles
Silver nanoparticles were synthesized as follow. Typically, 444 mg of polyvinylpyrrolidone (PVP, MW40000, Merck) was poured to 40 mL of polyethylene glycol (PEG, MW600, Merck) and stirred at 80 °C for 4 h. After that, 1 mL of AgNO3 solution in DW (0.5 M) was promptly injected to the above solution, followed by continue stirring at 80 °C to change the color of the solution from colorless to light yellow. The obtained solution was transferred to Teflon autoclave and kept at temperature of 220 °C for 24 h. After cooling down the solution to RT, it was centrifuged for 10 min to separate product from the surfactants and PEG. To further purification, the obtained Ag NPs were dissolved in DW and ethanol and centrifuged several times. Finally, the product was dried at 50 °C overnight.
Synthesis of rGO/NiOx/Ag ternary nanocomposites
The rGO/NiOx/Ag nanocomposite was synthesized via a hydrothermal method. 100 mg rGO/NiOx hybrid composite was dispersed in 50 mL deionized water by sonication at RT for 45 min. 10 mg Ag NPs was dispersed in 50 mL deionized water by sonication at RT for 30 min. The Ag dispersion was added to the rGO/NiOx solution. The slurry suspension liquid was refluxed at 90 °C for 24 h. Next, the prepared colloid was centrifuged and washed by deionized water several times and finally dried at 60 °C overnight. Fig. 1 shows schematic diagram of rGO/NiOx and rGO/NiOx/Ag materials.
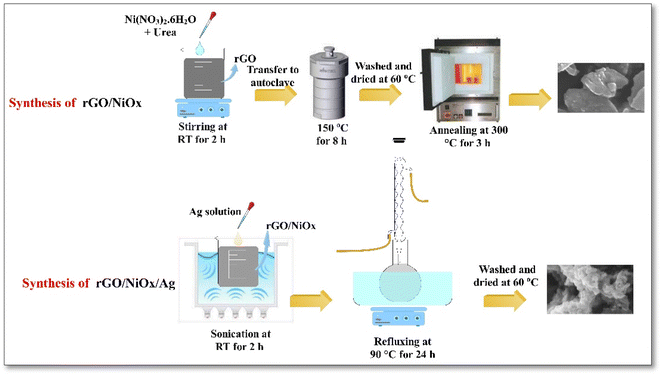 |
| Fig. 1 Schematic diagram for synthesis of rGO/NiOx and rGO/NiOx/Ag materials. | |
Photocatalytic activity of MB
Photocatalytic ability of materials for MB degradation was measured via recording of absorbance spectra trends versus time, while they were irradiated with a simulated sunlight. 50 mg of various nanomaterials (rGO, rGO/NiOx, and rGO/NiOx/Ag) were poured into 100 mL MB dye solution (10 ppm). Then, solutions were kept at dark conditions for 45 min, followed by exposing to illumination and recording UV-vis responses. The photocatalyst stability of rGO/NiOx/Ag material was investigated by running the photocatalytic reaction for 4 different times employing the same catalyst. 50 mg of the rGO/NiOx/Ag catalyst mixed in 100 mL of MB dye solution was centrifuged at 3000 rpm for 5 min after the final reaction. The recovered catalyst was again used for the second time after washing and heating at 75 °C, 6 h. This process was repeated for two more times to complete the reusability MB degradation performance of the rGO/NiOx/Ag system.
Characterization
TEM and FE-SEM images of materials were recorded with Philips BioTwin CM120 and TESCAN MIRA 3 instruments, respectively. Bruker, D8 advance X-ray diffractometer was employed to collect X-ray diffraction (XRD) patterens of materials. Absorbance spectra of samples were investigated with an LINE9600 spectrometer in a wavelength range of 200–800 nm. RAMAN spectra of materials were recorded with a Raman Ram-532-004 device. PL spectra of samples were collected with a PL CARY ECLIPSE spectrophotometer. Bet responses of samples were measured using a BELSORP Mini II device.
Results
Fig. 2a depicts the UV-vis absorption spectra of rGO, Ag-NPs, NiOx-NPs, rGO/NiOx, and rGO/NiOx/Ag nanocomposite. Pure NiOx nanoparticles show absorption in the UV region at 317 nm, where electrons move from the oxygen band to the NiOx valence band.36 Therefore, rGO/NiOx shows a higher absorption intensity than NiOx nanoparticles and pure rGO. When rGO/NiOx/Ag nanocomposite was compared to rGO/NiOx, the absorption edge was red-shifted. The absorption peaks appearing at 264 and 312 nm (Fig. 2a) confirm the formation of rGO/NiOx/Ag nanocomposite. In addition, by incorporation of Ag NPs on the rGO/NiOx surface, a shoulder peak at 400 nm is observed, which correlates to the local surface plasmon resonance (LSPR) of Ag NPs. The presence of Ag NPs on the rGO/NiOx material thanks to their LSPR increases the visible light absorption and assists to increase photocatalytic activity.37,38 The Tauc plot was used to measure synthesized samples' bandgap (Eg).39 Pure rGO has a bandgap of 1.59 eV, which is changed to 2.71 eV after NiOx is added to the rGO lattice (Fig. 2b). After inserting Ag into the rGO/NiOx lattice, the bandgap value of rGO/NiOx dropped to 2.51 eV. The narrow band gap of the rGO/NiOx/Ag nanocomposite connects rGO/NiOx and Ag, which causes Fermi levels between the valence and conduction bands.40 Doping Ag decreases the rGO/NiOx-based photocatalyst's optical bandgap and increases its absorption limit toward visible light.41 These photocatalysts benefit greatly from visible light and can outperform UV-only catalysts in terms of degrading activity.42–44
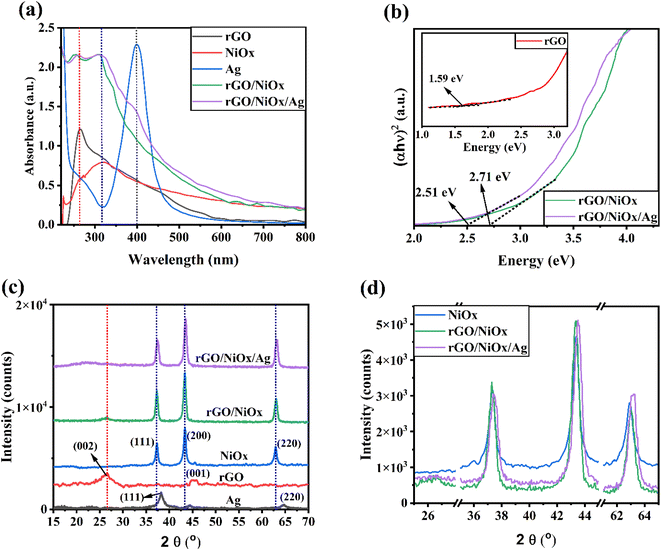 |
| Fig. 2 (a) Absorbance spectra, (b) Tauc, (c) XRD patterns, (d) zoomed view XRD patterns of different materials. | |
The crystal structure of the nanocomposite was characterized by X-ray diffraction study. Fig. 2c depicts the XRD patterns of synthesized Ag-NPs, rGO, NiOx-NPs, rGO/NiOx, and rGO/NiOx/Ag nanocomposites. It was found that the pure NiOx had sharp peaks at 37.20°, 43.42°, and 63.09°. The positions of all the peaks can be indexed to the cubic phase of NiOx (CPDS card no. 73-1519) and correspond too (111), (200), and (220).36 In the case of the rGO/NiOx nanocomposite, a new peak at 26.4° appeared, which corresponds to the decreased GO's (002) crystal plane.45 It is also found that, as the Ag-NPs incorporate, a notable peak shift is evident in the XRD pattern (Fig. 2d), confirming the incorporation of Ag into the crystal lattice of rGO/NiOx.
Fig. 3 depicts the Raman spectra of rGO, rGO/NiOx, and rGO/NiOx/Ag nanocomposites. Peaks for D and G bands were observed in rGO, rGO/NiOx, and rGO/NiOx/Ag nanocomposites, confirming the presence of sp3 hybridised carbon atoms with disordered bonding with oxygen atoms as D band at 1364 cm−1 and ordered sp2 hybridised carbon atoms as G band at 1596 cm−1 relating to A1g symmetry.46,47 Because of the one phonon (1P) mode of 1LO, the NiOx in the rGO/NiOx and rGO/NiOx/Ag nanocomposites displayed a high-intensity Raman peak at 487 cm−1.48 The computed D band and G band intensity ratios for rGO, rGO/NiOx, and rGO/NiOx/Ag nanocomposites were 0.910, 0.869, and 0.823, respectively. The intensity ratio difference can be attributed to the interaction of rGO with NiOx–Ag, implying the formation of the nanocomposite. In addition, the reduced ID/IG ratio in the rGO/NiOx/Ag can be correlated to improved conductivity, which has benefits for photocatalytic activity.
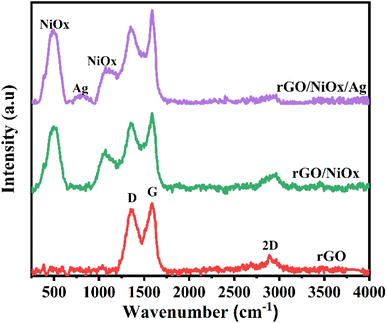 |
| Fig. 3 RAMAN spectra of different materials. | |
The structure and morphology of the Ag-NPs, rGO, NiOx, rGO/NiOx, and rGO/NiOx/Ag nanocomposite were determined by FE-SEM (Fig. 4). As shown in Fig. 4a, the morphology of the Ag-NPs nanoparticles is approximately spherical. rGO imaging with FESEM indicated a flat, 2D substance with a severely wrinkled surface (Fig. 4b). The rGO wrinkles are created by the reduction step, which sticks the graphene sheets together and results in a crumpled shape. This crumpled shape can increase surface area and enable molecule and ion adsorption, making rGO a viable material for application in photocatalytic of industrial wastewaters.49 The surface of NiOx-NPs shows spherical-shaped grains with uniform diameters packed together (Fig. 4c). The NiOx-NPs covered on the reduced graphene oxide are shown in Fig. 4d. The image clearly demonstrates the formation of tiny nanoparticles on the surface of the reduced GO sheets. The NiOx-NPs are practically all the same size, and their dispersion is uniform across the surface of the nanosheets. Improved morphology with a uniform, formed structure with multiple holes is visible for the rGO/NiOx/Ag ternary composites (Fig. 4e).
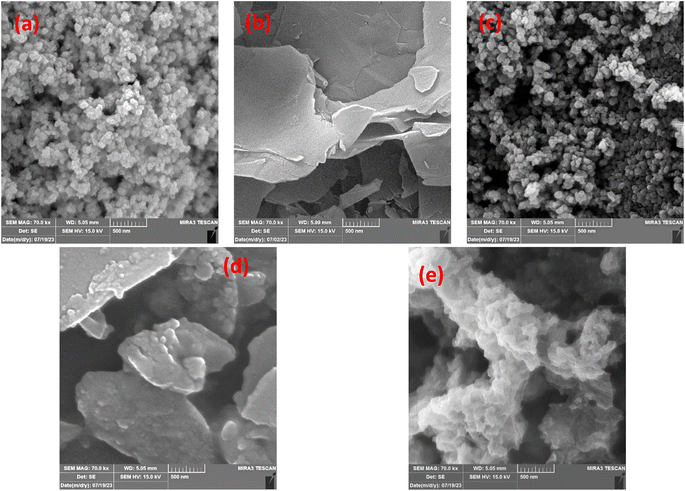 |
| Fig. 4 FE-SEM image of (a) Ag nanoparticles, (b) rGO sheets, (c) NiOx nanoparticles, (d) rGO/NiOx composites, and (e) rGO/NiOx/Ag ternary composites. | |
Fig. 5 shows elemental mapping images for the elements C, O, Ni, and Ag. The mapping study clearly demonstrates that the NiOx–Ag-NPs are evenly dispersed across the entire surface of these rGO sheets.
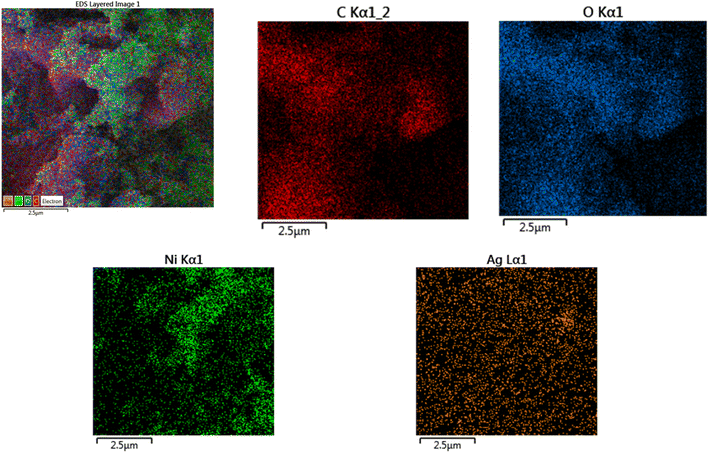 |
| Fig. 5 Mapping FESEM to show elements distribution in rGO/NiOx/Ag ternary composite. | |
Using TEM images, we can gain knowledge about the morphology and form of this nanocomposite (Fig. 6). Fig. 6 shows TEM image of Ag NPs, where they have sizes smaller than 10 nm. The size of Ag NPs from TEM image agrees with the obtained size for them from XRD pattern using Scherrer equation (size of ∼7 nm). Fig. 6b represents the exfoliated rGO sheet, whereas NiOx and Ag nanoparticles, anchored on the rGO nanosheet (Fig. 6c). Small spherical structures of NiOx and Ag attached to rGO sheets appear to be appropriate for avoiding GO photoreduction. The FE-SEM images were validated by the TEM images.
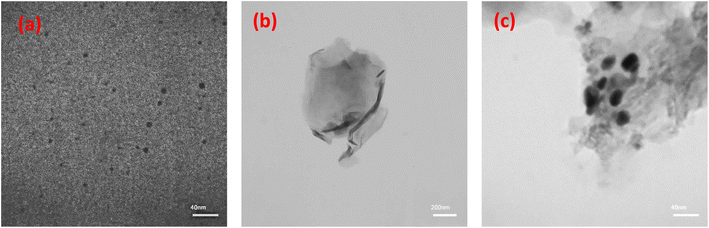 |
| Fig. 6 TEM images of (a) Ag nanoparticles, (b) rGO sheets and (c) rGO/NiO/Ag ternary composites. | |
We focus on the photo-assisted catalytic degradation of commercial methylene blue dye by rGO, rGO/NiOx, and rGO/NiOx/Ag nanocomposite. We expect that this material will show some advantages in phtocatalytic activity to limit the electron–hole recombination rate as well as enhance redox reactions because of its enhanced conductivity, chemical resistance resulting from NiOx and Ag connection with rGO, and suitable band gap for collecting visible photons. Photocatalytic activities of pure rGO, rGO/NiOx, and rGO/NiOx/Ag nanocomposites were assessed through methylene blue dye degradation. Fig. 7a depicts the degradation efficiency of methylene blue dye in different reaction systems. The photodegradation efficiency of methylene blue dye, on the other hand, was just 56% for pure rGO. The quick electron–hole pair recombination may explain rGO's low photocatalytic activity. As expected, rGO/NiOx demonstrated higher photoactivity than pure rGO, demonstrating that the addition of NiOx to rGO promoted charge carrier separation and increased rGO's photocatalytic properties.
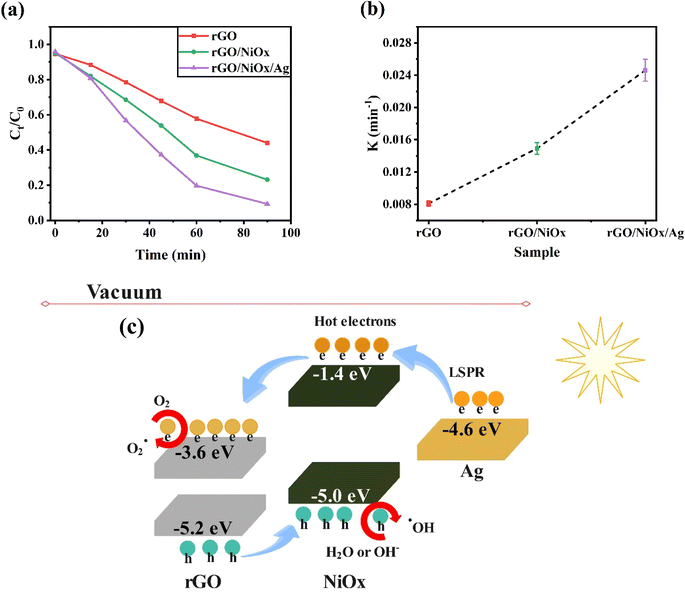 |
| Fig. 7 (a) MB solutions photodegradation efficiency in the presence of different photocatalyst materials. (b) Corresponding photodegradation kinetic rate values of different samples. (c) Possible charge transfer mechanism in rGO/NiOx/Ag photocatalyst during sunlight irradiance and degradation of methylene blue dye. | |
Interestingly, the rGO/NiOx/Ag nanocomposite had the highest degradation efficacy (91%) in degrading methylene blue dye, possibly due to the smallest recombination of photoinduced carriers of charge (Fig. 7a). The findings showed that the nanocomposite synthesized by silver decorating to rGO/NiOx nanocomposite was able to absorb visible light to produce carriers and degrade the methylene blue dye. The measurement of the rate constant findings (Fig. 7b) reveals that the rGO/NiOx/Ag nanocomposite has a high rate constant, which is due to its narrow optical bandgap, robust light absorption, and minimal electron–hole recombination.
The possible photocatalytic mechanism of the rGO/NiOx/Ag sample is depicted in Fig. 7c. By exposing simulated sunlight to the rGO/NiOx/Ag system, the LSPR is induced in Ag NPs, which contributes to form hot electrons in the ternary system. These electrons can be inject into the conduction band of NiOx and then transferred to the rGO surface. Injected electrons react with O2 and generate superoxide radicals
, which later contribute to the generation of hydroxyl radicals (˙OH). On the other hand, the holes from the rGO sheets can transfer to the valence band of NiOx material, which contributes to the oxidization of H2O/OH− to form ˙OH radicals. In continue, these
and ˙OH radicals react with MB dye molecules (RH) to degrade them into non-hazardous compounds.50,51 The reactions involved in the rGO/NiOx/Ag photocatalysis with the presence of MB molecules are given as follows:
The PL (photoluminescence) spectra were used to determine the speed of photo-generated charge recombination in rGO, rGO/NiOx, rGO/NiOx/Ag, and nanocomposite (Fig. 8a). In PL profile of samples there are two main peaks at around 420 and 485 nm, akin to the observed PL peaks material formed during reduction process52 and the PL peak of 420 nm is due to the smaller sp2 fragments.53 The PL intensity of the rGO/NiOx/Ag nanocomposite had been less than the rGO/NiOx nanocomposite and rGO, indicating a lower rate of recombination and more carrier separation. This is due to both Ag-NPs and NiOx-NPs exhibiting excellent electrical properties for the transport of electrons that are photoexcited and may function as acceptors of electrons alongside rGO sheets. The addition of NiOx and Ag to an rGO catalyst has a significant impact on electron–hole pair recombination and favors charge transfer at the interface, boosting the catalyst's stability. Furthermore, the mean pore diameter and specific area of the surface of the rGO, rGO/NiOx, and rGO/NiOx/Ag photocatalysts had been assessed using nitrogen adsorption–desorption curves generated from the BET test (Fig. 8b). The rGO, rGO/NiOx, and rGO/NiOx/Ag nanocomposite showed mean pore diameters of 8.99, 8.55, and 7.03 nm, respectively. In addition, their BET-specific surface area was 35.56, 60.83, and 76.05 m2 g−1, respectively. The obtained findings suggest that a higher specific area of the surface with a narrower pore width in the rGO/NiOx/Ag nanocomposite may produce bigger active sites and mesopores, enabling photon and dye injection. These situations improve the materials' photocatalytic activity.54,55
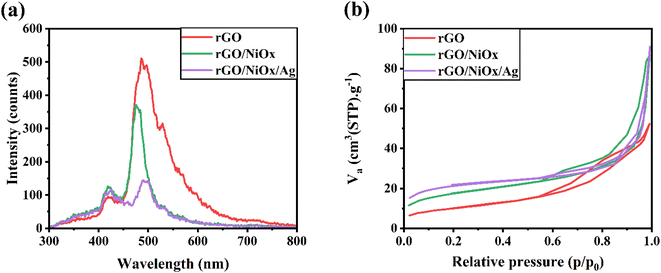 |
| Fig. 8 (a) PL and (b) BET curves of different samples. Excitation wavelength to measure PL spectra was 240 nm. | |
Fig. 9a depicts the methylene blue dye removal effectiveness of rGO/NiOx/Ag ternary composites versus time as the starting methylene blue dye concentration rose from 5 to 20 ppm. With increasing methylene blue dye concentration, the removal effectiveness of the rGO/NiOx/Ag nanocomposite is lowered. In fact, at high methylene blue dye concentrations, the leftover methylene blue dye molecules rise when the absorbent capacity of the catalyst achieves equilibrium. As a result, a smaller number of photons contact the surface of the photocatalyst, leading to poor methylene blue dye photodegradation. The reusability of the ternary rGO/NiOx/Ag material is examined by examining its photocatalytic performance for the MB degradation on four occasions under identical test circumstances (Fig. 9b). After four cycles, the degrading performance of the methylene blue dye dropped from 91% to 79%. The reusability test reveals that the developed ternary nanocomposite can be employed in the industry for long-term photodegradation.
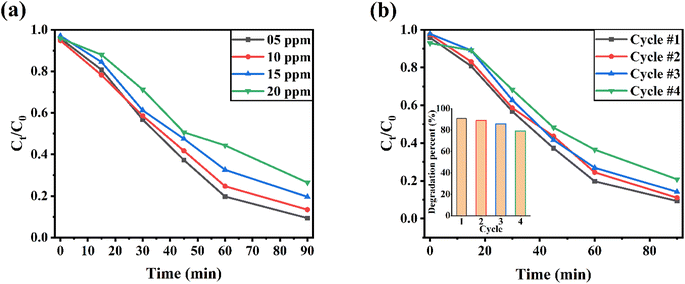 |
| Fig. 9 (a) MB dye photodegradation efficiency with various concentrations in the presence of 50 mg L−1 of rGO/NiOx/Ag ternary composites. (b) The reusability MB degradation performance of rGO/NiOx/Ag system. | |
Conclusion
In conclusion, the addition of NiOx and Ag nanoparticles to rGO improved the photocatalytic degradation of methylene blue dye under simulated sunshine irradiation. The rGO/NiOx/Ag nanocomposite had the greatest photoactivity (95%), which can be attributed to its excellent electron–hole pair separation rate. Furthermore, the k value of the rGO/NiOx/Ag nanocomposite was discovered to be 0.024 min−1, allowing for a threefold increase in photoactivity over rGO. Furthermore, after 4 cycles of repeating, the rGO/NiOx/Ag nanocomposite retained a degradation efficiency greater than 79%, demonstrating its strong stability. This research introduced a novel approach to the design of composite photocatalysts with good charge separation and expanded their applications in environmental remediation.
Data availability
The data that support the findings of this study are available from the corresponding author upon reasonable request.
Conflicts of interest
The authors declare that they have no conflict of interest.
Acknowledgements
The author K. M Batoo would like to thank Researchers Supporting Project No. (RSP2024R148), King Saud University, Riyadh, Saudi Arabia for the financial support.
References
- I. K. Konstantinou and T. A. Albanis, TiO2-assisted photocatalytic degradation of azo dyes in aqueous solution: kinetic and mechanistic investigations: a review, Appl. Catal., B, 2004, 49, 1–14 CrossRef CAS.
- F. Deng, L. Min, X. Luo, S. Wu and S. Luo, Visible-light photocatalytic degradation performances and thermal stability due to the synergetic effect of TiO2 with conductive copolymers of polyaniline and polypyrrole, Nanoscale, 2013, 5, 8703–8710 RSC.
- S. Xu, Y. Zhu, L. Jiang and Y. Dan, Visible light induced photocatalytic degradation of methyl orange by polythiophene/TiO2 composite particles, Water, Air, Soil Pollut., 2010, 213, 151–159 CrossRef CAS.
- D. Wang, Y. Wang, X. Li, Q. Luo, J. An and J. Yue, Sunlight photocatalytic activity of polypyrrole–TiO2 nanocomposites prepared by ‘in situ’method, Catal. Commun., 2008, 9, 1162–1166 CrossRef CAS.
- M. Khanzadeh, M. Dehghanipour, A. Darehkordi and F. Rahmani, Wavelength-dependent nonlinear optical properties of 8-(4-methoxyphenyl)-6-oxo-3-p-tolyl-6H-pyrido [1, 2-b][1, 2, 4] triazine-7, 9-dicarbonitrile, Can. J. Phys., 2018, 96, 1288–1294 CrossRef CAS.
- A. Kumar and G. Pandey, A review on the factors affecting the photocatalytic degradation of hazardous materials, Mater. Sci. Eng. C, 2017, 1, 1–10 Search PubMed.
- M. A. Brown and S. C. De Vito, Predicting azo dye toxicity, Crit. Rev. Environ. Sci. Technol., 1993, 23, 249–324 CrossRef CAS.
- S. Chen, J. Zhang, C. Zhang, Q. Yue, Y. Li and C. Li, Equilibrium and kinetic studies of methyl orange and methyl violet adsorption on activated carbon derived from Phragmites australis, Desalination, 2010, 252, 149–156 CrossRef CAS.
- M. M. Khin, A. S. Nair, V. J. Babu, R. Murugan and S. Ramakrishna, A review on nanomaterials for environmental remediation, Energy Environ. Sci., 2012, 5, 8075–8109 RSC.
- S. Parsons, Advanced Oxidation Processes for Water and Wastewater Treatment, IWA publishing, 2004 Search PubMed.
- J. C. Colmenares and R. Luque, Heterogeneous photocatalytic nanomaterials: prospects and challenges in selective transformations of biomass-derived compounds, Chem. Soc. Rev., 2014, 43, 765–778 RSC.
- R. Rameshbabu, N. Kumar, A. Karthigeyan and B. Neppolian, Visible light photocatalytic activities of ZnFe2O4/ZnO nanoparticles for the degradation of organic pollutants, Mater. Chem. Phys., 2016, 181, 106–115 CrossRef CAS.
- N. Kumar, A. Sen, K. Rajendran, R. Rameshbabu, J. Ragupathi, H. A. Therese and T. Maiyalagan, Morphology and phase tuning of α-and β-MnO2 nanocacti evolved at varying modes of acid count for their well-coordinated energy storage and visible-light-driven photocatalytic behaviour, RSC Adv., 2017, 7, 25041–25053 RSC.
- M. Saleem, J. Iqbal, A. Nawaz, B. Islam and I. Hussain, Synthesis, characterization, and performance evaluation of pristine and cerium-doped WO3 nanoparticles for photodegradation of methylene blue via solar irradiation, Int. J. Appl. Ceram. Technol., 2020, 17, 1918–1929 CrossRef CAS.
- C. Díaz, M. Segovia and M. L. Valenzuela, Solid state nanostructured metal oxides as photocatalysts and their application in pollutant degradation: a review, Photochem, 2022, 2, 609–627 CrossRef.
- G. Gnanamoorthy, V. K. Yadav, K. K. Yadav, K. Ramar, J. Alam, A. K. Shukla, F. A. A. Ali and M. Alhoshan, Fabrication of different SnO2 nanorods for enhanced photocatalytic degradation and antibacterial activity, Environ. Sci. Pollut. Res., 2021, 1–11 Search PubMed.
- A. A. Ezhilarasi, J. J. Vijaya, K. Kaviyarasu, L. J. Kennedy, R. J. Ramalingam and H. A. Al-Lohedan, Green synthesis of NiO nanoparticles using Aegle marmelos leaf extract for the evaluation of in-vitro cytotoxicity, antibacterial and photocatalytic properties, J. Photochem. Photobiol., B, 2018, 180, 39–50 CrossRef PubMed.
- M. A. Rahman, R. Radhakrishnan and R. Gopalakrishnan, Structural, optical, magnetic and antibacterial properties of Nd doped NiO nanoparticles prepared by co-precipitation method, J. Alloys Compd., 2018, 742, 421–429 CrossRef.
- M. Ramesh, H. Nagaraja, M. P. Rao, S. Anandan and N. Huang, Fabrication, characterization and catalytic activity of α-MnO2 nanowires for dye degradation of reactive black 5, Mater. Lett., 2016, 172, 85–89 CrossRef CAS.
- G. Gnanamoorthy, V. K. Yadav, D. Latha, V. Karthikeyan and V. Narayanan, Enhanced photocatalytic performance of ZnSnO3/rGO nanocomposite, Chem. Phys. Lett., 2020, 739, 137050 CrossRef CAS.
- S. Dong, X. Ding, T. Guo, X. Yue, X. Han and J. Sun, Self-assembled hollow sphere shaped Bi2WO6/RGO composites for efficient sunlight-driven photocatalytic degradation of organic pollutants, Chem. Eng. J., 2017, 316, 778–789 CrossRef CAS.
- M. Khanzadeh, M. Dehghanipour, M. Karimipour and M. Molaei, Improvement of nonlinear optical properties of graphene oxide in mixed with Ag2S@ZnS core-shells, Opt. Mater., 2017, 66, 664–670 CrossRef CAS.
- Q. A. Alsulami, A. Rajeh, M. A. Mannaa, S. M. Albukhari and D. F. Baamer, Preparation of highly efficient sunlight driven photodegradation of some organic pollutants and H2 evolution over rGO/FeVO4 nanocomposites, Int. J. Hydrogen Energy, 2021, 46, 27349–27363 CrossRef CAS.
- H. Safajou, M. Ghanbari, O. Amiri, H. Khojasteh, F. Namvar, S. Zinatloo-Ajabshir and M. Salavati-Niasari, Green synthesis and characterization of RGO/Cu nanocomposites as photocatalytic degradation of organic pollutants in waste-water, Int. J. Hydrogen Energy, 2021, 46, 20534–20546 CrossRef CAS.
- D. Deng, K. Novoselov, Q. Fu, N. Zheng, Z. Tian and X. Bao, Catalysis with two-dimensional materials and their heterostructures, Nat. Nanotechnol., 2016, 11, 218–230 CrossRef CAS PubMed.
- M. Faraji, M. Yousefi, S. Yousefzadeh, M. Zirak, N. Naseri, T. H. Jeon, W. Choi and A. Z. Moshfegh, Two-dimensional materials in semiconductor photoelectrocatalytic systems for water splitting, Energy Environ. Sci., 2019, 12, 59–95 RSC.
- G. Gnanamoorthy, V. Karthikeyan, Z. Lu and V. Narayanan, Construction of ZnNiO2/rGO nanocomposites with enhanced photocatalytic performance, Inorg. Chem. Commun., 2023, 156, 111203 CrossRef CAS.
- V. Etacheri, C. Di Valentin, J. Schneider, D. Bahnemann and S. C. Pillai, Visible-light activation of TiO2 photocatalysts: Advances in theory and experiments, J. Photochem. Photobiol. C: Photochem., 2015, 25, 1–29 CrossRef CAS.
- M. Dehghanipour, M. Khanzadeh, M. Karimipour and M. Molaei, Dependence of nonlinear optical properties of Ag2S@ZnS core-shells on Zinc precursor and capping agent, Opt Laser. Technol., 2018, 100, 286–293 CrossRef CAS.
- V. Thinh, V. Lam, T. Bach, N. Van, D. Manh, D. Tung, N. Lien, U. Thuy, T. Anh and N. Tung, Enhanced optical and photocatalytic properties of Au/Ag nanoparticle-decorated ZnO films, J. Electron. Mater., 2020, 49, 2625–2632 CrossRef CAS.
- S. Chidambaram, B. Pari, N. Kasi and S. Muthusamy, ZnO/Ag heterostructures embedded in Fe3O4 nanoparticles for magnetically recoverable photocatalysis, J. Alloys Compd., 2016, 665, 404–410 CrossRef CAS.
- M. Ahmad, E. Ahmed, Z. Hong, N. Khalid, W. Ahmed and A. Elhissi, Graphene–Ag/ZnO nanocomposites as high performance photocatalysts under visible light irradiation, J. Alloys Compd., 2013, 577, 717–727 CrossRef CAS.
- S. Koppala, Y. Xia, L. Zhang, J. Peng, Z. Chen and L. Xu, Hierarchical ZnO/Ag nanocomposites for plasmon-enhanced visible-light photocatalytic performance, Ceram. Int., 2019, 45, 15116–15121 CrossRef CAS.
- M. Kheirabadi, M. Samadi, E. Asadian, Y. Zhou, C. Dong, J. Zhang and A. Z. Moshfegh, Well-designed Ag/ZnO/3D graphene structure for dye removal: Adsorption, photocatalysis and physical separation capabilities, J. Colloid Interface Sci., 2019, 537, 66–78 CrossRef CAS PubMed.
- H. Mu, Y. Gu and H. Xie, Photocatalysis of Nickel-Based Graphene/Au/ZnO Nanocomposites, IEEE Sens. J., 2019, 19, 5376–5388 CAS.
- P. Vivek, R. Sivakumar, E. S. Esakki and S. Deivanayaki, Fabrication of NiO/RGO nanocomposite for enhancing photocatalytic performance through degradation of RhB, J. Phys. Chem. Solids, 2023, 176, 111255 CrossRef CAS.
- S. Lv, Y. Du, F. Wu, Y. Cai and T. Zhou, Review on LSPR assisted photocatalysis: effects of physical fields and opportunities in multifield decoupling, Nanoscale Adv., 2022, 4, 2608–2631 RSC.
- A. M. Golsheikh, N. Huang, H. Lim and R. Zakaria, One-pot sonochemical synthesis of reduced graphene oxide uniformly decorated with ultrafine silver nanoparticles for non-enzymatic detection of H2O2 and optical detection of mercury ions, RSC Adv., 2014, 4, 36401–36411 RSC.
- F. Arjmand, S. J. Fatemi, S. Maghsoudi and A. Naeimi, The first and cost effective nano-biocomposite, zinc porphyrin/CuO/reduced graphene oxide, based on Calotropis procera plant for perovskite solar cell as hole-transport layer under ambient conditions, J. Mater. Res. Technol., 2022, 16, 1008–1020 CrossRef CAS.
- A. C. Jeoffrey, S. J. Ramalingam, K. Murugaiah and A. Balu, One-pot green synthesis of rGO-NiO/CdO nanocomposite materials for the photocatalytic degradation of phenol from waste waters and the study of resistance against Staphylococcus aureus, Hybrid Adv., 2023, 100064 CrossRef.
- R. Vinoth, P. Karthik, K. Devan, B. Neppolian and M. Ashokkumar, TiO2–NiO p–n nanocomposite with enhanced sonophotocatalytic activity under diffused sunlight, Ultrason. Sonochem., 2017, 35, 655–663 CrossRef CAS PubMed.
- F. Kayaci, S. Vempati, C. Ozgit-Akgun, I. Donmez, N. Biyikli and T. Uyar, Selective isolation of the electron or hole in photocatalysis: ZnO–TiO2 and TiO2–ZnO core–shell structured heterojunction nanofibers via electrospinning and atomic layer deposition, Nanoscale, 2014, 6, 5735–5745 RSC.
- S. Ma, J. Xue, Y. Zhou and Z. Zhang, Photochemical synthesis of ZnO/Ag2O heterostructures with enhanced ultraviolet and visible photocatalytic activity, J. Mater. Chem. A, 2014, 2, 7272–7280 RSC.
- M. Deo, D. Shinde, A. Yengantiwar, J. Jog, B. Hannoyer, X. Sauvage, M. More and S. Ogale, Cu2O/ZnO hetero-nanobrush: hierarchical assembly, field emission and photocatalytic properties, J. Mater. Chem., 2012, 22, 17055–17062 RSC.
- T. K. Das, S. K. Ghosh and N. C. Das, Green synthesis of a reduced graphene oxide/silver nanoparticles-based catalyst for degradation of a wide range of organic pollutants, Nano-Struct. Nano-Objects, 2023, 34, 100960 CrossRef CAS.
- H. S. Ahn, J.-W. Jang, M. Seol, J. M. Kim, D.-J. Yun, C. Park, H. Kim, D. H. Youn, J. Y. Kim and G. Park, Self-assembled foam-like graphene networks formed through nucleate boiling, Sci. Rep., 2013, 3, 1396 CrossRef PubMed.
- N. Kumar, J. R. Rodriguez, V. G. Pol and A. Sen, Synergistically advancing Li storage property of hydrothermally grown 1D pristine MnO2 over a mesh-like interconnected framework of 2D graphene oxide, J. Solid State Electrochem., 2019, 23, 1443–1454 CrossRef CAS.
- P. Ravikumar, B. Kisan and A. Perumal, Enhanced room temperature ferromagnetism in antiferromagnetic NiO nanoparticles, AIP Adv., 2015, 5, 087116 CrossRef.
- M. Ikram, A. Raza, M. Imran, A. Ul-Hamid, A. Shahbaz and S. Ali, Hydrothermal synthesis of silver decorated reduced graphene oxide (rGO) nanoflakes with effective photocatalytic activity for wastewater treatment, Nanoscale Res. Lett., 2020, 15, 1–11 CrossRef PubMed.
- M. Kocijan, L. Ćurković, G. Gonçalves and M. Podlogar, The potential of rGO@TiO2 photocatalyst for the degradation of organic pollutants in water, Sustainability, 2022, 14, 12703 CrossRef CAS.
- J. Tian, X. Cao, T. Sun, J. Fan, H. Miao, Z. Chen, D. Li, E. Liu and Y. Zhu, S-scheme Co3(PO4)2/Twinned-Cd0.5Zn0.5S homo-heterojunction for enhanced photocatalytic H2 evolution, Chem. Eng. J., 2023, 471, 144587 CrossRef CAS.
- C. T. Chien, S. S. Li, W. J. Lai, Y. C. Yeh, H. A. Chen, I. S. Chen, L. C. Chen, K. H. Chen, T. Nemoto and S. Isoda, Tunable photoluminescence from graphene oxide, Angew. Chem., Int. Ed., 2012, 51, 6662–6666 CrossRef CAS PubMed.
- G. Eda, Y. Y. Lin, C. Mattevi, H. Yamaguchi, H. A. Chen, I. S. Chen, C. W. Chen and M. Chhowalla, Blue photoluminescence from chemically derived graphene oxide, Adv. Mater., 2010, 22, 505–509 CrossRef CAS PubMed.
- L. Zhang, H. Qi, Y. Zhao, L. Zhong, Y. Zhang, Y. Wang, J. Xue and Y. Li, Au nanoparticle modified three-dimensional network PVA/RGO/TiO2 composite for enhancing visible light photocatalytic performance, Appl. Surf. Sci., 2019, 498, 143855 CrossRef CAS.
- W. Zhang, X. Xiao, L. Zheng and C. Wan, Fabrication of TiO2/MoS2@zeolite photocatalyst and its photocatalytic activity for degradation of methyl orange under visible light, Appl. Surf. Sci., 2015, 358, 468–478 CrossRef CAS.
|
This journal is © The Royal Society of Chemistry 2024 |
Click here to see how this site uses Cookies. View our privacy policy here.