DOI:
10.1039/D4QO00106K
(Research Article)
Org. Chem. Front., 2024,
11, 2494-2501
CO2 and palladium enabled highly chemoselective hydroxylation of gem-difluorocyclopropanes†
Received
18th January 2024
, Accepted 21st February 2024
First published on 21st February 2024
Abstract
The CO2-mediated hydroxylation of gem-difluorocyclopropanes is herein described, under Pd(0) catalysis in the presence of H2O. The method affords a large series of valuable fluorinated cinnamyl alcohols in high yields and with broad functional group tolerance. It is moreover highly chemoselective, as the double C–O coupled ether side-product could be completely suppressed under the CO2 atmosphere. The reaction occurs through Pd-catalyzed C–C and C–F bond activation on the one hand, while CO2 is proposed to activate the weak water nucleophile on the other. This mild synthetic method should impact the fields of medicinal chemistry, organic synthesis, and sustainable processes and advance the concept of CO2 catalysis.
Introduction
Carbon dioxide (CO2) is an increasingly significant part of the atmosphere and is widely accessible, inexpensive, non-toxic, stable, and recyclable.1–5 In many reports, it is mostly utilized as a convenient C1 source.6–10 However, its catalytic activity, in particular in cooperation with transition metal co-catalysts, has received relatively sparse attention.11,12 In the literature, CO2 catalysis mainly focuses on the activation of substrates with high reactivity and strong nucleophilicity, such as alcohols and amines.13–24 Other classes of substrates remain far less explored (Fig. 1A). In most prior methods, CO2 transiently activates the electrophilicity of a substrate through CO2 adducts associated with starkly altered reactivity. Nevertheless, the use of CO2 as a catalyst is still underappreciated for the development of innovative synthetic methods, in particular for the activation of nucleophiles. In the present study, we utilized CO2 catalysis in order to activate one of the weakest and most important nucleophiles in organic synthesis: water (Fig. 1B).25–29
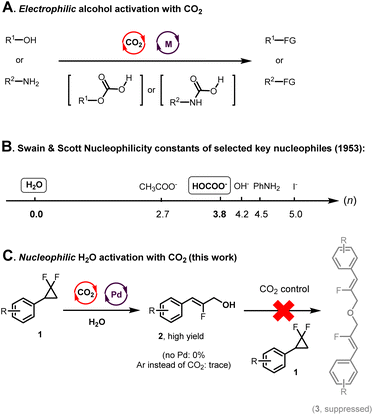 |
| Fig. 1 From CO2-catalyzed electrophilic activation of alcohols towards CO2-catalyzed nucleophilic activation of H2O. | |
The use of H2O as a nucleophile in Pd-catalyzed cross coupling chemistry remains a daunting challenge, in spite of elegant seminal works on the topic.30 Another difficulty resides in the fact that most C–OH coupling products tend to be far more nucleophilic than H2O itself, thus often leading to undesired multiple C–O bond forming escape reactions.31 This is especially the case in the absence of bulky substituents shielding the reaction site.31 We hypothesized that CO2 catalysis might both activate water's nucleophilicity as well as decrease that of the valuable C–OH coupling products.
In this context, we considered gem-difluorocyclopropane electrophiles,32–34 because these are known to readily react with potent nucleophiles under transition metal palladium catalysis.35–55 However, selectively producing hydroxylated products without further reactions constitutes a considerable challenge. We thus designed a method for the hydroxylation of gem-difluorocyclopropanes, which is enabled by CO2 and Pd(0)-catalysis, affording a large series of important fluorinated cinnamyl alcohols56–69 from water. The concept is based on the principle that CO2 might effectively enhance the poor nucleophilicity of water (n = 0) by generating far more nucleophilic transient carbonates (n = 3.8, Fig. 1B).25
Results and discussion
Based on literature precedents,70–72 we conceived that the Pd(0) active species would first perform the oxidative addition into the strained C–C bond of substrate 1 leading to strained palladacycle intermediate Int-I (Fig. 2). This would be followed by β-fluoride elimination,73 leading to fluoroallyl palladium species Int-II. Fluoride-carbonate exchange would then lead to Int-III, followed by C–O reductive elimination towards carbonated product Int-IV and the regenerated Pd(0) catalyst (Cycle A). The former would then release CO2 towards the final fluoroallyl alcohol product 2. The released CO2 would then reform the active carbonate intermediate (KHCO3) in the presence of excess water and K3PO4 base, thus closing the CO2 catalytic cycle (Cycle B).
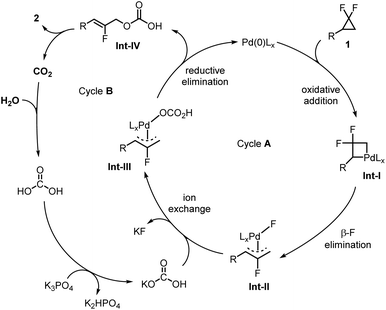 |
| Fig. 2 Proposed mechanism. | |
We therefore initially selected 4-methyl-gem-difluoro-cyclopropane (1a) as a model substrate. Based on a previous report from our group,70 we serendipitously realized that 1a produces fluoroallyl alcohol product 2a in high isolated yield (93%) when exposed to Pd(dba)2 (10 mol%), Xphos (20 mol%), water (10 equiv.), and K3PO4 (3 equiv.) in DMF under CO2 atmosphere (1 atm) at 80 °C for 12 h (Table 1, entry 1). The double C–O bond forming allyl ether byproduct 3a was not detected under those conditions. Interestingly, utilizing only 10 mol% of Xphos reduced the yield to only 70% (entry 2), presumably due to competing ligand exchange processes. This reaction cannot occur without a palladium precursor (entry 3). Moreover, omitting the addition of water severely reduced the yield (2a, 32%, entry 4), suggesting it to be a main hydroxyl source of the reaction. The residual product formation is likely due to traces of water in the other components of the reaction. Likewise, omitting the phosphate base is detrimental to the reaction's efficiency (2a, 30%, entry 5). Importantly, CO2 was found to be essential. Replacing it with an atmosphere of N2 inert gas almost shuts down the reaction (2a, 6%, entry 6), while air did not provide any desired product (entry 7). DMF proved to be an optimal solvent, in contrast for example to DMSO (entries 8–10). Finally, CO2 could not be replaced by any additives that we tried, whether Lewis or Brønsted acids, or other (entry 11).
Table 1 Reaction conditionsa
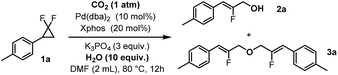
|
Entry |
Deviation from standard conditionsa |
2a
(%) |
3a
(%) |
1a (0.20 mmol), Pd catalyst precursor (10 mol%), Xphos ligand (20 mol%), K3PO4 (3 equiv.), H2O (10 equiv.), in DMF (2.0 mL) at 80 °C for 12 h.
Determined by 1H NMR, using 1,3,5-trimethoxybenzene as an internal standard.
Isolated yield.
Selected additives: Ag2O, Cu(OAc)2, NH4Cl, PhCO2H, EtCO2H, under N2.
|
1
|
None
|
98 (93)c
|
nd
|
2 |
Only 10 mol% Xphos |
70 |
nd |
3 |
No Pd |
nd |
nd |
4 |
No H2O |
32 |
nd |
5 |
No K3PO4 |
30 |
nd |
6 |
N2 instead of CO2 |
6 |
<5 |
7 |
Air instead of CO2 |
nd |
nd |
8 |
H2O instead of DMF |
8 |
<5 |
9 |
Toluene instead of DMF |
10 |
nd |
10 |
DMSO instead of DMF |
15 |
nd |
11 |
Additivesd instead of CO2 |
nd |
nd |
With the optimized conditions in hand, we then investigated the reaction scope with various gem-difluorocyclopropanes. Thus, we tested both electron-donating and withdrawing substituents at para-, meta- and ortho-positions of the arene substituent, providing the corresponding fluoro-cinnamyl alcohols in usually excellent isolated yields (2a–2zc, Fig. 3). The functional group tolerance was found outstanding for both electron-poor (R1 = CF3) and electron-rich substrates (R1 = alkyl, O-alkyl/aryl, N-alkyl), with the notable exception of halides (R1 = Cl, 2e). Various heterocycles were moreover very well accommodated such as benzofurane (2x), carbazole (2y) and benzodioxane (2w). Finally, a series of biologically active fragments such as DL-menthol (2za), DL-isoborneol (2zb) and a protected fructopyranose derivative (2zc) could all be tolerated in high yields (79–92%). Unfortunately, however, the alkyl-substituted gem-difluorocyclopropane corresponding to target product 2zd was recovered unreacted, indicating the importance of the aromatic substituent for the strained ring opening step. It should furthermore be noted that we also achieved the hydroxylation reaction of a structurally related geminal difluoroallyl derivative (4), which yielded the corresponding cinnamaldehyde (5, Fig. 3). There too, CO2 was found to be essential, as its replacement with an N2 atmosphere almost shuts down the reaction (Fig. 3). These results confirm the critical water activating role of CO2.
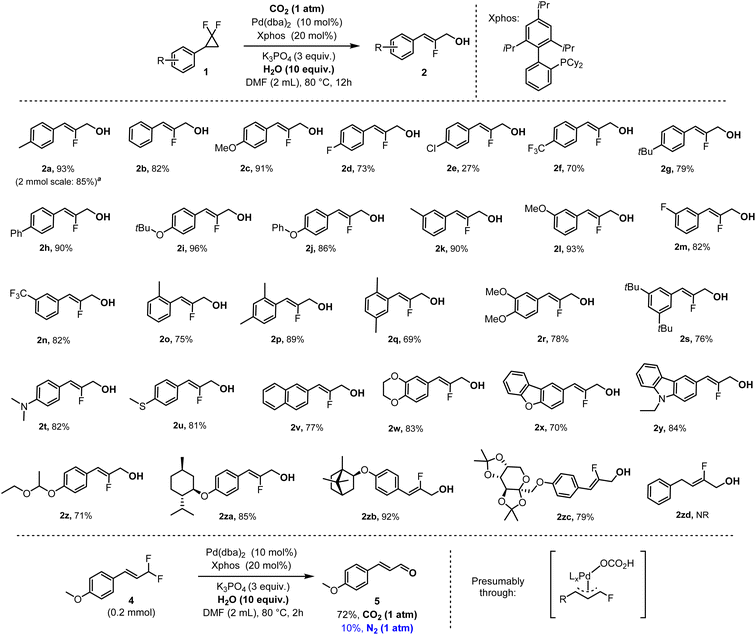 |
| Fig. 3 Substrate scope, isolated yields. Reaction conditions: 1a (0.20 mmol), Pd catalyst precursor (10 mol%), Xphos ligand (20 mol%), K3PO4 (3 equiv.), H2O (10 equiv.), in DMF (2.0 mL) at 80 °C for 12 h. a Large scale (2 mmol): performed in optimized conditions, in 10 mL DMF. | |
Next, we investigated whether this reaction could truly proceed under a catalytic loading of CO2. We therefore replaced CO2 with catalytic amounts of phenyl isocyanate, which undergoes hydrolysis under the reaction conditions towards the corresponding amine and CO2, a process known as the Hofmann degradation.71 This allowed us to carry out the reaction under precise catalytic loading of CO2 precursor. There too, considerably higher product yields were obtained with only 20 mol% isocyanate loading (2a, 58%, Fig. 4, eqn (1)), compared to the complete absence of CO2 or precursor thereof (2a, 6%, Table 1, entry 6). Fluoroallyl amine product 6a was also observed, however typically as a minor byproduct. These findings are in line with the proposed catalytic role of CO2 in this reaction. In order to further investigate this matter, we then attempted the reaction in the absence of CO2, under N2 inert gas, however in the presence of sur-stoichiometric carbonate salts (Fig. 4, eqn (2)). While the yields are nowhere near optimized conditions with CO2, at 24–40% depending on the utilized salt, these are still significantly greater than in the absence of both CO2 and carbonates (6%, Table 1, entry 6). These experiments therefore confirm that transient carbonates are likely essential intermediates in this reaction. However, clearly, CO2 still outperforms the herein investigated carbonate salts in terms of product yield, suggesting additional not yet well identified enhancing effects of carbon dioxide in the present reaction conditions. It should moreover be noted that the sur-stoichiometric carbonate salt experiment performs less well when water addition is omitted (25% compared to 40%, Fig. 4, eqn (3)), suggesting a potential cooperative role of water with the carbonate intermediate.
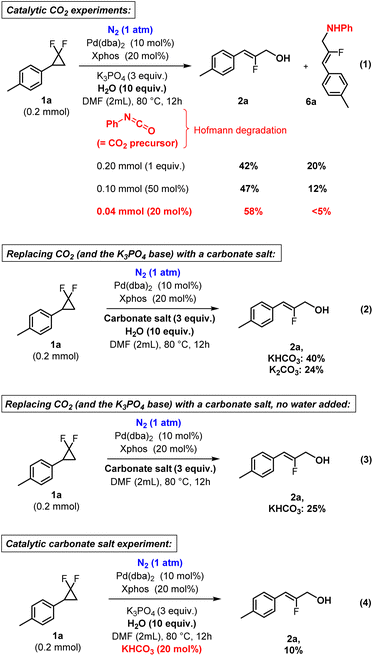 |
| Fig. 4 Mechanistic experiments regarding the catalytic role of CO2 and the intermediacy of carbonate salts, yields determined by 1H NMR using 1,3,5-trimethoxybenzene as an internal standard. | |
In order to further investigate the apparent superiority of CO2 mediation versus carbonate mediation, we performed a final key experiment with catalytic loading of a carbonate salt (20 mol%) under inert N2 gas, in otherwise standard conditions (Fig. 4, eqn (4)). This led to a severely decreased product yield (2a, 10%), thus unambiguously confirming the supposed superiority of CO2 over carbonates, at least in the current reaction conditions. At this stage, we cannot exclude that a method based solely on carbonate salts, without added CO2, might eventually also furnish a competent hydroxylation method, especially upon further optimization. However, this remains outside of the scope of this study.
Next, we engaged the reaction with 18O-labelled water (18O label: 97%), in otherwise standard reaction conditions (Fig. 5, eqn (1)). The significant 18O-incorporation into the product (see ESI†) is consistent with water being a hydroxyl source. Interestingly, however, the 18O incorporation is slightly under one third from that of the labelled H2(18O) reagent, indicating label scrambling with the non-labelled oxygen atoms of CO2. This is very much consistent with a carbonate intermediate, wherein each of the three resulting oxygen atoms has an equal chance of forming the C–O bond during the reductive elimination event at the Pd(II) center. When employing a catalytic amount of CO2, however, the 18O content in the product increased dramatically (18O: 77% incorporation, Fig. 5, eqn (2)). This is due to the reduced amount of (non-labelled) CO2, thus limiting the overall label scrambling within the carbonate intermediate. Both 18O label experiments (eqn (1) & (2)) are thus in excellent agreement with the presumed catalytic role of CO2 in this reaction.
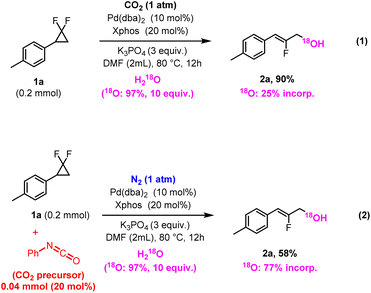 |
| Fig. 5
18O label experiments, isolated yields. | |
In order to further explore the synthetic utility of the method, a gram scale reaction was conducted for product 2a. This target was thus obtained in remarkably preserved 85% isolated yield (2 mmol scale, product 2a, 0.282 g, Fig. 3). Next, we attempted to force the reaction conditions towards the formation of double C–O coupling product 3a, in stepwise fashion (Fig. 6). This was carried out under both inert atmosphere (eqn (1)) as well as under CO2 (eqn (2)). From these experiments, it can be deduced that CO2 prevents the formation of double C–O coupling product 3a, presumably through transient carbonate ester protection, in contrast to N2. Increasing the temperature further to 110 °C under N2 atmosphere, along conditions previously reported by us for amines,70 afforded the double C–O coupling product 3a in 25% yield (Fig. 6, eqn (3)). Other side products could not be formally identified in these reaction mixtures (Fig. 6).
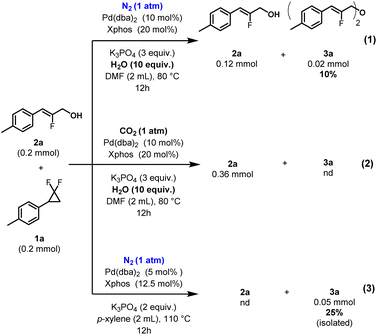 |
| Fig. 6 Pushing towards the ether product 3a. Unless otherwise stated, yields determined by 1H NMR using 1,3,5-trimethoxybenzene as an internal standard. | |
Finally, we performed a competition experiment between a potent aniline nucleophile (n = 4.5),25 and poorly nucleophilic water (n = 0),25 under perfectly identical conditions, either with or without CO2 atmosphere (Fig. 7). Interestingly, under N2 inert gas, aniline considerably outperforms water (6a
:
2a > 15
:
1). Under CO2 atmosphere, however, water becomes a much more competitive coupling partner compared to aniline (6a
:
2a ≈ 1
:
1), which is again consistent with the proposed water activation scenario of CO2.
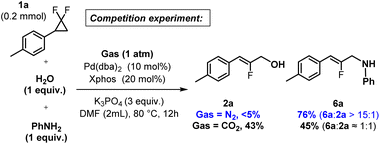 |
| Fig. 7 Nucleophile competition experiment: H2O versus aniline, with and without CO2, yields determined by 1H NMR using 1,3,5-trimethoxybenzene as an internal standard. | |
Conclusions
In conclusion, we developed a CO2-mediated, Pd(0) catalyzed method for the hydroxylation of gem-difluorocyclopropanes from water, affording a large series of valuable fluorinated cinnamyl alcohols in high yields and with broad functional group tolerance, including important bioactive scaffolds. Moreover, we characterized the nucleophile activating role of CO2 through key mechanistic experiments. The herein presented results should encourage the development of further challenging coupling reactions with weak X–H nucleophiles such as water, by means of CO2 catalysis,74–77 and in general the use of CO2 in synthetic method development.78
Conflicts of interest
There are no conflicts to declare.
Acknowledgements
DFG-funded Transregional Collaborative Research Center SFB/TRR 88 “Cooperative effects in homo and heterometallic complexes” (https://3MET.de), and the CSC (XW and FX, grants no. 202008330337 and 202208430011, respectively) are acknowledged for generous financial support.
Notes and references
- J. Artz, T. E. Müller, K. Thenert, J. Kleinekorte, R. Meys, A. Sternberg, A. Bardow and W. Leitner, Sustainable Conversion of Carbon Dioxide: An Integrated Review of Catalysis and Life Cycle Assessment, Chem. Rev., 2018, 118, 434 CrossRef CAS PubMed.
- Y. Yao, K. Ivanovski, J. Inekwe and R. Smyth, Human capital and CO2 emissions in the long run, Energy Econ., 2020, 91, 104907 CrossRef.
- K. Huang, C. L. Sun and Z. J. Shi, Transition-metal-catalyzed C–C bond formation through the fixation of carbon dioxide, Chem. Soc. Rev., 2011, 40, 2435 RSC.
- M. D. Burkart, N. Hazari, C. L. Tway and E. L. Zeitler, Opportunities and Challenges for Catalysis in Carbon Dioxide Utilization, ACS Catal., 2019, 9, 7937 CrossRef CAS.
- J.-H. Ye, T. Ju, H. Huang, L.-L. Liao and D.-G. Yu, Radical Carboxylative Cyclizations and Carboxylations with CO2, Acc. Chem. Res., 2021, 54, 2518 CrossRef CAS PubMed.
- Z. Zhang, J.-H. Ye, T. Ju, L.-L. Liao, H. Huang, Y.-Y. Gui, W.-J. Zhou and D.-G. Yu, Visible-Light-Driven Catalytic Reductive Carboxylation with CO2, ACS Catal., 2020, 10, 10871 CrossRef CAS.
- Y. Zhang, T. Zhang and S. Das, Catalytic transformation of CO2 into C1 chemicals using hydrosilanes as a reducing agent, Green Chem., 2020, 22, 1800 RSC.
- B. Limburg, À. Cristòfol, F. Della Monica and A. W. Kleij, Unlocking the Potential of Substrate-Directed CO2 Activation and Conversion: Pushing the Boundaries of Catalytic Cyclic Carbonate and Carbamate Formation, ChemSusChem, 2020, 13, 6056 CrossRef CAS PubMed.
- Q. Liu, L. Wu, R. Jackstell and M. Beller, Using carbon dioxide as a building block in organic synthesis, Nat. Commun., 2015, 6, 5933 CrossRef PubMed.
- M. Aresta, A. Dibenedetto and A. Angelini, Catalysis for the Valorization of Exhaust Carbon: from CO2 to Chemicals, Materials, and Fuels. Technological Use of CO2, Chem. Rev., 2014, 114, 1709 CrossRef CAS PubMed.
- P. K. Sahoo, Y. Zhang and S. Das, CO2-Promoted Reactions: An Emerging Concept for the Synthesis of Fine Chemicals and Pharmaceuticals, ACS Catal., 2021, 11, 3414 CrossRef CAS.
- C. Park and J. Lee, Recent achievements in CO2-assisted and CO2-catalyzed biomass conversion reactions, Green Chem., 2020, 22, 2628 RSC.
- M. Sakamoto, I. Shimizu and A. Yamamoto, Activation of C–O and C–N Bonds in Allylic Alcohols and Amines by Palladium Complexes Promoted by CO2. Synthetic Applications to Allylation of Nucleophiles, Carbonylation, and Allylamine Disproportionation, Bull. Chem. Soc. Jpn., 1996, 69, 1065 CrossRef CAS.
- S. Minakata, Y. Yoneda, Y. Oderaotoshi and M. Komatsu, Unprecedented CO2-Promoted Aminochlorination of Olefins with Chloramine-T, Org. Lett., 2006, 8, 967 CrossRef CAS PubMed.
- S. B. Lang, T. M. Locascio and J. A. Tunge, Activation of Alcohols with Carbon Dioxide: Intermolecular Allylation of Weakly Acidic Pronucleophiles, Org. Lett., 2014, 16, 4308 CrossRef CAS PubMed.
- Y. Wang, J. Zhang, J. Liu, C. Zhang, Z. Zhang, J. Xu, S. Xu, F. Wang and F. Wang, C-N and N-H Bond Metathesis Reactions Mediated by Carbon Dioxide, ChemSusChem, 2015, 8, 2066 CrossRef CAS PubMed.
- P. Hirapara, D. Riemer, N. Hazra, J. Gajera, M. Finger and S. Das, CO2-assisted synthesis of non-symmetric α-diketones directly from aldehydes via C–C bond formation, Green Chem., 2017, 19, 5356 RSC.
- M. Kapoor, P. Chand-Thakuri and M. C. Young, Carbon Dioxide-Mediated C(sp2)–H Arylation of Primary and Secondary Benzylamines, J. Am. Chem. Soc., 2019, 141, 7980 CrossRef CAS PubMed.
- J. Ye, I. Kalvet, F. Schoenebeck and T. Rovis, Direct α-alkylation of primary aliphatic amines enabled by CO2 and electrostatics, Nat. Chem., 2018, 10, 1037 CrossRef CAS PubMed.
- G. Pupo, R. Properzi and B. List, Asymmetric Catalysis with CO2: The Direct α-Allylation of Ketones, Angew. Chem., Int. Ed., 2016, 55, 6099 CrossRef CAS PubMed.
- D. Riemer, B. Mandaviya, W. Schilling, A. C. Götz, T. Kühl, M. Finger and S. Das, CO2-Catalyzed Oxidation of Benzylic and Allylic Alcohols with DMSO, ACS Catal., 2018, 8, 3030 CrossRef CAS.
- M. Kapoor, D. Liu and M. C. Young, Carbon Dioxide-Mediated C(sp3)–H Arylation of Amine Substrates, J. Am. Chem. Soc., 2018, 140, 6818 CrossRef CAS PubMed.
- T. Roy, M. J. Kim, Y. Yang, S. Kim, G. Kang, X. Ren, A. Kadziola, H. Y. Lee, M. H. Baik and J. W. Lee, Carbon Dioxide-Catalyzed Stereoselective Cyanation Reaction, ACS Catal., 2019, 9, 6006 CrossRef CAS.
- Y. Yang, J. Liu, F. S. Kamounah, G. Ciancaleoni and J.-W. Lee, A CO2-Catalyzed Transamidation Reaction, J. Org. Chem., 2021, 86, 16867 CrossRef CAS PubMed.
- C. G. Swain and C. B. Scott, Quantitative Correlation of Relative Rates. Comparison of Hydroxide Ion with Other Nucleophilic Reagents toward Alkyl Halides, Esters, Epoxides and Acyl Halides, J. Am. Chem. Soc., 1953, 75, 141 CrossRef CAS.
- H. Mayr, T. Bug, M. F. Gotta, N. Hering, B. Irrgang, B. Janker, B. Kempf, R. Loos, A. R. Ofial, G. Remennikov and H. Schimmel, Reference Scales for the Characterization of Cationic Electrophiles and Neutral Nucleophiles, J. Am. Chem. Soc., 2001, 123, 9500 CrossRef CAS PubMed.
- R. Lucius, R. Loos and H. Mayr, Kinetic Studies of Carbocation–Carbanion Combinations: Key to a General Concept of Polar Organic Reactivity, Angew. Chem., Int. Ed., 2002, 41, 91 CrossRef CAS.
- H. Mayr and A. R. Ofial, Kinetics of electrophile-nucleophile combinations: A general approach to polar organic reactivity, Pure Appl. Chem., 2005, 77, 1807 CrossRef CAS.
- H. Mayr and A. R. Ofial, Do general nucleophilicity scales exist?, J. Phys. Org. Chem., 2008, 21, 584 CrossRef CAS.
- S. Enthaler and A. Company, Palladium-catalysed hydroxylation and alkoxylation, Chem. Soc. Rev., 2011, 40, 4912 RSC.
- For an early representative example see: K. W. Anderson, T. Ikawa, R. E. Tundel and S. L. Buchwald, The Selective Reaction of Aryl Halides with KOH: Synthesis of Phenols, Aromatic Ethers, and Benzofurans, J. Am. Chem. Soc., 2006, 128, 10694 CrossRef CAS PubMed.
- D. Seyferth, H. Dertouzos, R. Suzuki and J. Y. P. Mui, Halomethyl-metal compounds. XIII. Preparation of gem-difluorocyclopropanes by iodide ion-induced CF2 transfer from trimethyl(trifluoromethyl)tin, J. Org. Chem., 1967, 32, 2980 CrossRef CAS.
- A. P. Thankachan, K. S. Sindhu, K. K. Krishnan and G. Anilkumar, Recent advances in the syntheses, transformations and applications of 1,1-dihalocyclopropanes, Org. Biomol. Chem., 2015, 13, 8780 RSC.
-
D. M. Volochnyuk and O. O. Grygorenko, in Emerging Fluorinated Motifs: Synthesis, Properties, and Applications, ed. D. Cahard and J.-A. Ma, Wiley, 2020, vol. 1, p. 135 Search PubMed.
- J. Xu, E. A. Ahmed, B. Xiao, Q. Q. Lu, Y. L. Wang, C. G. Yu and Y. Fu, Pd-Catalyzed Regioselective Activation of gem-Difluorinated Cyclopropanes: A Highly Efficient Approach to 2-Fluorinated Allylic Scaffolds, Angew. Chem., Int. Ed., 2015, 54, 8231 CrossRef CAS PubMed.
- L. Lv, H. Qian, Y. Ma, S. Huang, X. Yan and Z. Li, Ligand-controlled regioselective and chemodivergent defluorinative functionalization of gem-difluorocyclopropanes with simple ketones, Chem. Sci., 2021, 12, 15511 RSC.
- J. Wenz, C. A. Rettenmeier, H. Wadepohl and L. H. Gade, Catalytic C–F bond activation of geminal difluorocyclopropanes by nickel(I) complexes via a radical mechanism, Chem. Commun., 2016, 52, 202 RSC.
- E. A. M. A. Ahmed, A. M. Y. Suliman, T. J. Gong and Y. Fu, Palladium-Catalyzed Stereoselective Defluorination Arylation/Alkenylation/Alkylation of gem-Difluorinated Cyclopropanes, Org. Lett., 2019, 21, 5645 CrossRef CAS PubMed.
- E. A. M. A. Ahmed, A. M. Y. Suliman, T. J. Gong and Y. Fu, Access to Divergent Fluorinated Enynes and Arenes via Palladium-Catalyzed Ring-Opening Alkynylation of gem-Difluorinated Cyclopropanes, Org. Lett., 2020, 22, 1414 CrossRef CAS PubMed.
- A. M. Y. Suliman, E. A. M. A. Ahmed, T. J. Gong and Y. Fu, Cu/Pd-Catalyzed cis-Borylfluoroallylation of Alkynes for the Synthesis of Boryl-Substituted Monofluoroalkenes, Org. Lett., 2021, 23, 3259 CrossRef CAS PubMed.
- P. X. Zhou, X. Yang, J. Wang, C. Ge, W. Feng, Y. M. Liang and Y. Zhang, Palladium-Catalyzed C–H Allylation of Electron-Deficient Polyfluoroarenes with gem-Difluorinated Cyclopropanes, Org. Lett., 2021, 23, 4920 CrossRef CAS PubMed.
- A. M. Y. Suliman, E. A. M. A. Ahmed, T. J. Gong and Y. Fu, Three-component reaction of gem-difluorinated cyclopropanes with alkenes and B2pin2 for the synthesis of monofluoroalkenes, Chem. Commun., 2021, 57, 6400 RSC.
- L. Lv and C. J. Li, Palladium-Catalyzed Defluorinative Alkylation of gem-Difluorocyclopropanes: Switching Regioselectivity via Simple Hydrazones, Angew. Chem., Int. Ed., 2021, 60, 13098 CrossRef CAS PubMed.
- Z. Fu, J. Zhu, S. Guo and A. Lin, Palladium-catalyzed allylic alkylation dearomatization of β-naphthols and indoles with gem-difluorinated cyclopropanes, Chem. Commun., 2021, 57, 1262 RSC.
- B. Xiong, X. Chen, J. Liu, X. Zhang, Y. Xia and Z. Lian, Stereoselective gem-Difluorovinylation of gem-Difluorinated Cyclopropanes Enabled by Ni/Pd Cooperative Catalysis, ACS Catal., 2021, 11, 11960 CrossRef CAS.
- Z. T. Jiang, J. Huang, Y. Zeng, F. Hu and Y. Xia, Rhodium Catalyzed Regioselective C–H Allylation of Simple Arenes via C–C Bond Activation of Gem-difluorinated Cyclopropanes, Angew. Chem., Int. Ed., 2021, 60, 10626 CrossRef CAS PubMed.
- L. Lv, H. Qian, A. B. Crowell, S. Chen and Z. Li, Pd/NHC-Controlled Regiodivergent Defluorinative Allylation of gem-Difluorocyclopropanes with Allylboronates, ACS Catal., 2022, 12, 6495 CrossRef CAS.
- Y. Zeng, H. Gao, Y. Zhu, Z.-T. Jiang, G. Lu and Y. Xia, Site-Divergent Alkenyl C–H Fluoroallylation of Olefins Enabled by Tunable Rhodium Catalysis, ACS Catal., 2022, 12, 8857 CrossRef CAS.
- Y. Ai, H. Yang, C. Duan, X. Li and S. Yu, Cobalt-Catalyzed Fluoroallyllation of Carbonyls via C–C Activation of gem-Difluorocyclopropanes, Org. Lett., 2022, 24, 5051 CrossRef CAS PubMed.
- Z.-T. Jiang, Z. Chen, Y. Zeng, J.-L. Shi and Y. Xia, Enantioselective Formation of All-Carbon Quaternary Stereocenters in gem-Difluorinated Cyclopropanes via Rhodium-Catalyzed Stereoablative Kinetic Resolution, Org. Lett., 2022, 24, 6176 CrossRef CAS PubMed.
- Y. Zeng, H. Yang, J. Du, Q. Huang, G. Huang and Y. Xia, Rh-catalyzed regio-switchable cross-coupling of gem-difluorinated cyclopropanes with allylboronates to structurally diverse fluorinated dienes, Chem. Sci., 2022, 13, 12419 RSC.
- X. Wu, Y. Zeng, Z.-T. Jiang, Y. Zhu, L. Xie and Y. Xia, Lewis Acid-Catalyzed Ring-Opening Cross-Coupling Reaction of gem-Difluorinated Cyclopropanes Enabled by C–F Bond Activation, Org. Lett., 2022, 24, 8429 CrossRef CAS PubMed.
- Y.-R. Zhao, Z.-Y. Ma, L. Liu, P. Gao, X.-H. Duan and M. Hu, Synthesis of α-Difluoromethylene Ethers via Photoredox-Induced
Hyperconjugative Ring Opening of gem-Difluorocyclopropanes, J. Org. Chem., 2023, 88, 3787 CrossRef CAS PubMed.
- H. Qian, H. D. Nguyen, L. Lv, S. Chen and Z. Li, Chemo-, Stereo- and Regioselective Fluoroallylation/Annulation of Hydrazones with gem-Difluorocyclopropanes via Tunable Palladium/NHC Catalysis, Angew. Chem., Int. Ed., 2023, 62, e202303271 CrossRef CAS PubMed.
- Y. Zeng and Y. Xia, Rhodium-Catalyzed Regio- and Diastereoselective [3+2] Cycloaddition of gem-Difluorinated Cyclopropanes with Internal Olefins, Angew. Chem., Int. Ed., 2023, 62, e202307129 CrossRef CAS PubMed.
- Selected synthetic routes: T. Dubuffet, C. Bidon, P. Martinet, R. Sauvetre and J. F. Normant, Préparation d'alcools allyliques fluorés, J. Organomet. Chem., 1990, 393, 161 CrossRef CAS.
- T. Hanamoto, K. Nishiyama, H. Tateishi and M. Kondo, New Route for the Synthesis of Mono-fluorinated Allyl Alcohols Using the Stereoselective Wittig Olefination via Reaction of (α-flurovinyl)triphenylphosphonium Triflate, Synlett, 2001, 1320 CrossRef CAS.
- R. Zemmouri, M. Kajjout, Y. Castanet, S. Eddarir and C. Rolando, Palladium-Catalyzed Stereoconvergent Formylation of (E/Z)-β-Bromo-β-fluorostyrenes: Straightforward Access to (Z)-α-Fluorocinnamic Aldehydes and (Z)-β-Fluorocinnamic Alcohols, J. Org. Chem., 2011, 76, 7691 CrossRef CAS PubMed.
- M. A. Novikov, N. V. Volchkov, M. B. Lipkind and O. M. Nefedov, Copper(I)-catalyzed solvolysis of gem-chlorofluoro- and gem-bromofluorocyclopropanes. Preparation of 2-fluoroallylic ethers, esters and alcohols, J. Fluor. Chem., 2015, 180, 131 CrossRef CAS.
- X.-Y. Lu, M.-T. Gao, L.-J. Yu, H.-Y. Pan, X. Zhang, R. Huang, K. Yang, F.-Y. Shui, Y.-W. Song and G.-X. Yang, Synthesis of fluorinated allylic alcohols via photoinduced decarboxylative cross-coupling of α-fluoroacrylic acids and alcohols, Org. Chem. Front., 2023, 10, 1788 RSC.
- Importance of cinnamyl alcohols as intermediates for the synthesis of drug molecules, flavors, and fungicides, selected references: R. Zhu, H. Liu, C. Liu, L. Wang, R. Ma, B. Chen, L. Li, J. Niu, M. Fu, D. Zhang and S. Gao, Cinnamaldehyde in diabetes: A review of pharmacology, pharmacokinetics and safety, Pharmacol. Res., 2017, 122, 78 CrossRef CAS PubMed.
- S. Shreaz, W. A. Wani, J. M. Behbehani, V. Raja, M. Irshad, M. Karched, I. Ali, W. A. Siddiqi and L. T. Hun, Cinnamaldehyde and its derivatives, a novel class of antifungal agents, Fitoterapia, 2016, 112, 116 CrossRef CAS PubMed.
- P. S. Babu, S. Prabuseenivasan and S. Ignacimuthu, Cinnamaldehyde—A potential antidiabetic agent, Phytomedicine, 2007, 14, 15 CrossRef CAS PubMed.
- S. C. Ho, K. S. Chang and P. W. Chang, Inhibition of neuroinflammation by cinnamon and its main components, Food Chem., 2013, 138, 2275 CrossRef CAS PubMed.
- Importance of fluorine containing drugs, selected references: H. Mei, J. Han, S. Fustero, M. Medio-Simon, D. M. Sedgwick, C. Santi, R. Ruzziconi and V. A. Soloshonok, Fluorine-Containing Drugs Approved by the FDA in 2018, Chem. – Eur. J., 2019, 25, 11797 CrossRef CAS PubMed.
- H. Chen, S. Viel, F. Ziarelli and L. Peng,
19F NMR: a valuable tool for studying biological events, Chem. Soc. Rev., 2013, 42, 7971 RSC.
- N. C. Yoder and K. Kumar, Fluorinated amino acids in protein design and engineering, Chem. Soc. Rev., 2002, 31, 335 RSC.
- M. Salwiczek, E. K. Nyakatura, U. I. Gerling, S. Ye and B. Koksch, Fluorinated amino acids: compatibility with native protein structures and effects on protein–protein interactions, Chem. Soc. Rev., 2012, 41, 2135 RSC.
- E. N. G. Marsh, Fluorinated Proteins: From Design and Synthesis to Structure and Stability, Acc. Chem. Res., 2014, 47, 2878 CrossRef CAS PubMed.
- X. Wang and F. W. Patureau, Pd-catalyzed access to mono- and di-fluoroallylic amines from primary anilines, Chem. Commun., 2023, 59, 486 RSC.
- E. S. Wallis and J. F. Lane, The Hofmann Reaction, Org. React., 1946, 3, 267 Search PubMed.
- J. Piera, A. Persson, X. Caldentey and J.-E. Bäckvall, Water as Nucleophile in Palladium-Catalyzed Oxidative Carbohydroxylation of Allene-Substituted Conjugated Dienes, J. Am. Chem. Soc., 2007, 129, 14120 CrossRef CAS PubMed.
- T. Fujita, K. Fuchibe and J. Ichikawa, Transition-Metal-Mediated and -Catalyzed C–F Bond Activation by Fluorine Elimination, Angew. Chem., Int. Ed., 2019, 58, 390 CrossRef CAS PubMed.
- For selected recent works, see: Y. Zhao, X. Guo, S. Li, Y. Fan, G.-C. Ji, M. Jiang, Y. Yang and Y.-Y. Jiang, Transient Stabilization Effect of CO2 in the Electrochemical Hydrogenation of Azo Compounds and the Reductive Coupling of α-Ketoesters, Angew. Chem., Int. Ed., 2022, 61, e202213636 CrossRef CAS PubMed.
- S. Okumura, T. Takahashi, K. Torii and Y. Uozumi, Photocatalytic Cross-Pinacol Coupling Promoted by Carbon Dioxide, Chem. – Eur. J., 2023, 29, e202300840 CrossRef CAS PubMed.
- S. Chen and C. Xi, CO2 promoted photoredox/Ni-catalyzed semi-reduction of alkynes with H2O, Green Chem., 2023, 25, 7978 RSC.
- J. Yang, W.-H. Li, H.-T. Tang, Y.-M. Pan, D. Wang and Y. Li, CO2-mediated organocatalytic chlorine evolution under industrial conditions, Nature, 2023, 617, 519 CrossRef CAS PubMed.
- For a selected recent review, see: C.-K. Ran, H.-Z. Xiao, L.-L. Liao, T. Ju, W. Zhang and D.-G. Yu, Progress and challenges in dicarboxylation with CO2, Natl. Sci. Open, 2023, 2, 20220024 CrossRef.
|
This journal is © the Partner Organisations 2024 |