DOI:
10.1039/D3QO01662E
(Research Article)
Org. Chem. Front., 2024,
11, 106-112
Unveiling the potency of a phenalenyl-based photocatalyst for intramolecular dehydrogenative lactonization†‡
Received
10th October 2023
, Accepted 2nd November 2023
First published on 7th November 2023
Abstract
Here, an intramolecular dehydrogenative lactonization reaction has been accomplished by combining a phenalenyl-based organic photocatalyst and a Co(III) co-catalyst. Due to its extremely high excited state redox potential, the phenalenyl motif could directly oxidise carboxylic acid derivatives to generate carboxyl free radicals without the assistance of an external base. This protocol allows access to multiple 4-aryl-2H-chromen-2-ones by using the external oxidant K2S2O8 and shows diverse functional group tolerance for biaryl-2-carboxylic acids. Moreover, the reaction was performed on a bulk scale with a very good yield.
In the past few decades, visible-light-induced photoredox catalysis has become a powerful synthetic tool for creating molecular diversity.1,2 Although polypyridyl complexes of heavier transition metals3 like Ru and Ir that use the energy of photons to architect several molecular complexities have dominated photocatalysis in the past, it is clear that an organic photocatalyst with careful design can offer the following benefits: (a) sustainability, (b) tolerance of a wide range of functional groups, (c) cost effectiveness, and (d) lesser unwanted side reactions. As a result, successful efforts have been made to design organic photocatalysts4 which essentially facilitate several chemical transformations that were previously challenging and difficult to carry out using standard chemical protocols. Despite the fact that these organic photocatalysts have led to significant improvements in a number of chemical transformations, efforts are ongoing to create novel organic photocatalysts5 with excited state potentials up to +2.5 V vs. Ag/AgCl (Scheme 1a), enabling the oxidation of readily available starting materials for the creation of high-energy radical centres.
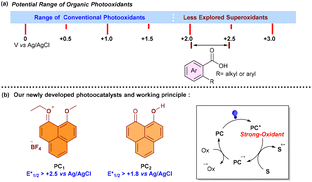 |
| Scheme 1 Potential range of conventional photocatalysts and our newly developed photocatalyst. | |
In recent years, the phenalenyl moiety6 has been studied extensively as a Lewis acid7 and a strong reducing agent8 in the ground state to replicate a number of transition-metal catalysed reactions.
With our ongoing efforts to design new photochemical transformations, while demonstrating the photoactivity of this moiety, a very low magnitude of reduction potential (−0.21 V vs. Ag/AgCl, Fig. 1a) for PC1 was observed. This low reduction potential led to a high redox potential (
vs. Ag/AgCl) of PC1 in the excited state. In the UV-visible spectrum, the presence of bands around 420–480 nm makes this bench stable PC1 susceptible to absorbing blue light (Fig. 1b). When we recorded the emission spectra to check the photostability of PC1, no decrease in the emission intensity of PC1 was noticed after 5 times excitation at 440 nm (Fig. 1c), which indicated that photobleaching did not occur with PC1. Furthermore, from the fluorescence decay curve, the emission lifetime of PC1 was found to be τ = 2.85 ns (amplitude weighted), which indicates that it might act as a potent photocatalyst (Fig. 1d). In fact, we have recently been able to offer a phenalenyl-based scaffold (Scheme 1b) as a potent photooxidant for the azolation of an unactivated feedstock arenes.9 Therefore, with the knowledge of the above photophysical properties and our literature precedent, one might anticipate that this phenalenyl photocatalyst (PC1) would readily oxidise carboxylic acid derivatives (with Eox > +2.0 V vs. Ag/AgCl) in the presence of light in order to generate a high energy carboxyl free radical and we reasoned that PC1 could be used for intramolecular cyclization to convert biaryl-2-carboxylic acids into the bioactive benzo-3,4-coumarin core (Scheme 2).
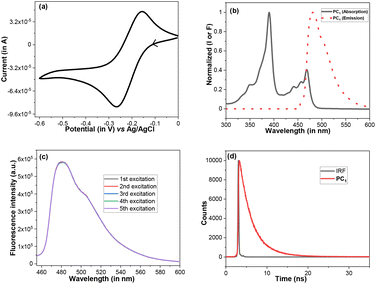 |
| Fig. 1 Properties of the photocatalyst (PC1): (a) one electron reduction of PC1, (b) normalized absorption and emission, (c) photostability of the catalyst, and (d) fluorescence decay curve (lifetime of PC1). | |
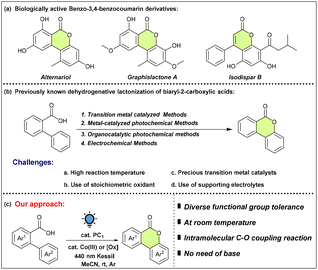 |
| Scheme 2 Literature precedents and our approach. | |
Numerous attempts to create such coumarin scaffolds have met with success owing to the extensive application of the benzo-3,4-coumarin skeletal unit in a broad range of pharmaceuticals, fluorescent materials, and other fine chemicals (Scheme 2a).10 The most common methods typically call for harsh reaction conditions and the use of transition metals (Cu, Co, and Ag) and lanthanides (Ce).11 Nevertheless, photoinduced LMCT of Cu, Ce and Fe at ambient temperature is well known12 for intramolecular lactonization. While Mes-Acr+ and other organic photocatalytic pathways13 have received a lot of attention for this transformation, an oxidising agent in stoichiometric proportions is necessary. While it is critical to gain an appreciation for the benefits of metal mediated and electrically driven14 synthesis of coumarin derivatives, the use of stoichiometric amount of electrolytes/additives remained a concern that necessitated atom-economical approaches. Considering these constraints, we set out to evaluate the efficiency of a phenalenyl based organic photocatalyst to induce intramolecular dehydrogenative lactonization.
To reach our goal, we employed 2-phenylbenzoic acid (1a) as the model substrate under the photochemical conditions. To our delight, by utilizing PC1 (5 mol%) as the photocatalyst and CoIII(dmgH)2PyCl (10 mol%) as the co-catalyst in MeCN with 440 nm Kessil lamp irradiation for 24 h under an argon atmosphere, we obtained the desired product 2a in 98% yield (Table 1, entry 1). On varying the solvents from MeCN to DCE, DMF and MeOH, the yield decreased to 92%, 80% and 77%, respectively (Table 1, entry 2). When the reaction mixture was irradiated with 456 nm and 427 nm light, the formation of the desired product decreased slightly and 2a was produced in 91% and 96% yields, respectively (Table 1, entry 3). Furthermore, on changing the co-catalyst to CoIII(acac)3, the yield of 2a reduced drastically (Table 1, entry 4). On changing the photocatalyst from PC1 to PC2, we were unable to detect traces of the desired product (Table 1, entry 5). Control experiments revealed that the presence of the photocatalyst and co-catalyst and visible light irradiation was crucial for the dehydrogenative lactonization under ambient conditions (Table 1, entries 6–8). More importantly, the literature13d suggests that a base is necessary to reduce the oxidation potential of 1a. However, PC1 having a very high excited state potential could directly oxidise 1a. Therefore, this protocol does not necessitate an extraneous base for the transformation, making it more atom economical.
Table 1 Optimization of the reaction parametersa
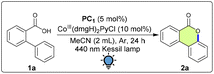
|
Entry |
Variation from the standard conditions |
Yieldb (%) |
Standard reaction conditions unless specified: [1,1′-biphenyl]-2-carboxylic acid (1a, 0.2 mmol), PC1 (5 mol%), CoIII(dmgH)2PyCl (10 mol%), MeCN (2 mL), 440 nm Kessil lamp irradiation, 24 h, room temperature (rt), Ar atmosphere.
Yield of 2a determined by gas chromatography (GC) using benzophenone as the internal standard.
|
1 |
None |
98 |
2 |
DCE, DMF, MeOH instead of MeCN |
92, 80, 77 |
3 |
427 nm and 456 nm |
96, 91 |
4 |
CoIII(acac)3 instead of CoIII(dmgH)2PyCl |
<5 |
5 |
PC2 instead of PC1 |
n.d. |
6 |
No PC1 |
<5 |
7 |
No CoIII(dmgH)2PyCl |
8 |
8 |
No light irradiation |
0 |
With the optimized conditions in hand, we sought to explore the substrate scope of this photoinduced dehydrogenative lactonization protocol. Substituents on the aryl rings of 2-phenylbenzoic acid significantly determined the outcome of the reaction (Table 2). As a result, the electronic and steric effects of various groups attached to the reactants were examined, leading to the production of a wide range of products with yields ranging from good to excellent (up to 96%). Initially, the substrates in which electron-donating substituents were attached to the Ar2 ring were examined. To our delight, the desired products having electron-donating –Me (2b, 85%), –OMe (2c, 83%) and –tBu (2d, 82%) groups at the 4-position of Ar2 were obtained in good yields. Furthermore, excellent yields were obtained when substrates with electron-withdrawing groups such as –CF3 (2e, 85%), –OCF3 (2f, 89%), –F (2g, 90%) and –Cl (2h, 84%) at the 4-position of the Ar2 ring were subjected to the lactonization conditions. When the reactant containing a 4-Ph group on the Ar2 ring was employed in the reaction, the corresponding product 2i was obtained in 77% yield. When biaryl-2-carboxylic acid bearing a methyl substituent at the meta position was employed under the reaction conditions, the desired product was formed in a good yield (2j, 74%) with the regioisomeric ratio (rr) = 2.3
:
1. Furthermore, on varying the substituents on the Ar1 and Ar2 rings simultaneously, we obtained the corresponding products in good to excellent yields. When we made a substitution on the Ar1 ring with the –Me group and no group on the Ar2 ring, we obtained the desired product 2k in 89% yield. Next, keeping the –Me group fixed, groups on the Ar2 ring were varied from –Me, –OMe, –F to –Cl, giving products 2l (80%), 2m (81%), 2n (90%) and 2o (88%). When naphthalene was utilized as one of the aryl groups, the desired product 2p was furnished in a good yield (74%).
Table 2 Substrate scope for the synthesis of benzo-3,4-coumarinsa,b
Standard reaction conditions unless specified: [1,1′-biaryl]-2-carboxylic acid (1, 0.2 mmol), PC1 (5 mol%), CoIII(dmgH)2PyCl (10 mol%), MeCN (2 mL), 440 nm Kessil lamp irradiation, 24 h, room temperature (rt), Ar atmosphere.
Isolated yields from the two reaction mixtures at the 0.2 mmol scale each.
|
|
After achieving success in generalizing the scope of 2-arylbenzoic acids, we moved on to diversify the substrate scope by using 3,3-diarylacrylic acid derivatives to synthesize 4-aryl coumarins. It is worth noting that the 4-aryl coumarin skeleton is known as a member of the flavonoid family and is present in many natural products having mostly anticancer properties and many other biological activities.15 However, under the optimized conditions, 3,3-diphenylacrylic acid did not lead to efficient conversion to the desired product 2q. Therefore, we screened different reaction parameters, and it was observed that when K2S2O8 was used as the oxidant (Table 3), the desired product 2q was obtained in an excellent yield (90%). Therefore, we utilized the newly developed optimized conditions for the lactonization of 3,3-diarylacrylic acids. In this transformation as well, the electronics of the carboxylic acid moiety played a pivotal role in the formation of the desired products. Electron-donating substituents such as 4-Me, 3,5-dimethyl and tBu yielded the corresponding products 2r (86%), 2s (83%) and 2t (83%) in good yields. However, when electron-withdrawing groups were embedded in the aryl core, comparatively higher yields of the desired products were observed. When the aryl rings were substituted with 4-OCF3 and 4-CF3 groups, the desired products 2u and 2v were obtained in 88% and 85% yields, respectively. Excellent yields were also obtained in the case of halo-substituents such as –F (2w, 92%), –Cl (2x, 94%) and –Br (2y, 88%).
Table 3 Substrate scope for the synthesis of 4-aryl-2H-chromen-2-onesa,b
Standard reaction conditions unless specified: 3,3-diarylacrylic acids (1, 0.2 mmol), PC1 (5 mol%), K2S2O8 (2.0 equiv.), MeCN (2 mL), 440 nm Kessil lamp irradiation, 24 h, room temperature (rt), Ar atmosphere.
Isolated yields from the two reaction mixtures at the 0.2 mmol scale each.
|
|
Once the scope of the reaction was understood, numerous spectroscopic studies were performed to deduce the reaction mechanism. No discernible changes in the absorption spectra were initially seen when we conducted UV-Vis investigations (see the ESI‡) of PC1 with the subsequent addition of 1a, indicating that there was no ground state complexation between PC1 and 1a. Fluorescence quenching studies were then carried out to determine whether the interactions were occurring at the excited state, and these studies revealed a gradual decline in the fluorescence intensity of PC1 upon sequential addition of 1a (see the ESI‡). Since we obtained a straight line with a positive slope (Fig. 2a) when we plotted the Stern–Volmer graph, it is possible to infer from these studies that PC1 and 1a are interacting at the excited state. Furthermore, no signal was detected during the EPR experiments when PC1 and 1a were combined (Fig. 2b, black trace). However, after exposing this mixture to 440 nm radiation for 6 h, a clear signal was obtained with a g value of 2.007, indicating the formation of a delocalized carbon-centered radical species16 which was believed to be the radical anion species (PC1˙−) of PC1 in the ground state (Fig. 2b, red trace) after comparing it to the simulated spectrum (Fig. 2b, blue trace).
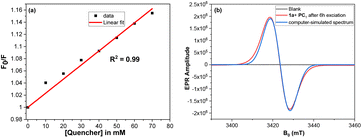 |
| Fig. 2 (a) Fluorescence quenching study (Stern–Volmer plot) and (b) EPR analysis. | |
In order to further support the radical formation during the course of the reaction, the model reaction was performed in the presence of commonly used radical scavengers like butylated hydroxytoluene (BHT) and (2,2,6,6-tetramethylpiperidin-1-yl)oxyl (TEMPO). In both cases, the formation of a small amount of products (Scheme 3) suggested that radical intermediacy was the dominant pathway for the reaction to occur.
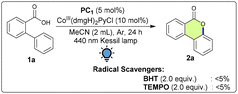 |
| Scheme 3 Radical scavenging analysis. | |
Based on the above results, a plausible mechanism has been shown in Scheme 4. When exposed to visible light, PC1 first experiences photoexcitation, resulting in PC1*, which is then intercepted by 1a to produce intermediate I and itself is converted to PC1˙−.
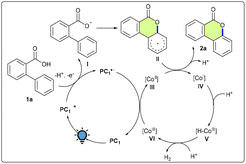 |
| Scheme 4 Proposed mechanism. | |
Simultaneously, I transforms into II through intramolecular cyclization. Intermediate II then undergoes SET with intermediate III to give IV and finally produce 2a. Intermediate IV captures the proton to produce V, which is converted to VI with the expulsion of H2.
Additionally, we were able to scale up (Scheme 5) the reaction to the 5.0 mmol scale with a good yield of the desired product 2a.
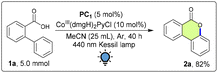 |
| Scheme 5 Bulk scale synthesis. | |
Conclusions
In summary, by combining a phenalenyl-based organic photocatalyst with a Co(III/II) catalyst, we have demonstrated a new photochemical method for intramolecular dehydrogenative lactonization to synthesize bioactive coumarin molecules. Along with gram scale lactonization, the scope of the reaction is broad as several electron-donating and electron-withdrawing groups, as well as reactive functionalities, are tolerated in this procedure. The feasible mechanism was finally discovered to be proceeding through the reductive quenching of PC1, which was extensively studied using CV, UV-visible spectra analysis, and EPR analysis.
Author contributions
SRR conceived the idea. PPS and VP performed the experiments. PPS and VP prepared the ESI.‡ All authors discussed the results and contributed to the conceptualization of the project and editing the manuscript.
Conflicts of interest
The authors declare no conflict of interest.
Acknowledgements
SRR thanks SERB (CRG/2022/002306) for financial support. VP thanks MHRD (GoI) for the Prime Minister's Research Fellowship. The authors acknowledge CRF, IIT Delhi for instrument facilities.
References
-
(a) Q.-Q. Zhou, Y.-Q. Zou, L.-Q. Lu and W.-J. Xiao, Visible-Light-Induced Organic Photochemical Reactions through Energy-Transfer Pathways, Angew. Chem., Int. Ed., 2019, 58, 1586–1604 CrossRef CAS PubMed;
(b) H. D. Roth, The Beginnings of Organic Photochemistry, Angew. Chem., Int. Ed. Engl., 1989, 28, 1193–1207 CrossRef;
(c) L. Marzo, S. K. Pagire, O. Reiser and B. König, Visible-Light Photocatalysis: Does It Make a Difference in Organic Synthesis?, Angew. Chem., Int. Ed., 2018, 57, 10034–10072 CrossRef CAS PubMed;
(d) S. Singh, V. J. Roy, N. Dagar, P. P. Sen and S. R. Roy, Photocatalysis in Dual Catalysis Systems for Carbon-Nitrogen Bond Formation, Adv. Synth. Catal., 2021, 363, 937–979 CrossRef CAS;
(e) C. K. Prier, D. A. Rankic and D. W. C. MacMillan, Visible Light Photoredox Catalysis with Transition Metal Complexes: Applications in Organic Synthesis, Chem. Rev., 2013, 113, 5322–5363 CrossRef CAS PubMed;
(f) L. Buzzetti, G. E. M. Crisenza and P. Melchiorre, Mechanistic Studies in Photocatalysis, Angew. Chem., Int. Ed., 2019, 58, 3730–3747 CrossRef CAS PubMed;
(g) G. E. M. Crisenza and P. Melchiorre, Chemistry Glows Green with Photoredox Catalysis, Nat. Commun., 2020, 11, 803 CrossRef PubMed.
-
(a) C. Cavedon, P. H. Seeberger and B. Pieber, Photochemical Strategies for Carbon–Heteroatom Bond Formation, Eur. J. Org. Chem., 2020, 2020, 1379–1392 CrossRef CAS;
(b) P. L. Gkizis, Photochemical Cross-Coupling Enables Csp3−C Bond Formation by C−H Homolytic Cleavage: New Developments and Perspectives, Eur. J. Org. Chem., 2022, 2022, e202201139 CrossRef CAS;
(c) D. Ravelli, S. Protti and M. Fagnoni, Carbon–Carbon Bond Forming Reactions via Photogenerated Intermediates, Chem. Rev., 2016, 116, 9850–9913 CrossRef CAS PubMed.
-
(a) C. R. Bock, J. A. Connor, A. R. Gutierrez, T. J. Meyer, D. G. Whitten, B. P. Sullivan and J. K. Nagle, Estimation of Excited-State Redox Potentials by Electron-Transfer Quenching. Application of Electron-Transfer Theory to Excited-State Redox Processes, J. Am. Chem. Soc., 1979, 101, 4815–4824 CrossRef CAS;
(b) M. Graetzel, Artificial Photosynthesis: Water Cleavage into Hydrogen and Oxygen by Visible Light, Acc. Chem. Res., 1981, 14, 376–384 CrossRef CAS;
(c) T. J. Meyer, Chemical Approaches to Artificial Photosynthesis, Acc. Chem. Res., 1989, 22, 163–170 CrossRef CAS.
-
(a) T. Bortolato, S. Cuadros, G. Simionato and L. Dell'Amico, The Advent and Development of Organophotoredox Catalysis, Chem. Commun., 2022, 58, 1263–1283 RSC;
(b) S. G. E. Amos, M. Garreau, L. Buzzetti and J. Waser, Photocatalysis with Organic Dyes: Facile Access to Reactive Intermediates for Synthesis, Beilstein J. Org. Chem., 2020, 16, 1163–1187 CrossRef CAS PubMed;
(c) A. Vega-Peñaloza, J. Mateos, X. Companyó, M. Escudero-Casao and L. Dell'Amico, A Rational Approach to Organo-Photocatalysis: Novel Designs and Structure-Property Relationships, Angew. Chem., Int. Ed., 2021, 60, 1082–1097 CrossRef PubMed;
(d) N. A. Romero and D. A. Nicewicz, Organic Photoredox Catalysis, Chem. Rev., 2016, 116, 10075–10166 CrossRef CAS PubMed;
(e) D. A. Nicewicz and T. M. Nguyen, Recent Applications of Organic Dyes as Photoredox Catalysts in Organic Synthesis, ACS Catal., 2014, 4, 355–360 CrossRef CAS;
(f) M. K. Bogdos, E. Pinard and J. A. Murphy, Applications of Organocatalysed Visible-Light Photoredox Reactions for Medicinal Chemistry, Beilstein J. Org. Chem., 2018, 14, 2035–2064 CrossRef CAS PubMed.
-
(a) P. Natarajan and B. König, Excited-State 2,3-Dichloro-5,6-Dicyano-1,4-Benzoquinone (DDQ*) Initiated Organic Synthetic Transformations under Visible-Light Irradiation, Eur. J. Org. Chem., 2021, 2021, 2145–2161 CrossRef CAS;
(b) K. Targos, O. P. Williams and Z. K. Wickens, Unveiling Potent Photooxidation Behavior of Catalytic Photoreductants, J. Am. Chem. Soc., 2021, 143, 4125–4132 CrossRef CAS PubMed;
(c) E. Speckmeier, T. G. Fischer and K. Zeitler, A Toolbox Approach To Construct Broadly Applicable Metal-Free Catalysts for Photoredox Chemistry: Deliberate Tuning of Redox Potentials and Importance of Halogens in Donor–Acceptor Cyanoarenes, J. Am. Chem. Soc., 2018, 140, 15353–15365 CrossRef CAS PubMed.
-
(a) T. Amos and L. C. Snyder, Unrestricted Hartree—Fock Calculations. I. An Improved Method of Computing Spin Properties, J. Chem. Phys., 1964, 41, 1773–1783 CrossRef CAS;
(b) K. Fukui, T. Yonezawa and H. Shingu, A Molecular Orbital Theory of Reactivity in Aromatic Hydrocarbons, J. Chem. Phys., 1952, 20, 722–725 CrossRef CAS;
(c) M. Bhunia, S. R. Sahoo, B. K. Shaw, S. Vaidya, A. Pariyar, G. Vijaykumar, D. Adhikari and S. K. Mandal, Storing Redox Equivalent in the Phenalenyl Backbone towards Catalytic Multi-Electron Reduction, Chem. Sci., 2019, 10, 7433–7441 RSC;
(d) A. Mukherjee, S. C. Sau and S. K. Mandal, Exploring Closed-Shell Cationic Phenalenyl: From Catalysis to Spin Electronics, Acc. Chem. Res., 2017, 50, 1679–1691 CrossRef CAS PubMed;
(e) R. C. Haddon, Design of Organic Metals and Superconductors, Nature, 1975, 256, 394–396 CrossRef CAS;
(f) M. E. Itkis, X. Chi, A. W. Cordes and R. C. Haddon, Magneto-Opto-Electronic Bistability in a Phenalenyl-Based Neutral Radical, Science, 2002, 296, 1443–1445 CrossRef CAS PubMed;
(g) S. K. Pal, M. E. Itkis, F. S. Tham, R. W. Reed, R. T. Oakley and R. C. Haddon, Resonating Valence-Bond Ground State in a Phenalenyl-Based Neutral Radical Conductor, Science, 2005, 309, 281–284 CrossRef CAS PubMed;
(h) K. V. Raman, A. M. Kamerbeek, A. Mukherjee, N. Atodiresei, T. K. Sen, P. Lazić, V. Caciuc, R. Michel, D. Stalke, S. K. Mandal, S. Blügel, M. Münzenberg and J. S. Moodera, Interface-Engineered Templates for Molecular Spin Memory Devices, Nature, 2013, 493, 509–513 CrossRef CAS PubMed;
(i) W. Fujita and K. Awaga, Room-Temperature Magnetic Bistability in Organic Radical Crystals, Science, 1999, 286, 261–263 CrossRef CAS PubMed.
-
(a) J. Ahmed, S. Chakraborty, A. Jose, P. Sreejyothi and S. K. Mandal, Integrating Organic Lewis Acid and Redox Catalysis: The Phenalenyl Cation in Dual Role, J. Am. Chem. Soc., 2018, 140, 8330–8339 CrossRef CAS PubMed;
(b) S. R. Roy, A. Nijamudheen, A. Pariyar, A. Ghosh, P. K. Vardhanapu, P. K. Mandal, A. Datta and S. K. Mandal, Phenalenyl in a Different Role: Catalytic Activation through the Nonbonding Molecular Orbital, ACS Catal., 2014, 4, 4307–4319 CrossRef CAS.
-
(a) S. Sil, A. S. Bhaskaran, S. Chakraborty, B. Singh, R. Kuniyil and S. K. Mandal, Reduced-Phenalenyl-Based Molecule as a Super Electron Donor for Radical-Mediated C–N Coupling Catalysis at Room Temperature, J. Am. Chem. Soc., 2022, 144, 22611–22621 CrossRef CAS PubMed;
(b) S. Chakraborty, A. Das and S. K. Mandal, Redox-Active Ligand Based Mn(i)-Catalyst for Hydrosilylative Ester Reduction, Chem. Commun., 2021, 57, 12671–12674 RSC;
(c) B. Singh, J. Ahmed, A. Biswas, R. Paira and S. K. Mandal, Reduced Phenalenyl in Catalytic Dehalogenative Deuteration and Hydrodehalogenation of Aryl Halides, J. Org. Chem., 2021, 86, 7242–7255 CrossRef CAS PubMed;
(d) A. Banik and S. K. Mondal, Tuning Redox States of Phenalenyl-Based Molecules by Consecutive Reduction toward Transition Metal-Free Heck-Type C–C Cross-Coupling, ACS Catal., 2022, 12, 5000–5012 CrossRef CAS;
(e) J. Ahmed, P. Datta, A. Das, S. Jomy and S. K. Mandal, Switching between Mono and Doubly Reduced Odd Alternant Hydrocarbon: Designing a Redox Catalyst, Chem. Sci., 2021, 12, 3039–3049 RSC;
(f) J. Ahmed and S. K. Mandal, Phenalenyl Radical: Smallest Polycyclic Odd Alternant Hydrocarbon Present in the Graphene Sheet, Chem. Rev., 2022, 122, 11369–11431 CrossRef CAS.
- P. P. Sen and S. R. Roy, Introducing Phenalenyl-Based Organic Lewis Acid as a Photocatalyst to Facilitate Oxidative Azolation of Unactivated Arenes, Org. Lett., 2023, 25, 1895–1900 CrossRef CAS PubMed.
-
(a) N. Kim, M. J. Sohn, H. Koshino, E. H. Kim and W. G. Kim, Verrulactone C with an Unprecedented Dispiro Skeleton, a New Inhibitor of Staphylococcus Aureus Enoyl-ACP Reductase, from Penicillium Verruculosum F375, Bioorg. Med. Chem. Lett., 2014, 24, 83–86 CrossRef CAS PubMed;
(b) A. J. Demuner, L. C. Barbosa, A. C. Miranda, G. C. Geraldo, C. M. da Silva, S. Giberti, M. Bertazzini and G. Forlani, The Fungal Phytotoxin Alternariol 9-Methyl Ether and Some of Its Synthetic Analogues Inhibit the Photosynthetic Electron Transport Chain, J. Nat. Prod., 2013, 76, 2234–2245 CrossRef CAS PubMed;
(c) J. M. Schmidt, G. B. Tremblay, M. Page, J. Mercure, M. Feher, R. Dunn-Dufault, M. G. Peter and P. R. Redden, Synthesis and Evaluation of a Novel Nonsteroidal-Specific Endothelial Cell Proliferation Inhibitor, J. Med. Chem., 2003, 46, 1289–1292 CrossRef CAS PubMed;
(d) K. Koch, J. Podlech, E. Pfeiffer and M. Metzler, Total Synthesis of Alternariol, J. Org. Chem., 2005, 70, 3275–3276 CrossRef CAS PubMed;
(e) W. Sun, L. D. Cama, E. T. Birzin, S. Warrier, L. Locco, R. Mosley, M. L. Hammond and S. P. Rohrer, 6H-Benzo[c]Chromen-6-One Derivatives as Selective ERβ Agonists, Bioorg. Med. Chem. Lett., 2006, 16, 1468–1472 CrossRef CAS PubMed;
(f) S. P. Fletcher, F. Dumur, M. M. Pollard and B. L. Feringa, A Reversible, Unidirectional Molecular Rotary Motor Driven by Chemical Energy, Science, 2005, 310, 80–82 CrossRef CAS PubMed.
-
(a) J. Gallardo-Donaire and R. Martin, Cu-Catalyzed Mild C(Sp2)–H Functionalization Assisted by Carboxylic Acids En Route to Hydroxylated Arenes, J. Am. Chem. Soc., 2013, 135, 9350–9353 CrossRef CAS PubMed;
(b) X. Wang, J. Gallardo-Donaire and R. Martin, Mild ArI-Catalyzed C(Sp2)-H or C(Sp3)-H Functionalization/C-O Formation: An Intriguing Catalyst-Controlled Selectivity Switch, Angew. Chem., Int. Ed., 2014, 53, 11084–11087 CrossRef CAS PubMed;
(c) Y. Wang, A. V. Gulevich and V. Gevorgyan, General and Practical Carboxyl-Group-Directed Remote C-H Oxygenation Reactions of Arenes, Chem. – Eur. J., 2013, 19, 15836–15840 CrossRef CAS PubMed;
(d) Y. Li, Y. J. Ding, J. Y. Wang, Y. M. Su and X. S. Wang, Pd-Catalyzed C–H Lactonization for Expedient Synthesis of Biaryl Lactones and Total Synthesis of Cannabinol, Org. Lett., 2013, 15, 2574–2577 CrossRef CAS PubMed;
(e) J.-J. Dai, W.-T. Xu, Y.-D. Wu, W.-M. Zhang, Y. Gong, X.-P. He, X.-Q. Zhang and H.-J. Xu, Silver-Catalyzed C(Sp2)–H Functionalization/C–O Cyclization Reaction at Room Temperature, J. Org. Chem., 2015, 80, 911–919 CrossRef CAS PubMed;
(f) M. Chao, H. Wang, H. Zhang, F. Zhong, Z. Luo, F. Wu, F. Sun, J. Jiang, X. He, S. Zhang, P. Gong, B. Wang and D. Shen, Cobalt(II)-Catalyzed Oxidation of 2-Aryl Benzoic Acids to Access Biaryl Lactones, Appl. Organomet. Chem., 2022, 36(9), e6809 CrossRef CAS;
(g) M. Chao, F. Wang, L. Xu, Y. Ju, Z. Chen, B. Wang, P. Gong, J. You, M. Jin and D. Shen, Cerium Ammonium Nitrate-Mediated Access to Biaryl Lactones: Substrate Scopes and Mechanism Studies, J. Org. Chem., 2021, 86, 13371–13380 CrossRef CAS PubMed;
(h) P. Gao and Y. Wei, NIS-Mediated Oxidative Lactonization of 2-Arylbenzoic Acids for the Synthesis of Dibenzopyranones under Metal-Free Conditions, Synthesis, 2014, 46, 343–347 Search PubMed.
-
(a) P. P. Sen and S. R. Roy, Harnessing the Energy of Visible Light to Promote LMCT of Cu(II) Species for Intramolecular Dehydrogenative Lactonization of Biaryl-2-Carboxylic Acids, Organometallics, 2023, 42, 1658–1666 CrossRef CAS;
(b) K. Wadekar, S. Aswale and V. R. Yatham, Cerium Photocatalyzed Dehydrogenative Lactonization of 2-Arylbenzoic Acids, Org. Biomol. Chem., 2020, 18, 983–987 RSC;
(c) S. Xia, K. Hu, C. Lei and J. Jin, Intramolecular Aromatic C–H Acyloxylation Enabled by Iron Photocatalysis, Org. Lett., 2020, 22, 1385–1389 CrossRef CAS PubMed.
-
(a) N. P. Ramirez, I. Bosque and J. C. Gonzalez-Gomez, Photocatalytic Dehydrogenative Lactonization of 2-Arylbenzoic Acids, Org. Lett., 2015, 17, 4550–4553 CrossRef CAS PubMed;
(b) A. Shao, J. Zhan, N. Li, C.-W. Chiang and A. Lei, External Oxidant-Free Dehydrogenative Lactonization of 2-Arylbenzoic Acids via Visible-Light Photocatalysis, J. Org. Chem., 2018, 83, 3582–3589 CrossRef CAS PubMed;
(c) M. Zhang, R. Ruzi, N. Li, J. Xie and C. Zhu, Photoredox and Cobalt Co-Catalyzed C(Sp2)–H Functionalization/C–O Bond Formation for Synthesis of Lactones under Oxidant- and Acceptor-Free Conditions, Org. Chem. Front., 2018, 5, 749–752 RSC;
(d) Q. Yang, Z. Jia, L. Li, L. Zhang and S. Luo, Visible-Light Promoted Arene C–H/C–X Lactonization via Carboxylic Radical Aromatic Substitution, Org. Chem. Front., 2018, 5, 237–241 RSC;
(e) T. Morack, J. B. Metternich and R. Gilmour, Vitamin Catalysis: Direct, Photocatalytic Synthesis of Benzocoumarins via (−)-Riboflavin-Mediated Electron Transfer, Org. Lett., 2018, 20, 1316–1319 CrossRef CAS PubMed;
(f) Y. Wang, S. Wang, B. Chen, M. Li, X. Hu, B. Hu, L. Jin, N. Sun and Z. Shen, Visible-Light-Induced Arene C(Sp2)–H Lactonization Promoted by DDQ and Tert-Butyl Nitrite, Synlett, 2020, 31, 261–266 CrossRef CAS.
-
(a) S. Zhang, L. Li, H. Wang, Q. Li, W. Liu, K. Xu and C. Zeng, Scalable Electrochemical Dehydrogenative Lactonization of C(sp2/sp3)–H Bonds, Org. Lett., 2018, 20, 252–255 CrossRef CAS PubMed;
(b) A. Shao, N. Li, Y. Gao, J. Zhan, C.-W. Chiang and A. Lei, Electrochemical Intramolecular C—H/O—H Cross-Coupling of 2-Arylbenzoic Acids, Chin. J. Chem., 2018, 36, 619–624 CrossRef CAS;
(c) X.-Z. Tao, J.-J. Dai, J. Zhou, J. Xu and H.-J. Xu, Electrochemical C−O Bond Formation: Facile Access to Aromatic Lactones, Chem. – Eur. J., 2018, 24, 6932–6935 CrossRef CAS PubMed;
(d) L. Li, Q. Yang, Z. Jia and S. Luo, Organocatalytic Electrochemical C–H Lactonization of Aromatic Carboxylic Acids, Synthesis, 2018, 50, 2924–2929 CrossRef CAS;
(e) L. Zhang, Z. Zhang, J. Hong, J. Yu, J. Zhang and F. Mo, Oxidant-Free C(Sp2)–H Functionalization/C–O Bond Formation: A Kolbe Oxidative Cyclization Process, J. Org. Chem., 2018, 83, 3200–3207 CrossRef CAS PubMed.
-
(a) B. I. Alo, A. Kandil, P. A. Patil, M. J. Sharp, M. A. Siddiqui and V. Snieckus, Sequential Directed Ortho Metalation-Boronic Acid Cross-Coupling Reactions. A General Regiospecific Route to Oxygenated Dibenzo[b,d]Pyran-6-Ones Related to Ellagic Acid, J. Org. Chem., 1991, 56, 3763–3768 CrossRef CAS;
(b) N. Tibrewal, P. Pahari, G. Wang, M. K. Kharel, C. Morris, T. Downey, Y. Hou, T. S. Bugni and J. Rohr, Baeyer–Villiger C–C Bond Cleavage Reaction in Gilvocarcin and Jadomycin Biosynthesis, J. Am. Chem. Soc., 2012, 134, 18181–18184 CrossRef CAS PubMed;
(c) R. Omar, L. Li, T. Yuan and N. P. Seeram, α-Glucosidase Inhibitory Hydrolyzable Tannins from Eugenia Jambolana Seeds, J. Nat. Prod., 2012, 75, 1505–1509 CrossRef CAS PubMed;
(d) C.-W. Yang, T.-H. Hsia, C.-C. Chen, C.-K. Lai and R.-S. Liu, Synthesis and Columnar Mesophase of Fluorescent Liquid Crystals Bearing a C2-Symmetric Chiral Core, Org. Lett., 2008, 10, 4069–4072 CrossRef CAS PubMed.
- P. B. Sogo, M. Nakazaki and M. Calvin, Free Radical from Perinaphthene, J. Chem. Phys., 1957, 26, 1343–1345 CrossRef CAS.
Footnotes |
† Dedicated to Prof. Goverdhan Mehta on the occasion of his 80th birthday. |
‡ Electronic supplementary information (ESI) available. See DOI: https://doi.org/10.1039/d3qo01662e |
§ These authors contributed equally. |
|
This journal is © the Partner Organisations 2024 |