DOI:
10.1039/D3QO01579C
(Research Article)
Org. Chem. Front., 2024,
11, 277-283
Carbon atom insertion into N-heterocyclic carbenes to yield 3,4-dihydroquinoxalin-2(1H)-ones†
Received
26th September 2023
, Accepted 8th November 2023
First published on 10th November 2023
Abstract
Carbon atom insertion into a cyclic framework is an attractive form of molecular editing since it can modify the core skeleton of a molecule, allowing for controlled increases in molecular size and complexity. We have discovered that when benzimidazoliums and 2-(methylsulfonyl)chromones were mixed under basic conditions, followed by the addition of a basic aqueous solution/mixture, the carbon atom located at position 2 of the chromone could be inserted into the in situ-generated N-heterocyclic carbenes (NHCs) to afford (Z)-3-(2-phenyl-2-oxoethylidene)-3,4-dihydroquinoxalin-2(1H)-ones, a scaffold found in numbers of bioactive compounds. Under the same conditions, 1-methyl-3-phenylbenzimidazolium iodide provided an unexpected pentacyclic product with a [1]benzopyrano[2,3-b]phenazine framework in an excellent yield presumably via a pathway involving a single-carbon transfer from the NHC.
Introduction
Molecular editing is a powerful strategy for accessing new areas of chemical space.1 Unlike peripheral editing strategies such as C–H functionalization, it can directly alter the skeletal core of a molecule via the insertion,2 deletion,3 or exchange4 of one or more atoms. The development of new molecular editing methods for highly functionalized molecules is of particular interest for drug discovery.5
Among the methods of molecular editing, single-atom insertion reactions hold the potential for the greatest leap in molecular complexity from the lead scaffold. Insertion of single atoms bearing substituents can expand both the skeletal and peripheral structures at the same time, reducing further synthetic steps. Despite the concept being commonly applied in reactions with carbonyl compounds, for instance in Beckmann rearrangement2a and Baeyer–Villiger oxidation,2b single-atom insertions into aromatic skeletal cores are still uncommon. Furthermore, in comparison to the introduction of heteroatoms, reports on the insertion of single carbon atoms are relatively scarce.
Carbon atom insertions with aromatic compounds can be traced back to 1881 with the Ciamician–Dennstedt rearrangement2c reaction. In this reaction, dichlorocarbene provides a carbon source for the introduction of a carbon atom into pyrroles to form 3-chloropyridines. Despite the potential synthetic utility of this method, the competing Reimer–Tiemann reaction leads to low yields and has hindered the widespread adoption of this method (Scheme 1).6
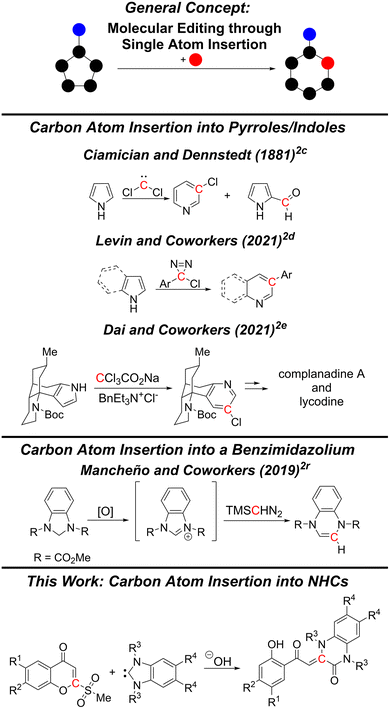 |
| Scheme 1 Selected previous works and this work. | |
Recent work by Levin and coworkers has notably improved the classical Ciamician–Dennstedt reaction. In their work, they reported the use of chlorodiazirines as carbene precursors to synthesize 3-arylpyridines and 3-arylquinolines in typically moderate to good yields from pyrroles and indoles, respectively.2d The application of this named reaction in the total syntheses of complanadine A and lycodine by Dai and coworkers demonstrates the great synthetic utility of not only this reaction, but also of single-carbon atom insertion reactions in general.2e
While the majority of the reports on carbon atom insertion reactions into aromatic compounds has focused on pyrroles or indoles, several papers have been published which have expanded the substrate scope.1 In 2019, Mancheño and coworkers provided, to the best of our knowledge, the only example of a ring expansion of a benzimidazolium through the insertion of a carbon atom from an external molecule.2r While their report is mostly dedicated to the synthesis of benzo[b]azepines from hydroquinolines through a carbon atom insertion reaction using TMSCHN2 as the external carbon atom source, they demonstrated that an in situ generated benzimidazolium could also undergo ring expansion under the same conditions. This transformation is particularly notable as it stands in stark contrast to the typical ring expansion reactions of benzimidazoliums, where the introduced atom originates from the parent molecule and not from an external molecule.7
Herein, we report a reaction between benzimidazolium-derived N-heterocyclic carbenes (NHCs) and 2-(methylsulfonyl)chromones where the carbon atom at position 2 of the chromone is inserted into the NHC to yield 3,4-dihydroquinoxalin-2(1H)-ones. This class of quinoxalinones is particularly attractive due to the range of biologically active derivatives which have been reported, including anticancer,8 analgesic,9 eyes absent homologue 2 (Eya2; promising therapeutic target for cancer) inhibitory,10 and c-Jun N-terminal kinase 3 (JNK3; therapeutic target for Alzheimer's disease) inhibitory compounds11 (Fig. 1).
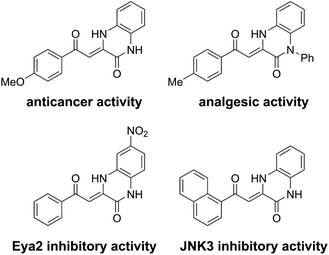 |
| Fig. 1 Examples of biologically active compounds containing the 3-methylidene-3,4-dihydroquinoxalin-2(1H)-one motif. | |
Results and discussion
Over the course of our laboratory's research into NHC-catalyzed reactions, we observed an unexpected side product when 2-(methylsulfonyl)chromone 1a and 1,3-dimethylbenzimidazolium iodide 2a were applied in the same reaction. The X-ray structure of this side product showed it to be quinoxalinone 3a (Fig. 2). It was observed that the carbon atom at position 2 of the chromone was inserted into the benzimidazolium and that there was an additional oxygen atom not from the original chromone structure.
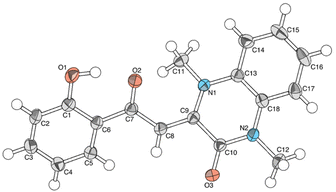 |
| Fig. 2 X-ray crystallographic analysis of 3a. | |
Two key interpretations were made from this structure. First, the construction of the quinoxalinone is likely initiated by a direct substitution reaction between chromone 1a and the NHC. Second, residual water in the solvent was likely necessary for this transformation to occur. Based on these interpretations, a reaction mechanism was postulated as follows (Scheme 2).
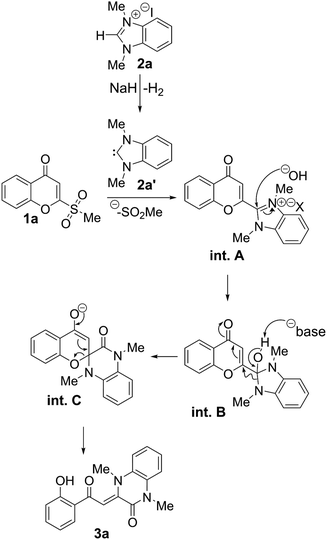 |
| Scheme 2 Proposed mechanism. | |
First, deprotonation of the benzimidazolium salt 2a by a base (NaH in this case) leads to the formation of the NHC 2a′, which undergoes a substitution reaction with chromone 1a to generate the chromonylbenzimidazolium salt intermediate int. A. A hydroxide anion (generated in situ by deprotonation of the residual water by NaH) attacks int. A at the iminium moiety to form int. B. Deprotonation of the hydroxyl group on int. B leads to the formation of the amide moiety and the introduction of the carbon atom at position 2 of the chromone into the benzimidazole core to form the spiro intermediate int. C. Reformation of the α,β-unsaturated structure opens the spiropyran ring in int. C to afford the final quinoxalinone 3a.
Based on this mechanism, a two-step reaction to synthesize quinoxalinones from 2-(methylsulfonyl)chromones 1 and benzimidazoliums was designed. First, the chromone and benzimidazolium salt would be reacted together with 2 equivalents of NaH. After complete consumption of the starting chromone is observed by thin-layer chromatography, the reaction mixture would then be treated with an aqueous solution of NaOH in the second step to initiate the carbon atom insertion sequence, which would afford the desired quinoxalinones.
Chromone 1a and NHC precursor 2a were chosen as the substrates for the initial test reaction and optimization of the reaction conditions. The first experiment was performed in DMF at 25 °C at 0.3 mmol scale to afford quinoxalinone 3a in 28% yield (Table 1, entry 1). Decreases in temperature of step 2 down to 5 °C improved yields up to 75% (entries 2–5). At 0 °C, a 54% yield was obtained (entry 6). An increase in the temperature of step 2 had a negative effect on the yield, providing 3a in only 20% yield (entry 7).
Table 1 Screening of reaction conditions
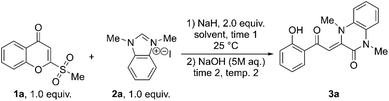
|
Entrya |
Solvent |
Time 1 (h) |
Time 2 (h) |
Temp. 2 (°C) |
Yield (%) |
Entries 1–10 and 12: 0.3 mmol scale.
NMP = N-methyl-2-pyrrolidone.
1.0 mmol scale.
Step 1 temp: 70 °C.
|
1 |
DMF |
3 |
18 |
25 |
28 |
2 |
DMF |
3 |
18 |
20 |
42 |
3 |
DMF |
3 |
18 |
15 |
54 |
4 |
DMF |
3 |
18 |
10 |
72 |
5 |
DMF |
3 |
23 |
5 |
75 |
6 |
DMF |
3 |
24 |
0 |
54 |
7 |
DMF |
3 |
18 |
50 |
20 |
8 |
MeCN |
4 |
18 |
5 |
61 |
9 |
Dioxane |
24 |
48 |
5 |
61 |
10 |
NMPb |
24 |
48 |
5 |
99 |
11c |
NMP |
24 |
24 |
5 |
94 |
12d |
NMP |
1 |
18 |
5 |
44 |
Solvents other than DMF were then tested. 3a was obtained in 61% yield when acetonitrile or 1,4-dioxane was used (entries 8 and 9). The use of NMP provided the best result with a 99% yield of 3a (entry 10). An increased reaction scale from 0.3 mmol to 1.0 mmol had no significant effect on the yield, as 3a was obtained in 94% yield (entry 11). An attempt to speed up the first step by heating at 70 °C resulted in a decreased yield (44%) of 3a (entry 12).
Other substrates—imidazolium, triazolium, thiazolium, and benzothiazolium salts—were tested, however, no corresponding carbon-insertion products were detected. While the majority of the reactions generated complex mixtures, the use of 3-methylbenzothiazolium iodide 2b provided the S,N-keteneacetal 3b′ in 28% yield (Scheme 3).
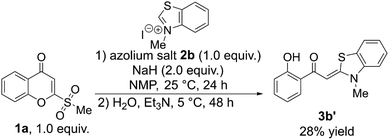 |
| Scheme 3 Synthesis of the unexpected product 3b′. | |
Other benzimidazolium salts were then tested (Scheme 4). 1,3-Dibenzylbenzimidazolium iodide provided the corresponding product 3c in 54% yield. By contrast, more sterically demanding 1,3-diisopropylbenzimidazolium bromide had a significant negative impact on the reaction, as no corresponding product was observed. Both 5,6-dichloro-1,3-dimethylbenzimidazolium iodide and 5,6-dimethoxy-1,3-dimethylbenzimidazolium iodide provided the corresponding products 3d and 3e in high yields (81% and 78%, respectively). However, when 4,7-dimethoxy-1,3-dimethylbenzimidazolium iodide was tested in the reaction, a complex mixture of products was obtained. An unexpected quinoxalinone (4a), which bears two identical substituents at position 3, originating from two molecules of 1a, was isolated in 25% yield when 1-benzyl-3-methylbenzimidazolium iodide was used (a possible mechanism for the formation of 4a is described in the ESI, Scheme S1†).
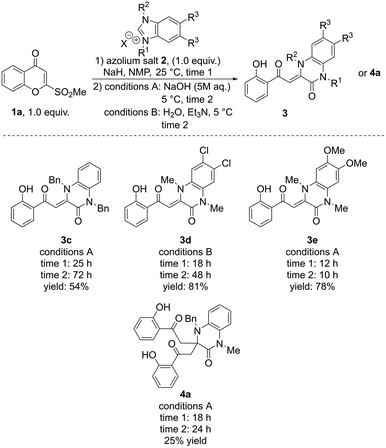 |
| Scheme 4 Screening of benzimidazolium salts. Full screening scope can be viewed in the ESI.† | |
When N-phenyl-substituted benzimidazolium salts were applied in this reaction, no desired quinoxalinone products were detected. 1-Methyl-3-phenylbenzimidazolium iodide 2c afforded chromeno[2,3-b]phenazine 4b in 94% yield (Scheme 5). The structure of 4b was confirmed by X-ray analysis. Although the mechanism is currently not elucidated, the C13 (or C5a) of 4b is likely transferred from the C2 of the benzimidazolium (the carbene center),12 indicating two molecules of each substrate are involved in the formation. It appears that the N-phenyl substituent changes the electronic properties of the desired quinoxalinone product, which leads to the occurrence of additional reactions. When 1-benzyl-3-phenylbenzimidazolium bromide 2d was used, benzimidazol-2-one 4c was obtained as the major product in 55% yield along with a complex mixture of products. The extra steric bulk of the benzyl group of 2d in comparison with 2c likely prevents the desired transformation from occurring.
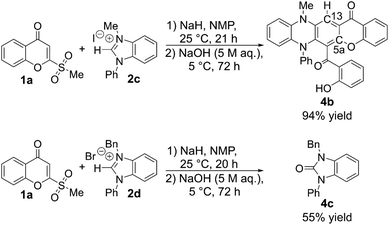 |
| Scheme 5 Application of N-phenyl-substituted benzimidazolium salts. | |
Our attention next turned to the screening of chromone substrates in the reaction with 2a (Scheme 6). Substituents at position 6 of the chromone were first tested. A chromone with a methyl group provided the corresponding product 3f in 45% yield. With the introduction of halogen atoms, milder conditions were needed to obtain moderate to high yields. The use of a mixture of H2O and Et3N in the second step in place of NaOH allowed for the synthesis of chloro-, bromo-, and fluoro-substituted products (3g, 3h, and 3i, respectively) in moderate to high yields (57%, 80%, and 46%, respectively). An electron donating methoxy group at position 6 of the chromone was also well tolerated, with the corresponding product 3j being obtained in 73% yield. Chromones with a chloro or methoxy group at position 7 could also be applied to this reaction affording quinoxalinones 3k and 3l in good yields (74% and 85%, respectively). The application of a naphthyl-fused chromone provided only a complex mixture of products.
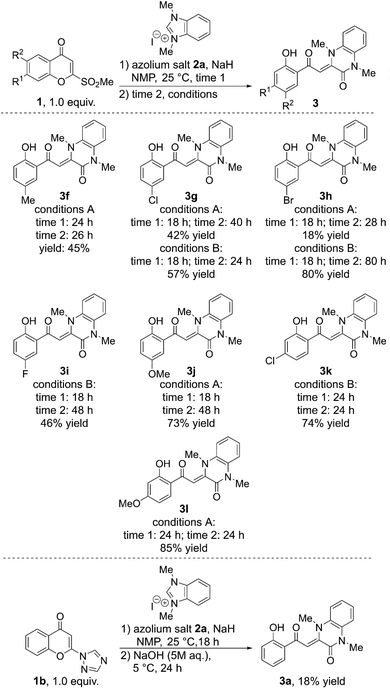 |
| Scheme 6 Screening of chromone substrates. Conditions A: NaOH, 5 °C; conditions B: H2O, Et3N (2 equivalents), 5 °C. | |
To investigate the influence of the substituents at position 3, chromones with a 3-methyl or 3-chloro group were applied to the reaction, however, no desired quinoxalinones were isolated. Additionally, although the yield of 3a was low (18%), a 1,2,4-triazolyl group13 also functioned as a leaving group at position 2 instead of the methylsulfonyl group.
Conclusion
In summary, we have successfully developed a method of synthesizing 3,4-dihydroquinoxalin-2(1H)-ones via a carbon atom insertion reaction. In situ generated NHCs react with 2-(methylsulfonyl)chromones, which presumably generates the key chromonylbenzimidazolium intermediates. The subsequent addition of an aqueous NaOH solution or a H2O/Et3N mixture initiates a ring expansion where the carbon atom located at position 2 of the chromone is inserted into the imidazole ring to afford the quinoxalinones. The benzimidazole framework appeared to be a key to this reaction, since other NHC precursor salts provided no desired products. A novel heteropentacycle 4b was unexpectedly, but efficiently, constructed in the reaction with 1-methyl-3-phenylbenzimidazolium iodide. The pentacycle was likely constructed from two molecules of the chromone and two molecules of the derived NHC. However, for one of the NHC molecules, only the C2 atom seems to be transferred into the product. Further studies into how this polycyclic compound was formed are currently underway. This work demonstrates the potential of NHCs as substrates to provide highly functionalized products in an atom-economic manner via carbon atom insertion reactions.
Author contributions
F. K. conducted the initial substrate scope experiments and collected the NMR data for compound 1c. J. S. L. conducted the reaction optimization and additional substrate scope experiments, purification, and measurement of the characterization data (except X-ray). N. S. conducted the X-ray diffraction measurements and recrystallized compound 4b. J. S. L. and Y. S. wrote the manuscript. Y. S. conceptualized the project.
Conflicts of interest
There are no conflicts to declare.
Note added after first publication
This article replaces the version published on 14th November 2023, which contained errors in the footnotes for Table 1.
Acknowledgements
We would like thank Sophia University for financial support and Ms Emiko Okano of the Faculty of Science and Technology, Sophia University, for mass spectrometry measurements. We also thank Mr Vincent Rinaolo, a graduate student of the Department of Chemistry, Princeton University, for proofing the manuscript and Mohammed Mahdaly and Cai Zhaoyu of the Faculty of Science and Technology, Sophia University, for their assistance.
References
-
(a) J. Jurczyk, J. Woo, S. F. Kim, B. D. Dherange, R. Sarpong and M. D. Levin, Single-Atom Logic for Heterocycle Editing, Nat. Synth., 2022, 1, 352–364 CrossRef PubMed;
(b) C. Hui, Z. Wang, S. Wang and C. Xu, Molecular Editing in Natural Product Synthesis, Org. Chem. Front., 2022, 9, 1451–1457 RSC;
(c) B. W. Joynson and L. T. Ball, Skeletal Editing: Interconversion of Arenes and Heteroarenes, Helv. Chim. Acta, 2023, 106, e202200182 CrossRef CAS.
-
(a) E. Beckmann, Zur Kenntniss Der Isonitrosoverbindungen, Ber. Dtsch. Chem. Ges., 1886, 19, 988–993 CrossRef;
(b) For a review of Baeyer-Villiger oxidations, see: M. Renz and B. Meunier, 100 Years of Baeyer–Villiger Oxidations, Eur. J. Org. Chem., 1999, 1999, 737–750 CrossRef;
(c) G. L. Ciamician and M. Dennstedt, Ueber Die Einwirkung Des Chloroforms Auf Die Kaliumverbindung Pyrrols, Ber. Dtsch. Chem. Ges., 1881, 14, 1153–1163 CrossRef;
(d) B. D. Dherange, P. Q. Kelly, J. P. Liles, M. S. Sigman and M. D. Levin, Carbon Atom Insertion into Pyrroles and Indoles Promoted by Chlorodiazirines, J. Am. Chem. Soc., 2021, 143, 11337–11344 CrossRef CAS;
(e) D. Ma, B. S. Martin, K. S. Gallagher, T. Saito and M. Dai, One-Carbon Insertion and Polarity Inversion Enabled a Pyrrole Strategy to the Total Syntheses of Pyridine-Containing Lycopodium Alkaloids: Complanadine A and Lycodine, J. Am. Chem. Soc., 2021, 143, 16383–16387 CrossRef CAS PubMed;
(f) H. Saito, S. Otsuka, K. Nogi and H. Yorimitsu, Nickel-Catalyzed Boron Insertion into the C2–O Bond of Benzofurans, J. Am. Chem. Soc., 2016, 138, 15315–15318 CrossRef CAS;
(g) H. Lyu, I. Kevlishvili, X. Yu, P. Liu and G. Dong, Boron Insertion into Alkyl Ether Bonds via Zinc/Nickel Tandem Catalysis, Science, 2021, 372, 175–182 CrossRef CAS;
(h) J. J. Clarke, P. Eisenberger, S. S. Piotrkowski and C. M. Crudden, Azaborines: Synthesis and Use in the Generation of Stabilized Boron-Substituted Carbocations, Dalton Trans., 2018, 47, 1791–1795 RSC;
(i) J. C. Reisenbauer, O. Green, A. Franchino, P. Finkelstein and B. Morandi, Late-Stage Diversification of Indole Skeletons through Nitrogen Atom Insertion, Science, 2022, 377, 1104–1109 CrossRef CAS PubMed;
(j) P. Amice, L. Blanco and J. M. Conia, Enol Silyl Ethers and Their Use for the Synthesis of α-Halo-α,β-Unsaturated Carbonyl Compounds, Synthesis, 1976, 196–197 CrossRef CAS;
(k) W. E. Parham, R. W. Soeder, J. R. Throckmorton, K. Kuncl and R. M. Dodson, Reactions of Enol Ethers with Carbenes. V. Rearrangements of Dihalocyclopropanes Derived from Six-, Seven-, and Eight-Membered Cyclic Enol Ethers, J. Am. Chem. Soc., 1965, 87, 321–328 CrossRef CAS;
(l) M. Ohno, A New Ring-Expansion Reaction from Enamine and Dichlorocarbene, Tetrahedron Lett., 1963, 4, 1753–1755 CrossRef;
(m) R. C. De Selms, A New Method of Ring Expansion, Tetrahedron Lett., 1966, 7, 1965–1968 CrossRef;
(n) B. W. Joynson, G. R. Cumming and L. T. Ball, Photochemically Mediated Ring Expansion of Indoles and Pyrroles with Chlorodiazirines: Synthetic Methodology and Thermal Hazard Assessment, Angew. Chem., Int. Ed., 2023, 62, e2023050 CrossRef;
(o) K. Nishihara, T. Kurogi and H. Yorimitsu, Aromatic metamorphosis of an indole into 2-quinolone, dihydrobenzazasiline, and dihydrobenzazagermine, ARKIVOC, 2023, 2, 202312017 Search PubMed;
(p) B. Wang, M. Li, S. Xu, H. Song and B. Wang, A General Synthetic Route to 6,6-Substituted-6H-dibenzo[b,d]pyrans from Dibenzofuran, J. Org. Chem., 2006, 71, 8291–8293 CrossRef CAS PubMed;
(q) M. Yus, F. Foubelo and J. V. Ferrández, Stereoselective Reductive Opening of 2,3-Benzofuran − A Two-Step Synthesis of 2H-Chromenes Including Deoxycordiachromene, Eur. J. Org. Chem., 2001, 2001, 2809–2813 CrossRef;
(r) S. Stockerl, T. Danelzik, D. G. Piekarski and O. García Mancheño, Mild, Metal-Free Oxidative Ring-Expansion Approach for the Synthesis of Benzo[b]azepines, Org. Lett., 2019, 21, 4535–4539 CrossRef CAS PubMed.
-
(a) X. Yu, J. Hu, Z. Shen, H. Zhang, J.-M. Gao and W. Xie, Stereospecific Construction of Contiguous Quaternary All–carbon Centers by Oxidative Ring Contraction, Angew. Chem., Int. Ed., 2017, 56, 350–353 CrossRef CAS;
(b) D. Ng, Z. Yang and M. A. Garcia-Garibay, Total Synthesis of (±)-Herbertenolide by Stereospecific Formation of Vicinal Quaternary Centers in a Crystalline Ketone, Org. Lett., 2004, 6, 645–647 CrossRef CAS;
(c) A. Natarajan, D. Ng, Z. Yang and M. A. Garcia-Garibay, Parallel Syntheses of (+)- and (−)-α-Cuparenone by Radical Combination in Crystalline Solids, Angew. Chem., Int. Ed., 2007, 46, 6485–6487 CrossRef CAS;
(d) J. B. Roque, Y. Kuroda, L. T. Göttemann and R. Sarpong, Deconstructive Diversification of Cyclic Amines, Nature, 2018, 564, 244–248 CrossRef CAS;
(e) C. Zippel, J. Seibert and S. Bräse, Skeletal Editing—Nitrogen Deletion of Secondary Amines by Anomeric Amide Reagents, Angew. Chem., Int. Ed., 2021, 60, 19522–19524 CrossRef CAS;
(f) S. H. Kennedy, B. D. Dherange, K. J. Berger and M. D. Levin, Skeletal Editing through Direct Nitrogen Deletion of Secondary Amines, Nature, 2021, 593, 223–227 CrossRef CAS;
(g) H. Qin, W. Cai, S. Wang, T. Guo, G. Li and H. Lu, N–atom Deletion in Nitrogen Heterocycles, Angew. Chem., Int. Ed., 2021, 60, 20678–20683 CrossRef CAS;
(h) C. Hui, L. Brieger, C. Strohmann and A. P. Antonchick, Stereoselective Synthesis of Cyclobutanes by Contraction of Pyrrolidines, J. Am. Chem. Soc., 2021, 143, 18864–18870 CrossRef CAS;
(i) Z.-C. Cao and Z.-J. Shi, Deoxygenation of Ethers to Form Carbon–Carbon Bonds via Nickel Catalysis, J. Am. Chem. Soc., 2017, 139, 6546–6549 CrossRef CAS.
-
(a) A. R. Fout, B. C. Bailey, J. Tomaszewski and D. J. Mindiola, Cyclic Denitrogenation of N-Heterocycles Applying a Homogeneous Titanium Reagent, J. Am. Chem. Soc., 2007, 129, 12640–12641 CrossRef CAS;
(b) T. Morofuji, K. Inagawa and N. Kano, Sequential Ring-Opening and Ring-Closing Reactions for Converting Para-Substituted Pyridines into Meta-Substituted Anilines, Org. Lett., 2021, 23, 6126–6130 CrossRef CAS PubMed;
(c) S. C. Patel and N. Z. Burns, Conversion of Aryl Azides to Aminopyridines, J. Am. Chem. Soc., 2022, 144, 17797–17802 CrossRef CAS PubMed;
(d) T. Morofuji, S. Nagai, A. Watanabe, K. Inagawa and N. Kano, Streptocyanine as an Activation Mode of Amine Catalysis for the Conversion of Pyridine Rings to Benzene Rings, Chem. Sci., 2023, 14, 485–490 RSC.
- K. R. Campos, P. J. Coleman, J. C. Alvarez, S. D. Dreher, R. M. Garbaccio, N. K. Terrett, R. D. Tillyer, M. D. Truppo and E. R. Parmee, The Importance of Synthetic Chemistry in the Pharmaceutical Industry, Science, 2019, 363, eaat0805 CrossRef CAS PubMed.
- For a description of Ciamician-Dennstedt rearrangement and its limitations see:
Comprehensive Organic Name Reactions and Reagents, ed. Z. Wang, John Wiley & Sons, Hoboken, 2010, 646–648 Search PubMed.
-
(a) I. R. Ager, A. C. Barnes, G. W. Danswan, P. W. Hairsine, D. P. Kay, P. D. Kennewell, S. S. Matharu, P. Miller and P. Robson, Synthesis and Oral Antiallergic Activity of Carboxylic Acids Derived from Imidazo[2,1-c,][1,4]Benzoxazines, Imidazo[1,2-a]Quinolines, Imidazo[1,2-a]Quinoxalines, Imidazo[1,2-a]Quinoxalinones, Pyrrolo[1,2-a]Quinoxalinones, Pyrrolo[2,3-a]Quinoxalinones, and Imidazo[2,1-b]Benzothiazoles, J. Med. Chem., 1988, 31, 1098–1115 CrossRef CAS;
(b) Y. Matsuda, M. Yamashita, K. Takahashi, S. Ide, K. Torisu and K. Furuno, Thermal Reaction of Benzimidazolium N-Allylides, Heterocycles, 1992, 33, 295–302 CrossRef CAS;
(c) H. Quast, S. Ivanova, E.-M. Peters, K. Peters and H. G. V. Schnering, Ring Expansion of Heterocyclic Ketene N,X-Acetals and 2-Alkylidenedihydroindoles with Methanesulphonyl Azide by [3 + 2] Cycloaddition and Subsequent Extrusion of Molecular Nitrogen, Liebigs Ann., 1996, 1996, 1541–1549 CrossRef;
(d) H. Quast and S. Ivanova, Ring Expansion vs. Cleavage of the Exocyclic Double Bond of 2-Cycloalkylidenedihydrobenzothiazoles and -Benzimidazoles by Methanesulfonyl Azide, Eur. J. Org. Chem., 2000, 1229–1233 CrossRef CAS;
(e) A. Nicolescu, E. Georgescu, F. Dumitrascu, F. Georgescu, F. Teodorescu, C. Draghici, M. R. Caira and C. Deleanu, Exocyclic Enamines of Pyrrolo[1,2-a]Quinoxalines Generated by 1,3-Dipolar Cycloaddition Reactions of Benzimidazolium Ylides to Activated Alkynes, Rev. Chim., 2020, 71, 197–209 CrossRef CAS;
(f) E. Georgescu, A. Nicolescu, F. Georgescu, F. Teodorescu, D. Marinescu, A.-M. Macsim and C. Deleanu, New Highlights of the Syntheses of Pyrrolo[1,2-a]Quinoxalin-4-Ones, Beilstein J. Org. Chem., 2014, 10, 2377–2387 CrossRef;
(g) E. Georgescu, A. Nicolescu, F. Georgescu, F. Teodorescu, S. Shova, A. T. Marinoiu, F. Dumitrascu and C. Deleanu, Fine Tuning the Outcome of 1,3-Dipolar Cycloaddition Reactions of Benzimidazolium Ylides to Activated Alkynes, Tetrahedron, 2016, 72, 2507–2520 CrossRef CAS;
(h) C. Moldoveanu, G. Zbancioc, D. Mantu, D. Maftei and I. Mangalagiu, The Cycloaddition of the Benzimidazolium Ylides with Alkynes: New Mechanistic Insights, PLoS One, 2016, 11, e0156129 CrossRef PubMed;
(i) M. Caira, F. Dumitrascu, D. Dumitrescu, E. Georgescu and C. Draghici, A New Synthesis of Pyrroles from Benzimidazolium N-Cyanomethyl Ylides and Alkyne Dipolarophiles, Synlett, 2017, 28, 2241–2246 CrossRef;
(j) D. Diaconu, D. Amăriucăi-Mantu, V. Mangalagiu, V. Antoci, G. Zbancioc and I. I. Mangalagiu, Ultrasound Assisted Synthesis of Hybrid Quinoline-Imidazole Derivatives: A Green Synthetic Approach, RSC Adv., 2021, 11, 38297–38301 RSC.
- J. Petronijević, N. Janković, T. P. Stanojković, N. Joksimović, N. Đ. Grozdanić, M. Vraneš, A. Tot and Z. Bugarčić, Biological Evaluation of Selected 3,4–dihydro–2(1H)–quinoxalinones and 3,4–dihydro–1,4–benzoxazin–2–ones: Molecular Docking Study, Arch. Pharm., 2018, 351, 1700308 CrossRef PubMed.
- I. V. Mashevskaya, R. R. Makhmudov, G. A. Aleksandrova, O. V. Golovnina, A. V. Duvalov and A. N. Maslivets, Synthesis and Study of the Antibacterial and Analgesic Activity of 3-Acyl-1,2,4,5-tetrahydro-[1,2-a]quinoxaline-1,2,4-triones, Pharm. Chem. J., 2001, 35, 196–198 CrossRef CAS.
- H. Park, S.-K. Jung, K. R. Yu, J. H. Kim, Y.-S. Kim, J. H. Ko, B. C. Park and S. J. Kim, Structure-Based Virtual Screening Approach to the Discovery of Novel Inhibitors of Eyes Absent 2 Phosphatase with Various Metal Chelating Moieties: Virtual Screening of Eya2 Inhibitors, Chem. Biol. Drug Des., 2011, 78, 642–650 CrossRef CAS.
- X. Dou, H. Huang, Y. Li, L. Jiang, Y. Wang, H. Jin, N. Jiao, L. Zhang, L. Zhang and Z. Liu, Multistage Screening Reveals 3-Substituted Indolin-2-One Derivatives as Novel and Isoform-Selective c-Jun N-Terminal Kinase 3 (JNK3) Inhibitors: Implications to Drug Discovery for Potential Treatment of Neurodegenerative Diseases, J. Med. Chem., 2019, 62, 6645–6664 CrossRef CAS.
-
(a) M. Kamitani, B. Nakayasu, H. Fujimoto, K. Yasui, T. Kodama and M. Tobisu, Single–Carbon Atom Transfer to α,β-Unsaturated
Amides from N-Heterocyclic Carbenes, Science, 2023, 379, 484–488 CrossRef CAS;
(b) H. Fujimoto, B. Nakayasu and M. Tobisu, Synthesis of γ-Lactams from Acrylamides by Single-Carbon Atom Doping Annulation, J. Am. Chem. Soc., 2023, 145, 19518–19522 CrossRef CAS.
- R. Samanta, R. Narayan, J. O. Bauer, C. Strohmann, S. Sievers and A. P. Antonchick, Oxidative Regioselective Amination of Chromones Exposes Potent Inhibitors of the Hedgehog Signaling Pathway, Chem. Commun., 2015, 51, 925–928 RSC.
Footnote |
† Electronic supplementary information (ESI) available: Full experimental procedures, substrate scope and characterization data. CCDC 2291928–2291932. For ESI and crystallographic data in CIF or other electronic format see DOI: https://doi.org/10.1039/d3qo01579c |
|
This journal is © the Partner Organisations 2024 |