DOI:
10.1039/D4QM00590B
(Research Article)
Mater. Chem. Front., 2024,
8, 3150-3156
Highly selective separation of toluene and methylcyclohexane based on nonporous adaptive crystals of hybrid[3]arene†
Received
12th July 2024
, Accepted 21st August 2024
First published on 28th August 2024
Introduction
The separation of toluene (Tol) and methylcyclohexane (MCH) is crucial in the domains of chemistry and petrochemistry.1 Tol is an organic compound that is used as a raw material for various chemicals, including pesticides, dyes and synthetic resins.2,3 MCH is obtained by hydrogenation of Tol, which is widely used as an effective solvent in the manufacture of pharmaceutical intermediates, nylons, and varnishes.4,5 However, due to incomplete hydrogenation, Tol will be mixed with MCH, resulting in a mixture of Tol and MCH. In order to obtain high purity of MCH, Tol needs to be separated from the mixture of Tol and MCH.6 Extractive and azeotropic distillations are the primary industrial techniques used to separate the mixture containing Tol and MCH.7 However, because of the close boiling points of Tol (383.75 K) and MCH (374.15 K), these methods require considerable energy, complicated processes, and high operating costs.8–10 Developing economically viable methods for separating the mixture of Tol and MCH can reduce global energy consumption. Therefore, the development of novel and energy-efficient separation technologies is essential for the effective separation of Tol and MCH.
Adsorptive separation methods using porous materials offer an economical alternative to traditional separation techniques.11–17 Zeolites, porous organic cages, metal–organic frameworks (MOFs), and covalent organic frameworks (COFs) have enabled the cost-effective and energy-efficient separation of a wide range of aromatic and aliphatic compounds.18–28 Hitherto, the adsorptive separation of Tol and MCH has been mainly achieved using porous materials such as MOFs and COFs.29,30 However, there are still some drawbacks associated with the utilization of porous materials in practical applications. For example, MOFs exhibit comparatively poor chemical stability, resulting in low reusability.31–34 While the stability of COFs is limited by the formation of reversible covalent bonds required for crystallization.35 Therefore, there is an urgent need to develop adsorption materials with simple synthesis process and easy recovery for the separation of Tol and MCH.
In recent years, nonporous adaptive crystals (NACs) have performed well in separating hydrocarbons.36–43 Unlike traditional porous materials, NACs are initially nonporous.44–50 However, as the guest molecules are induced, NACs form intrinsic or extrinsic pores to load guest molecules, accompanied by crystal structure transformation.51–65 Due to their distinctive characteristics, NACs are employed as new supramolecular adsorptive separation materials.66–69 Hybrid[n]arenes represent an innovative category of supramolecular hosts with the potential to be designed as NACs materials.70,71 The unique structural properties and versatility of these NACs materials make them suitable for applications in the field of adsorptive separation.72
Hybrid[3]arene H, a novel and important hybrid[n]arenes family member, has been synthesized and designed into NACs material for the first time by our group.73 In the previous report, we successfully achieved the separation of benzene and cyclohexane using NACs of H (Hα) as the adsorptive separation materials.74 Additionally, Hα have shown potential for adsorptive separation of other important hydrocarbons.75,76 Herein, we discovered that Hα were capable of effectively separating Tol and MCH (Fig. 1). Specifically, Hα efficiently separated Tol from the mixture of Tol and MCH (v
:
v = 1
:
1) with a purity of 100% via vapor–solid adsorption and a purity of 98.98% via liquid–solid adsorption. Additionally, the adsorbed Tol could be effectively removed by heating, thus recovering Hα without loss of adsorption properties.
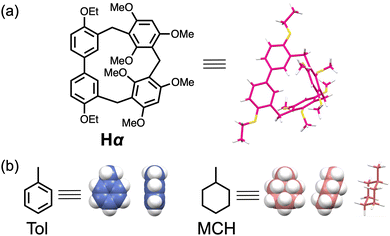 |
| Fig. 1 Chemical structures of: (a) NACs of hybrid[3]arene H (Hα); (b) toluene (Tol) and methylcyclohexane (MCH). | |
Results and discussion
Hybrid[3]arene H was synthesized and activated using previous methods.73 To characterize NACs of H (Hα), 1H NMR and thermogravimetric analysis (TGA) were employed (Fig. S1 and S2, ESI†), confirming the removal of the solvent. Hα were shown to be crystalline analyzed by powder X-ray diffraction (PXRD) (Fig. S3, ESI†).
Single-component time-dependent vapor–solid adsorption experiments were carried out to explore the ability of Hα to capture Tol or MCH vapor. The results revealed a gradual increase in the adsorption of Tol vapor by Hα over time, reaching the saturation point within about 12 h (Fig. 2a). According to the 1H NMR, the amount of Tol vapor adsorbed by Hα was determined to be 0.8 Tol/Hα, whereas the amount of MCH vapor adsorbed by Hα was negligible (Fig. S4 and S5, ESI†). TGA further demonstrated that Hα had the capability to adsorb Tol vapor, with a weight loss of 12.22% below 150 °C, corresponding to 0.9 Tol/Hα (Fig. S6, ESI†). However, no weight loss of Hα was observed until 300 °C after adsorption of MCH vapor (Fig. S7, ESI†). Based on these results, it could be inferred that Hα adsorbed Tol vapor but not MCH vapor. Besides, the PXRD pattern of Hα apparently changed after the capture of Tol vapor (Fig. 2b, III), indicating that Hα adsorbed Tol vapor to form a novel guest-loaded crystal structure. In contrast, the PXRD pattern of Hα remained unchanged following the adsorption of MCH vapor (Fig. 2b, II). The results validated the adsorption ability of Hα to Tol vapor.
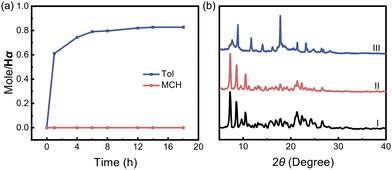 |
| Fig. 2 (a) Time-dependent vapor–solid adsorption of Hα for single-component Tol or MCH vapor. (b) PXRD patterns of: (I) origin Hα; (II) Hα after adsorption of MCH vapor; (III) Hα after adsorption of Tol vapor. | |
Single crystal X-ray diffraction (SCXRD) analysis revealed the host–guest interaction between H and Tol. Single crystal of Tol-loaded H (Tol@H) was obtained through slow volatilization of the Tol in which H was dissolved at room temperature. From the crystal structure of Tol@H, H molecules were arranged in infinite channels. The Tol molecule was located between two H molecules, rather than within the cavity of H, forming a host–guest complex with H in a ratio of 1
:
1 (Fig. 3a–c). It is noteworthy that the primary driving force for the formation of the host–guest complex after Tol adsorption was C–H⋯π and C–H⋯O interactions between H molecule and Tol molecule (Fig. 3d and Fig. S8, ESI†). Moreover, the PXRD pattern of Hα after the adsorption of Tol was identical to that simulated from the crystal structure of Tol@H (Fig. S9, ESI†), indicating that the crystal structure of Hα changed to Tol@H. In addition, visual studies of weak intermolecular interactions based on density-functional theory calculations revealed that the host–guest complex of Tol@H was stabilized by C–H⋯π and C–H⋯O interactions (Fig. S10, ESI†). The calculation result was consistent with the host–guest interaction between H and Tol obtained through SCXRD analysis.
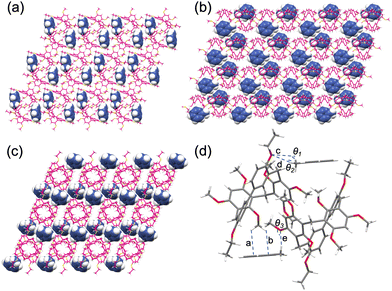 |
| Fig. 3 Single crystal structure of Tol@H at different views: (a) along the a-axis; (b) along the b-axis; (c) along the c-axis. (d) Illustration of C–H⋯π and C–H⋯O interactions between H and Tol, H–π-plane distances: a = 2.921 Å, b = 2.784 Å; H–O distances: c = 2.660 Å; d = 2.882 Å; e = 2.914 Å; C–H⋯O angles: θ1 = 108.40°; θ2 = 93.79°; θ3 =149.74°. See ESI† for the structural detail and CCDC number. | |
Hirshfeld surface analysis further demonstrated the host–guest interaction in Tol@H.77 The two-dimensional fingerprint plots showed the relative contributions of various interactions in the crystal structure of Tol@H. The results revealed that the C–H⋯π interaction contributed the most (over 37%), which ensured the stability of Tol@H (Fig. 4a and b). In addition, the contribution of the C–H⋯O interaction was 5.6% (Fig. 4c). These results suggested that the interaction between Tol and H was mainly stabilized by C–H⋯π and C–H⋯O interactions (Fig. 4d).
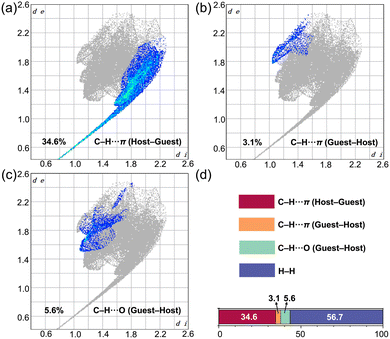 |
| Fig. 4 (a)–(c) Two-dimensional fingerprint plots of Tol@H and the relative contributions of various interactions in the crystal structure of Tol@H. (d) Percentage contributions of various interactions in Tol@H based on Hirshfeld surface area analysis. | |
Given the adsorption capacity of Hα, we wondered whether Hα could effectively separate the mixture of Tol and MCH. Time-dependent vapor–solid adsorption experiments were conducted on the vapor mixture of Tol and MCH (v
:
v = 1
:
1) by Hα. Over a period of time, the amount of Tol vapor adsorbed by Hα steadily increased, reaching the saturation point after about 20 h. However, the MCH vapor adsorbed by Hα could be negligible (Fig. 5a). These results agreed with the single-component adsorption experiments in which Hα captured Tol vapor but not MCH vapor. At the saturation point, the amount of Tol adsorbed by Hα was about 0.8 Tol/Hα, as determined by 1H NMR (Fig. S11, ESI†). TGA results indicated a weight loss of 10.62% below 150 °C, and it was determined that one Hα molecule adsorbed 0.8 Tol molecule (Fig. S12, ESI†). Similarly, after exposing Hα to the vapor mixture of Tol and MCH, the resulting PXRD pattern of Hα matched that of Hα adsorbed for Tol vapor (Fig. 5b). Meanwhile, the PXRD pattern was simulated using the single crystal data of Tol@H and subsequently compared with the pattern of Hα following the adsorption of the vapor mixture of Tol and MCH. The finding showed that the two patterns were consistent (Fig. S13, ESI†), suggesting a structure transition from Hα to Tol@H. In addition, head space gas chromatography (HS-GC) verified that Tol vapor was adsorbed by Hα with 100% purity (Fig. 5c and Fig. S14, ESI†), confirming the outstanding selectivity of Hα to Tol vapor. The results suggested that Hα were capable of selectively capturing Tol from the vapor mixture of Tol and MCH (Fig. 5e).
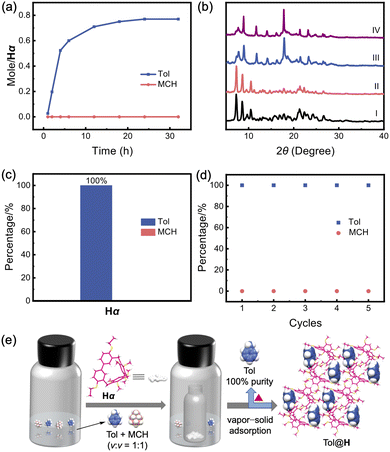 |
| Fig. 5 (a) Time-dependent vapor–solid adsorption of Hα for the vapor mixture of Tol and MCH (v : v = 1 : 1). (b) PXRD patterns of: (I) origin Hα; (II) Hα after adsorption of MCH vapor; (III) Hα after adsorption of Tol vapor; (IV) Hα after adsorption of the vapor mixture of Tol and MCH. (c) Relative uptakes of Tol and MCH vapors adsorbed by Hα after 24 h by head space gas chromatography. (d) Relative uptakes of Tol and MCH vapors adsorbed by Hα after 5 cycles. (e) Schematic diagrams representing the selective adsorption of Tol from a vapor mixture of Tol and MCH (v : v = 1 : 1) using Hα and the subsequent release of Tol upon heating. | |
The recyclability of adsorbents is crucial to evaluate their suitability in practical industrial processes.78 After heating Tol@H for 2 h, the adsorbed Tol was removed and new crystal was formed. The newly formed crystal was Hα, as confirmed by the PXRD analysis (Fig. S15, ESI†). Moreover, 1H NMR and HS-GC tests confirmed that the newly formed Hα could still selectively capture Tol from the mixture of Tol and MCH without losing performance after recycling for 5 times (Fig. 5d and Fig. S16 and S17, ESI†).
Furthermore, due to the remarkable selective adsorption capacity of Hα for Tol in vapor–solid adsorption studies, we were intrigued by the possibility of Hα effectively separating Tol from the liquid mixture of Tol and MCH. After 60 min of single-component liquid–solid adsorption, the amount of Tol liquid adsorbed by Hα was saturated with 0.9 Tol/Hα (Fig. 6a and Fig. S18, ESI†). However, the amount of MCH liquid adsorbed by Hα was neglected, as proven by 1H NMR (Fig. S19, ESI†), implying that Hα could adsorb Tol liquid but not MCH liquid. Besides, the PXRD pattern of Hα showed no significant change after adsorption of MCH liquid, but there was an evident change after adsorption of Tol liquid (Fig. 6b and Fig. S20, ESI†). This suggested that the adsorption of Tol liquid by Hα resulted in the formation of a new crystal structure.
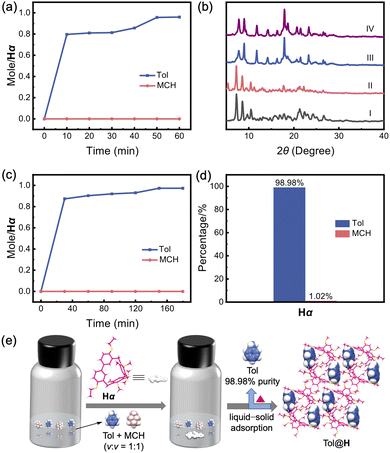 |
| Fig. 6 (a) Time-dependent liquid–solid adsorption of Hα for single-component Tol or MCH liquid. (b) PXRD patterns of: (I) origin Hα; (II) Hα after adsorption of MCH liquid; (III) Hα after adsorption of Tol liquid; (IV) Hα after adsorption of the liquid mixture of Tol and MCH. (c) Time-dependent liquid–solid adsorption of Hα for the liquid mixture of Tol and MCH (v : v = 1 : 1). (d) Relative uptakes of Tol and MCH liquids adsorbed by Hα after 140 min by head space gas chromatography. (e) Schematic diagrams representing the selective adsorption of Tol from a liquid mixture of Tol and MCH (v : v = 1 : 1) using Hα and the subsequent release of Tol upon heating. | |
Subsequently, time-dependent liquid–solid adsorption experiments were conducted on the liquid mixture of Tol and MCH (v
:
v = 1
:
1). The Tol liquid was absorbed by Hα, resulting in a 0.9 Tol/Hα amount at saturation after 140 min. Meanwhile, the MCH liquid adsorbed by Hα was negligible, as evidenced by 1H NMR (Fig. 6c and Fig. S21, ESI†). After exposure to the liquid mixture of Tol and MCH, the PXRD pattern of Hα was consistent with the simulated pattern based on single crystal data of Tol@H (Fig. S22, ESI†), indicating a crystal structure transition from Hα to Tol@H. The HS-GC experiment showed that Hα had good selectivity for Tol liquid, achieving the adsorption purity of 98.98% (Fig. 6d and Fig. S23, ESI†). These experimental results indicated that Hα preferred Tol liquid, demonstrating the high selectivity in liquid–solid adsorption (Fig. 6e).
Conclusions
In summary, for the first time, we have developed a novel approach for separating Tol from both vapor and liquid mixtures of Tol and MCH utilizing nonporous adaptive crystals of hybrid[3]arene H (Hα). Hα can separate Tol from the vapor and liquid mixtures of Tol and MCH (v
:
v = 1
:
1) with purities of 100% and 98.98%, respectively, showing that Hα are excellent materials for adsorption and separation. The selectivity can be attributed to the stable crystal structure formed when the preferential guest molecule, Tol, is adsorbed. In addition, the reversible transformation between the guest-containing structure and the non-guest-containing structure endows Hα with high recyclability. Owing to the straightforward synthesis process, outstanding separation efficiency and excellent recovery property, Hα hold great promise for appliances in chemical industries. In future studies, efforts will be made to develop more applications of Hα for adsorptive separations involving isotopes, configuration isomers, and other complex substances.
Data availability
The data that support the findings of this study are available in the ESI† of this article. Experimental details, NMR spectra, crystallography and other materials. X-ray crystallographic data for Tol@H.
Conflicts of interest
There are no conflicts to declare.
Acknowledgements
This work was supported by the National Natural Science Foundation of China (22101043), the Fundamental Research Funds for the Central Universities (N2205013, N232410019, N2405013), Natural Science Foundation of Liaoning Province (2023-MSBA-068), the Opening Fund of State Key Laboratory of Heavy Oil Processing (SKLHOP202203006) and Northeastern University. Special thanks are due to the instrumental or data analysis from Analytical and Testing, Northeastern University.
References
- D. S. Sholl and R. P. Lively, Seven Chemical Separations to Change the World, Nature, 2016, 532, 435–437 CrossRef PubMed.
- M. Huda, K. Minamisawa, T. Tsukamoto, M. Tanabe and K. Yamamoto, Aerobic Toluene Oxidation Catalyzed by Subnano Metal Particles, Angew. Chem., Int. Ed., 2019, 58, 1002–1006 CrossRef CAS PubMed.
- C.-C. Chen, M. Dai, L. Zhang, J. Zhao, W. Zeng, M. Shi, J.-W. Huang, W. Liu, R.-T. Guo and A. Li, Molecular Basis for a Toluene Monooxygenase to Govern Substrate Selectivity, ACS Catal., 2022, 12, 2831–2839 CrossRef CAS.
- A. A. Taimoor, I. Pitault and F. C. Meunier, Correlation between Deactivation and Pt-Carbonyl Formation during Toluene Hydrogenation Using a H2/CO2 Mixture, J. Catal., 2011, 278, 153–161 CrossRef CAS.
- L. Chen, P. Verma, K. Hou, Z. Qi, S. Zhang, Y.-S. Liu, J. Guo, V. Stavila, M. D. Allendorf, L. Zheng, M. Salmeron, D. Prendergast, G. A. Somorjai and J. Su, Reversible Dehydrogenation and Rehydrogenation of Cyclohexane and Methylcyclohexane by Single-Site Platinum Catalyst, Nat. Commun., 2022, 13, 1092 CrossRef CAS PubMed.
- P. Wang, T. Minegishi, G. Ma, K. Takanabe, Y. Satou, S. Maekawa, Y. Kobori, J. Kubota and K. Domen, Photoelectrochemical Conversion of Toluene to Methylcyclohexane as an Organic Hydride by Cu2ZnSnS4-Based Photoelectrode Assemblies, J. Am. Chem. Soc., 2012, 134, 2469–2472 CrossRef CAS PubMed.
- R. Kumar, R. K. Velamati and S. Kumar, Combustion of Methyl Cyclohexane at Elevated Temperatures to Investigate Burning Velocity for Surrogate Fuel Development, J. Hazard. Mater., 2021, 406, 124627 CrossRef CAS PubMed.
- P. G. Tiverios and V. Van Brunt, Extractive Distillation Solvent Characterization and Shortcut Design Procedure for Methylcyclohexane-Toluene Mixtures, Ind. Eng. Chem. Res., 2000, 39, 1614–1623 CrossRef CAS.
- F. S. Oliveira, A. B. Pereiro, L. P. Rebelo and I. M. Marrucho, Deep Eutectic Solvents as Extraction Media for Azeotropic Mixtures, Green Chem., 2013, 15, 1326–1330 RSC.
- E. Quijada-Maldonado, G. W. Meindersma and A. B. de Haan, Pilot Plant Study on the Extractive Distillation of Toluene–Methylcyclohexane Mixtures Using NMP and the Ionic Liquid [Hmim][TCB] as Solvents, Sep. Purif. Technol., 2016, 166, 196–204 CrossRef CAS.
- B. Smit and T. L. M. Maesen, Molecular Simulations of Zeolites: Adsorption, Diffusion, and Shape Selectivity, Chem. Rev., 2008, 108, 4125–4184 CrossRef CAS PubMed.
- T. A. Makal, J.-R. Li, W. Lu and H.-C. Zhou, Methane Storage in Advanced Porous Materials, Chem. Soc. Rev., 2012, 41, 7761–7779 RSC.
- W. J. Roth, B. Gil, W. Makowski, B. Marszalek and P. Eliášová, Layer Like Porous Materials with Hierarchical Structure, Chem. Soc. Rev., 2016, 45, 3400–3438 RSC.
- X. Meng, H.-N. Wang, S.-Y. Song and H.-J. Zhang, Proton-Conducting Crystalline Porous Materials, Chem. Soc. Rev., 2017, 46, 464–480 RSC.
- Z. Li and Y.-W. Yang, Macrocycle-Based Porous Organic Polymers for Separation, Sensing, and Catalysis, Adv. Mater., 2022, 34, 2107401 CrossRef CAS PubMed.
- Z. Chen, K. O. Kirlikovali, K. B. Idrees, M. C. Wasson and O. K. Farha, Porous Materials for Hydrogen Storage, Chem, 2022, 8, 693–716 CAS.
- J. Li and B. Chen, Flexible Hydrogen-Bonded Organic Frameworks (HOFs): Opportunities and Challenges, Chem. Sci., 2024, 15, 9874–9892 RSC.
- J.-R. Li, R. J. Kuppler and H.-C. Zhou, Selective Gas Adsorption and Separation in Metal–Organic Frameworks, Chem. Soc. Rev., 2009, 38, 1477–1504 RSC.
- Y. Xu, Y. Li, Y. Han, X. Son and J. Yu, A Gallogermanate Zeolite with Eleven-Membered-Ring Channels, Angew. Chem., Int. Ed., 2013, 52, 5501–5503 CrossRef CAS PubMed.
- H. Furukawa, K. E. Cordova, M. O’Keeffe and O. M. Yaghi, The Chemistry and Applications of Metal–Organic Frameworks, Science, 2013, 341, 1230444 CrossRef PubMed.
- P. J. Bereciartua, Á. Cantín, A. Corma, J. L. Jordá, M. Palomino, F. Rey, S. Valencia, E. W. Corcoran, P. Kortunov, P. I. Ravikovitch, A. Burton, C. Yoon, Y. Wang, C. Paur, J. Guzman, A. R. Bishop and G. L. Casty, Control of Zeolite Framework Flexibility and Pore Topology for Separation of Ethane and Ethylene, Science, 2017, 358, 1068–1071 CrossRef CAS PubMed.
- K. Dey, M. Pal, K. C. Rout, S. Kunjattu, A. Das, R. Mukherjee, U. K. Kharul and R. Banerjee, Selective Molecular Separation by Interfacially Crystallized Covalent Organic Framework Thin Films, J. Am. Chem. Soc., 2017, 139, 13083–13091 CrossRef CAS PubMed.
- Z. Wang, S. Zhang, Y. Chen, Z. Zhang and S. Ma, Covalent Organic Frameworks for Separation Applications, Chem. Soc. Rev., 2020, 49, 708–735 RSC.
- X. Chi, M. Li, J. Di, P. Bai, L. Song, X. Wang, F. Li, S. Liang, J. Xu and J. Yu, A Highly Stable and Flexible Zeolite Electrolyte Solid-State Li-Air Battery, Nature, 2021, 592, 551–557 CrossRef CAS PubMed.
- K. Su, W. Wang, S. Du, C. Ji and D. Yuan, Efficient Ethylene Purification by a Robust Ethane-Trapping Porous Organic Cage, Nat. Commun., 2021, 12, 3703 CrossRef CAS PubMed.
- Y. Li and J. Yu, Emerging Applications of Zeolites in Catalysis, Separation and Host–Guest Assembly, Nat. Rev. Mater., 2021, 6, 1156–1174 CrossRef CAS.
- P.-F. Cui, X.-R. Liu, Y.-J. Lin, Z.-H. Li and G.-X. Jin, Highly Selective Separation of Benzene and Cyclohexane in a Spatially Confined Carborane Metallacage, J. Am. Chem. Soc., 2022, 144, 6558–6565 CrossRef CAS PubMed.
- J.-R. Wang, K. Song, T.-X. Luan, K. Cheng, Q. Wang, Y. Wang, W. W. Yu, P.-Z. Li and Y. Zhao, Robust Links in Photoactive Covalent Organic Frameworks Enable Effective Photocatalytic Reactions under Harsh Conditions, Nat. Commun., 2024, 15, 1267 CrossRef CAS PubMed.
- Y. Xiong, Q. Feng, L. Lu, X. Qiu, S. Knoedler, A. C. Panayi, D. Jiang, Y. Rinkevich, Z. Lin, B. Mi, G. Liu and Y. Zhao, Metal–Organic Frameworks and Their Composites for Chronic Wound Healing: from Bench to Bedside, Adv. Mater., 2024, 36, 2302587 CrossRef CAS PubMed.
- C. Liu, J. Hou, M. Yan, J. Zhang, H. G. Alemayehu, W. Zheng, P. Liu, Z. Tang and L. Li, Regulating the Layered Stacking of a Covalent Triazine Framework Membrane for Aromatic/Aliphatic Separation, Angew. Chem., Int. Ed., 2024, 63, e202320137 CrossRef CAS PubMed.
- D. Wu, K. Yang, Z. Zhang, Y. Feng, L. Rao, X. Chen and G. Yu, Metal-Free Bioorthogonal Click Chemistry in Cancer Theranostics, Chem. Soc. Rev., 2022, 51, 1336–1376 RSC.
- J. R. Long and O. M. Yaghi, The Pervasive Chemistry of Metal–Organic Frameworks, Chem. Soc. Rev., 2009, 38, 1213–1214 RSC.
- L. K. Macreadie, R. Babarao, C. J. Setter, S. J. Lee, O. T. Qazvini, A. J. Seeber, J. Tsanaktsidis, S. G. Telfer, S. R. Batten and M. R. Hill, Enhancing Multicomponent Metal–Organic Frameworks for Low Pressure Liquid Organic Hydrogen Carrier Separations, Angew. Chem., Int. Ed., 2020, 59, 6090–6098 CrossRef CAS PubMed.
- Z. He, X. Huang, C. Wang, X. Li, Y. Liu, Z. Zhou, S. Wang, F. Zhang, Z. Wang, O. Jacobson, J.-J. Zhu, G. Yu, Y. Dai and X. Chen, A Catalase-Like Metal–Organic Framework Nanohybrid for O2-Evolving Synergistic Chemoradiotherapy, Angew. Chem., Int. Ed., 2019, 58, 8752–8756 CrossRef CAS PubMed.
- M. Liu, L. Guo, S. Jin and B. Tan, Covalent Triazine Frameworks: Synthesis and Applications, J. Mater. Chem. A, 2019, 7, 5153–5172 RSC.
- P. K. Thallapally, L. Dobrzańska, T. R. Gingrich, T. B. Wirsig, L. J. Barbour and J. L. Atwood, Acetylene Absorption and Binding in a Nonporous Crystal Lattice, Angew. Chem., Int. Ed., 2006, 45, 6506–6509 CrossRef CAS PubMed.
- M. Yan, Y. Wang and J. Zhou, Separation of Toluene and Alcohol Azeotropes by Nonporous Adaptive Crystals of Pillar[n]arenes with Analytical Purity of 100%, Cell Rep. Phys. Sci., 2023, 4, 101637 CrossRef CAS.
- X.-Y. Hu, T. Xiao, C. Lin, F. Huang and L. Wang, Dynamic Supramolecular Complexes Constructed by Orthogonal Self-Assembly, Acc. Chem. Res., 2014, 47, 2041–2051 CrossRef CAS PubMed.
- W. Yang, K. Samanta, X. Wan, T. U. Thikekar, Y. Chao, S. Li, K. Du, J. Xu, Y. Gao, H. Zuilhof and A. C.-H. Sue, Tiara[5]arenes: Synthesis, Solid-State Conformational Studies, Host–Guest Properties, and Application as Nonporous Adaptive Crystals, Angew. Chem., Int. Ed., 2020, 59, 3994–3999 CrossRef CAS PubMed.
- H. Yao, Y. Wang, M. Quan, M. U. Farooq, L.-P. Yang and W. Jiang, Adsorptive Separation of Benzene, Cyclohexene, and Cyclohexane by Amorphous Nonporous Amide Naphthotube Solids, Angew. Chem., Int. Ed., 2020, 59, 19945–19950 CrossRef CAS PubMed.
- J.-R. Wu and Y.-W. Yang, Synthetic Macrocycle-Based Nonporous Adaptive Crystals for Molecular Separation, Angew. Chem., Int. Ed., 2021, 60, 1690–1701 CrossRef CAS PubMed.
- M. Yan, Y. Wang, J. Chen and J. Zhou, Potential of Nonporous Adaptive Crystals for Hydrocarbon Separation, Chem. Soc. Rev., 2023, 52, 6075–6119 RSC.
- K. Jie, Y. Zhou, E. Li and F. Huang, Nonporous Adaptive Crystals of Pillararenes, Acc. Chem. Res., 2018, 51, 2064–2072 CrossRef CAS PubMed.
- Y. Ding, L. O. Alimi, B. Moosa, C. Maaliki, J. Jacquemin, F. Huang and N. M. Khashab, Selective Adsorptive Separation of Cyclohexane over Benzene Using Thienothiophene Cages, Chem. Sci., 2021, 12, 5315–5318 RSC.
- S. N. Talapaneni, D. Kim, G. Barin, O. Buyukcakir, S. H. Je and A. Coskun, Pillar[5]arene Based Conjugated Microporous Polymers for Propane/Methane Separation through Host–Guest Complexation, Chem. Mater., 2016, 28, 4460–4466 CrossRef CAS.
- J.-R. Wu and Y.-W. Yang, Geminiarene: Molecular Scale Dual Selectivity for Chlorobenzene and Chlorocyclohexane Fractionation, J. Am. Chem. Soc., 2019, 141, 12280–12287 CrossRef CAS PubMed.
- M. Wang, J. Zhou, E. Li, Y. Zhou, Q. Li and F. Huang, Separation of Monochlorotoluene Isomers by Nonporous Adaptive Crystals of Perethylated Pillar[5]arene and Pillar[6]arene, J. Am. Chem. Soc., 2019, 141, 17102–17106 CrossRef CAS PubMed.
- M. Yan, S. Wu, Y. Wang, M. Liang, M. Wang, W. Hu, G. Yu, Z. Mao, F. Huang and J. Zhou, Recent Progress of Supramolecular Chemotherapy Based on Host–Guest Interactions, Adv. Mater., 2024, 36, 2304249 CrossRef CAS PubMed.
- X. Zhao, Y. Liu, Z. Y. Zhang, Y. Wang, X. Jia and C. Li, One-Pot and Shape-Controlled Synthesis of Organic Cages, Angew. Chem., Int. Ed., 2021, 60, 17904–17909 CrossRef CAS PubMed.
- M. Wang, S. Fang, S. Yang, Q. Li, N. M. Khashab, J. Zhou and F. Huang, Separation of Ethyltoluene Isomers by Nonporous Adaptive Crystals of Perethylated and Perbromoethylated Pillararenes, Mater. Today Chem., 2022, 24, 100919 CrossRef CAS.
- B. Lu, X. Yan, J. Wang, D. Jing, J. Bei, Y. Cai and Y. Yao, Rim-Differentiated Pillar[5]arene Based Nonporous Adaptive Crystals, Chem. Commun., 2022, 58, 2480–2483 RSC.
- S. Wang, S. Mukherjee, E. Patyk-Kaźmierczak, S. Darwish, A. Yang, Q. Bajpai and M. J. Zaworotko, Highly Selective, High-Capacity Separation of o-xylene from C8 Aromatics by a Switching Adsorbent Layered Material, Angew. Chem., Int. Ed., 2019, 58, 6630–6634 CrossRef CAS PubMed.
- N. Sun, S.-Q. Wang, R. Zou, W.-G. Cui, A. Zhang, T. Zhang, Q. Li, Z.-Z. Zhuang, Y.-H. Zhang, J. Xu, M. J. Zaworotko and X.-H. Bu, Benchmark Selectivity p-xylene Separation by a Non-Porous Molecular Solid Through Liquid or Vapor Extraction, Chem. Sci., 2019, 10, 8850–8854 RSC.
- M. Wang, Q. Li, E. Li, J. Liu, J. Zhou and F. Huang, Vapochromic Behaviors of a Solid-State Supramolecular Polymer Based on Exo-Wall Complexation of Perethylated Pillar[5]arene with 1,2,4,5-Tetracyanobenzene, Angew. Chem., Int. Ed., 2021, 60, 8115–8120 CrossRef CAS PubMed.
- D. Luo, J. Tian, J. L. Sessler and X. Chi, Nonporous Adaptive Calix[4]pyrrole Crystals for Polar Compound Separations, J. Am. Chem. Soc., 2021, 143, 18849–18853 CrossRef CAS PubMed.
- J. Chen, S. Wu, Y. Wang and J. Zhou, Near-Perfect Separation of Alicyclic Ketones and Alicyclic Alcohols by Nonporous Adaptive Crystals of Perethylated Pillar[5]arene and Pillar[6]arene, Chin. Chem. Lett., 2024 DOI:10.1016/j.cclet.2024.110102.
- H.-Y. Zhou, D.-W. Zhang, M. Li and C.-F. Chen, A Calix[3]acridan-Based Host–Guest Cocrystal Exhibiting Efficient Thermally Activated Delayed Fluorescence, Angew. Chem., Int. Ed., 2022, 61, e202117872 CrossRef CAS PubMed.
- H.-Y. Zhou and C.-F. Chen, Adsorptive Separation of Picoline Isomers by Adaptive Calix[3]acridan Crystals, Chem. Commun., 2022, 58, 4356–4359 RSC.
- Y. Zhao, H. Xiao, C.-H. Tung, L.-Z. Wu and H. Cong, Adsorptive Separation of Cyclohexanol and Cyclohexanone by Nonporous Adaptive Crystals of RhombicArene, Chem. Sci., 2021, 12, 15528–15532 RSC.
- Z.-Y. Zhang and C. Li, Biphen[n]arenes: Modular Synthesis, Customizable Cavity Sizes, and Diverse Skeletons, Acc. Chem. Res., 2022, 55, 916–929 CrossRef CAS PubMed.
- G. Zhang, X. Zhu, L. O. Alimi, X. Liu and A. Chen, Adsorptive Molecular Sieving of Aromatic over Cyclic Aliphatic via Intrinsic/Extrinsic Approach, J. Mater. Chem. A, 2024, 12, 6875–6879 RSC.
- W. Yang, W. Zhang, J. Chen and J. Zhou, Mono-Functionalized Pillar[n]arenes: Syntheses, Host–Guest Properties and Applications, Chin. Chem. Lett., 2024, 35, 108740 CrossRef CAS.
- Z. Liu, S. K. M. Nalluri and J. F. Stoddart, Surveying Macrocyclic Chemistry: from Flexible Crown Ethers to Rigid Cyclophanes, Chem. Soc. Rev., 2017, 46, 2459–2478 RSC.
- Y. Wang, K. Xu, B. Li, L. Cui, J. Li, X. Jia, H. Zhao, J. Fang and C. Li, Efficient Separation of cis- and trans-1,2-Dichloroethene Isomers by Adaptive Biphen[3]arene Crystals, Angew. Chem., Int. Ed., 2019, 58, 10281–10284 CrossRef CAS PubMed.
- J. Zhou, L. Rao, G. Yu, T. R. Cook, X. Chen and F. Huang, Supramolecular Cancer Nanotheranostics, Chem. Soc. Rev., 2021, 50, 2839–2891 RSC.
- M. Yan and J. Zhou, Methylene-Bridged Naphthotubes: New Macrocyclic Arenes with Great Potential for Supramolecular Chemistry, Org. Chem. Front., 2023, 10, 2340–2345 RSC.
- L. Yue, K. Yang, X.-Y. Lou, Y.-W. Yang and R. Wang, Versatile Roles of Macrocycles in Organic-Inorganic Hybrid Materials for Biomedical Applications, Matter, 2020, 3, 1557–1588 CrossRef.
- W. Zhu, E. Li, J. Zhou, Y. Zhou, X. Sheng and F. Huang, Highly Selective Removal of Heterocyclic Impurities from Toluene by Nonporous Adaptive Crystals of Perethylated Pillar[6]arene, Mater. Chem. Front., 2020, 4, 2325–2329 RSC.
- E. Li, W. Zhu, S. Fang, K. Jie and F. Huang, Reimplementing Guest Shape Sorting of Nonporous Adaptive Crystals via Substituent-Size-Dependent Solid-Vapor Postsynthetic Modification, Angew. Chem., Int. Ed., 2022, 61, e202211780 CrossRef CAS PubMed.
- Z. Zhang, J. M. Lim, M. Ishida, V. V. Roznyatovskiy, V. M. Lynch, H.-Y. Gong, X. Yang, D. Kim and J. L. Sessler, Cyclo[m]pyridine[n]pyrroles: Hybrid Macrocycles That Display Expanded π-Conjugation upon Protonation, J. Am. Chem. Soc., 2012, 134, 4076–4079 CrossRef CAS PubMed.
- J. Chen, W. Zhang, W. Yang, F. Xi, H. He, M. Liang, Q. Dong, J. Hou, M. Wang, G. Yu and J. Zhou, Separation of Benzene and Toluene Associated with Vapochromic Behaviors by Hybrid[4]arene-Based Co-crystals, Nat. Commun., 2024, 15, 1260 CrossRef CAS PubMed.
- I.-T. Ho, Z. Zhang, M. Ishida, V. M. Lynch, W.-Y. Cha, Y. M. Sung, D. Kim and J. L. Sessler, A Hybrid Macrocycle with a Pyridine Subunit Displays Aromatic Character upon Uranyl Cation Complexation, J. Am. Chem. Soc., 2014, 136, 4281–4286 CrossRef CAS PubMed.
- J. Zhou, J. Yang, B. Hua, L. Shao, Z. Zhang and G. Yu, The Synthesis, Structure, and Molecular Recognition Properties of a [2]Calix[1]biphenyl-Type Hybrid[3]arene, Chem. Commun., 2016, 52, 1622–1624 RSC.
- J. Zhou, G. Yu, Q. Li, M. Wang and F. Huang, Separation of Benzene and Cyclohexane by Nonporous Adaptive Crystals of a Hybrid[3]arene, J. Am. Chem. Soc., 2020, 142, 2228–2232 CrossRef CAS PubMed.
- Y. Wang, S. Wu, S. Wei, Z. Wang and J. Zhou, Selectivity Separation of Ortho-Chlorotoluene Using Nonporous Adaptive Crystals of Hybrid[3]arene, Chem. Mater., 2024, 36, 1631–1638 CrossRef CAS.
- Y. Wang, Z. Wang, S. Wei, S. Wu, M. Wang, G. Yu, P. Chen, X. Liu and J. Zhou, Efficient Separation of Xylene Isomers by Nonporous Adaptive Crystals of Hybrid[3]arene in both Vapor and Liquid Phases, Mater. Chem. Front., 2024, 8, 2273–2281 RSC.
- P. R. Spackman, M. J. Turner, J. J. McKinnon, S. K. Wolff, D. J. Grimwood, D. Jayatilakab and M. A. Spackmanb, CrystalExplorer: a Program for Hirshfeld Surface Analysis, Visualization and Quantitative Analysis of Molecular Crystals, J. Appl. Cryst., 2021, 54, 1006–1011 CrossRef CAS PubMed.
- A. V. Baskar, N. Bolan, S. A. Hoang, P. Sooriyakumar, M. Kumar, L. Singh, T. Jasemizad, L. P. Padhye, G. Singh, A. Vinu, B. Sarkar, M. B. Kirkham, J. Rinklebe, S. Wang and H. Wang, Recovery, Regeneration and Sustainable Management of Spent Adsorbents from Wastewater Treatment Streams: A Review, Sci. Total Environ., 2022, 822, 153555 CrossRef CAS PubMed.
|
This journal is © the Partner Organisations 2024 |
Click here to see how this site uses Cookies. View our privacy policy here.