DOI:
10.1039/D4QM00087K
(Research Article)
Mater. Chem. Front., 2024,
8, 2341-2349
Tetrazine-derived chromones as conditionally activated solvatochromic fluorescent probes for dual imaging of lipid droplets and mitochondria†
Received
30th January 2024
, Accepted 3rd April 2024
First published on 4th April 2024
Abstract
Lipid droplets (LDs) are crucial organelles that play important roles in various physiological and pathological processes. The LD–mitochondria interplay is pivotal for cellular energy metabolism and lipid homeostasis. It is thus vital to develop high-performance LD–mitochondria dual imaging probes for studying their biological functions. Herein, we reported a new class of conditionally activated fluorogenic probes based on the conjugation of chromone and tetrazine. These probes could be either photoactivated to generate photostable and solvatochromic photoproducts for visualizing LDs and real-time, in situ monitoring of ferroptosis-induced LD dynamics with a high level of spatiotemporal control or bioorthogonally activated for mitochondria-specific imaging based on a pre-targeted approach. Most importantly, this strategy was successfully applied to label LDs and mitochondria simultaneously and realized two-color imaging in the lambda mode, which showed the great potential of using fluorescence imaging to explore the functions of LDs and related organelles.
Introduction
Lipid droplets (LDs), the main neutral lipid storage site in cells, are crucial organelles that play important roles in numerous physiological functions, including protein storage, cell metabolism, and membrane synthesis.1–3 Recently, LDs have been found to be associated with a number of pathological conditions like cancer, diabetes, liver diseases, cardiovascular diseases, and neurodegenerative diseases.4 Additionally, LDs are highly dynamic organelles that have been found to interact with other organelles such as mitochondria, lysosomes, and endoplasmic reticulum (ER) in certain biological processes.5 Specifically, the interplay between LDs and the mitochondria has drawn considerable attention due to its pivotal role in maintaining lipid and energy homeostasis.6 Therefore, visualizing and dynamically tracking LDs and mitochondria simultaneously is of particular importance for biomedical research and early clinical diagnosis.
Recently, fluorescent probes have become valuable tools for versatile bioimaging in living cells.7 As LDs provide an inner hydrophobic and viscous environment, LD-specific fluorescent probes are usually hydrophobic dyes with the solvatochromic effect. The commercially available fluorescent dyes, Nile Red and BODIPY 493/503 green, are the most commonly used LD markers. However, Nile Red suffers from inferior selectivity to LDs8 and BODIPY 493/503 green has small Stokes shifts, which cause severe self-absorption.9 Although various fluorophores have been reported for staining LDs,10,11 development of specific bioprobes with high spatiotemporal resolution and long-term imaging capabilities for exploring the complicated functions and dynamics of LDs is still an urgent need. Besides, the dual labelling tools for mitochondria and LDs are rarely reported.12–16 It is necessary to develop multifunctional fluorescent probes that can simultaneously target both mitochondria and LDs.
Photoactivatable fluorophores are advanced imaging tools that could switch from a weak or non-emissive state into an emissive state upon light irradiation.17 Photoactivatable fluorophores have found widespread application in chemical biology, as they have great advantages in light-controlled imaging with high spatial and temporal resolution in complex biological systems. Tetrazine, the most popular bioorthogonal group for developing fluorogenic probes owing to its fast reaction kinetics and excellent fluorescence quenching efficiency,18,19 has been recently reported as a general phototrigger for designing photoactivatable fluorophores.20 Upon illumination, the tetrazine quencher is photodecomposed to nitriles, thereby restoring the fluorescence of fluorophores. Since very few photoactivatable fluorophores were reported for visualizing LDs,16,21,22 it is possible and highly desirable to develop tetrazine-based photoactivatable LD-specific probes to investigate their biological functions.
In this study, towards the development of high-performance organelle labeling probes, we designed a series of tetrazine-derived chromones as activatable solvatochromic fluorescent probes, which could be photo-activated under light irradiation for LD-specific imaging based on their hydrophobic scaffold or bioorthogonally activated via the inverse electron-demand Diels–Alder (iEDDA) reaction for mitochondrial imaging through a pre-targeting strategy (Scheme 1). Using these probes, we succeeded in monitoring the number and dynamics of LDs during ferroptosis by the real-time and in situ tracking and realizing simultaneous labeling of LDs and mitochondria.
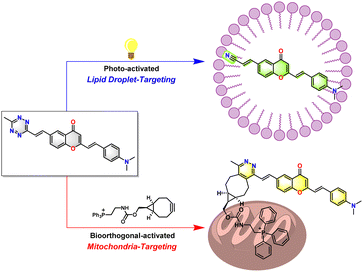 |
| Scheme 1 Conditionally activated chromone–tetrazine fluorogenic probes. | |
Results and discussion
Design and synthesis of tetrazine probes
At the outset, we designed three tetrazine-modified chromone probes (DMAC-Tz1/2/3, Scheme 2) using a monochromophoric strategy23 by installing tetrazine onto the 6th or 7th position of a chromone derivative, 2-(4-(dimethylamino)styryl)-4H-chromen-4-one (DMAC), which has been reported to be a solvatochromic fluorescent probe for quantifying the polarity of aggregated proteins.24 The synthetic procedures of the three probes are disclosed in the ESI.† Briefly, 2-methyl-6/7-formyl-4H-chromen-4-one was firstly prepared based on the reported method.25 Then the alkene double bonds were built through the Horner–Wadsworth–Emmons (HWE) reaction (6th or 7th position) or Knoevenagel condensation (2nd position), to afford DMAC-Tz1/2/3.
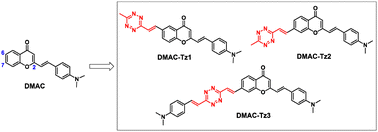 |
| Scheme 2 Design of tetrazine-modified chromone probes. | |
Photoactivated fluorogenic features
With these chromone probes in hand, we evaluated their photoactivatable fluorescence enhancement characteristics and solvatochromic properties. All three probes showed negligible emission in various solvents, proving the splendid fluorescence quenching effect of the tetrazine moiety (Fig. S1, ESI†). Then we irradiated the probes in THF at 254 nm using a hand-held UV lamp for 0–120 min (Fig. 1a). To our delight, a gradually increased fluorescence was observed for all three probes upon irradiation, which was attributed to the photolysis of tetrazine to generate DMAC-CN1 (for DMAC-Tz1) and DMAC-CN2 (for DMAC-Tz2 and DMAC-Tz3) (Fig. 1b–d and Fig. S2, ESI†). Among them, DMAC-Tz1 possessed the highest emission intensity and the fastest photolysis kinetics, reaching maximum intensity at 60 min (Fig. 1e). The decline of fluorescence of DMAC-Tz1 after 60 min irradiation may be owing to the photobleaching of the photolysis product, DMAC-CN1, exposed to 254 nm UV light for too long time (Fig. S3, ESI†). Since the 405 nm wavelength is commonly available in the confocal microscopes, we also tested the change in the fluorescence of DMAC-Tz1/2/3 upon 405 nm light irradiation. As shown in Fig. S4 (ESI†), their fluorescence gradually increased as irradiation time grew, indicating that the probes could also be photolyzed by 405 nm light and suitable for microscopic imaging.
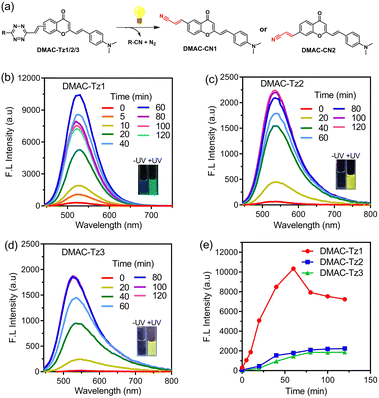 |
| Fig. 1 (a) Photolysis of DMAC-Tz1/2/3 to generate DMAC-CN1/2. The emission spectra of DMAC-Tz1 (b), DMAC-Tz2 (c), and DMAC-Tz3 (d) in THF (10 μM) upon irradiation with 254 nm UV light for 0–120 min (λexc = 405 nm). (e) Plot of fluorescence intensity at maximum emission of each probe versus the irradiation time. | |
To intensively investigate the solvatochromic effect, we synthesized the photolysis products, DMAC-CN1 and DMAC-CN2 (see ESI†), and assessed their photophysical properties in various solvents with different polarities (Table S1, ESI†). Both probes exhibited polarity-sensitive emission behavior owing to the intramolecular charge transfer (ICT) mechanism originating from their donor–π–acceptor (D–π–A) structures. As the solvent polarity increased (from toluene to H2O), their absorption maxima displayed slight changes (Fig. S5a, b and Table S1, ESI†), while their emission maxima were dramatically red-shifted (for DMAC-CN1, from 496 nm to 635 nm and for DMAC-CN2, from 515 nm to 652 nm) (Fig. 2a, b and Fig. S5c, d, and Table S1, ESI†). The large Stokes shifts (74–217 nm for DMAC-CN1 and 85–202 nm for DMAC-CN2) could minimize background signals, which are favorable for live-cell imaging. In addition, we correlated the maximum emission wavelength of DMAC-CN1/2 with the ET(30) value of solvents (Fig. 2c and d), a solvent polarity parameter that represents solute–solvent interactions on a microscopic level.26 As the ET(30) value increases, the λem of the probes increases linearly, verifying that DMAC-CN1 and DMAC-CN2 were sensitive to solvent polarity. However, there is still difference in emission intensity for these two probes. Compared with DMAC-CN1, DMAC-CN2 was almost nonfluorescent in polar solvents such as H2O, MeOH, DMSO, and CH3CN (Fig. 2c, d and Fig. S5c, d, ESI†). Meanwhile, we measured the emission of DMAC-CN1/2 in a proportionally mixed solution of dioxane and H2O with different water fractions (fw), to test their fluorescence sensitivity toward water-containing microenvironments. For DMAC-CN1, the emission intensity was first increased upon enhancing fw from 0 to 10%, and then gradual decrease of fluorescence and red-shift of the emission were observed upon further increase of fw to 100% (Fig. 2g and i). On the other hand, for DMAC-CN2, the fluorescence intensity declined extremely followed by bathochromic shift of the emission once 10% water fraction was introduced (Fig. 2h and j). Taken together, DMAC-CN2 is more sensitive to slight polarity changes than DMAC-CN1.
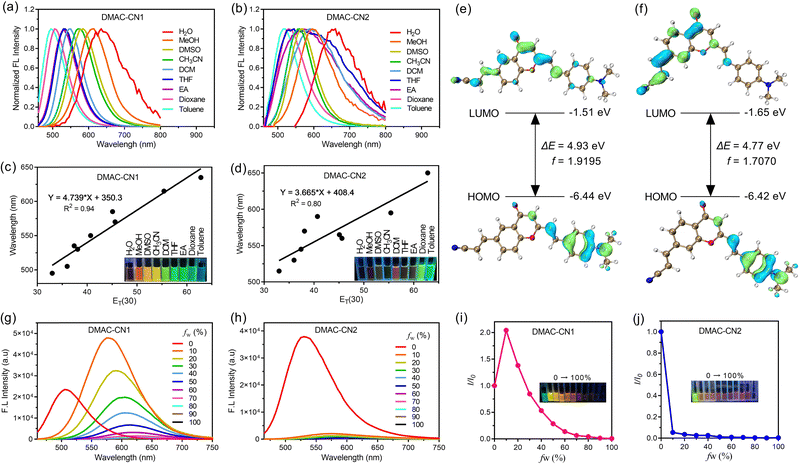 |
| Fig. 2 Normalized fluorescence emission spectra of DMAC-CN1 (a) and DMAC-CN2 (b) in various solvents (λexc = 405 nm). Fluorescence images and plot of the emission maximum wavelength of DMAC-CN1 (c) and DMAC-CN2 (d) in different solvents with ET(30). The optimized molecular orbital amplitude plots of the HOMOs and LUMOs of DMAC-CN1 (e) and DMAC-CN2 (f). Fluorescence emission spectra of DMAC-CN1 (g) and DMAC-CN2 (h) in dioxane/H2O mixed solution (10 μM) with different water fractions (0–100%). Plot of relative emission intensity (I/I0) of DMAC-CN1 (i) and DMAC-CN2 (j) vs. different water fractions. | |
The turn-on effect of DMAC-CN1/2 towards DMAC-Tz1/2/3 could be explained by density functional theory (DFT) calculations. As shown in Fig. S6 and Table S2 (ESI†), for DMAC-Tz1/2/3, incorporation of tetrazine into chromone makes the optically inactive n → π* transition dominant for the S0–S1 transition, resulting in low oscillator strength (the f value ranges from 0.0055 to 0.0058), and quenches the fluorescence of chromophores. After photolysis (DMAC-CN1/2), destruction of tetrazine makes the optically active π → π* transition the major S0–S1 transition, which exhibited high f values (from 1.7070 to 1.9195), thus turning on the fluorescence (Fig. 2e and f). Besides, for DMAC-CN1/2, the highest occupied molecular orbital (HOMO) was mainly located in the electron-donating N,N-(dimethylamino)styryl moiety, while the lowest unoccupied molecular orbital (LUMO) was mainly localized in the electron-withdrawing chromone scaffold and enenitrile moiety (Fig. 2e and f).27 This orbital distribution feature clearly validates the ICT process at the excited state and accounts for the solvatochromic characteristics of probes.
Bioorthogonally activated fluorogenic properties
On the other hand, we investigated the iEDDA reactions of DMAC-Tz1/2/3 with a strained alkyne – (1R,8S,9R)-bicyclo[6.1.0]non-4-yn-9-ylmethanol (BCN-OH) (Fig. 3a). Among three probes, DMAC-Tz1 reacted rapidly and smoothly, with a second order kinetic constant of 1.28 M−1 s−1 (Fig. 3b and Fig. S7, ESI†), and the reaction was almost unaffected by nucleophilic amino acids (e.g., Ser, Glu, Lys, His, Arg, Met, and Cys), verifying the biocompatibility of this reaction (Fig. S8, ESI†). Upon reaction with BCN-OH, remarkable fluorescence enhancement was noticed, indicating that converting tetrazine to pyridazine relieves the quenching effect and restores fluorescence (Fig. 3c–e and Fig. S9, ESI†). Moreover, the cycloadducts, DMAC-Pz1 and DMAC-Pz2, exhibited similar photophysical properties and solvatochromic behaviors with DMAC-CN1 and DMAC-CN2, respectively (Fig. S10 and S11, ESI†), because they share a close D–π–A structure. For DMAC-Pz3, the emission peak was greatly red-shifted owing to its extended π-conjugation system (Fig. S10h and i, ESI†).
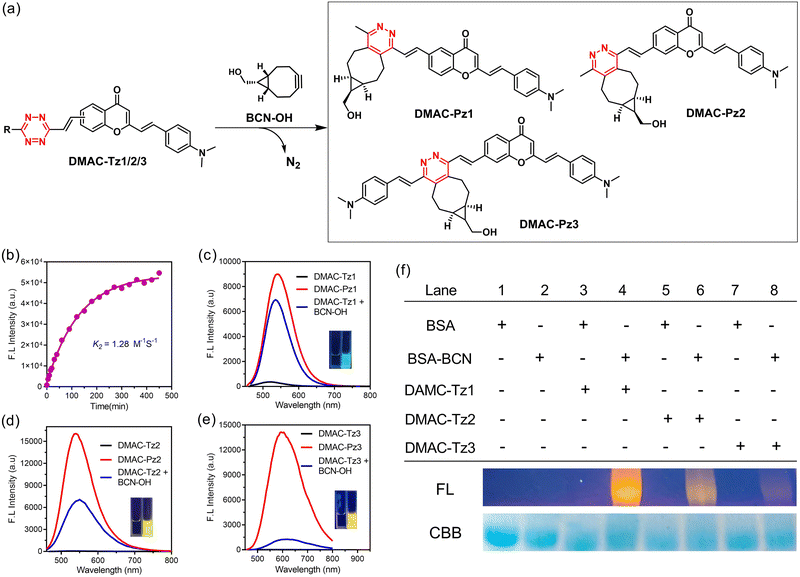 |
| Fig. 3 (a) Bioorthogonal reactions between DMAC-Tz1/2/3 and BCN-OH. (b) Analysis of reaction kinetics for the reaction between DMAC-Tz1 (0.1 mM) and BCN-OH (1 mM) in DMSO. Fluorescence emission spectra and fluorescence images of 10 μM DMAC-Tz1/Pz1 (c), DMAC-Tz2/Pz2 (d), and DMAC-Tz3/Pz3 (e), and the in situ fluorescence enhancement after reaction with BCN-OH (100 μM) in THF. (f) In-gel visualization of the labeling of BSA-BCN with DMAC-Tz1/2/3. | |
To test the labeling feasibility of the iEDDA reactions of these tetrazine probes in a biological system, we prepared BCN-conjugated bovine serum albumin (BSA) protein (BSA–BCN) and reacted it with DMAC-Tz1/2/3 followed by SDS-PAGE and in-gel fluorescence analyses. As shown in Fig. 3f, all three probes allowed effectively labeling of BSA–BCN, among which DMAC-Tz1 showed the highest labeling efficiency (lane 4), in accordance with its rapid reaction kinetics and high fluorescence quantum yield. It is worth noting that negligible fluorescence signals were detected for unmodified BSA controls (lanes 3, 5, and 7), confirming the specificity of this labeling strategy.
Photoactivated LD imaging
Having established the excellent photoactivation and bioorthogonal-activation properties of these chromone-tetrazine probes, we sought to explore their utility in biological applications. Based on MTT assay, no significant influence on the cell viability was found when the DMAC-Tz1/2 concentration of up to 50 μM was used, proving that the probes have very low cytotoxicity (Fig. S12, ESI†).
The photoactivatable LD imaging of DMAC-Tz1/2 was then performed using HeLa cells as a model cancer cell line. After incubation with 1 μM DMAC-Tz1/2 at 37 °C for 1 h, the intracellular photoactivated fluorescence of HeLa cells was monitored by confocal laser scanning microscopy (CLSM), and the time-lapsed fluorescence images were acquired. As shown in Fig. 4a and Fig. S13a (ESI†), both probes have weak fluorescence prior to illumination, but displayed a fast light-up process following irradiation with 405 nm laser from the CLSM, suggesting good photoactivation of DMAC-Tz1/2. The fluorescence intensity of HeLa cells increased gradually as the irradiation time prolonged and reached a plateau at 210 s, resulting in a 6-fold high light-up ratio (Fig. 4b). A co-localization experiment revealed that the green fluorescence of photoactivated DMAC-Tz1/2 fits well with the red fluorescence of the commercially LD marker, Nile Red (Fig. 4c and 5a, b, d), with a high Pearson's coefficient of 0.94 for each probe (Fig. 5c and e), highlighting the LD-specific targeting of DMAC-Tz1/2.
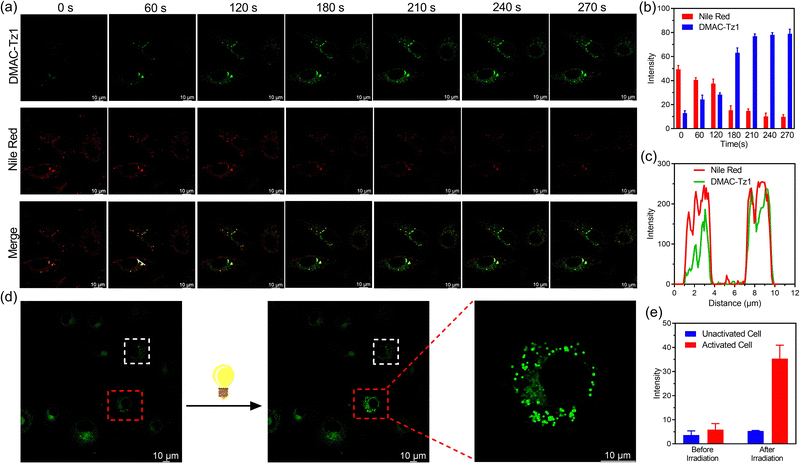 |
| Fig. 4 (a) CLSM images of HeLa cells co-stained with DMAC-Tz1 and Nile Red under 405 nm laser scanning for 0–270 s. For the green channel, λex = 405 nm, λem = 480–540 nm; for the red channel, λex = 552 nm, λem = 560–660 nm. Scale bar: 10 μm. DMAC-Tz1 : 1 μM; Nile Red: 10 μM. (b) The quantification of fluorescence intensity of HeLa cells with the increasing irradiation time under 405 nm laser scanning processed by ImageJ. (c) Plot of intensities along the white line in (a). (d) Spatially controlled photoactivation of DMAC-Tz1 in HeLa cells. The cell in the red-dotted square area was exposed to 405 nm laser for 120 s followed by fluorescence acquisition. (e) The quantification of fluorescence intensity of the activated cell and the non-activated cell before and after irradiation processed by ImageJ. | |
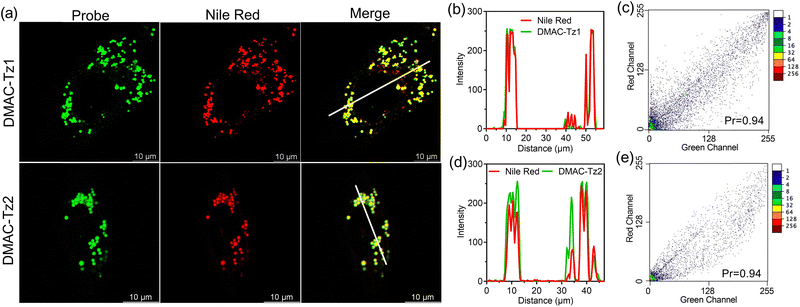 |
| Fig. 5 (a) CLSM images of HeLa cells co-stained with DMAC-Tz1/2 and Nile Red. For the green channel, λex = 405 nm, λem = 480–540 nm; for the red channel, λex = 552 nm, λem = 560–660 nm. Scale bar: 10 μm. DMAC-Tz1 : 1 μM; Nile Red: 10 μM. (b) and (d) Plot of intensities along the white line in (a). Plot of the fluorescence correlation between Nile Red and DMAC-Tz1 (c) and DMAC-Tz2 (e). | |
To further investigate the spatiotemporal controllability of this photoactivated labelling method, we treated HeLa cells with 1 μM DMAC-Tz1 for 1 h followed by washing away the excess reagent with PBS. Afterward, a single cell among a population of cells (indicated by a red-dotted square in Fig. 4d) was selectively laser irradiated at 405 nm for 120 s to trigger photolysis. Strong turn-on fluorescence was only observed in the laser-irradiated cell and not in the adjacent cells (indicated by a white-dotted square in Fig. 4d), and quantification of the cytosolic fluorescence in the activated cell revealed a 6-fold enhancement (Fig. 4e). These outcomes revealed that reliable spatiotemporal labelling of LDs in living cells can be achieved by photoactivation of DMAC-Tz1.
Moreover, green fluorescence signals of DMAC-CN1/2 could also be found to match well with Nile Red (Fig. S14a, c and S15a, c, ESI†), illustrating that the enhanced fluorescence of HeLa cells treated with DMAC-Tz1/2 could be attributed to the fast and effective transformation of DMAC-Tz1/2 into DMAC-CN1/2 via photolysis. After irradiation at 405 nm for 240 s, the intracellular fluorescence of DMAC-CN1/2 remains considerably strong, revealing their notable photostability (Fig. S14b and S15b, ESI†). In comparison, Nile Red displayed significantly reduced fluorescence during this period (reduced by 73–83%), which is ascribed to its poor resistance to photobleaching. These results demonstrated that DMAC-Tz1 and DMAC-Tz2 were highly suitable for long-term, high-quality tracking of LD dynamics.
Monitoring LD dynamics in ferroptosis
Ferroptosis is a unique iron-dependent form of nonapoptotic cell death due to the large accumulation of lipid peroxides, which is closely related to a number of physiological and pathological processes.28,29 Recently, lipid droplets have been demonstrated to be associated with the regulation of ferroptosis.30 Excessive accumulation of lipid peroxides during ferroptosis could trigger rapid response of LDs, which leads to changes in the LD number and polarity. To monitor the variations of LD during ferroptosis, HeLa cells were treated with DMAC-Tz1 (1 μM) for 1 h and then incubated with 10 μM erastin, a well-known ferroptosis inducer,31 for 0–2 h, followed by photoactivable CLSM imaging. Bright green fluorescent puncta were observed inside cells, and the fluorescence increased greatly over time, indicating a sharp rise in the number of LDs (Fig. 6a and b). Once the ferroptosis inhibitor, Ferrostatin 1 (Fer-1),32 was incubated with HeLa cells together with erastin and DMAC-Tz1, there was no obvious changes in the intracellular fluorescence signals at different times (Fig. 6a and b). These outcomes demonstrated that ferroptosis can accelerate the production of LDs and DMAC-Tz1 is a powerful tool for in situ, real-time monitoring of the dynamic behaviors of LDs during ferroptosis.
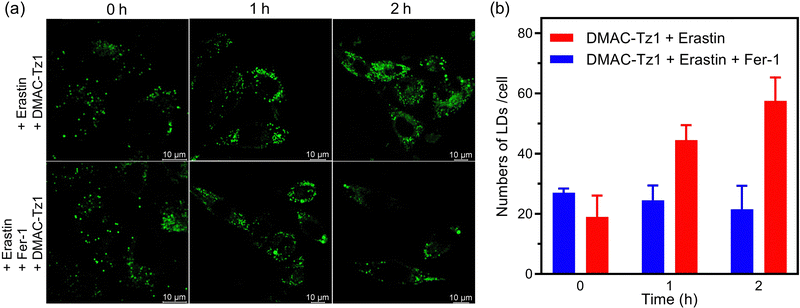 |
| Fig. 6 (a) CLSM images of HeLa cells incubated with 1 μM DMAC-Tz1 for 1 h, and then treated with 10 μM erastin (upper) or incubated with 10 μM erastin and 15 μM Fer-1 (below) for 0–2 h. λex = 405 nm, λem = 480–540 nm. Scale bar: 10 μm. (b) The quantification of the number of LDs per cell at different times processed by ImageJ. | |
Simultaneous imaging of LDs and mitochondria
For mitochondrial imaging, a combinatorial strategy of pre-targeting and bioorthogonal-labeling was employed (Fig. 7a). We firstly incubated the BCN–triphenylphosphonium conjugate, BCN–TPP,33 with HeLa cells to pre-target BCN into mitochondria. Then the probe with highest labeling efficiency, DMAC-Tz1, was added to the cells for in situ bioorthogonal labeling, followed by CLSM imaging without further washing steps. As shown in Fig. 7b, after excitation at 405 nm, strong fluorescence signals of filament-like morphology were observed in HeLa cells and overlapped well with the commercial mitochondria tracker, Mito Tracker Red (MTR, Pearson's correlation coefficient = 0.95), suggesting the accumulation of the probe in mitochondria (Fig. 7c and d).
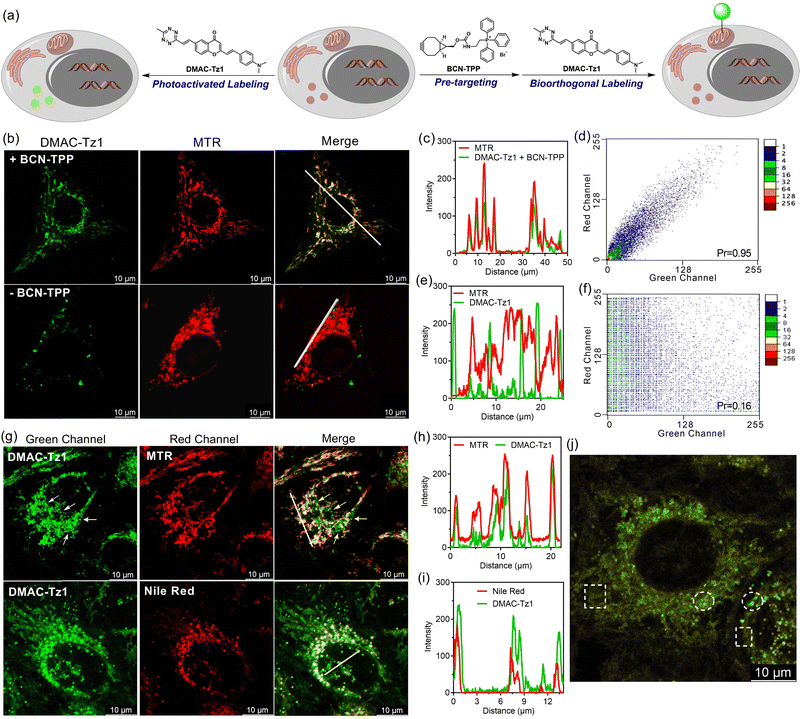 |
| Fig. 7 (a) Schematic diagram of the bioorthogonal imaging of mitochondria and photoactivated imaging of LDs in live HeLa cells. (b) CLSM images of HeLa cells co-stained with DMAC-Tz1/BCN-TPP and MTR. For the green channel, λex = 405 nm, λem = 480–540 nm; for the red channel, λex = 579 nm, λem = 585–660 nm. Scale bar: 10 μm. DMAC-Tz1 : 1 μM; BCN-TPP: 1 μM; MTR: 200 nM. (c) and (e) Plot of intensities along the white line in (b). Plot of the fluorescence correlation between MTR and DMAC-Tz1 in the presence (d) or absence (f) of BCN-TPP. (g) CLSM images of HeLa cells co-stained with DMAC-Tz1/BCN-TPP and MTR or Nile Red. For the green channel, λex = 405 nm, λem = 480–640 nm; for the MTR channel, λex = 579 nm, λem = 585–660 nm; for the Nile Red channel, λex = 552 nm, λem = 560–660 nm. Scale bar: 10 μm. DMAC-Tz1: 2 μM; BCN-TPP: 1 μM; MTR: 200 nM; Nile Red: 10 μM. (h) and (i) Plot of intensities along the white line in (g). (j) Real-color fluorescence image of the HeLa cell stained with DMAC-Tz1/BCN-TPP in the lambda mode. λex = 405 nm. | |
It is worth noting that in the absence of BCN-TPP, DMAC-Tz1 could not label mitochondria due to the lack of the targeting group, but could still stain LDs through its hydrophobicity-based targeting followed by photoactivated imaging (Fig. 7b, e and f). Inspired by this result, an effort was made to test whether DMAC-Tz1 could be used to dual label mitochondria and LDs in different activation pathways. Gratifyingly, when excess DMAC-Tz1 towards BCN-TPP was used (2
:
1 ratio), apart from the signals of mitochondria (overlapped with MTR, Fig. 7g and h), we also found dozens of intracellular green fluorescent spots after a fast turn-on process (indicated by white arrows in Fig. 7g), which nicely colocalized with the LD marker, Nile Red (Fig. 7i). Herein, DMAC-Tz1 served as a bifunctional fluorescent probe: one part reacted with BCN-TPP for bioorthogonal labeling of mitochondria, and the unreacted part targeted hydrophobic LDs for photoactivatable imaging. To achieve two-color imaging of these two organelles, we then studied real-color imaging of the probe by using the lambda imaging mode, which can discriminate one stained region from another with distinct emission colors, therefore being powerful for dual labeling of different organelles.34 Upon excitation at 405 nm, bright green emission was observed in globular LDs (indicated by the white circles) and weak yellow emission in the filament-like mitochondria region (indicated by the white squares) (Fig. 7j). After analyzing the emission maximum wavelengths of DMAC-CN1 and DMAC-Pz1 in different solvents (Fig. S11, ESI†), we conclude that the strong green emission characteristics of LDs are similar to those of DMAC-CN1 in the nonpolar solvents, toluene or dioxane (emission around 520 nm), while the weak yellow emission features of mitochondria seem to be similar to those of DMAC-Pz1 in polar solvents such as MeOH or DMSO (emission at 565–600 nm). These outcomes indicated that DMAC-Tz1 could be applied for simultaneous imaging of LDs and mitochondria in two different colors by using the lambda mode.
Conclusions
In summary, three tetrazine-modified chromone derivatives (DMAC-Tz1/2/3) were rationally designed and synthesized as conditionally activated fluorogenic probes. First, they could be photoactivated to produce DMAC-CN1/2, which showed apparent solvatochromic features and excellent photostability and succeeded in visualizing LDs and real-time, in situ monitoring of ferroptosis-induced LD dynamics with a high spatiotemporal resolution. Second, they could be bioorthogonally activated by pre-targeted BCN-TPP for mitochondria-specific imaging. In particular, DMAC-Tz1 allowed concurrent labeling of both LDs and mitochondria through different activation pathways and realized two-color imaging in the lambda mode. Therefore, we anticipate that these unique probes could provide practical tools for the in-depth understanding of the biological functions of LDs and related organelles.
Conflicts of interest
There are no conflicts to declare.
Acknowledgements
This work was sponsored by the Beijing Nova Program (Z201100006820049, 20220484234), CAMS Innovation Fund for Medical Sciences (2022-I2M-1-013), and Special Research Fund for Central Universities, Peking Union Medical College (3332022043).
Notes and references
- A. R. Thiam, R. V. Farese Jr and T. C. Walther, The biophysics and cell biology of lipid droplets, Nat. Rev. Mol. Cell Biol., 2013, 14, 775–786 CrossRef CAS PubMed
.
- T. C. Walther and R. V. Farese, Lipid Droplets and Cellular Lipid Metabolism, Annu. Rev. Biochem., 2012, 81, 687–714 CrossRef CAS PubMed
.
- J. A. Olzmann and P. Carvalho, Dynamics and functions of lipid droplets, Nat. Rev. Mol. Cell Biol., 2018, 20, 137–155 CrossRef PubMed
.
- N. Krahmer, R. V. Farese and T. C. Walther, Balancing the fat: lipid droplets and human disease, EMBO Mol. Med., 2013, 5, 973–983 CrossRef PubMed
.
- A. D. Barbosa, D. B. Savage and S. Siniossoglou, Lipid droplet–organelle interactions: emerging roles in lipid metabolism, Curr. Opin. Cell Biol., 2015, 35, 91–97 CrossRef CAS PubMed
.
- I. Y. Benador, M. Veliova, M. Liesa and O. S. Shirihai, Mitochondria Bound to Lipid Droplets: Where Mitochondrial Dynamics Regulate Lipid Storage and Utilization, Cell Metab., 2019, 29, 827–835 CrossRef CAS PubMed
.
- H. Fang, Y. Chen, Z. Jiang, W. He and Z. Guo, Fluorescent Probes for Biological Species and Microenvironments: from Rational Design to Bioimaging Applications, Acc. Chem. Res., 2023, 56, 258–269 CrossRef CAS PubMed
.
- P. Greenspan, E. P. Mayer and S. D. Fowler, Nile Red: A Selective Fluorescent Stain for Intracellular Lipid Droplets, J. Cell Biol., 1985, 100, 965–973 CrossRef CAS PubMed
.
- E. E. Spangenburg, S. J. P. Pratt, L. M. Wohlers and R. M. Lovering, Use of BODIPY (493/503) to Visualize Intramuscular Lipid Droplets in Skeletal Muscle, J. Biomed. Biotechnol., 2011, 2011, 1–8 CrossRef PubMed
.
- H. Tian, A. C. Sedgwick, H.-H. Han, S. Sen, G.-R. Chen, Y. Zang, J. L. Sessler, T. D. James, J. Li and X.-P. He, Fluorescent probes for the imaging of lipid droplets in live cells, Coord. Chem. Rev., 2021, 427, 213577 CrossRef CAS
.
- Y. Zhao, W. Shi, X. Li and H. Ma, Recent advances in fluorescent probes for lipid droplets, Chem. Commun., 2022, 58, 1495–1509 RSC
.
- X. Li, C. Long, Y. Cui, F. Tao, X. Yu and W. Lin, Charge-Dependent Strategy Enables a Single Fluorescent Probe to Study the Interaction Relationship between Mitochondria and Lipid Droplets, ACS Sens., 2021, 6, 1595–1603 CrossRef CAS PubMed
.
- L. B. H. Pham, K. L. Chung, S. Kim and J. Lee, Multifunctional photosensitizers for the activation and visualization of pyroptotic cell death under photodynamic therapy, Sens. Actuators, B, 2024, 399, 134799 CrossRef CAS
.
- L. Zhu, L. Huang, W. Su, X. Liang and W. Lin, A Fluorescent Probe Targeting Mitochondria and Lipid Droplets for Visualization of Cell Death, Chem. – Asian J., 2022, 17, e202101304 CrossRef CAS PubMed
.
- L. Wang, Y. Ma, Z. Li, S. Li and W. Lin, A multifunctional fluorescent probe for dual-color visualization of intracellular mitochondria and lipid droplets and monitoring of SO2 in vivo, Chem. Eng. J., 2023, 451, 139023 CrossRef CAS
.
- Z. Zhang, W. Pan, Y. Xie, K. Liu, M. Gao and Y. Wang, Photoactivatable tandem fluorescence imaging of organelles and their interplay monitoring, Mater. Chem. Front., 2022, 6, 3662–3668 RSC
.
- Q. Shao and B. Xing, Photoactive molecules for applications in molecular imaging and cell biology, Chem. Soc. Rev., 2010, 39, 2835–2846 RSC
.
- W. Mao, J. Tang, L. Dai, X. He, J. Li, L. Cai, P. Liao, R. Jiang, J. Zhou and H. Wu, A General Strategy to Design Highly Fluorogenic Far-Red and Near-Infrared Tetrazine Bioorthogonal Probes, Angew. Chem., Int. Ed., 2020, 60, 2393–2397 CrossRef PubMed
.
- W. Mao, W. Chi, X. He, C. Wang, X. Wang, H. Yang, X. Liu and H. Wu, Overcoming Spectral Dependence: A General Strategy for Developing Far-Red and Near-Infrared Ultra-Fluorogenic Tetrazine Bioorthogonal Probes, Angew. Chem., Int. Ed., 2022, 61, e202117386 CrossRef CAS PubMed
.
- A. Loredo, J. Tang, L. Wang, K.-L. Wu, Z. Peng and H. Xiao, Tetrazine as a general phototrigger to turn on fluorophores, Chem. Sci., 2020, 11, 4410–4415 RSC
.
- M. Gao, H. Su, Y. Lin, X. Ling, S. Li, A. Qin and B. Z. Tang, Photoactivatable aggregation-induced emission probes for lipid droplets-specific live cell imaging, Chem. Sci., 2017, 8, 1763–1768 RSC
.
- X. Chen, Z. Zhang, W. Luo, Z. Zhuang, Z. Zhao, L. Wang, D. Wang and B. Z. Tang, A photoactivatable theranostic probe for simultaneous oxidative stress-triggered multi-color cellular imaging and photodynamic therapy, Biomaterials, 2022, 287, 121680 CrossRef CAS PubMed
.
- D. Kim, H. Son and S. B. Park, Ultrafluorogenic Monochromophore-Type BODIPY-Tetrazine Series for Dual-Color Bioorthogonal Imaging with a Single Probe, Angew. Chem., Int. Ed., 2023, 62, e202310665 CrossRef CAS PubMed
.
- W. Wan, L. Zeng, W. Jin, X. Chen, D. Shen, Y. Huang, M. Wang, Y. Bai, H. Lyu, X. Dong, Z. Gao, L. Wang, X. Liu and Y. Liu, A Solvatochromic Fluorescent Probe Reveals Polarity Heterogeneity upon Protein Aggregation in Cells, Angew. Chem., Int. Ed., 2021, 60, 25865–25871 CrossRef CAS PubMed
.
- H. Göker, D. W. Boykin and S. Yıldız, Synthesis and potent antimicrobial activity of some novel 2-phenyl or methyl-4H-1-benzopyran-4-ones carrying amidinobenzimidazoles, Bioorg. Med. Chem., 2005, 13, 1707–1714 CrossRef PubMed
.
- J. P. Cerón-Carrasco, D. Jacquemin, C. Laurence, A. Planchat, C. Reichardt and K. Sraïdi, Solvent polarity scales: determination of new ET(30) values for 84 organic solvents, J. Phys. Org. Chem., 2014, 27, 512–518 CrossRef
.
- T. Lu and F. Chen, Multiwfn: A multifunctional wavefunction analyzer, J. Comput. Chem., 2011, 33, 580–592 CrossRef PubMed
.
- Y. Xie, W. Hou, X. Song, Y. Yu, J. Huang, X. Sun, R. Kang and D. Tang, Ferroptosis: process and function, Cell Death Differ., 2016, 23, 369–379 CrossRef CAS PubMed
.
- B. R. Stockwell, J. P. Friedmann Angeli, H. Bayir, A. I. Bush, M. Conrad, S. J. Dixon, S. Fulda, S. Gascón, S. K. Hatzios, V. E. Kagan, K. Noel, X. Jiang, A. Linkermann, M. E. Murphy, M. Overholtzer, A. Oyagi, G. C. Pagnussat, J. Park, Q. Ran, C. S. Rosenfeld, K. Salnikow, D. Tang, F. M. Torti, S. V. Torti, S. Toyokuni, K. A. Woerpel and D. D. Zhang, Ferroptosis: A Regulated Cell Death Nexus Linking Metabolism, Redox Biology, and Disease, Cell, 2017, 171, 273–285 CrossRef CAS PubMed
.
- D. Li and Y. Li, Signal Transduction Targeted Ther., 2020, 5, 108 CrossRef PubMed
.
- Y. Du and Z. Guo, Recent progress in ferroptosis: inducers and inhibitors, Cell Death Discovery, 2022, 8, 501 CrossRef CAS PubMed
.
- O. Zilka, R. Shah, B. Li, J. P. Friedmann Angeli, M. Griesser, M. Conrad and D. A. Pratt, On the Mechanism of Cytoprotection by Ferrostatin-1 and Liproxstatin-1 and the Role of Lipid Peroxidation in Ferroptotic Cell Death, ACS Cent. Sci., 2017, 3, 232–243 CrossRef CAS PubMed
.
- Y. Wang, Y. Teng, H. Yang, X. Li, D. Yin and Y. Tian, Bioorthogonally applicable multicolor fluorogenic naphthalimide-tetrazine probes with aggregation-induced emission characters, Chem. Commun., 2022, 58, 949–952 RSC
.
- L. Guo, M. Tian, Z. Zhang, Q. Lu, Z. Liu, G. Niu and X. Yu, Simultaneous Two-Color Visualization of Lipid Droplets and Endoplasmic Reticulum and Their Interplay by Single Fluorescent Probes in Lambda Mode, J. Am. Chem. Soc., 2021, 143, 3169–3179 CrossRef CAS PubMed
.
Footnote |
† Electronic supplementary information (ESI) available: Supplementary figures, tables, experimental procedures, and NMR spectra. See DOI: https://doi.org/10.1039/d4qm00087k |
|
This journal is © the Partner Organisations 2024 |