DOI:
10.1039/D3QM01082A
(Research Article)
Mater. Chem. Front., 2024,
8, 282-286
Nitrogen-coordinated ruthenium on porous carbon enhanced hydrogen evolution reactions
Received
7th October 2023
, Accepted 31st October 2023
First published on 20th November 2023
Abstract
Hydrogen evolution reactions (HERs) play an important role in sustainable energy conversion technologies. Single-atom metal-based catalysts show better performance than bulk-phase catalysts in the HER process. However, it is still highly desirable to search an alternative catalyst to commercial Pt-based catalysts owing to the scarcity problem of Pt. In this work, theoretical calculations were performed for carbon-supported nitrogen-coordinated Ru single-atom systems. The calculation results indicated that the four-nitrogen coordinated pyrrolic-Ru configuration shows the best catalytic performance for the HER process, which has an energy barrier of +0.09 eV. The nitrogen-coordinated system significantly enhances the Ru bulk system with an energy barrier of −0.62 eV. Electron charge density analysis revealed that the N-coordinated configuration gives rise to electron accumulation on Ru-centered areas, which is favorable for hydrogen adsorption during HERs.
Introduction
The emission of a large number of greenhouse gases is a significant reason for global warming, which is a serious threat to human survival and development.1 As a solution, the conservation of green energy from water splitting using fuel cells is an attractive strategy to overcome the current environmental problem. Nowadays, hydrogen evolution reactions (HERs) for water splitting play a vital role in sustainable hydrogen and energy cycle via photochemical, thermochemical, biochemical, and electrochemical methods.2–4 The electrochemical method of HERs has been widely explored in the past several years owing to some particular advantages, including high turnover numbers, low cost and environmental benignness.5 In practical applications, the HER requires an efficient and durable catalyst for room-temperature water electrolysis under either acidic or alkaline conditions. During the HER process, the catalyst should trigger proton reduction with minimal overpotential and fast electron-transfer kinetics to be an efficient catalyst.6–8 Thus, platinum-based catalysts are generally desirable at present, but they still suffer from issues regarding stability under alkaline conditions and cost. In the past few decades, non-precious metal-based catalysts have been studied. However, these are susceptible to the dull electron-transfer kinetics of oxygen evolution reactions and acidic corrosion,9 which are inferior to platinum-based catalysts. Moreover, these catalysts work at higher overpotentials with lower stability. Although the electrochemical heterogeneous HER is sensitive to the pH of the solution, it is reported that these ruthenium-based catalysts could serve in both acidic and alkaline media.10–12 As the concept of single-atom catalysis raised in the past several decades, the interactions between the atoms during catalytic reactions were clearly illustrated. The thermodynamical properties corresponding to the HER process could be calculated more precisely via theoretical modelling in order to evaluate the performance of catalysis. It is worth noting that regardless of whether in acidic or alkaline media, the chemisorption free energy of hydrogen to the surface of catalyst remains, essentially to be considered as a crucial descriptor of the efficiency of the HER process.13 With the theoretical modelling results, including the activation energy barriers for the adsorption and dissociation of water molecules, which could not be easily obtained from modern characterization techniques, the mechanism as well as active sites of the HER process would be uncovered. In another sense, these approaches would give an alternative criterion for designing new catalysts for heterogeneous HER catalysis.
The four nitrogen-coordinated (Co–N4) active configuration in cobalt phthalocyanine (CoPc) was first discovered to catalyze oxygen reduction reactions (ORRs) in the 1960s.14 Consequently, N-coordinated metals (M–N4) like Fe–N4 have been developed for catalysts. However, the poor electronic conductivity of these organic N-coordinated species limited the reaction performance. Nowadays, N-doped porous carbon materials are regarded as ideal substrates for electrocatalysts, especially supporting metal nanomaterials towards various electrocatalytic reactions including ORRs, oxygen evolution reaction (OERs), and HERs. The carbon-based catalysts with excellent conductivity and superior stability resolve the major issue of M–N4 macrocycle complexes. Recently, nitrogen-doped single-atom catalysts within the basement of graphite have been chosen for many studies because of the effective atomic utilization and unique electronic structures.15–17 The single metal–nitrogen (either pyridinic N or pyrrolic N) structures have been proved to show high catalytic performances in various applications.18 Moreover, the interactions between the single metal atom and nitrogen would significantly coordinate the electronic structures during catalytic reactions. Meanwhile, it is also important to explore durable and earth-abundant substitutes for the commercial platinum-based catalysts. Ruthenium possessing similar bond strengths to H is considered an economic alternative and investigated in numerous studies.19 Many works show that the performances of Ru-based catalysts are comparable to the commercial Pt/C catalyst for the HER.20
In this work, for clarifying the thermodynamic process in N-coordinated nitrogen-doped Ru on porous carbon-catalysed HERs, density functional theory (DFT) calculations were carried out to explore the detailed mechanism. The hydrogen adsorption step is the essential step of the reaction, which is consistent with the reported works.12,13 Meanwhile, we calculated the catalytic performance of three Ru-based models based on the principles proposed by Nørskov.21 The results indicated that the nitrogen-coordinated Ru system significantly enhances the HER performance. Moreover, the four-nitrogen-coordinated Ru configuration shows the best HER performance. The nitrogen-coordinated configuration enhances charge accumulation in Ru, which is the key factor for enhancement. These results would provide theoretical support for designing metal-based catalysts in the future.
Computation details
Density functional theory calculations were carried out using the Vienna Ab initio Simulation Package (VASP)22 program with projected augmented wave (PAW) potentials23 with a cutoff energy of 400 eV. The Perdew–Burke–Ernzerhof (PBE)24 functional was used within the formulation of generalized gradient approximation (GGA)25 for the exchange–correlation term. The energy difference of 1.0 × 10−5 eV per atom and max force of 0.02 eV Å−1 were set as the convergence criteria for geometry optimization. The transition states of the reaction pathway were searched using the dimer method,26 in which all the force components both along and perpendicular to the tangent of the reaction pathway were relaxed to be less than 0.02 eV Å−1. The electron charge density analysis of each optimized structure and transition state within the same convergence level was carried out in order to verify that the stationary points exhibit no imaginary frequency, while each transition state exhibits only one imaginary frequency.
In the calculation models, a four-layered carbon matrix supercell was established for the N-coordinating Ru/N/C system to study the HER mechanism. The bottom two layers were fixed in bulk positions, while the top two layers were allowed to relax. The Ru–N coordinated system was located on the top layer. A grid of 4 × 4 × 1 Gamma k-point sampling27 was used for the slabs with a vacuum level of 10 Å to eliminate the periodic image interactions. For comparison, the carbon-only model was also built, and the original and relaxed geometric structures are shown in Fig. 1. It is worth noting that the Ru atom lifts above the top carbon layer in all three models, which might promote the hydrogen adsorption process.
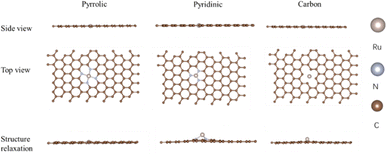 |
| Fig. 1 The geometric structure of the top layer of the three models; the blonde sphere represents Ru, silver sphere represents nitrogen, and the brown sphere represents carbon. | |
For comparison, we also established three different surface-terminated Ru bulks (100, 101, and 100). The models are shown in Fig. 2. As Ru is the hexagonal phase, the 101 and 011 indexes are equal to each other.
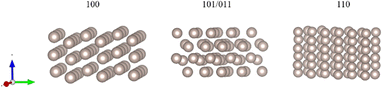 |
| Fig. 2 The geometric structure of the three different terminated surfaces. From left to right: (100), (101/011), and (110). | |
H adsorption was considered the key descriptor for HERs as suggested.13 We studied the reaction mechanisms to understand the performance of different surface configuration catalysts for driving the HER. The broadly accepted HER mechanism consists of two steps. That is the Volmer step and subsequent Heyrovsky step or Tafel step. Thus, the HER process can be described by the Volmer–Heyrovsky or Volmer–Tafel mechanism.
The Volmer step is the electrochemical hydrogen adsorption:
H+ + M + e− → M–H* (acidic solution) |
H2O + M + e− → M–H* + OH− (alkaline solution) |
After chemical desorption, the Tafel step or Heyrovsky step occurred as follows:
2M–H* → 2M + H2 (both acidic and alkaline solution) |
M–H* + H2O + e− → M* + H2 + OH− (alkaline solution) |
where the symbol “*” denotes the active site.
The hydrogen adsorption Gibbs free energy is defined as follows:
where Δ
EH is the difference in energies and defined as follows:
where
EH and
Esub are the total energies of the models with and without the hydrogen atom, respectively, and
EH2 means the total energy of H
2 in gas phase. ΔZPE and Δ
S are the changes in zero-point energy and entropy between the adsorbed phase and gas phase of hydrogen, respectively.
Results and discussion
Usually, the Tafel step or Heyrovsky step is very easy to proceed, as mentioned above. The energy barrier is relatively lower than the Volmer step.28 Thus, we considered the H adsorption as the key descriptor for the HER process, which is also shown in Fig. 3. The energy barrier for pyrrolic-Ru, pyridinic-Ru and carbon–Ru is +0.09, −0.24 and +0.33 eV, respectively. The results indicated that the pyrrolic-Ru configuration presents the lowest energy barrier. Basically, the HER performance on the carbon–Ru system is not good as the other two models. The role of porous carbons is considered as conductive substrates. The electron density difference (Fig. 4) of N-coordinated Ru shows electron accumulation at the top of the Ru site, which implies the effectiveness of Ru incorporation for promoting the HER catalytic performance. It is also worth noting that the electron charge also accumulates around the nitrogen sites, which are towards the Ru atom. As can be seen in Fig. 5, the N-coordinated system accumulates charge on the Ru-centred areas, which would significantly enhance the adsorption of hydrogen, compared to the carbon-based Ru system. The nitrogen atoms also have a higher charge density, which suggests the interaction between Ru and N atoms. The Bader charge analysis for pyrrolic-Ru is +0.90e, while is slightly greater than +0.84e of pyridinic-Ru. All these results also indicated that the pyrrolic-Ru system shows the best HER performance of the three models via the unique electronic structures with the four-N coordinated configuration. The Ru atom accumulates electron charge and gives rise to the chance of hydrogen adsorption.
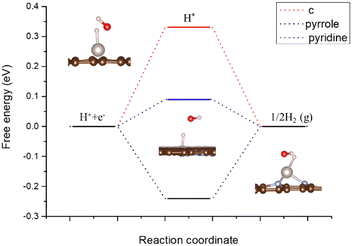 |
| Fig. 3 Free energy profiles and configurations of the elementary steps for HERs on pyrrolic-Ru (blue line), pyridinic-Ru (111) (black line), and carbon–Ru (red line) surfaces under acidic and alkaline conditions at equilibrium (U = 0). | |
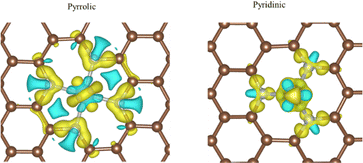 |
| Fig. 4 The electron charge density difference between pyrrolic-Ru and pyridinic-Ru models. The increase in electron density is indicated by yellow color, while the decrease is indicated by cyan color. | |
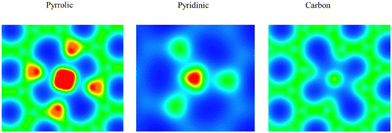 |
| Fig. 5 The electron charge density of pyrrolic-Ru and pyridinic-Ru models. The higher electron density is indicated by red color, while the lower electron density is indicated by blue color. | |
We also calculated the H adsorption in Ru bulk in order to compare with the N-coordinated Ru systems. According to previous studies, the primary termination surface indices for Ru bulk are (100), (101)/(011) and (110). Then, we calculated the surface deformation energy and surface energy for the three configurations. The deformation energy turns out to be −0.56 eV, −3.03 eV and −0.99 eV for the 100, 101, and 110 indices, respectively. In addition, the surface energy is 2.97 J m−2, 2.86 J m−2 and 3.43 J m−2. Absolutely, the 101/011 terminated surface is the most stable configuration with the lowest total energy. The H adsorption energy for 101 terminated Ru bulk is −0.62 eV. This barrier is much larger than either pyrrolic-Ru or pyridinic-Ru system. There is no doubt that the N-coordinated system is beneficial for the hydrogen adsorption process for pyrrolic-Ru and pyridinic-Ru systems, which could be the efficient way to improve the HER performance on those metal-based catalysts.
Conclusions
We have systemically investigated N-coordinated Ru catalysts on porous carbons for hydrogen evolution reactions via the first-principles calculation. Our calculations revealed that the H-adsorption step is the key descriptor. Both the N-coordinated Ru systems showed better performance based on calculation results. Moreover, pyrrolic-Ru is better than the pyridinic-Ru system. The electron charge density analysis and charge difference analysis both showed that the N-coordinated systems accumulate charge on the Ru atom and consequently enhance the HER performance. This HER performance of the N-coordinated single-atom configuration is not only better than that of the carbon-based Ru system, but also greater than the Ru bulk materials. Besides, the four N-coordinated system shows the best HER performance.
Author contributions
X. C. performed the DFT calculations, collected and analysed the data, and wrote the paper. K.W. conceived the ideas. X. X. gave supervision.
Conflicts of interest
There are no conflicts to declare.
Acknowledgements
This research was funded by National Key R&D Program of China (2020YFA0406202); Natural Science Foundation of China, grant number 22008006, 22090042 and 21731001; and the Fundamental Research Funds for the Central Universities, grant number FRF-IDRY-20-005 and FRF-TP-20-077A1.
Notes and references
- C. G. Morales-Guio, L. A. Stern and X. Hu, Nanostructured hydrotreating catalysts for electrochemical hydrogen evolution, Chem. Soc. Rev., 2014, 43, 6555–6569 RSC.
- J. A. Turner, Sustainable hydrogen production, Science, 2004, 305, 972–974 CrossRef CAS.
- M. S. Dresselhaus and I. L. Thomas, Alternative energy technologies, Nature, 2001, 414, 332–337 CrossRef CAS.
- J. K. Norskov and C. H. Christensen, Toward efficient hydrogen production at surfaces, Science, 2006, 312, 1322–1323 CrossRef CAS PubMed.
- L. Chen, X. Dong, Y. Wang and Y. Xia, Separating hydrogen and oxygen evolution in alkaline water electrolysis using nickel hydroxide, Nat. Commun., 2016, 7, 11741 CrossRef CAS.
- P. Rüetschi and P. Delahay, Hydrogen overvoltage and electrode material. A theoretical analysis, J. Chem. Phys., 1995, 23, 195–199 CrossRef.
- B. E. Conway and J. O. Bockris, Electrolytic hydrogen evolution kinetics and its relation to the electronic and adsorptive properties of the metal, J. Chem. Phys., 1957, 26, 532–541 CrossRef CAS.
- R. Parsons, The rate of electrolytic hydrogen evolution and the heat of adsorption of hydrogen, J. Chem. Soc., Faraday Trans., 1958, 54, 1053–1063 RSC.
- L. C. Seitz,
et al., A highly active and stable IrOx/SrIrO3 catalyst for the oxygen evolution reaction, Science, 2016, 353, 1011–1014 CrossRef CAS.
- W. J. Mitchell, J. Xie, T. A. Jachimowski and W. H. Weinberg, Carbon monoxide hydrogenation on the Ru(001) surface at low temperature using gas-phase atomic hydrogen: spectroscopic evidence for the carbonyl insertion mechanism on a transition metal surface, J. Am. Chem. Soc., 1995, 117, 2606–2617 CrossRef CAS.
- J. Mahmood, F. Li, S. M. Jung, M. S. Okyay, I. Ahmad and S. J. Kim,
et al., An efficient and pH-universal ruthenium-based catalyst for the hydrogen evolution reaction, Nat. Nanotechnol., 2017, 12(5), 441–446 CrossRef CAS.
- B. Lu, L. Guo, F. Wu, Y. Peng, J. E. Lu and T. J. Smart,
et al., Ruthenium atomically dispersed in carbon outperforms platinum toward hydrogen evolution in alkaline media, Nat. Commun., 2019, 10(1), 631 CrossRef CAS.
- J. K. Noskov, Trends in the Exchange Current for Hydrogen Evolution, J. Electrochem. Soc., 2005, 152(3), J23–J26 CrossRef.
- R. Jasinski, Cobalt Phthalocyanine as a Fuel Cell Cathode, J. Electrochem. Soc., 1965, 112, 526 CrossRef CAS.
- Y. Fang, Y. Xue, L. Hui, H. Yu, Y. Liu and C. Xing,
et al., In situ growth of graphdiyne based heterostructure: toward efficient overall water splitting, Nano Energy, 2019, 59, 591–597 CrossRef CAS.
- X. Y. Yu, X. Feng, Y. Jeon, B. Guan, X. W. Lou and U. Paik, Formation of Ni–Co–MoS2 nanoboxes with enhanced electrocatalytic activity for hydrogen evolution, Adv. Mater., 2016, 28(40), 9006–9011 CrossRef CAS.
- H. Fei,
et al., Atomic cobalt on nitrogen-doped graphene for hydrogen generation, Nat. Commun., 2015, 6, 8668 CrossRef CAS.
- Z. Zhuang, Y. Wang, C. Q. Xu, S. Liu, C. Chen and Q. Peng,
et al., Three-dimensional open nano-netcage electrocatalysts for efficient pH-universal overall water splitting, Nat. Commun., 2019, 10(1), 4875 CrossRef CAS.
- Q. Zhi, S. Qin, W. Liu, R. Jiang, T. Sun and K. Wang,
et al., Ultralow loading of ruthenium nanoparticles on nitrogen-doped porous carbon enables ultrahigh mass activity for the hydrogen evolution reaction in alkaline media, Catal. Sci. Technol., 2021, 11(9), 3182–3188 RSC.
- J. N. Tiwari,
et al., Multicomponent electrocatalyst with ultralow Pt loading and high hydrogen evolution activity, Nat. Energy, 2018, 3, 773–782 CrossRef CAS.
- J. K. Nørskov, T. Bligaard and J. Rossmeisl,
et al., Towards the
computational design of solid catalysts, Nat. Chem., 2009, 1, 37–46 CrossRef PubMed.
-
(a) G. Kresse and J. Furthmüller, Efficiency of ab-initio total energy calculations for metals and semiconductors using a plane-wave basis set, Comput. Mater. Sci., 1996, 6, 15–50 CrossRef CAS;
(b) G. Kresse and J. Furthmüller, Efficient iterative schemes for ab initio total-energy calculations using a plane-wave basis set, Phys. Rev. B: Condens. Matter Mater. Phys., 1996, 54, 11169–11186 CrossRef CAS.
- P. E. Blochl, P. E. Projector augmented-wave method, Phys. Rev. B: Condens. Matter Mater. Phys., 1994, 50, 17953–17979 CrossRef.
- J. P. Perdew, K. Burke and M. Ernzerhof, Generalized gradient approximation made simple, Phys. Rev. Lett., 1996, 77, 3865–3868 CrossRef CAS.
- B. J. Delley, An all-electron numerical method for solving the local density functional for polyatomic molecules, Chem. Phys., 1990, 92, 508–517 CAS.
- H. J. Monkhorst and J. D. Pack, Special points for Brillouin-zone integrations, Phys. Rev. B: Solid State, 1976, 13(12), 5188–5192 CrossRef.
- G. Henkelman,
et al., A climbing image nudged elastic band method for finding saddle points and minimum energy paths, J. Chem. Phys., 2000, 113, 9901–9904 CrossRef CAS.
- M. Jamesh and X. Sun, Recent progress on earth abundant electrocatalysts for hydrogen evolution reaction (HER) in alkaline medium to achieve efficient water splitting—A review, J. Energy Chem., 2019, 34, 111–160 CrossRef.
|
This journal is © the Partner Organisations 2024 |
Click here to see how this site uses Cookies. View our privacy policy here.