DOI:
10.1039/D3QM00726J
(Review Article)
Mater. Chem. Front., 2024,
8, 82-103
The role of organic spacers in 2D/3D hybrid perovskite solar cells
Received
29th June 2023
, Accepted 18th September 2023
First published on 22nd September 2023
Abstract
The long-term stability of three-dimensional (3D) perovskite solar cells (PSCs) has become the major challenge for their commercial applications. To solve this problem, the development of 2D/3D hybrid perovskites by incorporating bulky organic spacers into 3D perovskite films has been proposed as an efficient strategy to combine the high efficiency of 3D PSCs and high stability of 2D PSCs. In this review, we present the structure of 3D and 2D perovskite materials, discuss the transformation process from 3D to 2D perovskite, and summarize the key organic spacers used in 2D/3D PSCs. Most importantly, based on the appropriate selection of functional groups in organic spacers, we highlight the role of organic spacers in the fabrication process and formation mechanism of different types of 2D/3D perovskite heterojunctions including the bulk incorporation model, surface treatment model, and bulk incorporation and surface treatment coexisting model as well as other feasible models.
1. Introduction
The power conversion efficiency (PCE) of three-dimensional (3D) organic–inorganic metal hybrid perovskite solar cells (PSCs) has increased sharply from the initial 3.8%1 to a certified value of 26.1%2 in the past decade. Moreover, many studies have demonstrated that the excellent PCE of 3D PSCs primarily stems from the unique properties of perovskite materials, such as high carrier mobility,3 high absorption coefficient,4 long carrier lifetime5,6 and low exciton binding energy.7 These optoelectronic characteristics make perovskite materials outstanding light absorbers for the next generation of photovoltaic devices. However, despite the ultrahigh PCE of state-of-the-art PSCs, which is comparable to that of silicon-based solar cells, long-term stability remains a major challenge for commercialization due to issues such as moisture8 and oxygen9 attack, heat stress,10 light soaking11 and ion migration.12
In comparison, low dimensional perovskites, especially 2D perovskites consisting of 2D phases and quasi-2D phases, have been proven to be more durable than 3D perovskites due to the incorporation of bulky and hydrophobic organic spacers.13 In 2014, Smith et al. demonstrated Ruddlesden–Popper (RP) type 2D PSCs by introducing a phenylethylammonium (PEA) organic spacer.14 Although the corresponding photovoltaic device exhibited a low PCE of 4.73%, the layered structure of 2D perovskite contributed to the formation of high-quality films that exhibited greater moisture resistance compared to the 3D MAPbI3 (MA refers to methylammonium) film. Consequently, researchers began to devote significant efforts to the study of 2D PSCs and subsequently developed various organic spacers.15–18 However, even though 2D perovskites demonstrate enhanced resilience to heat and humidity, the PCE of 2D PSCs still lags behind that of state-of-the-art 3D PSCs.19
To combine the high efficiency of 3D PSCs with superior stability of 2D PSCs, a dimensional engineering strategy has been proposed and developed. Through dimensional engineering, 2D/3D hybrid heterojunctions can be formed at different positions, wherein 3D perovskites ensure a wider absorption range for achieving high efficiency, while 2D perovskites guarantee that the device will not degrade rapidly under severe conditions. In addition to improving device stability, the construction of 2D/3D heterojunctions is also helpful in energy level regulation, ion migration suppression, defect passivation and residual stress release.20 Generally, 2D/3D heterojunctions with the aforementioned high performance primarily fall into three types: (1) arranged in the bulk of 3D perovskite (referred to as the bulk incorporation model), (2) located on the top of 3D perovskite (referred to as the surface treatment model), and (3) situated both in the bulk and on the top of 3D perovskite (referred to as the bulk incorporation and surface treatment coexisting model).
However, despite the development of many organic spacers for constructing 2D/3D heterojunctions, a clear summary of the role of different types of organic spacers in the preparation process of these 2D/3D perovskites is still lacking. In this review, we first introduce the structures of 3D and 2D perovskite materials and explain the transformation process from 3D to 2D perovskite. Subsequently, we summarize the primary organic spacers that have been used in reported 2D/3D PSCs so far and further analyze their effects on the photoelectric performance of devices. Finally, based on the appropriate selection of functional groups in organic spacers, we mainly focus on the role of organic spacers in the fabrication process and formation mechanism of various 2D/3D perovskite heterojunctions, including the bulk incorporation model, surface treatment model, bulk incorporation and surface treatment coexisting model, and other feasible models.
2. Structures of 3D and 2D perovskites
2.1. Structure of 3D perovskite
To gain a deeper understanding of the properties of perovskite materials, it is crucial to delve into their structures. Conventional perovskite materials are generally defined as a series of compounds with the formula of ABX3. In this formula, the A site typically represents a monovalent cation, such as CH3NH3+ (MA+), NH
CHNH3+ (FA+) and Cs+. The B site usually denotes a divalent metal cation (Pb2+, Sn2+), while X site refers to halogen ions including Cl−, Br−, and I−.21 Within the perovskite framework, B site ions coordinate with X site ions to form a [BX6]4− octahedron, in which the B site ions reside in the center of the octahedron and the X site ions occupy the apex positions. These [BX6]4− octahedrons are further interconnected by vertices, extending into 3D space. Meanwhile, A site cations occupy the vacant space within the network formed by the [BX6]4− octahedrons (Fig. 1).22
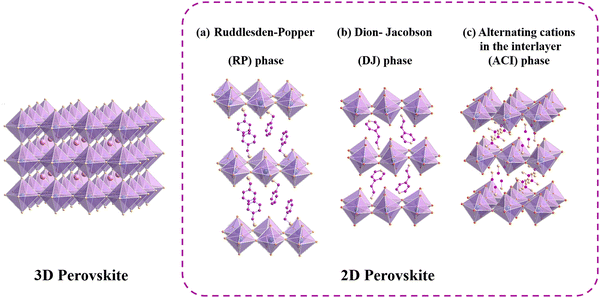 |
| Fig. 1 Representative structures for 3D perovskite and 2D perovskite of the RP phase (a), DJ phase (b) and ACI phase (c). | |
2.2. Structure of 2D perovskite
The tolerance factor (t) is often used to account for octahedral distortions in the crystal structure of perovskite to predict the 3D to 2D transition, in which t is defined by the following Goldschmidt's formula:23
The archetypal 3D perovskites have t values in the range of 0.8 < t < 1.1. When the A site cations become too large to fit well within the [BX6]4− octahedral cavity, the initial cubic structure of the 3D perovskite could transform into a layered 2D structure to maintain energy stability, thus leading to the deviation from the above range. Therefore, based on the arrangement of the 3D perovskite unit cells, 2D perovskites can be classified into (100), (110) and (111) orientations, where large organic spacers separate the inorganic layered [BX6]4− octahedron and tightly connect the different layers through hydrogen bonding and van der Waals forces.24 Depending on the types of organic spacers, 2D perovskite can be categorized into three main classifications: RP phase, Dion–Jacobson (DJ) phase, and alternating cations in the interlayer-space (ACI) phase (Fig. 1). These are expressed as A′2An−1BnX3n+1, A′′An−1BnX3n+1 and A′′′ABnX3n+1, respectively. Here, A′ represents monovalent organic cations like PEA and butylammonium (BA), A′′ denotes a divalent organic cation, and A′′′ typically refers to guanidinium (GA). In the case of ACI perovskite, the large GA spacer and small A cations are arranged in the spacer layer, exhibiting the dual characteristics of RP and DJ structures. The value of n signifies the number of inorganic layers or the metal-halide frameworks located between the organic spacer layers.25
2.3. Organic spacer cations
2D perovskite materials offer significant advantages in terms of environmental stability. However, the presence of large organic spacers in the structure hinders the migration of photogenerated carriers and increases the exciton binding energy, ultimately leading to inferior photovoltaic performance of devices.26 Therefore, the selection of effective organic spacers, while ensuring the high environmental stability of 2D perovskite and excellent photovoltaic performance of 3D perovskites, has emerged as a research focus for the development of efficient 2D/3D PSCs. In pursuit of this goal, scholars have conducted a series of investigations encompassing the regulation of organic spacers, optimization of surface morphology and orientation, and the development of a diverse range of organic spacers (Fig. 2 and 3).20
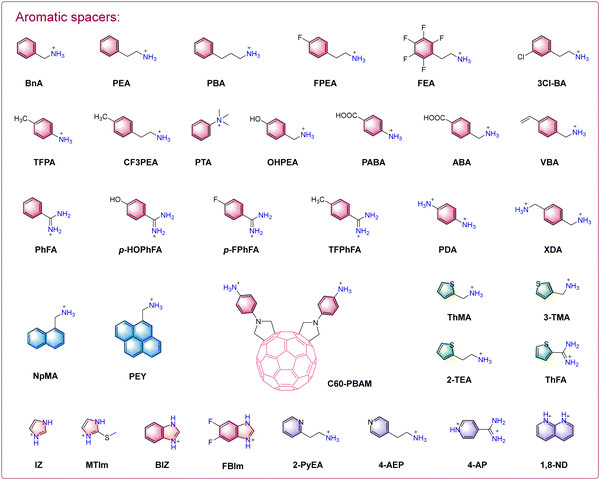 |
| Fig. 2 Representative aromatic spacers for 2D/3D hybrid PSCs. | |
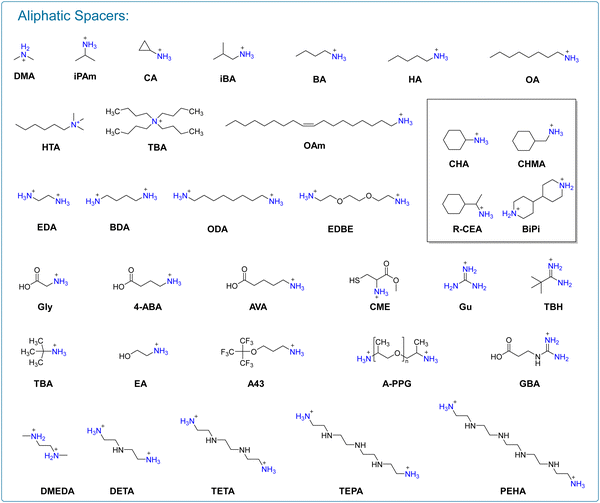 |
| Fig. 3 Representative aliphatic and alicyclic spacers for 2D/3D hybrid PSCs. | |
3. 2D/3D heterojunctions in mixed dimensional perovskite solar cells
Mixed dimensional 2D/3D perovskite films can be fabricated through two common processes: bulk incorporation27–31 and surface treatment.32–35 In the bulk incorporation method, small amounts of large organic ammonium salts or 2D perovskite seed crystals are added to the 3D perovskite precursor. This allows for the growth of highly crystalline and the large grained 2D/3D perovskite with favorable out-of-plane layer orientation.27,31 On the other hand, in surface treatment, 2D perovskite is formed in situ on the deposited 3D perovskite film by spin-coating a solution containing bulky organic ammonium salts36 or 2D perovskite seed crystals.34 Some researchers have also explored alternative methods such as evaporating bulky organic ammonium salts on the top of 3D perovskite films37,38 or hot-pressing 2D perovskite films onto 3D perovskite films to create 2D/3D heterojunctions.34 The performance enhancement achieved through the formation of a thin mixed 2D/3D heterojunction at the interface between the perovskite layer and the hole transport layer can be attributed to surface defect passivation, accelerated charge extraction, and the introduction of hydrophobic spacers to enhance humidity stability.
Based on the aforementioned statements, the main types of 2D/3D heterojunctions are illustrated in Fig. 4 as follows: (1) 2D/3D heterojunctions generated in the bulk of 3D perovskite films, causing the bulk incorporation model; (2) 2D/3D heterojunctions formed on the surface of 3D perovskites, leading to the surface treatment model; and (3) 2D/3D heterojunctions arranged on both the surface and bulk of 3D perovskite, resulting in the bulk incorporation and surface treatment coexisting model. We will analyze these structures and the role of large organic spacers in each structure in detail in the following discussion.
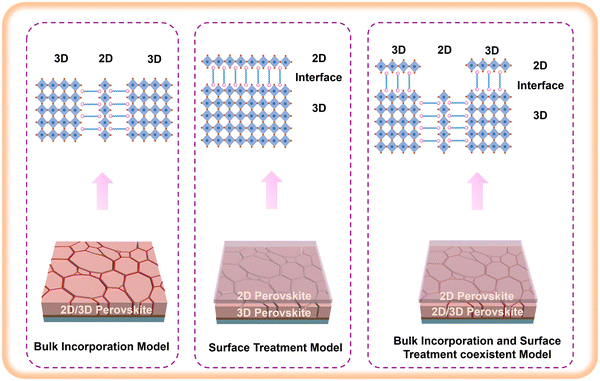 |
| Fig. 4 Schematic diagram of the bulk incorporation model, surface treatment model, and bulk incorporation and surface treatment coexisting model. | |
3.1. Bulk incorporation model
3.1.1. Aromatic spacers.
In 2018, Ho-Baillie et al. reported the benefits of incorporating phenethylamine iodide salt (PEAI) into the (FAPbI3)0.85(MAPbBr3)0.15 perovskite and demonstrated the presence of a higher-band-gap PEA-based quasi-2D perovskite at grain boundaries using Kelvin probe force microscopy (KPFM).39 After performing measurements in dark mode, the contact potential difference (CPD) at grain boundaries (CPDGB) and in the grain interior (CBDGI) were determined and the averaged differences of CPDGB and CBDGI were calculated. Notably, the value of ‘CPDGB – CBDGI′ in the film with 4.5% PEAI (−44 mV) was lower than that without PEAI (−13 mV), indicating a change in the electronic properties of the grain boundaries due to the formation of a quasi-2D phase at the grain boundaries. Additionally, in the same year, Yang et al. developed a fabrication technique for the pure α-phase FAPbI3 perovskite by incorporating PEA2PbI4 2D perovskite seed crystals into the precursor solution.28 In this process, 2D PEA2PbI4 passivated the defective grain boundaries of the 3D perovskite through aromatic rings and longer alkyl chains, resulting in a significantly improved hydrophobic property of the film. Furthermore, the 3D perovskite and PEA2PbI4 exhibited type I band alignment, which reduced charge recombination and facilitated charge separation and collection. Meanwhile, 2D perovskite regions at grain boundaries experienced a downward band bending under illumination, creating a high-potential barrier for holes and resulting in superior PL lifetime and photovoltaic performance.
In 2021, Liu et al. reported for the first time a class of aromatic formamidinium (ArFA) salts, which included benzamidine hydrochloride (PhFACl), 4-hydroxybenzamidinium hydrochloride (p-HOPhFACl), and 4-fluorobenzamidinium hydrochloride (p-FPhFACl), for 2D/3D hybrid PSCs (Fig. 5a).40 As shown in Fig. 5b, the 1H NMR spectra revealed strong NH⋯I hydrogen-bonding interactions between the iodide anions in the corner-sharing [PbI6]4− octahedron layers and the ammonium fragment of organic spacers. The crystallographic quality and orientation of the perovskite crystals were confirmed using grazing-incidence wide-angle X-ray scattering (GIWAX). In contrast to the diffraction rings observed in the pristine film, the sharp and distinct Bragg spots in the ArFA films (Fig. 5c) suggested that the incorporation of ArFA spacers led to a preferred vertical orientation of the crystal with respect to the substrate. As a result, the optimized PhFA-based 2D/3D PSC exhibited a champion PCE of 23.36% with a high short-circuit current density (JSC) of 24.67 mA cm−2, an increased open-circuit voltage (VOC) of 1.16 V and a notable fill factor (FF) of 81.54%.
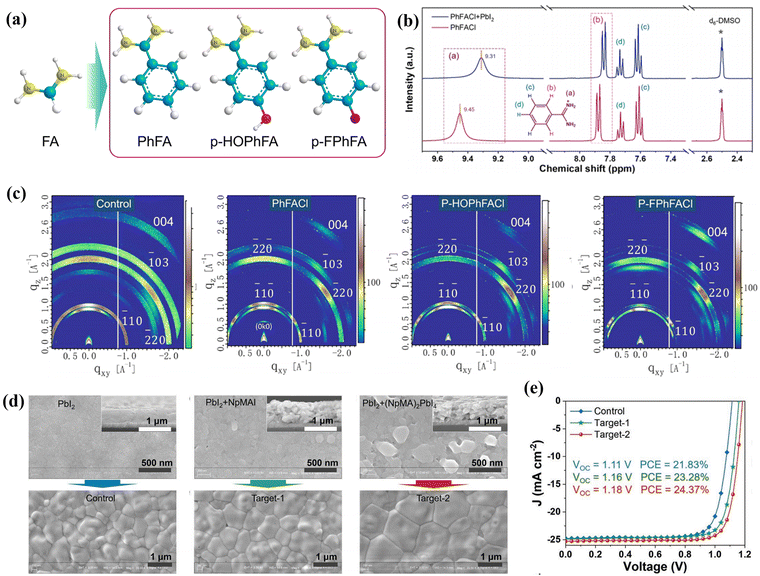 |
| Fig. 5 (a) Chemical structures of spacer cations FA, PhFA, p-HOPhFA, and p-FPhFA. (b) 1H NMR spectra of pristine PhFACl and mixed PhFACl/PbI2. (c) GIWAX patterns of the control, PhFACl-, p-HOPhFACl-, and p-FPhFACl-based perovskite films. Reproduced with permission.40 Copyright 2021, American Chemical Society. (d) Top-views and cross-sectional SEM images of pristine, NpMAI-modified and (NpMA)2PbI4-modified PbI2 films and SEM images of the corresponding perovskite films. (e) J–V curves of the pristine, NpMAI-modified and (NpMA)2PbI4-modified devices. Reproduced with permission.41 Copyright 2022, John Wiley & Sons, Inc. | |
In addition to the spacers with a single aromatic ring, multiple-ring aromatic spacers such as naphthyl-41 and pyrenyl-based spacers42 have also been applied in bulk 2D/3D PSCs. In 2022, Liu et al. reported the crystal growth regulation of 2D/3D perovskite films through a two-step spin-coating method by using 1-naphthalenemethylammonium iodide (NpMAI) or 2D perovskite (NpMA)2PbI4 as seed crystals in PbI2 precursor solution.41 They found that the pristine PbI2 film showed a dense film morphology, while the PbI2 film incorporated small quantity of (NpMA)2PbI4 or NpMAI, showing a mesoporous morphology, especially for the (NpMA)2PbI4 incorporated film. This mesoporous morphology benefitted the penetration and reaction of organic salts with PbI2 in the second deposition step, resulting in a higher quality perovskite film with large grain size (Fig. 5d). Moreover, the application of (NpMA)2PbI4 seed doping can not only improve the crystallinity of the perovskite film but also prolong the lifetime of charge carriers, thereby leading to a better photovoltaic performance of devices. Finally, the 2D/3D hybrid films with (NpMA)2PbI4 2D perovskite exhibited a superior PCE of 24.37%, higher than that of the NpMAI-modified film (23.28%) and the control one (21.83%) (Fig. 5e). For pyrenyl-based spacers, 1-(ammonium acetyl)pyrene (PEY) was proposed to fabricate MAPbI3-based 2D/3D perovskite by Hayase's group in 2018.42 They demonstrated the enhancement of ultraviolet irradiation and oxygen stability due to the large functional organic pyrene group with high humidity resistance and strong absorption in the ultraviolet region.
Furthermore, the spacers based on heterocyclic rings such as thiophene and pyridine can also regulate the crystal arrangement and orientation through π–π interactions as well as hydrogen bonding interactions. In 2019, Liu et al. demonstrated highly efficient and stable 2D/3D hybrid PSCs using 2-thiophenemethylammonium (ThMA) as a spacer.43 ThMA could partially insert into the 3D perovskite crystal lattice and form 2D perovskite phases, which aligned perpendicular to the substrate as confirmed by XRD and GIWAX measurements. Additionally, the introduction of a large ThMA spacer inhibited ion migration and improved the overall hydrophobic performance of 2D/3D films, leading to enhanced humidity stability in atmospheric environments. Remarkably, the ThMA spacer-based 2D/3D PSCs exhibited significantly improved performance, including a large VOC of 1.16 V, a JSC of 22.8 mA cm−2, and a very notable FF of 81.0%, resulting in an impressive PCE of 21.49%. In contrast to benzene-based spacers, the pyridine ring possesses a lone electron pair on the N atom, allowing pyridine to partially replace DMSO by coordinating with Pb2+.44 Moreover, the weak alkalinity of N atoms in the pyridine unit enables the interaction between the pyridine ring with an amino terminal group and the [PbI6]4− framework, ultimately forming DJ-type 2D perovskite.30 Recently, Zhao et al. conducted a detailed investigation of the crystallization dynamics of 2D/3D hybrid perovskite by designing a 2D/3D heterojunction based on the FAPbI3 3D perovskite and DJ-type (4AP)PbI4 2D perovskite (4AP = 4-amidinopyridine).30 As shown in Fig. 6a and b, the FA-rich α phase perovskite rapidly crystalized on the film's surface after dropping the anti-solvent, resulting in a sharp increase in PL intensity. Subsequently, the entrapped DMSO solvent volatilized from the bulk film during the annealing process, causing the surface perovskite to dissolve and recrystallize leading to a gradual decrease in PL intensity. Eventually, the evaporation of DMSO caused volume collapse of the internal perovskite, resulting in void formation at grain boundaries. In contrast, the addition of (4AP)PbI4 2D perovskite could slow down the nucleation and growth process of FA-rich α phase 3D perovskite, and the presence of (4AP)PbI4 provided a skeleton that aided in the hetero-nucleation of 3D perovskite and filled the voids at grain boundaries caused by DMSO evaporation, resulting in more uniform and larger grains. Through this method, the 2D/3D films achieved a PCE of 24.9% in a rigid device and a PCE of 22.3% in a flexible device, with the PCE achieved by rigid device setting a record for 2D/3D hybrid PSCs to date (Fig. 6c–e).
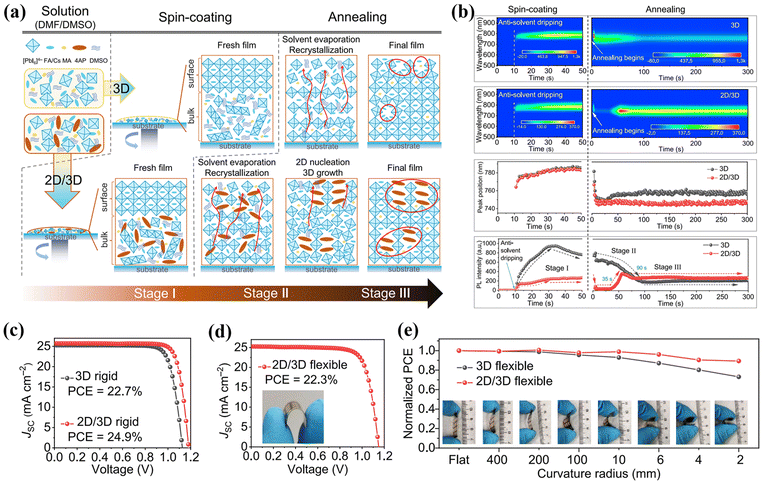 |
| Fig. 6 (a) Schematic illustration of the crystallization mechanism of 4-AP modified perovskite films. (b) In situ PL measurements for the formation kinetics of perovskite films. J–V curves of rigid solar cells(c) and flexible solar cell (d). (e) Normalized PCE with curvature from flat to R = 2 mm. Reproduced with permission.30 Copyright 2023, Elsevier. | |
3.1.2. Aliphatic spacers.
Among aliphatic molecules, BA is a classical organic spacer commonly used to fabricate RP type 2D perovskites,45–47 suggesting its potential for outstanding performance in 2D/3D perovskite systems. In 2017, Snaith et al. introduced BA spacer into a mixed-cation and mixed-halide FA0.83Cs0.17Pb(IyBr1−y)3 3D perovskite.27 Notably, plate-like 2D perovskites were observed to intersperse between highly orientated 3D perovskite grains, significantly enhancing crystallinity and suppressing non-radiative charge recombination through the formation of a classical type-I heterojunction (Fig. 7a–c). The device based on BA0.09(FA0.83Cs0.17)0.91Pb(I0.6Br0.4)3 with a wide band gap of 1.72 eV achieved an optimized PCE of 17.2%, while the device utilizing the BA0.05(FA0.83Cs0.17)0.95Pb(I0.8Br0.2)3 perovskite with a narrow band gap of 1.62 eV as the photoactive layer demonstrated a champion PCE of 20.6%. In 2021, Seok et al. found that the chemical reaction between the isopropyl alcohol (IPA) solvent and methylammonium chloride (MACl) in the precursor would generate isopropylammonium cations (iPAmH+) in a two-step spin-coating process. Furthermore, iPAmH+ could also be generated by adding IPA directly to the precursor in a one-step spin-coating process, where iPAmH+ played a role in stabilizing the α-phase FAPbI3.48 Based on these findings, they directly added 15 mol% isopropylammonium chloride (iPAmHCl) to the precursor for the fabrication of the FAPbI3 film, ensuring the specific effects of the iPAmH+ cation. Similar to MACl, a substantial amount of the introduced iPAmHCl volatilized during the annealing process, with little iPAmHCl remaining at the FAPbI3 grain boundaries, contributing to the stabilization of the α-phase and enabling the fabrication of PSCs with a certified PCE of 23.9%.
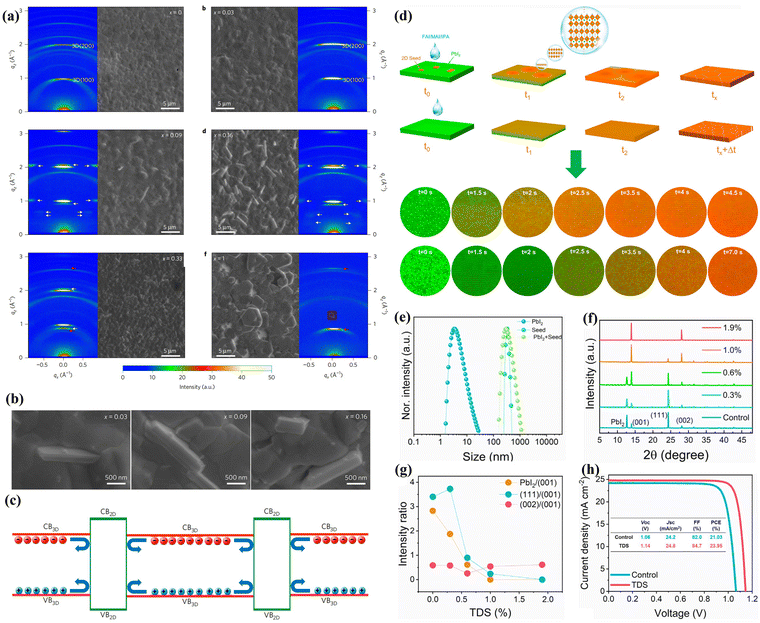 |
| Fig. 7 (a) SEM images and 2D X-ray diffraction measurements of post-annealed perovskite films with different BA concentrations. (b) Enlarged SEM images of x = 0.03, 0.09 and 0.16 films. (c) Proposed electronic band offsets of the 2D/3D heterojunction. Reproduced with permission.27 Copyright 2017, Springer Nature. (d) Schematic diagram of with/without BDA-seed-induced growth and the images of the PbI2 film with/without 2D seed under a confocal laser scanning microscope at different reaction times after depositing organic salts. (e) Dynamic light scattering spectra of the solutions. XRD patterns (f) and different peak intensity ratios (g) of perovskite films with various seed concentrations. (h) J–V curves of control and BDA-modified PSCs. Reproduced with permission.29 Copyright 2022, Elsevier. | |
The diammonium spacers with two ammonium groups can bond with a [PbI6]4− octahedron on two sides, forming a DJ-type 2D perovskite. This structure provides favorable nucleation sites for the highly oriented growth of 3D perovskites.49,50 In 2022, Zhao et al. introduced (BDA)PbI4 (BDA = butane-1,4-diaminium) seed crystals into the PbI2 precursor as nucleation centers for 2D/3D perovskite formation. The results revealed that the perovskite film bypassed the nucleation stage and directly entered the growth stage after being covered with organic salts.29 The process of seed crystal-induced crystallization rapidly consumed the solute and significantly decreased the solution concentration. Consequently, it inhibited the random nucleation of the remaining 3D perovskite region without seeds, leading to the dominance of seed crystal-induced crystallization in the film formation process. It is noteworthy that the (BDA)PbI4 itself inherently exhibited a high orientation of (00L). Therefore, the template-induced 3D perovskite demonstrated dominant and well-defined (001) and (002) peaks instead of the unfavorable (111) peaks. Eventually, the 2D phase transformed into grain boundaries after completing the orientation-induced growth, forming a type-I band alignment with 3D perovskite to passivate GB defects. This transformation resulted in a boost in PCE from 21.03% to 23.95% in mixed-dimensional PSCs (Fig. 7d-h). More reported organic spacer cations applied in bulk-incorporation type PSCs can be found in Table 1.
Table 1 An exhaustive list of recently reported organic spacer cations applied in bulk-incorporation type PSCs
Spacers |
Device architecture |
V
OC (V) |
FF (%) |
J
SC (mA cm−2) |
PCE (%) |
Ref. |
PEA |
ITO/SnO2/FA0.98Cs0.02PbI3/spiro-OMeTAD/Au |
1.130 |
76.11 |
24.00 |
20.64 |
28
|
PABA |
FTO/c-TiO2/MAPbI3/spiro-OMeTAD/Au |
1.080 |
77.62 |
20.70 |
17.35 |
51
|
PhFA |
ITO/SnO2/FA0.84MA0.16PbI3/spiro-OMeTAD/MoO3/Ag |
1.160 |
81.54 |
24.67 |
23.36 |
40
|
p-OHPhFA |
ITO/SnO2/FA0.84MA0.16PbI3/spiro-OMeTAD/MoO3/Ag |
1.160 |
81.89 |
23.21 |
22.05 |
40
|
p-FPhFA |
ITO/SnO2/FA0.84MA0.16PbI3/spiro-OMeTAD/MoO3/Ag |
1.160 |
81.73 |
23.24 |
22.03 |
40
|
MTIm |
FTO/c-TiO2/m-TiO2/[(MTIm)2(Cs0.05FA0.95)29Pb30I91]0.85(MAPbBr3)0.15/spiro-OMeTAD/Au |
1.140 |
79.12 |
23.56 |
21.25 |
52
|
4-AEP |
ITO/SnO2/MAPbI3/spiro-MeOTAD/Au |
1.080 |
81.00 |
23.58 |
20.70 |
44
|
4AP |
FTO/TiO2/FAPbI3/spiro-OMeTAD/Au |
1.180 |
81.81 |
25.70 |
24.90 |
30
|
ThMA |
ITO/SnO2/FA0.84MA0.16PbI3/spiro-OMeTAD/MoO3/Ag |
1.160 |
81.00 |
22.80 |
21.49 |
43
|
NpMA |
ITO/SnO2/FA0.84MA0.16PbI3/spiro-OMeTAD/MoO3/Ag |
1.180 |
81.36 |
25.30 |
24.37 |
41
|
PEY |
FTO/SnO2/(PEY2PbI4)0.02MAPbI3/spiro-OMeTAD/Au |
1.050 |
66.10 |
21.15 |
14.70 |
42
|
iPAm |
— |
1.159 |
79.80 |
25.79 |
23.85 |
48
|
BA |
FTO/SnO2/PCBM/BA0.05(FA0.83Cs0.17)0.95Pb(I0.8Br0.2)3/spiro-OMeTAD/Au |
1.140 |
80.00 |
22.70 |
20.60 |
27
|
TBA |
ITO/PEDOT:PSS/TBA2MA19Pb20I61/PCBM/BCP/Ag |
1.032 |
80.53 |
23.70 |
19.70 |
53
|
HEA |
FTO/c-TiO2/m-TiO2/(HEA)2(Cs0.1FA0.9)29Pb30I91/spiro-MeOTAD/Au |
1.100 |
79.63 |
22.81 |
19.84 |
54
|
Gly |
FTO/TiO2/CsPbI2Br(Gly-X)/spiro-OMeTAD/Au |
1.330 |
80.92 |
16.04 |
17.26 |
55
|
GBA |
PEN/ITO/PTAA/FA0.7MA0.25Cs0.05Pb(I0.93Br0.07)3/PCBM/BCP/Ag |
1.140 |
80.02 |
23.51 |
21.45 |
56
|
EDA |
ITO/PEDOT:PSS/FASnI3/PCBM/BCP/Ag |
0.634 |
69.43 |
19.32 |
8.47 |
49
|
BDA |
ITO/SnO2/FA0.75MA0.25PbI3/spiro-OMeTAD/Au |
1.140 |
84.70 |
24.20 |
23.95 |
29
|
ODA |
ITO/SnO2/Cs0.05FA0.85MA0.10Pb(I0.97Br0.03)3/spiro-OMeTAD/Ag |
1.120 |
75.61 |
24.80 |
20.94 |
50
|
TETA |
TO/SnO2/Cs0.05FA0.85MA0.10Pb(I0.97Br0.03)3/spiro-OMeTAD/Ag |
1.100 |
74.03 |
25.22 |
20.57 |
50
|
EDBE |
TO/SnO2/Cs0.05FA0.85MA0.10Pb(I0.97Br0.03)3/spiro-OMeTAD/Ag |
1.150 |
76.66 |
25.88 |
22.68 |
50
|
TBA |
ITO/PEDOT:PSS/TBA2MA19Pb20I61/PCBM/BCP/Ag |
1.032 |
80.53 |
23.70 |
19.70 |
53
|
BiPi |
FTO/TiO2/MAPbI3/spiro-OMeTAD/Au |
1.100 |
77.00 |
23.51 |
20.03 |
57
|
3.2. Surface treatment model
The surface passivation through the deposition of an organic spacer or a 2D perovskite layer is another crucial method to build 2D/3D heterojunctions in order to achieve both high efficiency and ultrastability in PSCs.58 In general, defects on the surface of 3D perovskite, such as halide vacancies and undercoordinated Pb2+, can be significantly passivated by introducing large spacer cations and their corresponding 2D perovskites. Simultaneously, the formation of 2D/3D heterojunctions through the surface passivation method rearranges the energy level structure at the interface between the 3D perovskite and the charge carrier transport layer, creating a staircase-like energy level structure that effectively enhances the carrier transport capacity. Additionally, the presence of large spacer cations at the interface can prevent water invasion and ion migration, thereby promoting excellent long-term stability of 2D/3D devices in various complex environments.
3.2.1. Aromatic spacers.
In fact, aromatic compounds have long been used to construct 2D/3D heterojunctions using a surface deposition method.59–61 In 2016, Docampo et al. reported 2D/3D perovskite by spin-coating a mixture solution of MAI:PEAI/IPA on the surface of 3D perovskite, and successfully observed (00l) diffraction peaks of (PEA)2(MA)4Pb5I16 by XRD measurement.59 Two years later, Wang et al. developed a method by spin-coating PEAI solution on 3D perovskite and in situ formed a layered 2D PEA2PbI4.62 Such a PEA2PbI4 2D perovskite capping layer induced Fermi-level splitting of the 2D/3D perovskite film under light illumination, leading to an enhanced VOC and thus a PCE of 18.51% in 2D/3D PSCs. In 2022, Sun et al. reported in situ formed 2D perovskite by the deposition of a mixed solution of a hole transport material (HTM) (spiro-OMeTAD) and PEA spacers.63 In this process, the high polar solvent like IPA was not used anymore, which reduced the damage to the 3D perovskite film, thus leading to a PCE of 21.09%.
In light of the unique properties of PEA spacers,64,65 researchers have explored the molecular structure and extended the use of phenyl spacers such as benzylammonium (BnA),66 phenylbutylammonium (PBA),67 4-fluorophen-ethylammonium (FPEA),68,69 and pentafluorophenylethylammonium (FEA),32 among others.33,35,70–74 In 2019, Grätzel et al. employed FEA in highly efficient bilayer 2D/3D PSCs and successfully demonstrated the dominant generation of 2D perovskite on the surface of the 2D/3D bilayer through X-ray reflectivity (XRR) and grazing incidence X-ray diffraction (GIXRD) characterization. The (FEA)2PbI4 2D perovskites exhibited approximately four layers in 2D/3D bilayers (about 8 nm) and displayed a larger angular distribution in the out-of-plane direction. Additionally, the perfluorinated 2D perovskite layer exhibited super hydrophobicity and inhibited ion motion, leading to comprehensive enhancement in moisture resistance and intrinsic stability of the obtained 2D/3D PSCs. In 2019, Sargent et al. creatively utilized 4-vinylbenzylammonium (VBA) to create well-ordered perovskite quantum wells (PQWs) (also known as low dimensional perovskites) on top of 3D perovskite layers.33 As shown in Fig. 8a–d, VBA featured an additional terminal vinyl group on the para position of the ammonium group in the benzene ring. The adjacent vinyl groups formed an extended conjugate system undergoing photochemical cross-linking under UV photoexcitation (∼250 nm) to produce new covalent bonds when forming 2D perovskites.75,76 These cross-linked VBA-based 2D/3D perovskite films exhibited improved crystallinity, resulting in enhanced VOC, negligible hysteresis, and increased hydrophobic properties compared to uncross-linked counterparts such as PEA-based and allylammonium (ALA)-based heterojunctions. To gain further insight into the formation mechanism of VBA-based 2D/3D heterojunction films, in situ GIWAX measurements were conducted to gather information regarding the orientation and d-spacing of diffractive species before, during, and after the exposure of 3D perovskites to VBA spacers.35 For the (MAPbBr3)0.05(FAPbI3)0.95 perovskite, upon exposure to VBA spacers, 2D perovskite with n = 3 initially formed and its dimension gradually decreased in subsequent stages, undergoing a progressive dimensional reduction from 3D fragments to n = 3 → 2 → 1 (Fig. 8e and f).
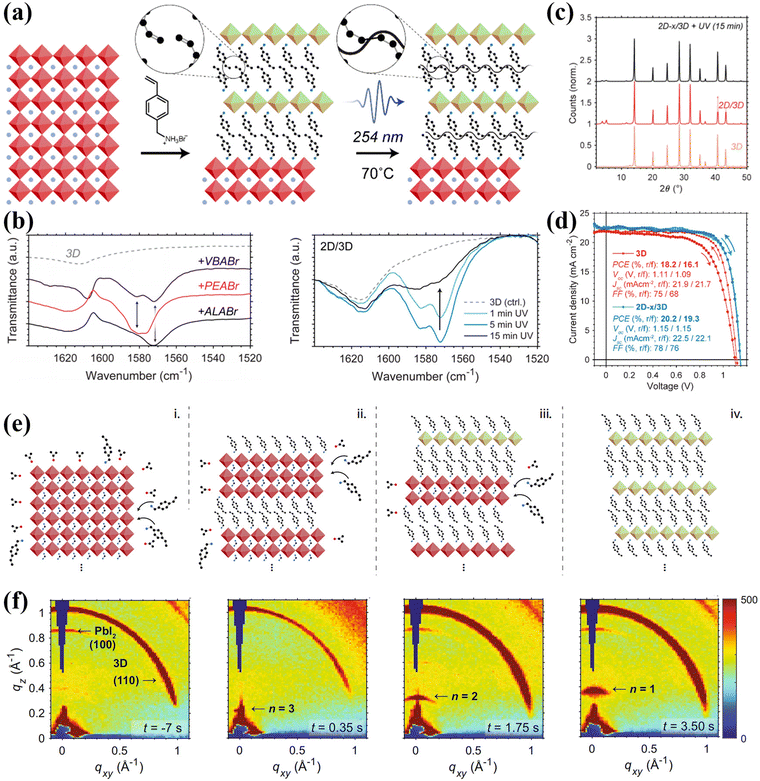 |
| Fig. 8 (a) Schematic illustrating the process by which formation of cross-linked 2D perovskites atop a 3D layer is achieved using VBABr. (b) Fourier transform infrared spectra of films treated with VBABr, PEABr, and ALABr and the 2D/3D VBABr-treated films with different durations of UV light (254 nm) exposure. (c) XRD patterns for the 3D film and the 2D/3D film with/without UV exposure. (d) J–V curves of VBA-based 2D/3D perovskites. Reproduced with permission.33 Copyright 2019, American Chemical Society. (e) Proposed 3D-to-2D conversion mechanism by VBA spacers. (f) In situ GIWAXS patterns for VBA-treated films. Reproduced with permission.35 Copyright 2021, Springer Nature. | |
Thiophenyl aromatic compounds are also important organic spacers for the construction of 2D/3D heterojunctions on the surface of 3D perovskite.36,77 As mentioned above, 2D/3D heterojunctions can regulate the arrangement of energy levels to enhance charge extraction. To investigate this mechanism more thoroughly, Grancini et al. developed an innovative technique called ultraviolet photoemission spectroscopy (UPS) depth profiling. This technique allowed them to probe the energetic landscape within the bulk of materials and across buried interfaces, accurately measuring the progression of the vacuum level and valence band positions as a function of film depth. They further utilized this technique to provide an in situ measurement of the interfacial energy level alignment in a 2D/3D system constructed using ThMAX (X = Cl, Br, or I) (Fig. 9a).78 The valence bands of 2D perovskites composed of ThMAI and ThMABr bent downward, resulting in an energy level alignment that promoted hole extraction. On the other hand, the valence bands of 2D perovskites composed of ThMACl showed an upward slope, creating a certain energy barrier for hole transport. Importantly, the 2D perovskites based on ThMAI and ThMABr exhibited a clear transition from n-type to p-type materials compared to the 3D perovskite. This transition led to the formation of a p–n junction at the interface between the 3D and 2D perovskites, significantly enhancing charge extraction and reducing interface recombination (Fig. 9b and c). Additionally, Grancini and colleagues also investigated the inhibitory effects of thiophenyl-based 2D perovskites on ion migration.79 Pure 2D perovskites based on ThMAI and 3-thiophenemethylammonium iodide (3-TMAI) can embed small MA cations that migrate from the 3D bulk underneath and immobilize them to form a new quasi-2D structure. In this transition process, the MA cations were consumed. On the other hand, 2D perovskites based on 2-thiopheneethylammonium iodide (2-TEAI) physically blocked the MA cations at the 2D/3D interface, inhibiting ion migration and preserving the purity of the 2D phase. Therefore, although different thiophenyl-based 2D perovskites employed different mechanisms to block ion migration, 2D/3D hybrid devices fabricated by using ThMAI, 3-TMAI and 2-TEAI all exhibited improved long-term stability compared to the pristine device.
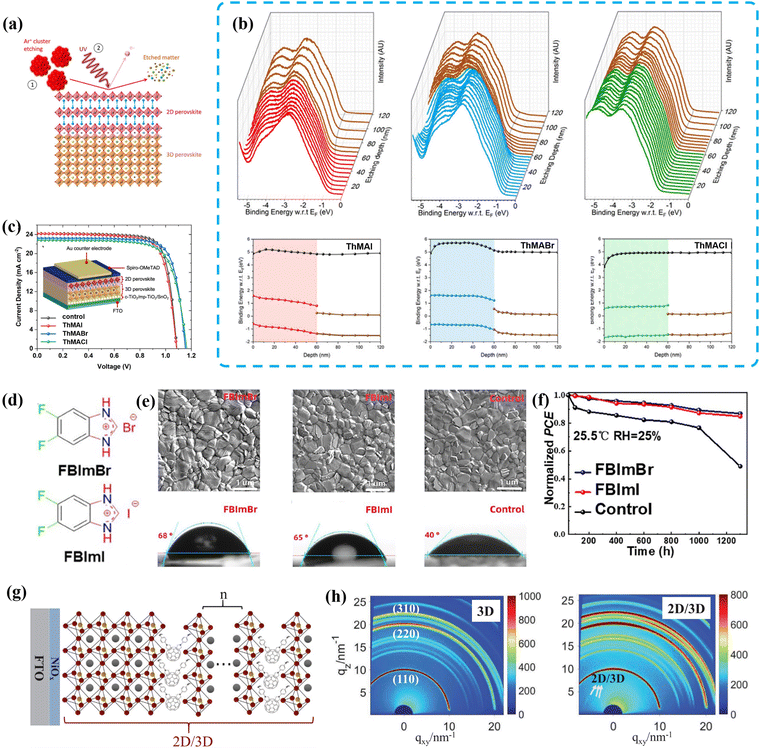 |
| Fig. 9 (a) Schematic representation of the UPS depth profiling technique. (b) Measured UPS spectra and the corresponding energetic level diagrams of ThMAI, ThMABr, and ThMACl with the 3D perovskite layer. (c) J–V curves of the champion cells of 3D and 2D/3D PSCs employing ThMAI, ThMABr, and ThMACl. Reproduced with permission.78 Copyright 2021, Elsevier. (d) Chemical structures of FBImBr and FBImI. (e) SEM images and water contact angle images of FBImBr, FBImI and control perovskite films. (f) Environmental stability for the FBImBr, FBImI and control devices. Reproduced with permission.80 Copyright 2023, Springer Nature. (g) Schematic illustration of the structure of Dion–Jacobson phase 2D/3D (C60-BPAM). (h) 2D-GIXRD profiles of 3D and 2D/3D perovskite films. Reproduced with permission.81 Copyright 2022, John Wiley & Sons, Inc. | |
Imidazole (IZ) is a unique diammonium molecule with an aromatic structure that can form additional hydrogen bonding with a [PbI6]4− framework. Due to its delocalized π bonding, it may exhibit low reactivity with its surroundings, leading to additional electrical conductivity.82,83 However, IZ has an unfavorable ionic radius of 258 pm, which is close to the upper limit of the tolerance factor. Therefore, it is not considered a suitable spacer for 2D perovskite fabrication.82 In 2022, Tu et al. successfully demonstrated the formation of 2D/3D heterojunctions through post-treatment with imidazole hydrobromide (IZBr) and achieved an outstanding PCE of 23.25% for MA-free (FA0.9Cs0.1PbI3) PSCs.84 Other imidazolium-based cations such as benzimidazolium (BIZ) and benzodiimidazolium (Bdi) spacers have larger ionic radii and stronger π conjugation effects, making them more suitable for 2D/3D heterojunction fabrication.85 In 2021, Feng et al. reported the facile in situ formation of a layered 2D (BIZ)2PbI3Br perovskite by incorporating benzimidazolium iodine (BIZI) on a 3D CsPbI2Br absorber. The resulting 2D (BIZ)2PbI3Br exhibited remarkable defect passivation and moisture resistance for the 3D perovskite, leading to improved photovoltaic performance in an inverted (p–i–n) PSC.86 More recently, based on benzimidazole, Chen et al. designed highly conjugated fluorinated benzimidazolium halides (FBImX, X = I or Br) as passivators for 2D/3D perovskite. By introducing double fluoride atoms in the meta-position of the imidazole group, fluoride effectively enhanced the hydrophobicity of the film and passivated both anion and cation vacancies present in 3D perovskite.80 As a result, the best PCE of 23.00% was achieved for FBImBr-incorporated PSCs, along with a high VOC of 1.17 V and an FF of 81.80%. The FBImBr-modified device exhibited excellent operational stability, maintaining 86% of the initial PCE after 1300 h under 25% relative humidity (Fig. 9d–f).
Generally, fullerene (C60)87 and its derivatives, such as phenyl-C61-butyric acid methyl ester (PCBM),88 C60-substituted benzoic acid self-assembled monolayer (C60-SAM)89 and [6,6]-phenyl-C61-butyric acid-dioctyl-3,3’-(5-hydroxy-1,3-phenylene)-bis(2-cyanoacrylate) ester (PCBB-2CN-2C8),90 are used as electron transport materials due to their high electron affinity and strong electron acceptance ability. However, the larger molecular size of C60 (with a van der Waals diameter of ∼7 Å) compared to the [PbI6]4− octahedron (with a size of 6.3 Å) makes it difficult for C60 to fit into the [PbI6]4− octahedron layer to form a 2D structure.81 To overcome this barrier, Yang et al. synthesized a novel amino-functionalized fullerene derivative called C60-BPAM, in which two amino groups were grafted onto C60 using a pyrrolidine–phenyl linker. They then fabricated a DJ-type (C60-BPAM)PbI4 2D perovskite on top of a 3D MAPbI3 perovskite by drop-casting an anti-solvent to form a 2D/3D heterojunction.81 As a result, C60-BPAM significantly facilitated electron transport and improved the humidity stability of the 2D perovskite structure due to the highly conductive and hydrophobic C60 tail. This strategy presents a promising new approach for the preparation of 2D/3D heterojunctions (Fig. 9g and h).
3.2.2. Aliphatic spacers.
Despite aliphatic spacers are considered to be weaker than aromatic spacers in terms of charge transport, their longer carbon tails endow them with higher structural degrees of freedom and hydrophobic properties, thereby enabling a wide range of applications.91 In 2018, Huang et al. utilized short-chain aliphatic BA and the corresponding iodide (BAI) as passivating agents to investigate 2D/3D stacking structures.92 They found that BA promoted the formation of a pure 2D BA2PbI4 perovskite by reacting with MAPbI3. The pure BA2PbI4 exhibited more organic spacers than the mixture of 2D perovskites, indicating superior protective effects. On the other hand, the reaction between BAI and MAPbI3 resulted in inferior (BA)2(MA)n−1PbnI3n+1 with various n values, leading to a rougher 2D perovskite layer compared to the BA-constructed 2D perovskite (Fig. 10a and b). In 2021, Noh et al. developed another method called the solid-state in-plane growth (SIG) process, which involved integrating a pre-formed 2D BA2PbI4 perovskite capping layer onto 3D perovskite surfaces through mechanical and thermal pressure.34 The SIG process allowed for controlled deposition of a pure and highly crystalline 2D (BA)2PbI4 perovskite layer on top of a 3D perovskite layer without the presence of any quasi-2D phases. As a result, the intact 2D/3D heterojunction enhanced the carrier lifetime and facilitated the design of the local electric field distribution at the junction, leading to a significant improvement in the VOC of PSCs (Fig. 10c–e). Recently, Mohite et al. explored the use of acetonitrile (MeCN) as a solvent to dissolve 2D perovskites (BA2MAn–1PbnI3n+1, n = 1 to 4) without destroying the underlying 3D perovskite.93 MeCN was found to possess an appropriate dielectric constant and Gutmann donor number. With an increase in the n value of the 2D perovskite, a transition from type I heterojunction to type II heterojunction was observed due to changes in energy level alignment. Among them, the type II heterojunction achieved with BA2MA2Pb3I10 (n = 3) and the 3D perovskite exhibited near-perfect alignment of the valence band edges for 3D and 2D perovskites. This alignment was considered ideal for hole extraction and created a significant energy barrier for electron transport. Consequently, the fabricated 2D/3D PSC incorporating a pure n = 3 2D component achieved a champion PCE of 24.5% with an impressive VOC of 1.20 V.
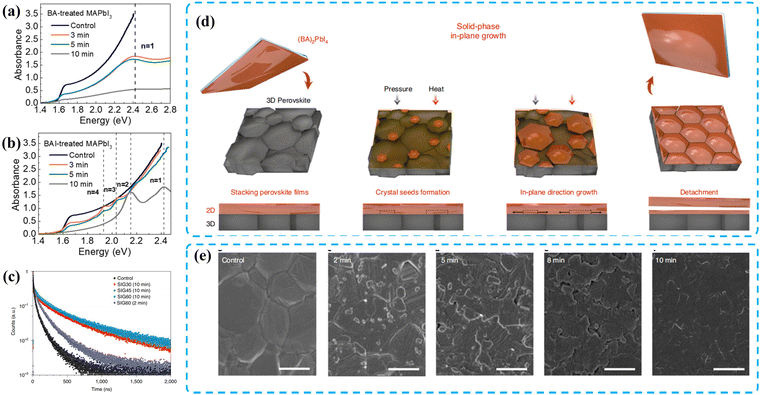 |
| Fig. 10 Absorption spectra of (a) BA-treated MAPbI3 films and (b) BAI-treated MAPbI3 films with different reaction times. Reproduced with permission.92 Copyright 2018, American Chemical Society. (c) Time-resolved photoluminescence decay curves of the control and SIG-processed 2D/3D films. (d) Top-view and cross-sectional sketches of the manufacturing of a (BA)2PbI4 film on a 3D perovskite substrate via the SIG method. (e) The 2D/3D perovskite film fabricated under SIG60 conditions after 2 min, 5 min, 8 min and 10 min processing times. Reproduced with permission.34 Copyright 2021, Springer Nature. | |
The long-chain in aliphatic organic spacers can provide the possibility of forming more reaction sites, which can strengthen the interaction between the spacers and inorganic [PbI6]4− framework, thereby improving the film's photophysical properties and device performance.91,94 In 2021, Lan et al. utilized a viscous polymer named polypropylene glycol bis (2-aminopropyl ether) (A-PPG) with a number-average molecular weight (Mn) of 400 to create a quasi-2D layer on the surface of 3D perovskite in situ. A-PPG had the ability to partially dissolve excess PbI2 and perovskite, and it was applied in a one step spin-coating process with the addition of chlorobenzene as the anti-solvent in advance.95 Moreover, unlike other spacer cations, the lone electron pairs of the ether-oxygen in A-PPG can also form coordination bonds with undercoordinated Pb2+, significantly contributing to defect passivation and reducing non-radiative recombination. Consequently, the A-PPG-modified 2D/3D PSC exhibited an optimized PCE of 22.24% with a distinguished VOC of 1.21 V. The device also maintained 90% of its original PCE in an ambient atmosphere after 50 days, demonstrating extraordinary humidity stability. More reported organic spacers applied in surface-treatment type PSCs are summarized in Table 2.
Table 2 An exhaustive list of recently reported organic spacer cations applied in surface-treatment type PSCs
Spacers |
Device architecture |
VOC (V) |
FF (%) |
JSC (mA cm−2) |
PCE (%) |
Ref. |
BnA |
FTO/c-TiO2/m-TiO2/SnO2/3D perovskite/BnA-based 2D perovskite/spiro-OMeTAD/Au |
1.078 |
78.80 |
24.48 |
20.79 |
66
|
PEA |
FTO/TiO2/Cs0.05(MA0.12FA0.88)0.95Pb(I0.88Br0.12)3/PEA2PbI4/PEAI-spiro-OMeTAD/Au |
1.170 |
79.00 |
22.93 |
21.09 |
63
|
PBA |
ITO/NiOX/(Cs0.07FA0.95PbI2.95)0.85(MAPbBr3)0.15/PBA-based 2D perovskite/PCBM:C60/Zr(acac)4/Au |
1.170 |
83.92 |
22.16 |
21.76 |
67
|
FPEA |
FTO/c-TiO2/m-TiO2/Cs0.1(FA0.83MA0.17)0.9Pb(I0.83Br0.17)3/FPEA2PbI4/spiro-OMeTAD/Au |
1.126 |
80.00 |
22.80 |
20.54 |
69
|
FEA |
FTO/c-TiO2/m-TiO2/FA0.92MA0.04Cs0.04PbI3/FEA-based 2D perovskite/spiro-OMeTAD/Au |
1.096 |
78.40 |
25.79 |
22.16 |
32
|
3Cl-BA |
FTO/c-TiO2/m-TiO2/MAPbI3/(3Cl-BA)2PbI4/carbon |
0.980 |
63.00 |
21.31 |
13.22 |
73
|
CF3PMA |
ITO/PTAA/MAPbI3/CF3PMA-based 2D perovskite/PCBM/BCP/Ag |
1.070 |
72.22 |
19.38 |
15.01 |
71
|
CF3PEA |
FTO/TiO2/FA0.85MA0.15PbI3/(CF3PEA)2FAPb2I7/spiro-OMeTAD/Au |
1.130 |
80.78 |
25.29 |
23.10 |
72
|
PTA |
FTO/c-TiO2/SnO2/MAPbI3/PTA-based 2D perovskite/spiro-MeOTAD/Ag |
1.104 |
81.90 |
23.39 |
21.26 |
96
|
OHPEA |
ITO/SnO2/Rb0.05Cs0.05[(FA0.83MA0.17)]0.9Pb(I0.83Br0.17)3/OHPEA-based 2D perovskite/spiro-MeOTAD/Ag |
1.220 |
75.50 |
23.25 |
21.38 |
97
|
ABA |
FTO/NiOX/MAPbI3/PCBM/Rhodamine 101/Ag |
1.105 |
81.90 |
23.42 |
21.18 |
70
|
VBA |
ITO/TiO2/(MAPbBr3)0.15(FAPbI3)0.85/VBA-based 2D perovskite/spiro-OMeTAD/Au |
1.150 |
78.00 |
22.50 |
20.20 |
33
|
TFPhFA |
FTO/SnO2/FA0.93MA0.07PbI3/spiro-MeOTAD/Au |
1.160 |
81.26 |
25.42 |
24.00 |
98
|
PDA |
FTO/TiO2/(Cs0.05(FA0.83MA0.17)0.95Pb(I0.83Br0.17)3/PDA-based 2D perovskite/spiro-MeOTAD/Ag |
1.145 |
77.89 |
23.78 |
21.15 |
74
|
XDA |
FTO/c-TiO2/SnO2/MAPbI3/XDA-based 2D perovskite/spiro-MeOTAD/Ag |
1.134 |
76.92 |
23.78 |
20.74 |
99
|
IZ |
ITO/SnO2/FA0.9Cs0.1PbI3/IZ-based 2D perovskite/spiro-MeOTAD/Ag |
1.172 |
80.76 |
24.57 |
23.25 |
84
|
BIZ |
ITO/NiOX/CsPbI2Br/(BIZ)2PbI3Br/PCBM/Ag |
1.151 |
79.79 |
15.59 |
14.32 |
86
|
FBIm |
ITO/SnO2/FAPbI3/(FBIm)2PbBr4/spiro-MeOTAD/MoO3/Ag |
1.170 |
81.80 |
24.03 |
23.00 |
80
|
ThMA |
FTO/c-TiO2/m-TiO2/SnO2/[(FAPbI3)0.87(MAPbBr3)0.13]0.92(CsPbI3)0.08/ThMA-based 2D perovskite/spiro-OMeTAD/Au |
1.150 |
78.00 |
23.20 |
20.80 |
78
|
3-TMA |
FTO/c-TiO2/m-TiO2/SnO2/MAPbI3/quasi-2D 3-TMA perovskite/spiro-MeOTAD/Au |
1.132 |
77.10 |
23.60 |
20.59 |
79
|
2-TEA |
FTO/c-TiO2/m-TiO2/SnO2/MAPbI3/(2-TEA)2PbI4/spiro-MeOTAD/Au |
1.117 |
73.70 |
23.60 |
19.42 |
79
|
OA |
FTO/SnO2/(FAPbI3)0.95(MAPbBr3)0.05/OA-based 2D perovskite/PTAA/Au |
1.180 |
80.80 |
24.70 |
23.60 |
100
|
1,8-ND |
ITO/SnO2/FA0.92MA0.08PbI3/spiro-OMeTAD/Au |
1.150 |
83.00 |
24.90 |
23.80 |
101
|
C60-BPAM |
FTO/NiOX/MAPbI3/(C60-BPAM)PbI4/PCBM/BCP/Ag |
1.090 |
78.53 |
23.61 |
20.21 |
81
|
CA |
ITO/PEDOT:PSS/MAPbI3/PCBM/Rhodamine 101/LiF/Ag |
0.920 |
77.26 |
19.29 |
13.86 |
60
|
BA |
FTO/SnO2/(FAPbI3)0.95(MAPbBr3)0.05/BA2PbI4/spiro-OMeTAD/Au |
1.185 |
83.90 |
24.70 |
24.59 |
34
|
HA |
FTO/SnO2/(FAPbI3)0.80(CsPbI3)0.07(MAPbBr3)0.13/HA-based 2D perovskite/spiro-OMeTAD/Ag |
1.140 |
76.00 |
23.76 |
20.62 |
102
|
HTA |
ITO/SnO2/(FAPbI3)0.95(MAPbBr3)0.05/HTA-based 2D perovskite/spiro-OMeTAD/Ag |
1.170 |
79.55 |
22.69 |
21.12 |
103
|
4-ABA |
FTO/SnO2/Cs0.05(FA0.83MA0.17)0.95Pb(I0.83Br0.17)3/4-ABA-based 2D perovskite/spiro-OMeTAD/Au |
1.200 |
78.55 |
24.57 |
23.16 |
104
|
AVA |
FTO/c-TiO2/m-TiO2/MAPbI3/AVA-based 2D perovskite/spiro-MeOTAD/Au |
1.060 |
76.00 |
22.30 |
18.00 |
105
|
A43 |
FTO/c-TiO2/m-TiO2/MA0.9FA0.1PbI3/(A43)2PbI4/spiro-MeOTAD/Au |
1.110 |
79.30 |
22.87 |
20.12 |
106
|
A-PPG |
FTO/TiO2/(FAPbI3)0.84(CsPbI3)0.06(MAPbBr3)0.10/A-PPG-based 2D perovskite/spiro-MeOTAD/Au |
1.210 |
78.97 |
23.27 |
22.24 |
95
|
DMEDA |
ITO/SnO2/MAPbI3/DMEDA-based 2D perovskite/spiro-OMeTAD/Ag |
1.140 |
78.00 |
22.57 |
20.18 |
107
|
DETA |
ITO/NiOX/Cs0.05(FA0.85MA0.15)0.95Pb(I0.85Br0.15)3/DETA-based 2D perovskite/PCBM/BCP/Ag |
1.100 |
83.33 |
21.73 |
19.94 |
94
|
TETA |
ITO/NiOX/Cs0.05(FA0.85MA0.15)0.95Pb(I0.85Br0.15)3/TETA-based 2D perovskite/PCBM/BCP/Ag |
1.110 |
83.90 |
21.61 |
20.13 |
94
|
TEPA |
ITO/NiOX/Cs0.05(FA0.85MA0.15)0.95Pb(I0.85Br0.15)3/TEPA-based 2D perovskite/PCBM/BCP/Ag |
1.120 |
84.81 |
22.39 |
21.28 |
94
|
PEHA |
ITO/NiOX/Cs0.05(FA0.85MA0.15)0.95Pb(I0.85Br0.15)3/PEHA-based 2D perovskite/PCBM/BCP/Ag |
1.130 |
84.90 |
22.63 |
21.79 |
94
|
EDA |
ITO/NiOX/PTAA/Cs0.05(FA0.95MA0.05)0.95Pb(I0.95Br0.05)3/EDA-based 2D perovskite/PCBM/BCP/Ag |
1.200 |
81.90 |
25.20 |
24.70 |
108
|
ODA |
FTO/c-TiO2/m-TiO2/(FA0.85MA0.15)Pb(I0.85Br0.15)3/(ODA)PbI4/spiro-MeOTAD/Au |
1.100 |
81.00 |
24.20 |
21.60 |
109
|
EDBE |
ITO/SnO2/Cs0.2FA0.8Pb(I0.7Br0.3)3/EDBE-based 2D perovskite/spiro-OMeTAD/Ag |
1.280 |
78.50 |
18.85 |
18.85 |
110
|
3.3. Bulk incorporation and surface treatment coexisting model
In view of the advantages of 2D/3D perovskite heterojunctions in modifying grain boundaries and passivating surface defects, researchers have started exploring the combination of these two methods to create a coexisting type 2D/3D heterojunctions. Specifically, the process involves adding spacers to the perovskite precursor, resulting in a 2D/3D heterojunction within the bulk of the deposited 3D perovskite film. Subsequently, a solution containing spacers (or 2D perovskites) is dropped onto the film surface after annealing, creating a capping 2D perovskite layer after post-treatment. Furthermore, there exist many other approaches for constructing the same structure, which we will discuss in the following discussion and mainly focus on aromatic and aliphatic organic spacers.
3.3.1. Aromatic spacers.
Lan et al. designed a pyridinyl organic ammonium salt named 2-(2-pyridyl)ethylamine iodide (2-PyEAI) and applied the above method to prepare a 2D/3D hybrid PSC with a high efficiency of 23.2%.111 Meanwhile, Liu et al. made groundbreaking advancements by developing 2-thiopheneformamidinium bromide (ThFABr) to passivate the inevitable defects present in both the bulk and surface of perovskite films, achieving an ultralong carrier lifetime exceeding 20 μs and carrier diffusion lengths longer than 6.5 μm (Fig. 11a and b).112 Typically, the carrier lifetime and diffusion length are closely related to the density of defects. As shown in Fig. 11c, the PL intensity enhanced more pronouncedly in the coexisting type 2D/3D PSC as the excitation fluence increased, indicating that the trap states were filled more rapidly during the excitation process, confirming the effective passivation of defects. Importantly, the passivation of defects was facilitated by the incorporation of ThFABr, resulting in the formation of 2D perovskites in the bulk and on the surface of 3D perovskites (Fig. 11d–f). Moreover, the thickness of perovskite films was generally less than 1 μm due to the limited carrier diffusion length. The ultra-long carrier lifetime and diffusion lengths made it possible to fabricate film thickness-insensitive devices for their future commercialization. Consequently, the coexisting type 2D/3D PSCs achieved a champion PCE of 24.69% (film thickness < 1 μm) and an excellent PCE of 24.15% (film thickness > 1.1 μm (Fig. 11g and h).
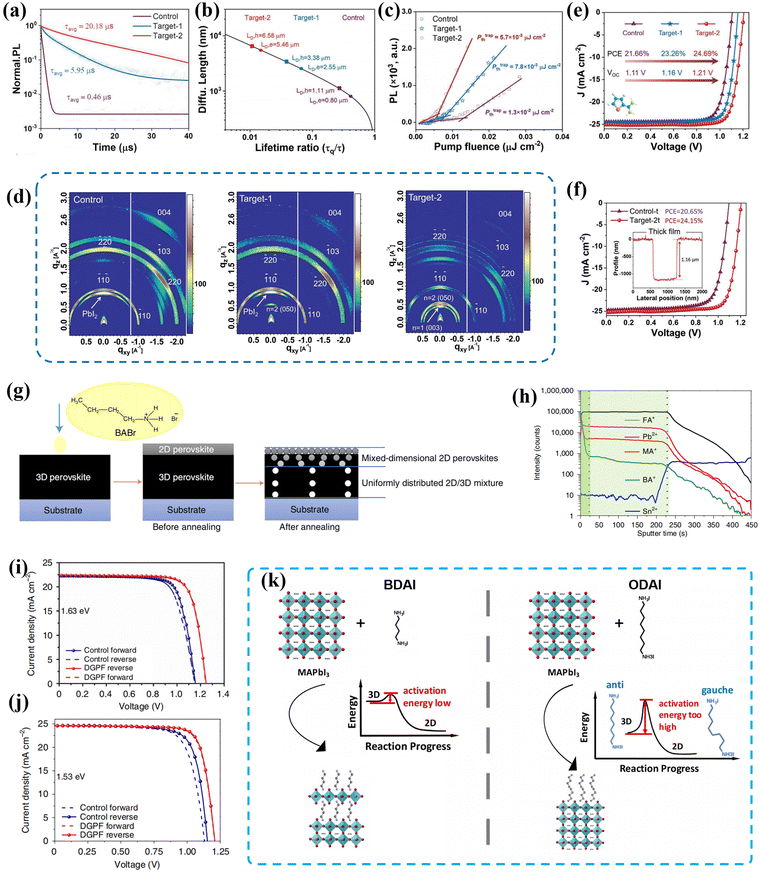 |
| Fig. 11 (a) TRPL decay spectra, (b) the diffusion length of holes and electrons, (c) pump fluence dependent PL intensity and (d) GIWAX measurements for the control, bulk incorporation type (target-1) and coexisting type (target-2) 2D/3D films using ThFABr. J–V curves of PSCs based on (e) control, target-1 and target-2 perovskites and (f) a thick perovskite film. Reproduced with permission.112 Copyright 2023, John Wiley & Sons, Inc. (g) Schematic diagram of the DGPF film. (h) ToF-SIMS depth profile of the DGPF perovskite film deposited on an ITO substrate. J–V curves based on the 1.63 eV(i) and 1.53 eV(j) perovskite. Reproduced with permission.113 Copyright 2021, Springer Nature. (k) Schematic demonstration of why BDA treatment can induce respective 2D hybrid perovskite formation, while ODA stays only at the surface or grain boundaries without affecting bulk properties. Reproduced with permission.114 Copyright 2016, American Chemical Society. | |
On the other hand, in 2021, He et al. reported a heterogeneous 2D/3D tin-halide PSC (FASnI3) through the substitution of FAI with FPEABr. They demonstrated that 2D components can be transferred from the bulk phase to the surface of the 3D perovskite during the grain growth process.115 It was observed that, on one hand, there was an extensive oxidation process from Sn2+ to Sn4+ during the aging of FASnI3. The authors proved that the introduction of FPEABr, which formed a 2D phase, prevents the oxidation of Sn2+ to Sn4+. On the other hand, the 2D tin-perovskite layer based on FPEA+ blocked the water penetration due to the presence of bonded F atoms, resulting in significantly improved humidity stability. Consequently, the highly oriented and stable 2D/3D PSC achieved an outstanding PCE of 14.81%, with a certified PCE of 14.03%, setting a new record for FASnI3-based PSC at that time.
3.3.2. Aliphatic spacers.
For aliphatic spacer cations, in 2021, Li et al. designed a dimensionally graded perovskite formation (DGPF) approach to achieve a homogeneously distributed self-passivated 2D/3D perovskite structure in the bulk. This structure was further capped by a stepped mixed-dimensional 2D perovskite with a wider-band gap through thermal annealing of the 3D perovskite layer covered with a spin-coated n-butylammonium bromide (BABr) solution.113 The introduction of the BA moiety immensely passivated antisite defects like PbBr and PbI, which were known as deep-level defects that can cause carrier recombination. Using the DGPF technique, the 2D/3D hybrid PSC demonstrated a maximum hysteresis-free PCE of 21.54% with a band gap-voltage offset (WOC) of 0.39 V in a 1.63 eV perovskite system (RbCsMAFA-based) and a champion PCE of 23.78% with a low WOC of 0.326 V in an approximately 1.53 eV perovskite system (Cs0.03(FA0.97MA0.03)0.97Pb(I0.97Br0.03)3) (Fig. 11g–j). However, not all organic spacers can be applied to generate 2D perovskites within the 3D perovskite bulk through post-treatment. Jen et al. found that the introduction of BDA with a short carbon chain (the same number of carbon atoms as BA) could promote the transformation from 3D perovskite to 2D perovskite during post-treatment, whereas the octane-1,8-diaminium (ODA) (with twice the number of carbon atoms compared to BA and BDA) could only insert into the grain boundaries within the 3D perovskite bulk without any dimension transformation.114 The fast dimension transformation caused by BDA could be attributed to the high thermodynamic stability of 2D perovskites and the low activation energy of the transformation process. In contrast, the failure of ODA to facilitate dimensional transformation could be ascribed to two factors: (1) thermodynamically, there was a huge activation energy involved in the transformation process from the MAPbI3 3D perovskite to ODAPbI4 2D perovskite, although the anti-gauche isomerization of ODA may increase the Gibbs free energy of the ODAPbI4 2D perovskite (still lower than that of MAPbI3); (2) kinetically, the transition from 3D to 2D perovskite occurred too slowly to be observed within a 10-second post-treatment time. This study emphasized the importance of reasonable molecular design for the surface modification and bulk passivation of perovskites (Fig. 11k).
3.3.3. Alicyclic spacers.
In contrast to aromatic compounds, which also have a ring structure, alicyclic spacers, such as cyclohexylammonium, exhibit stronger N–H bonding due to the localized electron density in the cycle structure. The stronger bonding is more favorable for defect passivation compared to aromatic spacers such as phenylammonium.116,117 As a result, in addition to long-chain aliphatic compounds, alicyclic spacer cations have been found to be suitable for producing 2D/3D heterojunctions.118,119 For example, in 2022, Wu's group applied an alicyclic organic salt called (R)-(−)-1-cyclohexylethylammonium iodide (R-CEAI) to fabricate multiple 2D/3D perovskite homojunctions using the DGPF method.120 Grazing incidence X-ray diffraction (GIXRD) characterization revealed residual stress in the R-CEAI-based perovskite films. The alleviated lattice distortion resulting from this stress was beneficial for carrier dynamics and device performance. Consequently, an R-CEAI-based PSC showed an improved PCE of 22.52% in comparison with the pristine PSC with a lower PCE of 19.43%.
In particular, the formation of coexisting 2D/3D heterojunctions opens up the possibility of combining different types of organic spacers. For instance, in 2022, Mai et al. selected oleylammonium (OAm) and PEA as perovskite bulk and surface passivation agents, respectively. These agents not only promoted the crystal growth in the out-of-plane orientation but also passivated defects at grain boundaries and the perovskite surface, leading to a reduction in trap density and nonradiative recombination.121 Consequently, the modified 2D/3D hybrid device with the architecture of FTO/NiOx/PTAA/Al2O3/perovskite/PCBM/BCP/Ag achieved a PCE of 20.52% without any hysteresis. More reported organic spacer cations applied in bulk incorporation and surface treatment coexisting type PSCs can be found in Table 3.
Table 3 An exhaustive list of recently reported organic spacer cations applied in bulk incorporation and surface treatment coexisting type PSCs
Spcers |
Device architecture |
V
OC (V) |
FF (%) |
J
SC (mA cm−2) |
PCE (%) |
Ref. |
FPEA |
ITO/PEDOT:PSS/FASnI3/ICBA/BCP/Al |
0.840 |
70.76 |
24.91 |
14.81 |
115
|
2-PyEA |
ITO/SnO2/FA0.92MA0.08PbI3/spiro-OMeTAD/Au |
1.140 |
82.10 |
24.80 |
23.20 |
111
|
DMA |
FTO/TiO2/Cs0.05FA0.85MA0.10Pb(I0.97Br0.03)3/spiro-OMeTAD/MoO3/Ag |
1.140 |
78.00 |
25.43 |
22.61 |
122
|
ThFA |
ITO/SnO2/FA0.84MA0.16PbI3/spiro-OMeTAD/MoO3/Ag |
1.210 |
81.62 |
25.07 |
24.69 |
112
|
BA |
ITO/SnO2/Cs0.03(FA0.97MA0.03)0.97Pb(I0.97Br0.03)3/spiro-OMeTAD/Au |
1.210 |
80.00 |
24.57 |
23.78 |
113
|
OA |
FTO/c-TiO2/PMMA:PCBM/Cs0.07Rb0.03FA0.765MA0.135PbI2.55Br0.45/spiro-OMeTAD/Au |
1.240 |
78.46 |
24.24 |
23.62 |
123
|
TBA |
FTO/PEDOT:PSS/FAPbI3/spiro-OMeTAD/Au |
0.990 |
71.20 |
24.23 |
17.02 |
124
|
CME |
FTO/c-TiO2/m-TiO2/Cs0.05FA0.80MA0.15Pb(I0.85Br0.15)3/spiro-OMeTAD/Au |
1.110 |
77.00 |
23.45 |
20.33 |
125
|
Gu |
ITO/SnO2/(FA0.93MA0.07Pb(I0.93Br0.07)3/spiro-OMeTAD/Au |
1.120 |
81.15 |
24.79 |
22.53 |
126
|
TBH |
(FAPbI3)0.84(CsPbI3)0.06(MAPbBr3)0.10 |
1.186 |
84.10 |
22.60 |
22.54 |
127
|
R-CEA |
FTO/TiO2/(FAPbI3)0.84(CsPbI3)0.05(MAPbBr3)0.11/spiro-OMeTAD/Au |
1.195 |
80.50 |
23.42 |
22.52 |
120
|
BDA |
ITO/Cu:NiOX/MAPbI3/PCBM/C60/Ag |
1.110 |
76.00 |
14.48 |
11.97 |
114
|
EDBE |
ITO/Cu:NiOX/MAPbI3/PCBM/C60/Ag |
1.160 |
71.00 |
4.33 |
3.44 |
114
|
3.4. Other models
In addition to the three mainstream 2D/3D heterojunction structures mentioned above, 2D perovskites can also be constructed at the bottom of 3D perovskites through self-assembly and/or in situ formation to passivate the defects of the buried interface in 3D PSCs.128 Yu et al. achieved this by fabricating 2D perovskite in situ at the buried interface through the interaction between the PEA spacers and 3D perovskite. They first deposited PEABr on an ITO substrate and then spin-coated the perovskite precursor. Moreover, Chen et al. demonstrated that the introduction of chiral cations, namely α-methylbenzylamine (S-/R-/rac-MBA), can induce the formation of 2D perovskites at the interface between PEDOT:PSS and FASnI3, resulting in better matched energy levels and improved interface contacts and leading to improved hole extraction from FASnI3 by PEDOT:PSS.129 To achieve this, MBA was added in advance to prepare a quasi-2D perovskite precursor with n = 5. The precursor primarily formed a 3D structure from the upper surface rather than quasi-2D perovskite when the anti-solvent was dropped, due to the lower formation energy of 3D perovskite. Subsequently, the MBA molecules acted as templates to guide the downward growth of 3D perovskite. Finally, at the end of solvent evaporation, the excess MBA spacers interacted with [PbI6]4− octahedron layers at the bottom of the 3D perovskite, thus forming a 2D perovskite at the buried bottom interface. Additionally, considering the significance of buried interface passivation, White et al. proposed a design of 2D/3D/2D structure for double-side surface modification using the BA spacer, achieving in a remarkable PCE of 22.77%.130 These studies have some implications for constructing 2D perovskites at the buried interface to reduce buried interface defects and enhance charge extraction.
4. Summary and outlook
In the past several years, significant advancements have been made in constructing 2D/3D heterojunctions by incorporating bulky and hydrophobic organic spacers into or onto 3D perovskite films. These advancements aim to enhance the photovoltaic performance and long-term stability of PSCs. However, due to the lack of a deep understanding in this field, we have presented the structural characteristics of both 3D and 2D perovskite materials, discussed the transformation process from 3D to 2D perovskite, summarized the key organic spacers used in fabricating 2D/3D PSCs, highlighted their roles in the formation mechanisms of different types of 2D/3D heterojunctions, and drawn the following conclusions:
2D/3D heterojunctions can be fabricated through bulk incorporation and surface treatment methods. In the bulk incorporation process, the formed 2D/3D heterojunctions play several roles: (1) passivating defects in bulk of 3D perovskite; (2) forming a distinctive structure, such as a porous structure for the PbI2 film; (3) providing seeds and serving as a template to assist 3D perovskite growth; and (4) regulating the crystallization rate and controlling the crystal orientation; and (5) forming type I heterojunctions and reducing carrier recombination at grain boundaries. On the other hand, in the surface treatment process, 2D components containing different organic spacers are deposited on top of 3D perovskite through post-treatment. The resulting 2D/3D heterojunctions formed by this method exhibit the following effects: (1) passivating defects on the perovskite film surface; (2) forming a hydrophobic capping layer to improve humidity stability; (3) inhibiting ion migration; and (4) generating type II heterojunctions to promote carrier transport. Furthermore, some researchers have combined these two methods to prepare coexisting 2D/3D heterojunctions, which offer advantages of both types of 2D/3D heterojunctions.
Looking ahead, the construction of 2D/3D heterostructures remains a crucial method to improve the comprehensive performance of PSCs, including high efficiency and long-term stability. While the stability of 2D/3D hybrid PSCs is higher than that of 3D PSCs, the maximum PCE of 2D/3D hybrid PSCs (PCE = 24.9%)30 is still behind that of the 3D PSCs (PCE = 26.1%).2 To bridge this efficiency gap, researchers need to dedicate more attention and efforts. Based on the contents of this review, we propose several possible strategies for enhancing 2D/3D hybrid PSCs. (1) Prioritizing aromatic spacers over aliphatic spacers: aromatic spacers possess π-conjugated structures with a higher dielectric constant, which benefits charge transfer. (2) Focusing on modifying 2D seed crystals rather than simple salt treatments: recent studies have shown that 2D seed crystals provide more advantages compared to salt treatments alone. (3) Developing a coexisting 2D/3D heterojunction model instead of a single 2D/3D heterojunction model: as described in this review, the coexisting 2D/3D heterojunction model can take advantages of the two other single models. (4) Constructing 2D/3D heterojunctions in perovskite solar modules or tandem solar cells: in order to realize commercialization, the preparation of the perovskite solar modules and tandem solar cells is an inevitable trend for the future. Therefore, further studies are necessary to develop preparation technologies for constructing 2D/3D heterojunctions in perovskite solar modules and tandem solar cells.
In conclusion, we believe that with the joint efforts of researchers, significant progress can be made in improving the efficiency and stability of 2D/3D PSCs, ultimately leading to their commercialization.
Conflicts of interest
There are no conflicts to declare.
Acknowledgements
This work was financially supported by the National Natural Science Foundation of China (Grant No. 52273182) and Natural Science Foundation of Hebei Province of China (Grant No. B2022408005).
References
- A. Kojima, K. Teshima, Y. Shirai and T. Miyasaka, Organometal Halide Perovskites as Visible-Light Sensitizers for Photovoltaic Cells, J. Am. Chem. Soc., 2009, 131, 6050–6051 CrossRef CAS.
- NREL, Best Research-Cell Efficiencies, https://www.nrel.gov/pv/cell-efficiency.html, access on 30 August 2023.
- C. Wehrenfennig, G. E. Eperon, M. B. Johnston, H. J. Snaith and L. M. Herz, High Charge Carrier Mobilities and Lifetimes in Organolead Trihalide Perovskites, Adv. Mater., 2014, 26, 1584–1589 CrossRef CAS.
- L. N. Li, X. Y. Shang, S. S. Wang, N. N. Dong, C. M. Ji, X. Y. Chen, S. E. Zhao, J. Wang, Z. H. Sun, M. C. Hong and J. H. Luo, Bilayered Hybrid Perovskite Ferroelectric with Giant Two-Photon Absorption, J. Am. Chem. Soc., 2018, 140, 6806–6809 CrossRef CAS.
- D. Stranks Samuel, E. Eperon Giles, G. Grancini, C. Menelaou, J. P. Alcocer Marcelo, T. Leijtens, M. Herz Laura, A. Petrozza and J. Snaith Henry, Electron-Hole Diffusion Lengths Exceeding 1 Micrometer in an Organometal Trihalide Perovskite Absorber, Science, 2013, 342, 341–344 CrossRef CAS PubMed.
- G. Xing, N. Mathews, S. Sun, S. S. Lim, Y. M. Lam, M. Grätzel, S. Mhaisalkar and T. C. Sum, Long-Range Balanced Electron- and Hole-Transport Lengths in Organic-Inorganic CH3NH3PbI3, Science, 2013, 342, 344–347 CrossRef CAS PubMed.
- M. Baranowski and P. Plochocka, Excitons in Metal-Halide Perovskites, Adv. Energy Mater., 2020, 10, 1903659 CrossRef CAS.
- R. Chen, Y. Hui, B. Wu, Y. Wang, X. Huang, Z. Xu, P. Ruan, W. Zhang, F. Cheng, W. Zhang, J. Yin, J. Li and N. Zheng, Moisture-tolerant and high-quality α-CsPbI3 films for efficient and stable perovskite solar modules, J. Mater. Chem. A, 2020, 8, 9597–9606 RSC.
- Q. Sun, P. Fassl, D. Becker-Koch, A. Bausch, B. Rivkin, S. Bai, P. E. Hopkinson, H. J. Snaith and Y. Vaynzof, Role of Microstructure in Oxygen Induced Photodegradation of Methylammonium Lead Triiodide Perovskite Films, Adv. Energy Mater., 2017, 7, 1700977 CrossRef.
- D. B. Khadka, Y. Shirai, M. Yanagida, K. Uto and K. Miyano, Analysis of degradation kinetics of halide perovskite solar cells induced by light and heat stress, Sol. Energy Mater. Sol. Cells, 2022, 246, 111899 CrossRef CAS.
- G. Abdelmageed, L. Jewell, K. Hellier, L. Seymour, B. Luo, F. Bridges, J. Z. Zhang and S. Carter, Mechanisms for light induced degradation in MAPbI3 perovskite thin films and solar cells, Appl. Phys. Lett., 2016, 109, 233905 CrossRef.
- B. Rivkin, P. Fassl, Q. Sun, A. D. Taylor, Z. Chen and Y. Vaynzof, Effect of Ion Migration-Induced Electrode Degradation on the Operational Stability of Perovskite Solar Cells, ACS Omega, 2018, 3, 10042–10047 CrossRef CAS.
- Y. Gao, R. Wang and Y. Liu, Progress of Two-dimensional Perovskite Solar Cells Based on Aromatic Organic Spacers, Chin. J. Lumin., 2023, 44, 449–465 CAS.
- I. C. Smith, E. T. Hoke, D. Solis-Ibarra, M. D. McGehee and H. I. Karunadasa, A layered hybrid perovskite solar-cell absorber with enhanced moisture stability, Angew. Chem., Int. Ed., 2014, 53, 11232–11235 CrossRef CAS PubMed.
- H. Lai, B. Kan, T. Liu, N. Zheng, Z. Xie, T. Zhou, X. Wan, X. Zhang, Y. Liu and Y. Chen, Two-Dimensional Ruddlesden-Popper Perovskite with Nanorod-like Morphology for Solar Cells with Efficiency Exceeding 15, J. Am. Chem. Soc., 2018, 140, 11639–11646 CrossRef CAS PubMed.
- D. Lu, G. Lv, Z. Xu, Y. Dong, X. Ji and Y. Liu, Thiophene-Based Two-Dimensional Dion-Jacobson Perovskite Solar Cells with over 15% Efficiency, J. Am. Chem. Soc., 2020, 142, 11114–11122 CrossRef CAS PubMed.
- Q. Li, Y. Dong, G. Lv, T. Liu, D. Lu, N. Zheng, X. Dong, Z. Xu, Z. Xie and Y. Liu, Fluorinated Aromatic Formamidinium Spacers Boost Efficiency of Layered Ruddlesden–Popper Perovskite Solar Cells, ACS Energy Lett., 2021, 6, 2072–2080 CrossRef CAS.
- R. Wang, X. Dong, Q. Ling, Q. Fu, Z. Hu, Z. Xu, H. Zhang, Q. Li and Y. Liu, Spacer Engineering for 2D Ruddlesden–Popper Perovskites with an Ultralong Carrier Lifetime of Over 18 μs Enable Efficient Solar Cells, ACS Energy Lett., 2022, 7, 3656–3665 CrossRef CAS.
- Y. Zhang and N.-G. Park, Quasi-Two-Dimensional Perovskite Solar Cells with Efficiency Exceeding 22%, ACS Energy Lett., 2022, 7, 757–765 CrossRef CAS.
- D. Li, Z. Xing, X. Meng, X. Hu, T. Hu and Y. Chen, Selection of Functional Spacer Cations for Efficient 2D/3D Perovskite Solar Cells, CCS Chem., 2023, 5, 781–801 CrossRef CAS.
- G. Grancini and M. K. Nazeeruddin, Dimensional tailoring of hybrid perovskites for photovoltaics, Nat. Rev. Mater., 2018, 4, 4–22 CrossRef.
- W. Travis, E. N. K. Glover, H. Bronstein, D. O. Scanlon and R. G. Palgrave, On the application of the tolerance factor to inorganic and hybrid halide perovskites: a revised system, Chem. Sci., 2016, 7, 4548–4556 RSC.
- A. Krishna, S. Gottis, M. K. Nazeeruddin and F. Sauvage, Mixed Dimensional 2D/3D Hybrid Perovskite Absorbers: The Future of Perovskite Solar Cells?, Adv. Funct. Mater., 2019, 29, 1806482 CrossRef.
- Y.-T. Li, L. Han, H. Liu, K. Sun, D. Luo, X.-L. Guo, D.-L. Yu and T.-L. Ren, Review on Organic–Inorganic Two-Dimensional Perovskite-Based Optoelectronic Devices, ACS Appl. Electron. Mater., 2022, 4, 547–567 CrossRef CAS.
- Y. Xu, M. Wang, Y. Lei, Z. Ci and Z. Jin, Crystallization Kinetics in 2D Perovskite Solar Cells, Adv. Energy Mater., 2020, 10, 2002558 CrossRef CAS.
- L. Yan, J. Ma, P. Li, S. Zang, L. Han, Y. Zhang and Y. Song, Charge-Carrier Transport in Quasi-2D Ruddlesden–Popper Perovskite Solar Cells, Adv. Mater., 2022, 34, 2106822 CrossRef CAS.
- Z. Wang, Q. Lin, F. P. Chmiel, N. Sakai, L. M. Herz and H. J. Snaith, Efficient ambient-air-stable solar cells with 2D–3D heterostructured butylammonium-caesium-formamidinium lead halide perovskites, Nat. Energy, 2017, 2, 17135 CrossRef CAS.
- J. W. Lee, Z. Dai, T. H. Han, C. Choi, S. Y. Chang, S. J. Lee, N. De Marco, H. Zhao, P. Sun, Y. Huang and Y. Yang, 2D perovskite stabilized phase-pure formamidinium perovskite solar cells, Nat. Commun., 2018, 9, 3021 CrossRef PubMed.
- C. Luo, G. Zheng, F. Gao, X. Wang, Y. Zhao, X. Gao and Q. Zhao, Facet orientation tailoring via 2D-seed- induced growth enables highly efficient and stable perovskite solar cells, Joule, 2022, 6, 240–257 CrossRef CAS.
- T. Yang, C. Ma, W. Cai, S. Wang, Y. Wu, J. Feng, N. Wu, H. Li, W. Huang, Z. Ding, L. Gao, S. Liu and K. Zhao, Amidino-based Dion-Jacobson 2D perovskite for efficient and stable 2D/3D heterostructure perovskite solar cells, Joule, 2023, 7, 574–586 CrossRef CAS.
- X. Zheng, Y. Hou, C. Bao, J. Yin, F. Yuan, Z. Huang, K. Song, J. Liu, J. Troughton, N. Gasparini, C. Zhou, Y. Lin, D.-J. Xue, B. Chen, A. K. Johnston, N. Wei, M. N. Hedhili, M. Wei, A. Y. Alsalloum, P. Maity, B. Turedi, C. Yang, D. Baran, T. D. Anthopoulos, Y. Han, Z.-H. Lu, O. F. Mohammed, F. Gao, E. H. Sargent and O. M. Bakr, Managing grains and interfaces via ligand anchoring enables 22.3%-efficiency inverted perovskite solar cells, Nat. Energy, 2020, 5, 131–140 CrossRef CAS.
- Y. Liu, S. Akin, L. Pan, R. Uchida, N. Arora, J. V. Milić, A. Hinderhofer, F. Schreiber, A. R. Uhl, S. M. Zakeeruddin, A. Hagfeldt, M. I. Dar and M. Grätzel, Ultrahydrophobic 3D/2D fluoroarene bilayer-based water-resistant perovskite solar cells with efficiencies exceeding 22%, Sci. Adv., 2019, 5, eaaw2543 CrossRef CAS PubMed.
- A. H. Proppe, M. Wei, B. Chen, R. Quintero-Bermudez, S. O. Kelley and E. H. Sargent, Photochemically Cross-Linked Quantum Well Ligands for 2D/3D Perovskite Photovoltaics with Improved Photovoltage and Stability, J. Am. Chem. Soc., 2019, 141, 14180–14189 CrossRef CAS PubMed.
- Y.-W. Jang, S. Lee, K. M. Yeom, K. Jeong, K. Choi, M. Choi and J. H. Noh, Intact 2D/3D halide junction perovskite solar cells via solid-phase in-plane growth, Nat. Energy, 2021, 6, 63–71 CrossRef CAS.
- A. H. Proppe, A. Johnston, S. Teale, A. Mahata, R. Quintero-Bermudez, E. H. Jung, L. Grater, T. Cui, T. Filleter, C. Y. Kim, S. O. Kelley, F. De Angelis and E. H. Sargent, Multication perovskite 2D/3D interfaces form via progressive dimensional reduction, Nat. Commun., 2021, 12, 3472 CrossRef CAS PubMed.
- Y. Du, D. Zhu, Q. Cai, S. Yuan, G. Shen, P. Dong, C. Mu, Y. Wang and X.-C. Ai, Spacer Engineering of Thiophene-Based Two-Dimensional/Three-Dimensional Hybrid Perovskites for Stable and Efficient Solar Cells, J. Phys. Chem. C, 2022, 126, 3351–3358 CrossRef CAS.
- M.-H. Li, H.-H. Yeh, Y.-H. Chiang, U. S. Jeng, C.-J. Su, H.-W. Shiu, Y.-J. Hsu, N. Kosugi, T. Ohigashi, Y.-A. Chen, P.-S. Shen, P. Chen and T.-F. Guo, Highly Efficient 2D/3D Hybrid Perovskite Solar Cells via Low-Pressure Vapor-Assisted Solution Process, Adv. Mater., 2018, 30, 1801401 CrossRef.
- M.-G. La-Placa, L. Gil-Escrig, D. Guo, F. Palazon, T. J. Savenije, M. Sessolo and H. J. Bolink, Vacuum-Deposited 2D/3D Perovskite Heterojunctions, ACS Energy Lett., 2019, 4, 2893–2901 CrossRef CAS.
- D. S. Lee, J. S. Yun, J. Kim, A. M. Soufiani, S. Chen, Y. Cho, X. Deng, J. Seidel, S. Lim, S. Huang and A. W. Y. Ho-Baillie, Passivation of Grain Boundaries by Phenethylammonium in Formamidinium-Methylammonium Lead Halide Perovskite Solar Cells, ACS Energy Lett., 2018, 3, 647–654 CrossRef CAS.
- T. Liu, J. Guo, D. Lu, Z. Xu, Q. Fu, N. Zheng, Z. Xie, X. Wan, X. Zhang, Y. Liu and Y. Chen, Spacer Engineering Using Aromatic Formamidinium in 2D/3D Hybrid Perovskites for Highly Efficient Solar Cells, ACS Nano, 2021, 15, 7811–7820 CrossRef CAS PubMed.
- T. Zhou, Z. Xu, R. Wang, X. Dong, Q. Fu and Y. Liu, Crystal Growth Regulation of 2D/3D Perovskite Films for Solar Cells with Both High Efficiency and Stability, Adv. Mater., 2022, 34, e2200705 CrossRef PubMed.
- F. Yang, P. Zhang, M. A. Kamarudin, G. Kapil, T. Ma and S. Hayase, Addition Effect of Pyreneammonium Iodide to Methylammonium Lead Halide Perovskite-2D/3D Heterostructured Perovskite with Enhanced Stability, Adv. Funct. Mater., 2018, 28, 1804856 CrossRef.
- T. Zhou, H. Lai, T. Liu, D. Lu, X. Wan, X. Zhang, Y. Liu and Y. Chen, Highly Efficient and Stable Solar Cells Based on Crystalline Oriented 2D/3D Hybrid Perovskite, Adv. Mater., 2019, 31, e1901242 CrossRef PubMed.
- Y. Li, J. Wu, Y. Zhang, L. Zhang, X. Zhou, B. Hu, Z. Jiang, J. Zeng, D. Wang, Y. Liu, S. Chen, Z. Liu, C. Liu, X. Wang and B. Xu, Whether organic spacer cations induced 2D/3D or quasi-2D/3D mixed dimensional perovskites?, Chem. Eng. J., 2022, 450, 137887 CrossRef CAS.
- Y. Chen, Y. Sun, J. Peng, W. Zhang, X. Su, K. Zheng, T. Pullerits and Z. Liang, Tailoring Organic Cation of 2D Air-Stable Organometal Halide Perovskites for Highly Efficient Planar Solar Cells, Adv. Energy Mater., 2017, 7, 1700162 CrossRef.
- X. Zhang, X. Ren, B. Liu, R. Munir, X. Zhu, D. Yang, J. Li, Y. Liu, D.-M. Smilgies, R. Li, Z. Yang, T. Niu, X. Wang, A. Amassian, K. Zhao and S. Liu, Stable high efficiency two-dimensional perovskite solar cells via cesium doping, Energy Environ. Sci., 2017, 10, 2095–2102 RSC.
- N. Zhou, Y. Shen, L. Li, S. Tan, N. Liu, G. Zheng, Q. Chen and H. Zhou, Exploration of Crystallization Kinetics in Quasi Two-Dimensional Perovskite and High Performance Solar Cells, J. Am. Chem. Soc., 2018, 140, 459–465 CrossRef CAS PubMed.
- B.-w Park, H. W. Kwon, Y. Lee, D. Y. Lee, M. G. Kim, G. Kim, K.-j Kim, Y. K. Kim, J. Im, T. J. Shin and S. I. Seok, Stabilization of formamidinium lead triiodide α-phase with isopropylammonium chloride for perovskite solar cells, Nat. Energy, 2021, 6, 419–428 CrossRef CAS.
- S. Sandhu, R. Singh, K. Yoo, M. Kumar and J.-J. Lee, Effect of binary additives in mixed 2D/3D Sn-based perovskite solar cells, J. Power Sources, 2021, 491, 229574 CrossRef CAS.
- Y. Zhong, G. Liu, Y. Su, W. Sheng, L. Gong, J. Zhang, L. Tan and Y. Chen, Diammonium Molecular Configuration-Induced Regulation of Crystal Orientation and Carrier Dynamics for Highly Efficient and Stable 2D/3D Perovskite Solar Cells, Angew. Chem., Int. Ed., 2022, 61, e202114588 CrossRef CAS PubMed.
- S.-C. Yun, S. Ma, H.-C. Kwon, K. Kim, G. Jang, H. Yang and J. Moon, Amino acid salt-driven planar hybrid perovskite solar cells with enhanced humidity stability, Nano Energy, 2019, 59, 481–491 CrossRef CAS.
- H. Zheng, X. Dong, W. Wu, G. Liu and X. Pan, Multifunctional Heterocyclic-Based Spacer Cation for Efficient and Stable 2D/3D Perovskite Solar Cells, ACS Appl. Mater. Interfaces, 2022, 14, 9183–9191 CrossRef CAS PubMed.
- N. Zhou, Y. Zhang, Z. Huang, Z. Guo, C. Zhu, J. He, Q. Chen, W. Sun and H. Zhou, Mobile Media Promotes Orientation of 2D/3D Hybrid Lead Halide Perovskite for Efficient Solar Cells, ACS Nano, 2021, 15, 8350–8362 CrossRef CAS PubMed.
- G. Liu, H. Zheng, X. Xu, L.-Z. Zhu, X. Zhang and X. Pan, Design of High-Efficiency and Environmentally Stable Mixed-Dimensional Perovskite Solar Cells Based on Cesium-Formamidinium Lead Halide Component, Chem. Mater., 2018, 30, 7691–7698 CrossRef CAS.
- J. Xu, J. Cui, S. Yang, Z. Liu, X. Guo, Y. Che, D. Xu, W. Zhao, N. Yuan, J. Ding and S. Liu, Stable High-Efficiency CsPbI2Br Solar Cells by Designed Passivation Using Multifunctional 2D Perovskite, Adv. Funct. Mater., 2022, 32, 2202829 CrossRef CAS.
- Z. Wang, Y. Lu, Z. Xu, J. Hu, Y. Chen, C. Zhang, Y. Wang, F. Guo and Y. Mai, An Embedding 2D/3D Heterostructure Enables High-Performance FA-Alloyed Flexible Perovskite Solar Cells with Efficiency over 20, Adv. Sci., 2021, 8, e2101856 CrossRef.
- Y. Li, J. Zhang, J. Xiang, H. Hu, H. Zhong and Y. Shi, A Novel 4,4'-Bipiperidine-Based Organic Salt for Efficient and Stable 2D-3D Perovskite Solar Cells, ACS Appl. Mater. Interfaces, 2022, 14, 22324–22331 CrossRef CAS.
- G. Wu, R. Liang, M. Ge, G. Sun, Y. Zhang and G. Xing, Surface Passivation Using 2D Perovskites toward Efficient and Stable Perovskite Solar Cells, Adv. Mater., 2022, 34, e2105635 CrossRef PubMed.
- Y. Hu, J. Schlipf, M. Wussler, M. L. Petrus, W. Jaegermann, T. Bein, P. Muller-Buschbaum and P. Docampo, Hybrid Perovskite/Perovskite Heterojunction Solar Cells, ACS Nano, 2016, 10, 5999–6007 CrossRef CAS PubMed.
- C. Ma, C. Leng, Y. Ji, X. Wei, K. Sun, L. Tang, J. Yang, W. Luo, C. Li, Y. Deng, S. Feng, J. Shen, S. Lu, C. Du and H. Shi, 2D/3D perovskite hybrids as moisture-tolerant and efficient light absorbers for solar cells, Nanoscale, 2016, 8, 18309–18314 RSC.
- G. Grancini, C. Roldan-Carmona, I. Zimmermann, E. Mosconi, X. Lee, D. Martineau, S. Narbey, F. Oswald, F. De Angelis, M. Graetzel and M. K. Nazeeruddin, One-Year stable perovskite solar cells by 2D/3D interface engineering, Nat. Commun., 2017, 8, 15684 CrossRef CAS PubMed.
- P. Chen, Y. Bai, S. Wang, M. Lyu, J. H. Yun and L. Wang, In Situ Growth of 2D Perovskite Capping Layer for Stable and Efficient Perovskite Solar Cells, Adv. Funct. Mater., 2018, 28, 1706923 CrossRef.
- F. Cao, H. Chen, S. Wang, P. Chen, C. Zhu, Z. Lan, W. Sun, Y. Li and J. Wu, One-step constructed dual interfacial layers for stable perovskite solar cells, Mater. Today Phys., 2022, 27, 100796 CrossRef CAS.
- Y. Liu, B. J. Kim, H. Wu, G. Boschloo and E. M. J. Johansson, Efficient and Stable FAPbBr3 Perovskite Solar Cells via Interface Modification by a Low-Dimensional Perovskite Layer, ACS
Appl. Energy Mater., 2021, 4, 9276–9282 CrossRef CAS.
- Y. Lv, Y. Shi, X. Song, J. Liu, M. Wang, S. Wang, Y. Feng, S. Jin and C. Hao, Bromine Doping as an Efficient Strategy to Reduce the Interfacial Defects in Hybrid Two-Dimensional/Three-Dimensional Stacking Perovskite Solar Cells, ACS Appl. Mater. Interfaces, 2018, 10, 31755–31764 CrossRef CAS PubMed.
- H. Kim, M. Pei, Y. Lee, A. A. Sutanto, S. Paek, V. I. E. Queloz, A. J. Huckaba, K. T. Cho, H. J. Yun, H. Yang and M. K. Nazeeruddin, Self-Crystallized Multifunctional 2D Perovskite for Efficient and Stable Perovskite Solar Cells, Adv. Funct. Mater., 2020, 30, 1910620 CrossRef CAS.
- C. Ji, C. Liang, Q. Song, H. Gong, N. Liu, F. You, D. Li and Z. He, Interface Engineering of 2D/3D Perovskite Heterojunction Improves Photovoltaic Efficiency and Stability, Solar RRL, 2021, 5, 2100072 CrossRef CAS.
- Y. Yu, R. Liu, C. Liu, T. Hou, Q. Wu, M. Zhang and H. Yu, Dimensional Engineering Enables 1.31 V Open-Circuit Voltage for Efficient and Stable Wide-Bandgap Halide Perovskite Solar Cells, Solar RRL, 2022, 6, 2200021 CrossRef CAS.
- Q. Zhou, L. Liang, J. Hu, B. Cao, L. Yang, T. Wu, X. Li, B. Zhang and P. Gao, High-Performance Perovskite Solar Cells with Enhanced Environmental Stability Based on a (p-FC6H4C2H4NH3)2[PbI4] Capping Layer, Adv. Energy Mater., 2019, 9, 1802595 CrossRef.
- R. Garai, R. K. Gupta, M. Hossain and P. K. Iyer, Surface recrystallized stable 2D–3D graded perovskite solar cells for efficiency beyond 21%, J. Mater. Chem. A, 2021, 9, 26069–26076 RSC.
- H. Yu, F. Xu, C. Li, B. Yuan, T. Liu, Z. Pan, Y. Zhou and B. Cao, In-situ fluorinated 2D/3D invert perovskite film solar cell with enhanced ambient stability, Sol. Energy, 2021, 221, 583–590 CrossRef CAS.
- Y. Cai, J. Wen, Z. Liu, F. Qian, C. Duan, K. He, W. Zhao, S. Zhan, S. Yang, J. Cui and S. Liu, Graded 2D/3D (CF3-PEA)2FA0.85MA0.15Pb2I7/FA0.85MA0.15PbI3 heterojunction for stable perovskite solar cell with an efficiency over 23.0%, J. Energy Chem., 2022, 65, 480–489 CrossRef CAS.
- H. Ran, L. Cao, Y. Zhao, M. Chen, P. Qi, H. Wu, Y. Lu, Y. Zhang, S. Wang and Y. Tang, Constructing 2D passivation layer on perovskites based on 3-chlorobenzylamine enables efficient and stable perovskite solar cells, J. Alloys Compd., 2022, 926, 166891 CrossRef CAS.
- M. D. Malouangou, Y. Zhang, Y. Yang, M. T. Mbumba, M. W. Akram, E. Rop, J. Tsiba Matondo and M. Guli, Enhancing the efficiency and stability of 2D-3D perovskite solar cells with embedded interface passivation with diammonium cation spacer, Sol. Energy Mater. Sol. Cells, 2023, 251, 112135 CrossRef CAS.
- W. H. Rodebush and I. Feldman, Ultraviolet Absorption Spectra of Organic Molecules. III. Mechanical Interference of Substituent Groups with Resonance Configurations, J. Am. Chem. Soc., 1946, 68, 896–899 CrossRef CAS PubMed.
- J.-P. Deng, W.-T. Yang and B. Rånby, Auto-Initiating Performance of Styrene on Surface Photografting Polymerization, Macromol. Rapid Commun., 2001, 22, 535–538 CrossRef CAS.
- C. Chen, J. Liang, J. Zhang, X. Liu, X. Yin, H. Cui, H. Wang, C. Wang, Z. Li, J. Gong, Q. Lin, W. Ke, C. Tao, B. Da, Z. Ding, X. Xiao and G. Fang, Interfacial engineering of a thiophene-based 2D/3D perovskite heterojunction for efficient and stable inverted wide-bandgap perovskite solar cells, Nano Energy, 2021, 90, 106608 CrossRef CAS.
- A. A. Sutanto, P. Caprioglio, N. Drigo, Y. J. Hofstetter, I. Garcia-Benito, V. I. E. Queloz, D. Neher, M. K. Nazeeruddin, M. Stolterfoht, Y. Vaynzof and G. Grancini, 2D/3D perovskite engineering eliminates interfacial recombination losses in hybrid perovskite solar cells, Chem, 2021, 7, 1903–1916 CAS.
- A. A. Sutanto, N. Drigo, V. I. E. Queloz, I. Garcia-Benito, A. R. Kirmani, L. J. Richter, P. A. Schouwink, K. T. Cho, S. Paek, M. K. Nazeeruddin and G. Grancini, Dynamical evolution of the 2D/3D interface: a hidden driver behind perovskite solar cell instability, J. Mater. Chem. A, 2020, 8, 2343–2348 RSC.
- Y. Huang, Z. Yuan, J. Yang, S. Yin, A. Liang, G. Xie, C. Feng, Z. Zhou, Q. Xue, Y. Pan, F. Huang and Y. Chen, Highly efficient perovskite solar cells by building 2D/3D perovskite heterojuction in situ for interfacial passivation and energy level adjustment, Sci. China: Chem., 2023, 66, 449–458 CrossRef CAS.
- W. Zhou, L. Jia, M. Chen, X. Li, Z. Su, Y. Shang, X. Jiang, X. Gao, T. Chen, M. Wang, Z. Zhu, Y. Lu and S. Yang, An Improbable Amino-Functionalized Fullerene Spacer Enables 2D/3D Hybrid Perovskite with Enhanced Electron Transport in Solar Cells, Adv. Funct. Mater., 2022, 32, 2201374 CrossRef CAS.
- Q. Wang, F. Lin, C.-C. Chueh, T. Zhao, M. Eslamian and A. K. Y. Jen, Enhancing efficiency of perovskite solar cells by reducing defects through imidazolium cation incorporation, Mater. Today Energy, 2018, 7, 161–168 CrossRef.
- J. Kim, T. Hwang, B. Lee, S. Lee, K. Park, H. H. Park and B. Park, An Aromatic Diamine Molecule as the A-Site Solute for Highly Durable and Efficient Perovskite Solar Cells, Small Methods, 2019, 3, 1800361 CrossRef.
- C. Wang, X. Wang, Z. He, B. Zhou, D. Qu, Y. Wang, H. Hu, Q. Hu and Y. Tu, Minimizing voltage deficit in Methylammonium-Free perovskite solar cells via surface reconstruction, Chem. Eng. J., 2022, 444, 136622 CrossRef CAS.
- I. Zimmermann, S. Aghazada and M. K. Nazeeruddin, Lead and HTM Free Stable Two-Dimensional Tin Perovskites with Suitable Band Gap for Solar Cell Applications, Angew. Chem., Int. Ed., 2019, 58, 1072–1076 CrossRef CAS PubMed.
- Q. Li, Q. Shu, Y. Wang, D.-Y. Zhou, F. Wang, Q. Yuan, S. Yi, H. Wang and L. Feng, Interfacial Engineering by In Situ Building of a 3D/2D Heterojunction for Inverted CsPbI2Br Solar Cells: Beyond Moisture Proof, ACS Appl. Energy Mater., 2021, 4, 10081–10090 CrossRef CAS.
- K. Wojciechowski, T. Leijtens, S. Siprova, C. Schlueter, M. T. Horantner, J. T. Wang, C. Z. Li, A. K. Jen, T. L. Lee and H. J. Snaith, C60 as an Efficient n-Type Compact Layer in Perovskite Solar Cells, J. Phys. Chem. Lett., 2015, 6, 2399–2405 CrossRef CAS.
- Y. Shao, Z. Xiao, C. Bi, Y. Yuan and J. Huang, Origin and elimination of photocurrent hysteresis by fullerene passivation in CH3NH3PbI3 planar heterojunction solar cells, Nat. Commun., 2014, 5, 5784 CrossRef CAS PubMed.
- Y. Bai, Q. Dong, Y. Shao, Y. Deng, Q. Wang, L. Shen, D. Wang, W. Wei and J. Huang, Enhancing stability and efficiency of perovskite solar cells with crosslinkable silane-functionalized and doped fullerene, Nat. Commun., 2016, 7, 12806 CrossRef CAS.
- Y. Li, Y. Zhao, Q. Chen, Y. M. Yang, Y. Liu, Z. Hong, Z. Liu, Y. T. Hsieh, L. Meng, Y. Li and Y. Yang, Multifunctional Fullerene Derivative for Interface Engineering in Perovskite Solar Cells, J. Am. Chem. Soc., 2015, 137, 15540 CrossRef CAS PubMed.
- W. Chi and S. K. Banerjee, Engineering strategies for two-dimensional perovskite solar cells, Trends Chem., 2022, 4, 1005–1020 CrossRef CAS.
- Y. Lin, Y. Bai, Y. Fang, Z. Chen, S. Yang, X. Zheng, S. Tang, Y. Liu, J. Zhao and J. Huang, Enhanced Thermal Stability in Perovskite Solar Cells by Assembling 2D/3D Stacking Structures, J. Phys. Chem. Lett., 2018, 9, 654–658 CrossRef CAS PubMed.
- S. Sidhik, Y. Wang, M. De Siena, R. Asadpour, A. J. Torma, T. Terlier, K. Ho, W. Li, A. B. Puthirath, X. Shuai, A. Agrawal, B. Traore, M. Jones, R. Giridharagopal, P. M. Ajayan, J. Strzalka, D. S. Ginger, C. Katan, M. A. Alam, J. Even, M. G. Kanatzidis and A. D. Mohite, Deterministic fabrication of 3D/2D perovskite bilayer stacks for durable and efficient solar cells, Science, 2022, 377, 1425–1430 CrossRef CAS PubMed.
- D. Zhang, X. Wang, Z. Fan, X. Xia and F. Li, Nondestructive Post-Treatment Enabled by In Situ Generated 2D Perovskites Derived from Multi-ammonium Molecule Vapor for High-Performance 2D/3D Bilayer Perovskite Solar Cells, ACS Appl. Mater. Interfaces, 2022, 14, 51053–51065 CrossRef CAS.
- C. Deng, J. Wu, Y. Du, Q. Chen, Z. Song, G. Li, X. Wang, J. Lin, W. Sun, M. Huang, Y. Huang, P. Gao and Z. Lan, Surface Reconstruction and In Situ Formation of 2D Layer for Efficient and Stable 2D/3D Perovskite Solar Cells, Small Methods, 2021, 5, e2101000 CrossRef.
- X. Wang, Y. Wang, T. Zhang, X. Liu and Y. Zhao, Steric Mixed-Cation 2D Perovskite as a Methylammonium Locker to Stabilize MAPbI(3), Angew. Chem., Int. Ed., 2020, 59, 1469–1473 CrossRef CAS PubMed.
- W. Fan, Y. Shen, K. Deng, Q. Chen and Y. Bai, A tailored spacer molecule in 2D/3D heterojunction for ultralow-voltage-loss and stable perovskite solar cells, J. Mater. Chem. A, 2021, 9, 26829–26838 RSC.
- X. Yue, X. Zhao, B. Fan, Y. Yang, L. Yan, S. Qu, H. Huang, Q. Zhang, H. Yan, P. Cui, J. Ji, J. Ma and M. Li, Surface Regulation through Dipolar Molecule Boosting the Efficiency of Mixed 2D/3D Perovskite Solar Cell to 24%, Adv. Funct. Mater., 2022, 33, 2209921 CrossRef.
- Yukta, N. Parikh, R. D. Chavan, P. Yadav, M. K. Nazeeruddin and S. Satapathi, Highly Efficient and Stable 2D Dion Jacobson/3D Perovskite Heterojunction Solar Cells, ACS Appl. Mater. Interfaces, 2022, 14, 29744–29753 CrossRef CAS.
- S. J. Sung, J. Im, G. Kim, C. S. Moon, J. J. Yoo, S. S. Shin, N. J. Jeon, B. S. Ma, D. J. Kim, T. S. Kim and J. Seo, Molecular Engineering for Function-Tailored Interface Modifier in High-Performance Perovskite Solar Cells, Adv. Energy Mater., 2022, 12, 2200758 CrossRef CAS.
- G. Li, J. Song, J. Wu, Y. Xu, C. Deng, Z. Song, X. Wang, Y. Du, Q. Chen, R. Li, W. Sun and Z. Lan, Surface defect passivation by 1,8-Naphthyridine for efficient and stable Formamidinium-based 2D/3D perovskite solar cells, Chem. Eng. J., 2022, 449, 137806 CrossRef CAS.
- Y. Lv, X. Song, Y. Yin, Y. Feng, H. Ma, C. Hao, S. Jin and Y. Shi, Hexylammonium Iodide Derived Two-Dimensional Perovskite as Interfacial Passivation Layer in Efficient Two-Dimensional/Three-Dimensional Perovskite Solar Cells, ACS Appl. Mater. Interfaces, 2020, 12, 698–705 CrossRef CAS.
- M. Singh, I. H. Ho, A. Singh, C.-W. Chan, J.-W. Yang, T.-F. Guo, H. Ahn, V. Tung, C. W. Chu and Y.-J. Lu, Unveiling Ultrafast Carrier Extraction in Highly Efficient 2D/3D Bilayer Perovskite Solar Cells, ACS Photonics, 2022, 9, 3584–3591 CrossRef CAS.
- B. Zhang, D. Gao, M. Li, X. Shang, Y. Li, C. Chen and T. Pauporte, Heterojunction In Situ Constructed by a Novel Amino Acid-Based Organic Spacer for Efficient and Stable Perovskite Solar Cells, ACS Appl. Mater. Interfaces, 2022, 14, 40902–40912 CrossRef CAS PubMed.
- T. Ye, A. Bruno, G. Han, T. M. Koh, J. Li, N. F. Jamaludin, C. Soci, S. G. Mhaisalkar and W. L. Leong, Efficient and Ambient-Air-Stable Solar Cell with Highly Oriented 2D@3D Perovskites, Adv. Funct. Mater., 2018, 28, 1801654 CrossRef.
- K. T. Cho, Y. Zhang, S. Orlandi, M. Cavazzini, I. Zimmermann, A. Lesch, N. Tabet, G. Pozzi, G. Grancini and M. K. Nazeeruddin, Water-Repellent Low-Dimensional Fluorous Perovskite as Interfacial Coating for 20% Efficient Solar Cells, Nano Lett., 2018, 18, 5467–5474 CrossRef CAS PubMed.
- Q. He, M. Worku, L. Xu, C. Zhou, H. Lin, A. J. Robb, K. Hanson, Y. Xin and B. Ma, Facile Formation of 2D-3D Heterojunctions on Perovskite Thin Film Surfaces for Efficient Solar Cells, ACS Appl. Mater. Interfaces, 2020, 12, 1159–1168 CrossRef CAS PubMed.
- Y. Huang, K. Yan, B. Niu, Z. Chen, E. Gu, H. Liu, B. Yan, J. Yao, H. Zhu, H. Chen and C.-Z. Li, Finite perovskite hierarchical structures via ligand confinement leading to efficient inverted perovskite solar cells, Energy Environ. Sci., 2023, 16, 557–564 RSC.
- X. Jiang, J. Zhang, S. Ahmad, D. Tu, X. Liu, G. Jia, X. Guo and C. Li, Dion-Jacobson 2D-3D perovskite solar cells with improved efficiency and stability, Nano Energy, 2020, 75, 104892 CrossRef CAS.
- Y. Yu, R. Liu, M. Xu and H. Yu, Diammonium-induced Dion-Jacobson 2D/3D wide-bandgap perovskite solar cells with enhanced efficiency and stability, EcoMat, 2022, 5, e12272 CrossRef.
- G. Li, J. Song, J. Wu, Z. Song, X. Wang, W. Sun, L. Fan, J. Lin, M. Huang, Z. Lan and P. Gao, Efficient and Stable 2D@3D/2D Perovskite Solar Cells Based on Dual Optimization of Grain Boundary and Interface, ACS Energy Lett., 2021, 6, 3614–3623 CrossRef CAS.
- J. Guo, B. Wang, D. Lu, T. Wang, T. Liu, R. Wang, X. Dong, T. Zhou, N. Zheng, Q. Fu, Z. Xie, X. Wan, G. Xing, Y. Chen and Y. Liu, Ultralong Carrier Lifetime Exceeding 20 micros in Lead Halide Perovskite Film Enable Efficient Solar Cells, Adv. Mater., 2023, e2212126 CrossRef PubMed.
- G. Yang, Z. Ren, K. Liu, M. Qin, W. Deng, H. Zhang, H. Wang, J. Liang, F. Ye, Q. Liang, H. Yin, Y. Chen, Y. Zhuang, S. Li, B. Gao, J. Wang, T. Shi, X. Wang, X. Lu, H. Wu, J. Hou, D. Lei, S. K. So, Y. Yang, G. Fang and G. Li, Stable and low-photovoltage-loss perovskite solar cells by multifunctional passivation, Nat. Photonics, 2021, 15, 681–689 CrossRef CAS.
- T. Zhao, C.-C. Chueh, Q. Chen, A. Rajagopal and A. K. Y. Jen, Defect Passivation of Organic–Inorganic Hybrid Perovskites by Diammonium Iodide toward High-Performance Photovoltaic Devices, ACS Energy Lett., 2016, 1, 757–763 CrossRef CAS.
- B. B. Yu, Z. Chen, Y. Zhu, Y. Wang, B. Han, G. Chen, X. Zhang, Z. Du and Z. He, Heterogeneous 2D/3D Tin-Halides Perovskite Solar Cells with Certified Conversion Efficiency Breaking 14, Adv. Mater., 2021, 33, e2102055 CrossRef PubMed.
- L. Iagher and L. Etgar, Effect of Cs on the Stability and Photovoltaic Performance of 2D/3D Perovskite-Based Solar Cells, ACS Energy Lett., 2018, 3, 366–372 CrossRef CAS.
- S.-H. Lee, S. Jeong, S. Seo, H. Shin, C. Ma and N.-G. Park, Acid Dissociation Constant: A Criterion for Selecting Passivation Agents in Perovskite Solar Cells, ACS Energy Lett., 2021, 1612–1621 CrossRef CAS.
- S. Jeong, S. Seo, H. Yang, H. Park, S. Shin, H. Ahn, D. Lee, J. H. Park, N. G. Park and H. Shin, Cyclohexylammonium-Based 2D/3D Perovskite Heterojunction with Funnel-Like Energy Band Alignment for Efficient Solar Cells (23.91%), Adv. Energy Mater., 2021, 11, 2102236 CrossRef CAS.
- S. Wang, F. Cao, P. Chen, R. He, A. Tong, Z. Lan, P. Gao, W. Sun and J. Wu, Two birds with one stone: Simultaneous realization of constructed 3D/2D heterojunction and p-doping of hole transport layer for highly efficient and stable perovskite solar cells, Chem. Eng. J., 2023, 453, 139721 CrossRef CAS.
- Y. Du, J. Wu, G. Li, X. Wang, Z. Song, C. Deng, Q. Chen, Y. Zou, W. Sun and Z. Lan, Bulky ammonium iodide and in-situ formed 2D Ruddlesden-Popper layer enhances the stability and efficiency of perovskite solar cells, J. Colloid Interface Sci., 2022, 614, 247–255 CrossRef CAS PubMed.
- H. Ju, Y. Ma, Y. Cao, Z. Wang, L. Liu, M. Wan, T. Mahmoudi, Y.-B. Hahn, Y. Wang and Y. Mai, Roles of Long-Chain Alkylamine Ligands in Triple-Halide Perovskites for Efficient NiOx-Based Inverted Perovskite Solar Cells, Solar RRL, 2022, 6, 2101082 CrossRef CAS.
- J. Sun, X. Zhang, X. Ling, Y. Yang, Y. Wang, J. Guo, S. Liu, J. Yuan and W. Ma, A penetrated 2D/3D hybrid heterojunction for high-performance perovskite solar cells, J. Mater. Chem. A, 2021, 9, 23019–23027 RSC.
- M. A. Mahmud, H. T. Pham, T. Duong, Y. Yin, J. Peng, Y. Wu, W. Liang, L. Li, A. Kumar, H. Shen, D. Walter, H. T. Nguyen, N. Mozaffari, G. D. Tabi, G. Andersson, K. R. Catchpole, K. J. Weber and T. P. White, Combined Bulk and Surface Passivation in Dimensionally Engineered 2D-3D Perovskite Films via Chlorine Diffusion, Adv. Funct. Mater., 2021, 31, 2104251 CrossRef CAS.
- M. Dehghanipour, A. Behjat and H. Amrollahi Bioki, Fabrication of stable and efficient 2D/3D perovskite solar cells through post-treatment with TBABF4, J. Mater. Chem. C, 2021, 9, 957–966 RSC.
- H. Xu, G. Liu, X. Xu, S. Xu, L. Zhang, X. Chen, H. Zheng and X. Pan, Hydrophobic 2D Perovskite-Modified Layer with Polyfunctional Groups for Enhanced Performance and High Moisture Stability of Perovskite Solar Cells, Solar RRL, 2020, 4, 2000647 CrossRef CAS.
- X. Zhang, W. Zhou, X. Chen, Y. Chen, X. Li, M. Wang, Y. Zhou, H. Yan, Z. Zheng and Y. Zhang, Dual Optimization of Bulk and Surface via Guanidine Halide for Efficient and Stable 2D/3D Hybrid Perovskite Solar Cells, Adv. Energy Mater., 2022, 12, 2201105 CrossRef CAS.
- B. Liu, J. Hu, D. He, L. Bai, Q. Zhou, W. Wang, C. Xu, Q. Song, D. Lee, P. Zhao, F. Hao, X. Niu, Z. Zang and J. Chen, Simultaneous Passivation of Bulk and Interface Defects with Gradient 2D/3D Heterojunction Engineering for Efficient and Stable Perovskite Solar Cells, ACS Appl. Mater. Interfaces, 2022, 14, 21079–21088 CrossRef CAS PubMed.
- C. Ge, Y. Z. B. Xue, L. Li, B. Tang and H. Hu, Recent Progress in 2D/3D Multidimensional Metal Halide Perovskites Solar Cells, Front. Mater., 2020, 7, 601179 CrossRef.
- W. Gao, H. Dong, N. Sun, L. Chao, W. Hui, Q. Wei, H. Li, Y. Xia, X. Gao, G. Xing, Z. Wu, L. Song, P. Müller-Buschbaum, C. Ran and Y. Chen, Chiral cation promoted interfacial charge extraction for efficient tin-based perovskite solar cells, J. Energy Chem., 2022, 68, 789–796 CrossRef CAS.
- M. A. Mahmud, T. Duong, Y. Yin, H. T. Pham, D. Walter, J. Peng, Y. Wu, L. Li, H. Shen, N. Wu, N. Mozaffari, G. Andersson, K. R. Catchpole, K. J. Weber and T. P. White, Double-Sided Surface Passivation of 3D Perovskite Film for High-Efficiency Mixed-Dimensional Perovskite Solar Cells, Adv. Funct. Mater., 2019, 30, 1907962 CrossRef.
|
This journal is © the Partner Organisations 2024 |