DOI:
10.1039/D4QI01601G
(Research Article)
Inorg. Chem. Front., 2024,
11, 5435-5448
Anticancer profile of coumarin 6-based Ir(III) photocatalysts under normoxia and hypoxia by ROS generation and NADH oxidation†
Received
25th June 2024
, Accepted 29th June 2024
First published on 1st July 2024
Abstract
Herein, we have designed, synthesized, and characterized two novel coumarin-6-based Ir(III) photo-catalysts viz. [Ir(CO6)2(dppz)]Cl (Ir1) and [Ir(CO6)2(aip)]Cl (Ir2), where CO6 = coumarin 6, dppz = dipyrido[3,2-a:2′,3′-c]phenazine, and aip = 2-(anthracen-9-yl)-1H-imidazo[4,5-f][1,10]phenanthroline. Coumarin 6 introduced a green light absorption property. When exposed to green light, complexes Ir1 and Ir2 demonstrated catalytic NADH photo-oxidation with a TOF of ca. 840–1000 h−1 in PBS, comparable to or higher than the highest achieved TOF with previously reported Ir(III)-based photo-catalysts for NADH oxidation. Ir1 and Ir2 also generated 1O2 on green light irradiation. Both the complexes showed remarkable photo-toxicities against human lung adenocarcinoma cells (A549) and human cervical cancer cells (HeLa) with the IC50 and PIs (dark IC50/light IC50) in the range of 0.5–1.6 μM and >28–71, respectively. Interestingly, these complexes did not show any notable toxicities toward the normal human bronchial epithelial (BEAS-2B) cell line under dark conditions. The selectivity index (SI = light IC50 in normal cells/light IC50 in cancer cells) of Ir1 and Ir2 for the killing of cancer cells was in the range of 7–19. Moreover, Ir1 and Ir2 also showed good phototoxicity against A549 cancer cells under hypoxic conditions, revealing their potential for hypoxic tumor treatment. The mechanistic investigation of the most potent complex Ir2 in A549 cells revealed that it localized in the mitochondria, generated ROS and oxidized NADH during light exposure, ultimately causing cell apoptosis via mitochondrial depolarization. This strategy of killing cancer cells by combining type-I and type-II mechanisms, i.e. via NADH oxidation, H2O2, ˙OH, and 1O2 generation, could provide efficient photo-activated chemotherapy.
1. Introduction
After the discovery of the anticancer activities of cis-platin, platinum-based chemotherapeutic drugs came into use in clinics for cancer chemotherapy.1,2 These drugs are now facing severe problems like drug resistance and lack of selectivity, which cause neurotoxicity, autotoxicity, nephrotoxicity, vomiting etc.3–6 These limitations restricted their usage towards various cancers.7,8 According to recent data, the global burden of cancer in 2040 will increase by 47% with respect to 2020.9 So, there is an immediate requirement for next-generation cancer therapy that can address these constraints of platinum-based drugs.10–22 The photocatalytic cancer drug development concept has originated recently to address these limitations.23–31 Photocatalytic cancer drugs can have multiple advantages, for example, (i) an effective low therapeutic dose, which could help to reduce inherent heavy metal toxicity, (ii) selective drug activation at the specified cancer site that may effectively reduce drug side effects, (iii) overcome drug resistance by combining type-I and type-II photosensitization pathways.23–32 It is important to discuss that the type I pathway mainly involves the electron transfer reactions between the photosensitizer and biomolecules, which ultimately generate ROS like H2O2, superoxide radical anion (O2˙−), or hydroxyl radicals (˙OH). Meanwhile, the type II mechanism is characterized by the energy transfer from 3PS to triplet oxygen (3O2), which ultimately produces singlet oxygen (1O2).33–38 Thus, a combination mechanism could provide multifunctional anticancer agents.
In photocatalytic cancer therapy, NADH has become the target for drug development due to its crucial role in various cellular processes such as the ATP generation process, cellular metabolism, the primary electron source of the electron transfer chain, and maintaining the redox balance.39–41 Cancer cells need a higher NADH concentration than normal cells for their fast cellular proliferation.42a Thus, intracellular NADH photo-oxidation is proven to be an efficient targeting anticancer mechanism.23–30
In photocatalytic anticancer research, mainly photosensitive Ir(III) and Ru(II) complexes have been explored as catalysts due to their low HOMO–LUMO energy gap, long-lived triplet excited state, and photo-stability.23–30 These complexes not only generate ROS, but also oxidize NADH on exposure to light to damage cancer cells.23–30 Ir(III) based photo-catalysts induced NADH oxidation via a single electron transfer mechanism.23–30 Sadler et al. reported that depletion in the concentration of NADH by the photoactive Ir(III) complex [Ir(ttpy)(pq)Cl]PF6 (where ‘ttpy’ represents 4′-(p-tolyl)-2,2′:6′,2′′-terpyridine and ‘pq’ represents 3-phenylisoquinoline) led to redox imbalance and metabolic disorder, which resulted in cell death.23 Subsequently, our group and Huang's group further established the usefulness of Ir(III) photocatalysts in catalytic cancer therapy.23–28 It is important to note that, to date, only 7 reports are available demonstrating the efficiency of Ir(III) complexes in photocatalytic cancer therapy (Chart 1).23–29
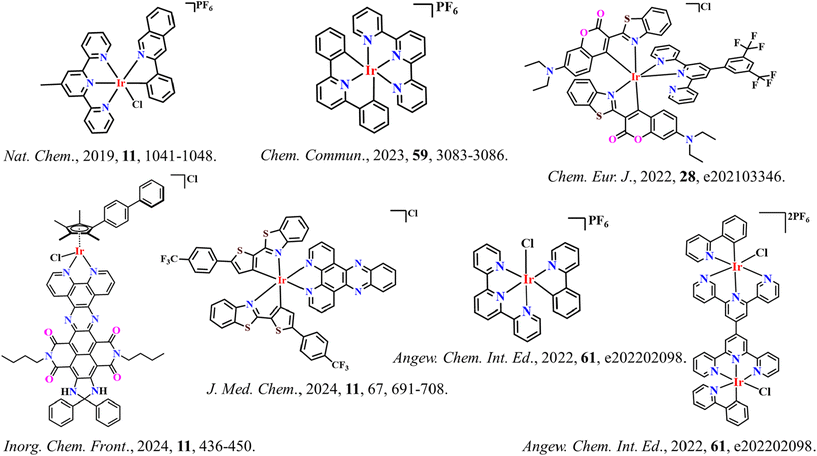 |
| Chart 1 Reported Ir(III)-based photocatalysts for photo-induced NADH oxidation. | |
Most of these reported photocatalysts absorbed 390–450 nm light; hence, blue light was used for photo-activation.23,26–29 Thus, one of the major challenges with Ir(III) photocatalysts is shifting the absorption maxima toward the red light region to achieve higher tissue penetration for treating deep-seated tumors.23,26–29 Another crucial challenge in this area is enhancing the NADH photo-oxidation efficiency of Ir(III) catalysts. So far, most of the reported Ir(III) catalysts had TOFs in the ca. 17–750 h−1 range.23,24,26–28 Increasing the NADH photo-oxidation TOF could also enhance the photo-cytotoxicity of the complexes.
Therefore, herein, to achieve the combined goal i.e., shifting of absorption toward the red light region via the green region and enhancement of TOFs of photo-induced NADH oxidation, we have designed and developed two novel Ir(III)-coumarin 6 cationic complexes, viz. [Ir(CO6)2(dppz)]Cl (Ir1) and [Ir(CO6)2(aip)]Cl (Ir2) (Fig. 1a and b), where CO6 = Coumarin 6, dppz = dipyrido[3,2-a:2′,3′-c]phenazine, and aip = 2-(anthracen-9-yl)-1H-imidazo[4,5-f][1,10]phenanthroline. Cationic Ir(III) complexes are reported to target mitochondria due to the negative mitochondrial membrane potential. Additionally, Ir(III) cationic complexes are also reported for enhanced emission, extended excited state lifetimes, and excellent photostability.42b–d Meanwhile, coumarin 6 shifted the absorption toward the green light region. Moreover, the coumarin 6-based fluorescence might help to study the complexes’ in-cell uptake and localization. It is important to note that several Ir(III)–coumarin conjugates were developed earlier for diverse applications such as in photoelectrochemical bioanalysis,43 as sensitizers of both n- and p-type DSSCs,44 oxygen sensing,45 singlet-oxygen generation,46–49 H2 evolution,50,51 anticancer applications,52–57 antibacterial therapy,58 as antioxidants,59 fluorescent probe sensors,60 and microRNA detectors.61 Here, the N,N-donor ligands enhance the lipophilicity of complexes. Also, the extended conjugation in dppz and aip could be used to achieve absorption in the green region by decreasing the HOMO–LUMO energy gap. Significant results of this work include (i) NADH photo-oxidation by the complexes with a very high TOF in an aqueous solution upon green light exposure, (ii) visible light-triggered anticancer activities of Ir1 and Ir2 at a sub-micromolar concentration with a notably high photo-cytotoxicity index (PI) and selectivity index (SI), and (iii) mitochondrial localization of Ir2 and thereafter ROS generation and NADH oxidation on light exposure to induce cell apoptosis via mitochondrial depolarization by combining type-I and type-II mechanisms.
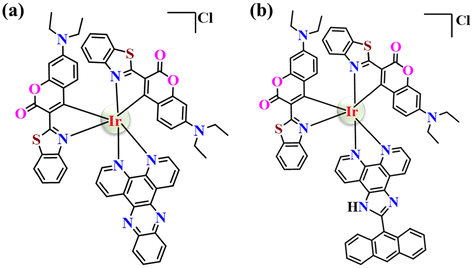 |
| Fig. 1 Structures of Ir1 (a) and Ir2 (b). | |
2. Results and discussion
2.1 Synthesis and characterization
Syntheses of the two novel Ir(III) photocatalysts viz., [Ir(CO6)2(dppz)]Cl (Ir1), and [Ir(CO6)2(aip)]Cl (Ir2) (Fig. 1a and b) involved the reaction of coumarin 6-coordinated Ir(III) μ-chloro-bridged dimers with different bidentate ligands in CHCl3 and MeOH (in a ratio of 3
:
1) under reflux conditions. The resulting complexes were purified through recrystallization from methanol. The complexes were characterized using 1H and 13C NMR, HR-MS, HPLC, UV-Vis, and fluorescence data (Fig. 2a and b, S1–S10, ESI†). Complexes Ir1 and Ir2 showed a molecular ion peak at m/z = 1171.25 and 1287.29 in mass spectra in acetonitrile, corresponding to the [M]+ ion (Fig. S1–S4, ESI†). Elemental analysis, 1H NMR and 13C NMR of Ir1 and Ir2 indicated high purity of the complexes (Fig. S5–S8, ESI†), which was further proved by HPLC analysis (Fig. S9 and S10, ESI†). The HPLC data of complex Ir2 indicated its ca. 99% purity, whereas, for complex Ir1, the purity was ca. 96% (Fig. S9 and S10, ESI†). The absorption spectra of complexes Ir1 and Ir2 (Fig. 2a) in MeOH showed a sharp band between 480–550 nm, corresponding to the intra-ligand π to π* transition in coumarin 6,25 and a broad band between 400–450 nm, corresponding to MLCT.25 Therefore, these complexes might be used to achieve green light-induced catalytic NADH oxidation and PDT activities. The UV-Vis absorption properties of Ir2 were also studied in PBS with various biologically important pH values such as 7.4, 7.0, and 6.0. As shown in Fig. S11,† a change in pH did not change the position of the absorption maxima. Additionally, between pH 7.4 to 7.0, there is not much change in the absorption intensity, while moving to 6.0, there was a minor change in the intensity, possibly due to the protonation of the imidazole nitrogen. Complexes Ir1 and Ir2 exhibited green fluorescence properties when excited at 460 nm, as shown in Fig. 2b. The source of this green fluorescence can be attributed to the presence of coumarin 6 within these complexes.25 Coumarin 6 is known to exhibit green fluorescence and is widely utilized in various applications due to this property.62,63 The green emissive nature of complexes was valuable for cellular imaging to obtain useful information about the in-cell uptake and localization of the complexes. In the dark or after light exposure, the minimal changes in the UV-Vis absorption spectra of complexes with time showed high stability of the complexes (2 μM) (Fig. S12–S15, ESI†). For effective photosensitizers, maintaining high levels of dark and light stability is crucial for their drug actions.23–32 Moreover, photo-stable photocatalysts could also sustain longer during catalysis.23–32 The efficient visible light absorption abilities and good photo-stabilities of Ir1 and Ir2 indicated that these complexes might be suitable for photo-activated anticancer responses on suitable visible light exposure via NADH photo-oxidation and ROS production like other reported Ir(III) photocatalysts.23–29
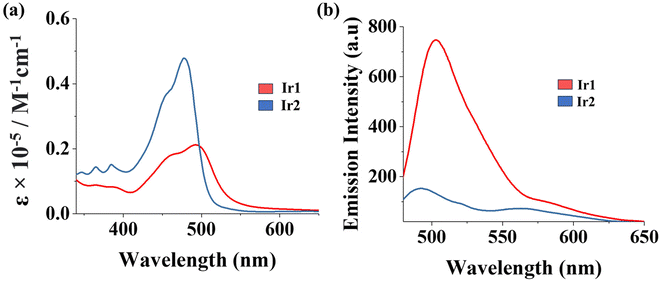 |
| Fig. 2 (a) Absorption spectra of complexes Ir1 and Ir2 in MeOH. (b) Emission spectra of complexes Ir1 and Ir2 (20 μM each) in DMSO : H2O (1 : 9, v/v) [λex = 460 nm]. | |
2.2 DFT calculation
To obtain detailed insight into the electronic structures of Ir1 and Ir2, density functional theory (DFT) calculations were employed at the B3LYP/LANL2DZ level using the Gaussian 16 software.28 The ground state optimized structures of Ir1 and Ir2 revealed a distorted octahedral geometry around the Ir(III) center as reported in the literature.25 The obtained frontier molecular orbitals from calculations, as shown in Fig. 3, presented the specific features for Ir1 and Ir2. In complex Ir1, the highest occupied molecular orbital (HOMO) was predominantly localized around the Ir(III) center and the coumarin 6 moiety, while the lowest unoccupied molecular orbital (LUMO) was mainly concentrated on the dppz moiety (Fig. 3). For complex Ir2, the HOMO was primarily based on the Ir(III) center and the coumarin 6 moiety similar to that in Ir1; however, the LUMO was centered on the phen moiety of the attached aip ligand (Fig. 3). The energy gap between HOMO and LUMO in complexes Ir1 and Ir2 was significantly lower (ΔEg < 2.3 eV), suggesting that both Ir1 and Ir2 are likely to be effective photosensitizers that can be activated with visible light. A catalyst that aspires to act as a PS must have a first excited triplet state greater than 0.98 eV with respect to S0, that is the energy differential between the ground state and the first excited singlet state of 3O2.63b Furthermore, the absorption maxima and ISC process form singlet to triplet excited states determine the efficacy of PS in the PDT process. Therefore, TD-DFT calculation was carried out to gain insight into the excited state properties of Ir1 and Ir2 (Fig. S16, ESI†). The calculated ten lowest vertical singlet–singlet/triplet transition energies are given in Tables S1 and S2.† The obtained result revealed that the lowest-lying triplet state of Ir1 and Ir2 is sufficient in energy to generate singlet oxygen (Fig. S16, ESI†). Moreover, it has been shown that the smaller energy gap (ΔES1-Tn < 0.3 eV) between S1 and Tn is potentially involved in ISC transitions.63c The possible ISC transition channels are given in Fig. S16.† The ISC transitions in Ir1 and Ir2 from S1 to Tn are mainly due to the narrow ΔEST.
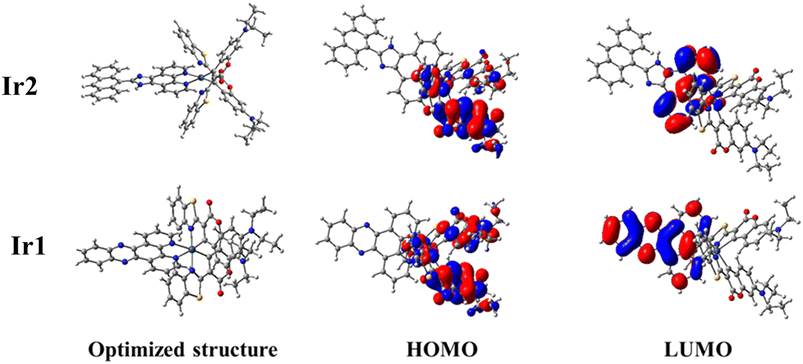 |
| Fig. 3 The energy-optimized structures, HOMO, and LUMO of complexes Ir1 and Ir2. | |
2.3 NADH photo-oxidation
As we discussed in the introduction, NADH plays a crucial role as an electron source in the mitochondrial electron transport chain (ETC), and its depletion can disrupt the hypoxic homeostasis of cancer cells.23–28 NADH has a crucial role in the ATP generation process, cellular metabolism, enzymatic functions like oxidoreductases, etc.23–28 Thus, recently, a novel anticancer mechanism has emerged involving in-cell photo-oxidation of NADH via an excited state single electron transfer pathway.23–28 The photocatalytic NADH oxidation potential of Ir1 (Fig. 4a) and Ir2 (Fig. 4b) was explored using UV-Vis spectroscopy by monitoring the time-dependent decrease in the NADH-based band at ca. 339 nm.23–28 When kept in the dark, complexes Ir1 and Ir2 (2 μM) showed no significant changes in the absorption spectra of NADH (240 μM) even after a 20-minute incubation period. However, under irradiation with green light (525 nm, 50.2 J cm−2), the complexes caused a significant decrease in the absorbance of NADH (Fig. 4a and b). The formation of NAD+ due to the NADH photo-oxidation was evident from the increase in the NAD+-based peak at ca. 265 nm (Fig. 4a and b). Ir2 showed the highest NADH photo-oxidation turnover number (TON), having a value of ca. 66 with a TOF of ca. 1000 h−1 (Table 1). These observed values are much higher than those of the previously reported Ir(III) photocatalysts, 1 (33.6 h−1), 2 (126.4 h−1), 3 (122.5 h−1) 4 (16.9 h−1), 5 (42.8 h−1), 6 (207.1 h−1) 10 (71.3 h−1), and 11 (270.6 h−1) (Table 1).23–25,27,28 Moreover, the TOF of Ir2 is comparable to the highest achieved TOF with previously reported blue light responsive Ir(III)-based photo-catalysts for NADH oxidation, 7 (1097.8 h−1), 8 (1060.4), and 9 (1357.2 h−1) (Table 1).25 In the aip ligand, the extended conjugation is more than dppz. So, the reduction potential might be higher in the case of Ir2. This was also supported by the cyclic voltammetry data (Fig. S17, ESI†). The higher reduction potential of Ir2 indicated its higher electron-accepting tendency than Ir1, making Ir2 a better NADH oxidant. Overall, the above results revealed that complexes Ir1 and Ir2 could be used as effective photo-catalysts for NADH oxidation and might alter the mitochondrial ETC, resulting in mitochondrial depolarization and cell death.23–29 Interestingly, during the photocatalytic NADH oxidation, the generation of hydrogen peroxide (H2O2) was detected using peroxide detection strips (Fig. 4c). Notably, no significant H2O2 production was observed in the dark (Fig. 4c). However, as the light exposure time was increased, the color intensity of the H2O2 strip increases, indicating more and more H2O2 generation (Fig. 4d). This was due to the increased consumption of NADH (Fig. 4e). This indicated that H2O2 was a byproduct of the NADH photocatalytic oxidation by the complexes via a type-I pathway.19,23,34 As reported by Sadler and coworkers for the Ir(III) complex,23 the photocatalytic mechanism here might involve steps like (i) the Ir(III) catalyst in the triplet excited state accepts an electron from NADH, resulting in the Ir(II) intermediate and NADH˙+ (ii) regeneration of the Ir(III) catalyst from the Ir(II) state by molecular oxygen, resulting in the superoxide anion radical O2˙− formation, and (iii) the generated O2˙− then accepts an electron and H+ from NADH˙+ to generate H2O2 and NAD+.
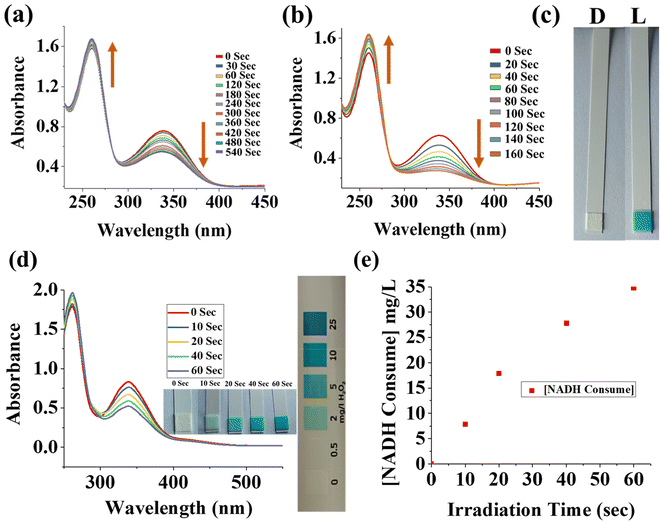 |
| Fig. 4 Green light-induced NADH (240 μM) oxidation by complex Ir1 (a) and Ir2 (b) (2 μM each), respectively, monitored by UV-Vis. Spectroscopy at different time intervals in 0.5% DMSO/99.5% PBS (v/v) solution (light source: 525 nm, 50.2 J cm−2). (c) Detection of H2O2 generation after irradiation of 1 min (light source: 525 nm, 50.2 J cm−2) for the reaction of complex Ir2 (2 μM) with NADH (240 μM) in 0.5% DMSO/99.5% PBS (v/v). (d) Detection of H2O2 generation after irradiation of various time intervals (light source: 525 nm, 50.2 J cm−2) for the reaction of complex Ir2 (2 μM) with NADH (260 μM) in 0.5% DMSO/99.5% PBS (v/v). (e) The concentration of NADH consumed vs. irradiation time plot for Ir2 (2 μM) upon 525 nm light (50.2 J cm−2) irradiation in 0.5% DMSO/99.5% PBS (v/v) solution. | |
Table 1 Comparison of the TON and TOF of Ir1 and Ir2 with other reported Ir(III) photo-catalysts for NADH photo-oxidation
Complexes |
TON |
TOF (h−1) |
CO6 = coumarin 6, ppy = 2-phenylpyridine, L1 = 4′-(3,5-di-tert-butylphenyl)-2,2′:6′,2′′-terpyridine, L2 = 4′-(3,5-bis(trifluoromethyl)phenyl)-2,2′:6′,2′′-terpyridine, L3 = 4-([2,2′:6′,2′′-terpyridin]-4′-yl)-N,N-dimethylaniline, piq = 1-phenylisoquinoline, Cpxbiph = biphenyltetramethylcyclopentadien-yl, Ce6 = chlorin e6. TON and TOFs of our complexes for the photocatalytic oxidation of NADH (240 μM) by complexes Ir1 and Ir2 (2 μM each) in 0.5% DMSO and 99.5% PBS solution, light source: 525 nm, 50.2 J cm−2. TON and TOFs for photocatalytic NADH oxidation (120 μM) by complex (3 μM) in 0.5% DMSO and 99.5% PBS, light source: 463 nm, 8.9 J cm−2 (taken from ref. 23). TON and TOFs for photocatalytic oxidation of NADH by complexes (1 μM) in PBS, light source: 525 nm, 29.56 J cm−2 (taken from ref. 28). TON and TOFs for photocatalytic oxidation of NADH (160 μM) by complexes (5 μM each) in PBS, light source: 465 nm, 1.2 J cm−2 (taken from ref. 27). TON and TOFs for photocatalytic oxidation of NADH (160 μM) by complexes (1 μM each) in PBS, light source: 465 nm, 29.56 J cm−2 (taken from ref. 25). TON and TOFs for photocatalytic oxidation of NADH (100 μM) by 11 (5 μM each) in water, light source: 635 nm, 145 mW cm−2 (taken from ref. 24). |
[Ir(CO6)2(dppz)]Cl (Ir1)a |
28.046 |
841.39 |
[Ir(CO6)2(aip)]Cl (Ir2)a |
66.9 |
1003.5 |
[Ir(ttpy)(pq)Cl]PF6 (1)b |
16.8 |
33.6 |
[Ir(tpy)(ppy)Cl]PF6 (2)c |
21.1 |
126.4 |
[Ir(tpy)(ppy)Cl]2(PF6)2 (3)c |
122.51 |
735.1 |
[Ir(ppy)2(N,N-Glc)]Cl (4)d |
8.4 |
16.9 |
[Ir(piq)2(N,N-Glc)]Cl (5)d |
21.4 |
42.8 |
[Ir(CO6)2(N,N-Glc)]Cl (6)d |
207.1 |
414.2 |
[Ir(CO6)2(L1)]Cl (7)e |
183.0 |
1097.8 |
[Ir(CO6)2(L2)]Cl (8)e |
176.7 |
1060.4 |
[Ir(CO6)2(L3)]Cl (9)e |
226.2 |
1357.2 |
[Ir(ppy)2(L2)]Cl (10)e |
11.9 |
71.3 |
[(η5-Cpxbiph)Ir(N^N-NDI)Cl]PF6 (11)f |
18 |
270.6 |
Ce6d |
24.5 |
49 |
2.4 Singlet oxygen generation
Ir(III) photocatalysts are known to generate singlet oxygen (1O2) on exposure to light via the type II mechanism (by transferring energy).23–321O2 generation tendency of complexes Ir1 and Ir2 were studied by monitoring the change in absorbance of the singlet oxygen sensor, 1,3-diphenylisobenzofuran (DPBF),64–68 under exposure to green light (Fig. 5a and b). In the presence of 1O2, DPBF is converted to DPBF-O2, resulting in a decrease in the absorbance of DPBF.64–68 The decrease in the absorbance of DPBF in the presence of Ir1 and Ir2 under green light irradiation clearly indicated their 1O2 generation tendency (Fig. 5a and b). The singlet oxygen generation tendency of complexes increased with an increase in the irradiation time, as was evident from the change in the absorption vs. irradiation time plot (Fig. 5c). Furthermore, the quantum yield of complexes Ir1 and Ir2 for 1O2 generation was 0.24 and 0.26, which are higher than that of the control complex [Ru(bpy)3]Cl2 (0.22 in 0.5% DMSO and 99.5% PBS, v/v) (Fig. S18, ESI†). This observation suggests that the complexes could act as good photosensitizers for PDT applications. Furthermore, the tendency of the complexes to generate the hydroxyl radical (˙OH) was examined by the methylene blue probe under green light exposure (525 nm, 50.2 J cm−2).31b When the methylene blue + Ir1/Ir2 in a solution of 0.5% DMSO + 99.5% PBS was exposed to light, the methylene blue-based absorbance between 650–700 nm decreased with time (Fig. S19, ESI†), indicating the ˙OH generation tendency of the complexes. Thus, Ir1 and Ir2 would show photocytotoxicity via type I (NADH oxidation, H2O2, and ˙OH generation) and type-II (1O2 production) pathways. This is a novel observation as most of the Ir(III) or Ru(II) based photosensitizers have been reported to show anticancer activity via the type-II pathway.10,13,18,42d
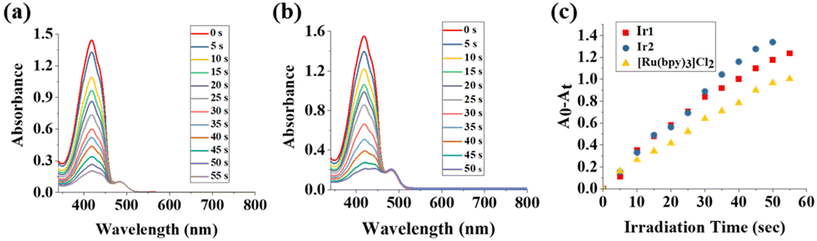 |
| Fig. 5 (a) and (b) represent 1O2 generation by complexes Ir1 and Ir2 (2 μM each) in a solution containing 0.5% DMSO/99.5% PBS (v/v) solution as was detected with DPBF (50 μM) and monitored with UV-Vis spectroscopy upon irradiation with 525 nm light (50.2 J cm−2) at different time intervals. (c) Change in absorbance vs. irradiation time plot for Ir1, Ir2, and reference [Ru(bpy)3]Cl2 (2 μM each) upon 525 nm light (50.2 J cm−2) irradiation in 0.5% DMSO/99.5% PBS (v/v) solution. | |
2.5 Photo-cytotoxicity
The excellent ROS generation and photocatalytic (NADH oxidation) activities of Ir1 and Ir2 prompted us to investigate the anticancer potential of complexes against various cancer cell lines after light exposure. The cytotoxicity of cisplatin against human bronchial epithelial BEAS-2B cells (Fig. S20, ESI†) and of complexes towards A549 (Fig. S21, ESI†) and HeLa (Fig. S22, ESI†) cancer cells and normal human bronchial epithelial BEAS-2B cells (Fig. S23, ESI†) in the dark as well as under light irradiation (10 J cm−2, wavelength 400–700 nm) were evaluated by MTT assay.28 The IC50 values in the dark and light under normoxia are presented in Table 2. As per the results, complexes Ir1 and Ir2 showed significantly higher toxicity in A549 and HeLa cancer cell lines as compared to normal human bronchial epithelial BEAS-2B cells under dark as well as light conditions. Furthermore, the light toxicity of complexes Ir1 and Ir2 in the above cell lines was significantly higher than that of their dark toxicity. Specifically, under light irradiation, complexes Ir1 and Ir2 showed remarkable cancer cell killing abilities with extremely low micromolar IC50 values (Table 2). Complex Ir2 demonstrated the highest cytotoxicity with IC50 < 1 μM against both cancer cell lines (Table 2). PIs (dark IC50/light IC50) of these complexes were also in the range of >28–71. Altogether, the above results confirmed the phototoxic nature of complexes Ir1 and Ir2 and their selectivity (SI > 7–19) towards cancer cell lines (Fig. S21 and S22, ESI†). Additionally, complex Ir2 exhibited comparable phototoxicity against A549 cancer cells to the previously reported Ir(III)-based photocatalyst 4 (Table 2).28 Complex Ir2 also demonstrated significantly higher phototoxicity than other previously reported Ir(III)-based photocatalysts, such as 1, 5, and 13 (Table 2).23,26,27 The IC50 value of complex Ir2 against A549 cancer cells under light irradiation was significantly lower than that of the clinically used photosensitizer 5-ALA,23 suggesting that complex Ir2 has the potential to be a more effective photosensitizer for in vivo applications. The sulforhodamine B (SRB) assay was also performed against A549 cells to validate the MTT assay results (Fig. S24, ESI†). Ir1 exhibited IC50 values of 45.9 ± 3.2 μM in the dark and 0.9 ± 0.3 μM under light (Table S3, ESI†). Ir2 showed IC50 values of 42.1 ± 2.7 μM in the dark and 0.7 ± 0.1 μM under light (Table S3, ESI†). Interesting to note that a similar result was also obtained by MTT assay. Since complex Ir2 was more active than Ir1, all further mechanistic studies were performed with only complex Ir2. It is well established that complexes that show NADH oxidation can also show anticancer activity in hypoxia.23,25 Therefore, we explored the photoinduced anticancer activity of complexes Ir1 and Ir2 against A549 cancerous cells under hypoxic conditions (Fig. S25, ESI†). Again, Ir2 demonstrated the highest photocytotoxicity (IC50 = 1.7 ± 0.1 μM) than complex Ir1 (IC50 = 3.3 ± 0.1 μM) (Fig. S25, Table S4, ESI†). The IC50 values obtained under dark and light conditions are presented in Table S4.† The photocytotoxicity of complexes in hypoxia is almost 4 times lower than that of normoxia. The reason behind this is the decrease in 1O2 generation due to the very low availability of oxygen, and the achieved activity is due to oxygen-independent photo-oxidation of NADH.69
Table 2 IC50 (μM) values of Ir1 and Ir2 and some selected Ir(III)-based photo-catalysts under normoxia
Complex |
A549 |
HeLa |
Beas 2B |
Dark |
Light |
PI |
SI |
Dark |
Light |
PI |
SI |
Dark |
Light |
White light irradiation (400–700 nm, 10 J cm−2). Light treatment: incubation time: 4 h, total irradiation = 10 J cm−2 over 1 h. Recovery time: 43 h. Dark treatment: incubation time: 4 h, recovery time: 44 h.
Blue light irradiation (465 nm, 8.9 J cm−2). Light treatment: incubation time: 2 h, total irradiation = 8.9 J cm−2 over 30 min. Recovery time: 46 h. Dark treatment: incubation time: 2 h, recovery time: 46 h (from ref. 23).
Blue light irradiation (465 nm, 11.7 J cm−2). Light treatment: incubation time: 16 h, total irradiation = 11.7 J cm−2 over 5 min. Recovery time: 32 h. Dark treatment: incubation time: 16 h, recovery time: 32 h (from ref. 27).
White light irradiation (400–700 nm, 17.2 J cm−2). Light treatment: incubation time: 4 h, light irradiation = 17.2 J cm−2. Recovery time: 44 h. Dark treatment: incubation time: 4 h, recovery time: 44 h (from ref. 26).
Blue light irradiation (465 nm, 8.9 J cm−2). Light treatment: incubation time: 4 h, light irradiation = 8.9 J cm−2. Recovery time: 44 h. Dark treatment: incubation time: 4 h, recovery time: 44 h (from ref. 23).
Blue light irradiation (465 nm, 8.9 J cm−2). Light treatment: incubation time: 4 h, light irradiation = 8.9 J cm−2. Recovery time: 46 h. Dark treatment: incubation time: 4 h, recovery time: 46 h (from ref. 23).
|
Ir1 a |
43.2 ± 1.7 |
0.9 ± 0.1 |
44.1 |
11.66 |
44.4 ± 2.1 |
1.6 ± 0.1 |
27.8 |
7.14 |
>50 |
11.4 ± 1.3 |
Ir2 a |
41.2 ± 1.5 |
0.6 ± 0.1 |
71.0 |
18.02 |
43.7 ± 1.8 |
0.9 ± 0.1 |
47.0 |
11.2 |
>50 |
10.4 ± 1.8 |
1 b |
43.6 ± 2.5 |
1.6 ± 0.1 |
71.0 |
|
35.4 ± 1.9 |
1.9 ± 0.3 |
18.6 |
|
|
|
4 c |
>100 |
18.6 ± 0.2 |
>5.4 |
|
|
|
|
|
|
|
5 c |
37.5 ± 1.2 |
0.9 ± 0.03 |
41.7 |
|
|
|
|
|
|
|
6 c |
55.0 ± 1.3 |
0.2 ± 0.01 |
275 |
|
|
|
|
|
|
|
[Ir(tpy)2](PF6)3 (12)d |
>100 |
>100 |
NA |
|
|
|
|
|
|
|
[Ir(Me2N-ph-tpy)(4′-ph-tpy)](PF6)3 (13)d |
98.6 ± 4.8 |
49.3 ± 0.74 |
2 |
|
|
|
|
|
|
|
5-ALAe |
>10 000 |
>10 000 |
NA |
NA |
>10 000 |
2510 ± 280 |
>4.0 |
NA |
NA |
NA |
cis-Platinf |
8.3 ± 1.2 |
8.6 ± 0.9 |
NA |
2.72 |
7.1 ± 0.8 |
6.0 ± 0.5 |
1.19 |
3.9 |
20.4 ± 1.6 |
23.4 ± 2.4 |
2.6 Cellular localization
Cellular uptake and localization of the photosensitizer are crucial to understand their anticancer mechanism.70–72 Complex Ir2 exhibited a green fluorescence due to the presence of the coumarin 6 moiety. This fluorescence property prompted us to explore the cellular uptake and localization by confocal imaging.25 The Hoechst dye and Mitotracker Red (MTR) dye were used to probe the cell nucleus and mitochondria, respectively.73–76 Notably, A549 cells treated with complex Ir2 showed green fluorescence, as represented by the complex Ir2 panel of Fig. 6. This confirmed the cellular uptake of Ir2 within 4 h. The merged panel of the Hoechst dye and Ir2 in Fig. 6 and S26, ESI† indicated the cytoplasmic localization of complex Ir2. Moreover, the merged panel of the MTR dye and Ir2 revealed that complex Ir2 primarily accumulated within the mitochondria (PCC value = 0.68) of A549 cells (as depicted in Fig. 6). Mitochondria play an essential role in cellular energy generation and are crucial for cell survival.73–76 The mitochondrial ETC is closely associated with the ATP generation process, and NADH is an important and essential constituent of the mitochondrial ETC.77 We have shown the high efficiency of complex Ir2 in catalytic NADH photo-oxidation (vide supra). Thus, complex Ir2 can oxidize NADH in mitochondria on light exposure like other reported Ir(III)-based photo-catalysts (e.g.1, 2, 3, 6, 7etc.) to create metabolic disorder.23,25,27,28 Moreover, the localization of complex Ir2 within the mitochondria serves to combat cis-platin resistance. By disrupting mitochondrial redox homeostasis through light-activated generation of singlet oxygen (1O2) and oxidation of NADH, complex Ir2 facilitates apoptosis induction in cells, thereby overcoming resistance to cis-platin.23–29
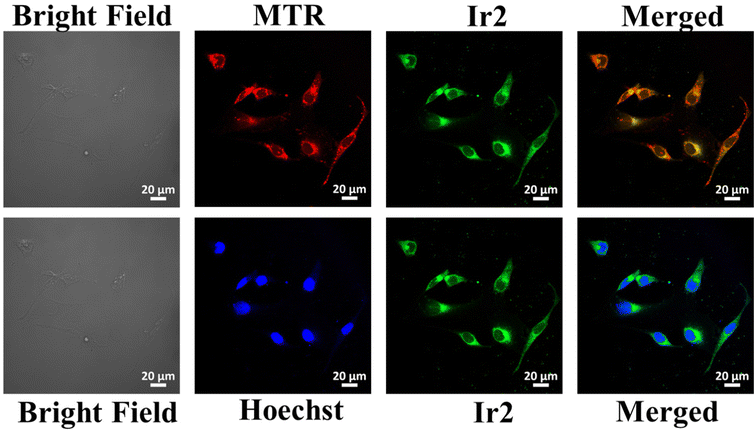 |
| Fig. 6 Cellular localization study of complex Ir2 in A549 cancer cells. A549 cells were incubated with 30 μM complex Ir2 for 4 h, followed by co-staining with the Hoechst dye (5 μg ml−1) or the MitoTracker dye (250 nM). The merged panel of the MitoTracker dye and complex Ir2 (MTR + Ir2) clearly indicates the mitochondrial localization of complex Ir2. Under the following conditions, confocal images were recorded: blue emission of the nucleus staining dye Hoechst (λex ∼ 405 nm, λem ∼ 460–475 nm), red emission of MTR (λex ∼ 630 nm, λem ∼ 650–665 nm), green emission of Ir2 (λex ∼ 488 nm, λem ∼ 500–520 nm). Scale bar: 20 μm. | |
2.7 In-cell NADH oxidation
As discussed previously, complex Ir2 facilitated the photooxidations of NADH under a cell-free system. In order to validate this observation under cellular conditions, the effect of complex Ir2 (25 μM) treatment on the levels of NAD+ and NADH was monitored in A549 cells under dark and light irradiation conditions. The results indicated that under dark conditions, complex Ir2 treatment did not cause any significant change in the ratio of NAD+ and NADH as compared to the untreated control group (Fig. 7a). The only light irradiation also did not show any significant change in the ratio of NAD+ and NADH as compared to the untreated dark control group (Fig. 7a). However, complex Ir2 treatment in the presence of light led to a significantly higher ratio of NAD+ and NADH as compared to the untreated light control group (Fig. 7a). Thus, it clearly suggests the ability of complex Ir2 to photo-oxidize NADH within the cells. It is important to discuss that researchers have mostly reported NADH photo-oxidation in solutions and have correlated in-solution NADH oxidation efficiency with anticancer activity.24–31 To the best of our knowledge, this is the 2nd time (after ref. 23) that NADH photo-oxidation in cells has been confirmed with any photocatalyst.
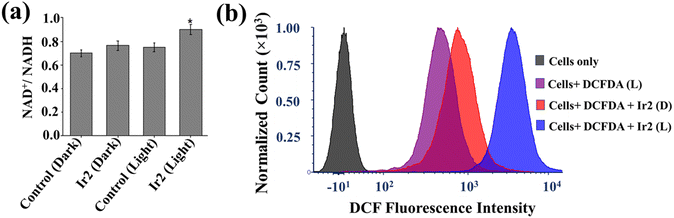 |
| Fig. 7 (a) The plot shows the ratio of NAD+ and NADH in A549 cells treated with complex Ir2 (25 μM) through spectrophotometric analysis. One set of cells was exposed to visible light (λ = 400–700 nm, 10 J cm−2) after 4 h of incubation with the complex, while the other set was kept continuously in the dark. The total incubation period following the addition of the complex was 24 h. The results are presented as mean ± SEM. *p < 0.05 as compared to the dark and light control group by student T test. (b) Intracellular generation of reactive oxygen species (ROS) in A549 cells induced by Ir2 (1 μM) using the DCFDA probe. | |
2.8 In-cell ROS generation
In an aqueous solution, in the absence of NADH, complex Ir2 produced 1O2 on light exposure via a type II pathway, whereas in the presence of NADH + Light, Ir2 also produced H2O2 (Fig. 4c and d) via a type-I pathway. Thus, this complex can show PDT activity through a combined pathway of type I + type II and ultimately could produce excessive ROS in cells.23–32 Therefore, the intracellular ROS generation tendency of complex Ir2 was examined by DCFDA assay.78,79 As depicted in Fig. 7b, when A549 cells were treated with Ir2 in the absence of light, there was a 1.6-fold increase in the DCF intensity. However, there was a 12-fold enhancement in the intensity of DCF in the presence of light. This indicates the light-induced ROS generation potential of complex Ir2 within the A549 cancerous cells. As complex Ir2 was mainly located within the cell mitochondria, therefore, we hypothesized that it might induce oxidative stress in mitochondria, leading to mitochondrial depolarization followed by cell apoptosis.25
2.9 Change in the mitochondrial membrane potential and cellular apoptosis
Complex Ir2, localized in the mitochondria and producing ROS upon exposure to visible light, can depolarize mitochondria after light irradiation. The JC-1 assay was used to explore the effects of complex Ir2 on the mitochondrial membrane potential.80 The JC-1 assay is well known to detect any change in the mitochondria membrane potential.80 This dye concentrates in normally functioning mitochondria and forms red aggregates, whereas in leaky mitochondria with compromised membranes, it is released into the cytosol and remains in a green monomeric state.80 When A549 cells were exposed to complex Ir2 under dark conditions, they exhibited red fluorescence (Fig. 8a) due to JC-1 in its aggregate form, suggesting intactness of the mitochondrial membrane potential and low/negligible dark toxicity of Ir2. Whereas, after light exposure, these cells showed a green fluorescence signal (Fig. 8a), suggesting the conversion of JC-1 into its monomeric form and hence mitochondrial depolarization. The positive control carbonyl cyanide m-chlorophenylhydrazone (CCCP) also produced a similar response (Fig. 8a).
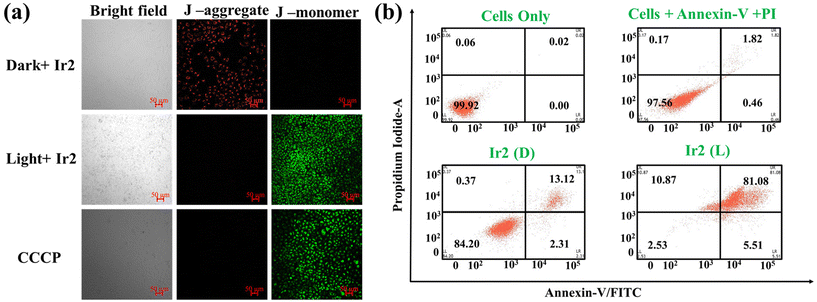 |
| Fig. 8 (a) Change in the mitochondrial membrane potential by complex Ir2 (1 μM) treated A549 cells as monitored by JC-1 assay, scale bar: 50 μm. Dark + Ir2 = A549 cells + Ir2, light + Ir2 = A549 cells + Ir2 + light. CCCP was used as the positive control. (b) Apoptotic cell death of A549 induced by Ir2 (1 μM) under light irradiation (400–700 nm, 10 J cm−2) by annexin V-FITC/propidium iodide (PI) assay. Scale bar: 50 μm. | |
Mitochondrial depolarization is widely recognized as a hallmark of cell apoptosis.80 To identify the type of cell death induced by Ir2 + light, annexin V-FITC/propidium iodide (PI) assay was carried out by FACS analysis (Fig. 8b).80 The annexin V-FITC/PI assay can precisely differentiate cell populations into various cell death types.80 When A549 cells were treated with Ir2 (1 μM) in the absence of light, only ca. 15% apoptotic (Annexin V+ve PI−ve and Annexin V+ve PI+ve) cells were found. The percentage of apoptotic (Annexin V+ve PI−ve and Annexin V+ve PI+ve) cells was increased to 87% after light exposure.
Furthermore, the apoptotic cell death pathway is mediated through the final effector protein caspase 3. Therefore, the activity of caspase 3 was monitored by treating A549 cells with complex Ir2 (25 μM) under dark and light exposure conditions (Fig. S27, ESI†). The time point for the estimation of caspase activity was 48 h after the desired treatments. The result indicated that treatment of complex Ir2 under dark conditions led to only a marginal increase in caspase 3 activity as compared to control cells. However, similar treatment on light exposure resulted in a significantly higher increase in caspase activity than under dark conditions. These findings thus clearly indicate complex Ir2 induced cellular apoptosis via ROS-triggered mitochondrial depolarization.
3. Conclusion
Herein, we have successfully synthesized and characterized two novel Ir(III)-based photocatalysts. The light-induced anticancer potential of complexes was enhanced by extending the conjugation within the ligand (e.g., dppz vs. aip). The absorption spectra of complexes Ir1 and Ir2 showed a coumarin 6-based sharp band between 450–550 nm and a MLCT band between 400–450 nm, which were used to achieve green light-induced catalytic NADH oxidation and visible light-induced anticancer activity. Ir2 turned out to be a highly efficient photocatalyst for NADH oxidation in an aqueous solution with a turnover number of ca. 66 and a TOF of ca. 1000 h−1, while Ir1 had a TON of ca. 28 and a TOF of ca. 841 h−1. Thus, extended conjugation in Ir2 increases the electron-accepting capability of Ir2 compared to Ir1. The catalytic activity of complex Ir2 was much higher than the previously reported Ir(III) photocatalysts, 1, 2, 4, 5, and 10.23,25,27,28 The TOF of Ir2 was similar to the highest achieved TOF with previously reported blue light-activated Ir(III)-based photo-catalysts for NADH oxidation.25 Most of the research based on photocatalytic therapy showed in solution NADH oxidation and correlated their efficiency with anticancer activity.24–31 This is the 2nd time in-cell NADH photo-oxidation was achieved with a catalyst, validating the concept of photocatalytic cancer therapy. The cellular localization study of the green fluorescent complex Ir2 in A549 cancer cells showed that Ir2 primarily accumulated within the mitochondria. Ir1 and Ir2 showed significantly higher toxicity in A549 and HeLa cancer cells than normal human bronchial epithelial BEAS-2B cells under light conditions. Complexes Ir1 and Ir2 demonstrated remarkably low micromolar photo-cytotoxicities against cancer cells in both normoxia and hypoxia. PI values (dark IC50/light IC50) of these complexes were also very high, in the range of >28–71. Ir2 had a bit higher phototoxicity than Ir1 which could be due to its more extended conjugation. The higher extended π-conjugation in Ir2 might enhance its lipophilicity, intracellular uptake, and photosensitivity.25,26,28,30–32 The apoptotic photo-activated anticancer activity of these complexes in normoxia mainly arises from their ability to generate ROS and oxidize NADH in-cell on light exposure, activating caspase 3. So far, in photocatalytic cancer therapy under normoxia, complexes have been reported to show anticancer activity through the combined effects of NADH oxidation and 1O2 generation. But here, Ir1 and Ir2 not only generated 1O2 and oxidized NADH, but also generated ˙OH radicals.
Author contributions
S. B. and A. K. Y. designed the studies and formulated the concept and overall project. A. K. Y., R. K., A. A. M., and S. A. synthesized and characterized the complexes. A. K. Y. did all the in-solution chemistry. A. K. did caspase 3 activation and in-cell NADH oxidation. A. U. and A. B. performed the HPLC studies and biological assays. R. K. and A. K. Y. performed the DFT and TD-DFT calculations. The manuscript was written through the contributions of all authors. All authors have approved the final version of the manuscript.
Data availability
The authors confirm that the data supporting the findings of this study are available within the article and its ESI.† The raw data supporting this study's findings are available from the corresponding authors upon reasonable request.
Conflicts of interest
The authors declare no conflict of interest.
Acknowledgements
We thank BRNS (54/14/08/2022-BRNS-R), the Government of India, for financial support. A. K. Y., R. K., and A. A. M. thank the Ministry of Education, the Government of India, for the Prime Minister's Research Fellowship. We sincerely thank Ashish Kumar Maurya for his support during this work.
References
- L. Kelland, The resurgence of platinum-based cancer chemotherapy, Nat. Rev. Cancer, 2007, 7, 573–584 CrossRef CAS PubMed
.
- K. D. Mjos and C. Orvig, Metallodrugs in Medicinal Inorganic Chemistry, Chem. Rev., 2014, 114, 4540–4563 CrossRef CAS
.
- S. Thota, D. A. Rodrigues, D. C. Crans and E. J. Barreiro, Ru(II) compounds: next-generation anticancer metallotherapeutics?, J. Med. Chem., 2018, 61, 5805–5821 CrossRef CAS
.
- S. Gadre, M. Manikandan, P. Duari, S. Chhatar, A. Sharma, S. Khatri, J. Kode, M. Barkume, N. K. Kasinathan, M. Nagare, M. Patkar, A. Ingle, M. Kumar, U. Kolthur-Seetharam and M. Patra, A rationally designed bimetallic platinum(II)-ferrocene antitumor agent Induces non-apoptotic cell death and exerts in vivo efficacy, Chem. – Eur. J., 2022, 28, e202201259 CrossRef CAS
.
- R. J. Mitchell, A. S. Gowda, A. G. Olivelli, A. J. Huckaba, S. Parkin, J. M. Unrine, V. Oza, J. S. Blackburn, F. Ladipo, D. K. Heidary and E. C. Glazer, Triarylphosphine-coordinated bipyridyl Ru(II) complexes Induce mitochondrial dysfunction, Inorg. Chem., 2023, 62, 10940–10954 CrossRef CAS
.
- Z. Tian, Y. Yang, L. Guo, G. Zhong, J. Li and Z. Liu, Dual-functional cyclometalated iridium imine NHC complexes: highly potent anticancer and antimetastatic agents, Inorg. Chem. Front., 2018, 5, 3106–3112 RSC
.
- M. V. Babak, Y. Zhi, B. Czarny, T. B. Toh, L. Hooi, E. K. H. Chow, W. H. Ang, D. Gibson and G. Pastorin, Dual-Targeting dual-action platinum(IV) platform for enhanced anticancer activity and reduced nephrotoxicity, Angew. Chem., Int. Ed., 2019, 58, 8109 CrossRef CAS PubMed
.
- Z. Liu and P. J. Sadler, Organoiridium Complexes: anticancer agents and catalysts, Acc. Chem. Res., 2014, 47, 1174–1185 CrossRef CAS PubMed
.
- H. Sung, J. Ferlay, R. L. Siegel, M. Laversanne, I. Soerjomataram, A. Jemal and F. Bray, global cancer statistics 2020: GLOBOCAN Estimates of Incidence and Mortality Worldwide for 36 Cancers in 185 Countries, CA Cancer J. Clin., 2021, 71, 209–249 CrossRef
.
- J. Karges, S. Kuang, F. Maschietto, O. Blacque, I. Ciofini, H. Chao and G. Gasser, Rationally designed ruthenium complexes for 1- and 2-photon photodynamic therapy, Nat. Commun., 2020, 11, 3262 CrossRef CAS
.
- J. Liu, C. Zhang, T. W. Rees, L. Ke, L. Ji and H. Chao, Harnessing ruthenium(II) as photodynamic agents: encouraging advances in cancer therapy, Coord. Chem. Rev., 2018, 363, 17–28 CrossRef CAS
.
- H. Zhou, D. Tang, Y. Yu, L. Zhang, B. Wang, J. Karges and H. Xiao, Theranostic imaging and multimodal photodynamic therapy and immunotherapy using the mTOR signaling pathway, Nat. Commun., 2023, 14, 5350 CrossRef CAS
.
- R. T. Ryan, K. C. Stevens, R. Calabro, S. Parkin, J. Mahmoud, D. Y. Kim, D. K. Heidary, E. C. Glazer and J. P. Selegue, Bis-tridentate N-Heterocyclic carbene Ru(II) complexes are promising new agents for photodynamic therapy, Inorg. Chem., 2020, 59, 8882–8892 CrossRef CAS
.
- A. Chettri, T. Yang, H. D. Cole, G. Shi, C. G. Cameron, S. A. McFarland and B. Dietzek-Ivanšić, Using biological photophysics to map the excited-state topology of molecular photosensitizers for photodynamic therapy, Angew. Chem., Int. Ed., 2023, 62, e202301452 CrossRef CAS
.
- A. S. Sharma, P. Sudhindra, N. Roy and P. Paira, Advances in novel iridium(III) based complexes for anticancer applications: A review, Inorg. Chim. Acta, 2020, 513, 119925 CrossRef
.
- H. D. Cole, J. A. Roque III, G. Shi, L. M. Lifshits, E. Ramasamy, P. C. Barrett, R. O. Hodges, C. G. Cameron and S. A. McFarland, Anticancer agent with inexplicable potency in extreme hypoxia: characterizing a light-triggered ruthenium ubertoxin, J. Am. Chem. Soc., 2022, 144, 9543–9547 CrossRef CAS PubMed
.
- Y. Zhang, B. T. Doan and G. Gasser, Metal-based photosensitizers as inducers of regulated cell death mechanisms, Chem. Rev., 2023, 123, 10135–10155 CrossRef CAS
.
- L. Wang, J. Karges, F. Wei, L. Xie, Z. Chen, G. Gasser, L. Ji and H. Chao, Mitochondria-localized iridium(III) photosensitizer for two-photon photodynamic immunotherapy against melanoma, Chem. Sci., 2023, 14, 1461–1471 RSC
.
- F. Heinemann, J. Karges and G. Gasser, Critical Overview of the use of Ru(II) polypyridyl complexes as photosensitizers in one-photon and two-photon photodynamic therapy, Acc. Chem. Res., 2017, 50, 2727–2736 CrossRef CAS PubMed
.
- L. Xie, L. Shi, K. Xiong, R. Guan, Y. Chen, J. Long, L. Ji and H. Chao, Synthesis, subcellular localization and anticancer mechanism studies of unsymmetrical Iridium(III) complexes, Eur. J. Inorg. Chem., 2023, 26, e202300001 CrossRef CAS
.
- R. Kushwaha, A. Kumar, S. Saha, S. Bajpai, A. K. Yadav and S. Banerjee, Os(II) complexes for catalytic anticancer therapy: recent update, Chem. Commun., 2022, 58, 4825–4836 RSC
.
- H. Huang, S. Banerjee and P. J. Sadler, Recent advances in the design of targeted Iridium(III), photosensitizers for photodynamic therapy, ChemBioChem, 2018, 19, 1574 CrossRef CAS PubMed
.
- H. Huang, S. Banerjee, K. Qiu, P. Zhang, O. Blacque, T. Malcomson, M. J. Paterson, G. J. Clarkson, M. Staniforth, V. G. Stavros, G. Gasser, H. Chao and P. J. Sadler, Targeted photoredox catalysis in cancer cells, Nat. Chem., 2019, 11, 1041–1048 CrossRef CAS
.
- Y. Yang, Y. Gao, J. Zhao and S. Gou, An electron-accepting half-sandwich iridium(III) complex for the treatment of hypoxic tumors via synergetic chemo- and phototherapy, Inorg. Chem. Front., 2024, 11, 436–450 RSC
.
- Z. Fan, J. Xie, T. Sadhukhan, C. Liang, C. Huang, W. Li, T. Li, P. Zhang, S. Banerjee, K. Raghavachari and H. Huang, Highly efficient Ir(III)–Coumarin photo–redox catalyst for synergetic multi–mode cancer photo–therapy, Chem. – Eur. J., 2022, 28, e202103346 CrossRef CAS
.
- L. Wei, R. Kushwaha, A. Dao, Z. Fan, S. Banerjee and H. Huang, Axisymmetric bis-tridentate Ir(III) photoredox catalysts for anticancer phototherapy under hypoxia, Chem. Commun., 2023, 59, 3083–3086 RSC
.
- Z. Zhu, L. Wei, Y. Lai, W. L. O. Carter, S. Banerjee, P. J. Sadler and H. Huang, Photocatalytic glucose-appended bio-compatible Ir(III) anticancer complexes, Dalton Trans., 2022, 51, 10875–10879 RSC
.
- Z. Fan, Y. Rong, T. Sadhukhan, S. Liang, W. Li, Z. Yuan, Z. Zhu, S. Guo, S. Ji, J. Wang, R. Kushwaha, S. Banerjee, K. Raghavachari and H. Huang, Single-cell quantification of a highly biocompatible dinuclear Iridium(III) complex for photocatalytic cancer therapy, Angew. Chem., Int. Ed., 2022, 61, e202202098 CrossRef CAS PubMed
.
- J. Kasparkova, G. A. Hernández, H. Kostrhunova, M. Goicuría, V. Novohradsky, D. Bautista, L. Markova, M. D. Santana, V. Brabec and J. Ruiz, Novel 2-(5-Arylthiophen-2-yl)-benzoazole cyclometalated Ir(III) dppz complexes exhibit selective phototoxicity in cancer cells by lysosomal damage and oncosis, J. Med. Chem., 2024, 11, 691–708 CrossRef PubMed
.
- S. Wei, H. Liang, A. Dao, Y. Xie, F. Cao, Q. Ren, A. K. Yadav, R. Kushwaha, A. A. Mandal, S. Banerjee, P. Zhang, S. Ji and H. Huang, Perturbing tumor cell metabolism with a Ru(II) photo-redox catalyst to reverse the multidrug resistance of lung cancer, Sci. China Chem., 2023, 66, 1482–1488 CrossRef CAS
.
-
(a) Z. Fan, J. Xie, R. Kushwaha, S. Liang, W. Li, A. A. Mandal, L. Wei, S. Banerjee and H. Huang, Anticancer screening of Ru(II) photoredox catalysts at single cancer cell level, Chem. – Asian J., 2023, 18, e202300047 CrossRef CAS PubMed
;
(b) A. A. Mandal, V. Singh, S. Saha, S. Peters, T. Sadhukhan, R. Kushwaha, A. K. Yadav, A. Mandal, A. Upadhyay, A. Bera, A. Dutta, B. Koch and S. Banerjee, Green Light-Triggered Photocatalytic Anticancer Activity of Terpyridine-Based Ru(II) Photocatalysts, Inorg. Chem., 2024, 63, 7493–7503 CrossRef CAS
.
- C. Ge, J. Zhu, A. Ouyang, N. Lu, Y. Wang, Q. Zhang and P. Zhang, Near-infrared phosphorescent terpyridine osmium(II) photosensitizer complexes for photodynamic and photooxidation therapy, Inorg. Chem. Front., 2020, 7, 4020–4027 RSC
.
- R. Bevernaegie, B. Doi, E. Bastien, A. Diman, A. Decottignies, O. Feron and B. Elias, Exploring the photo-toxicity of hypoxic active iridium(III)-based sensitizers in 3D tumor spheroids, J. Am. Chem. Soc., 2019, 141, 18486–18491 CrossRef CAS PubMed
.
- Q. Yao, J. Fan, S. Long, X. Zhao, H. Li, J. Du, K. Shao and X. Peng, The Concept and Examples of Type-III Photosensitizers for Cancer Photodynamic Therapy, Chem, 2022, 8, 197–209 CAS
.
- A. M. D. P. Bayona, P. Mroz, C. Thunshelle and M. R. Hamblin, Design features for optimization of tetrapyrrole macrocycles as antimicrobial and anticancer photosensitizers, Chem. Biol. Drug Des., 2017, 89, 192–206 CrossRef PubMed
.
- J. D. Knoll and C. Turro, Control and utilization of ruthenium and rhodium metal complex excited states for photoactivated cancer therapy, Coord. Chem. Rev., 2015, 282–283, 110–126 CrossRef CAS
.
- T. K. Horne and M. J. Cronje, Mechanistics and photo-energetics of macrocycles and photodynamic therapy: an overview of aspects to consider for research, Chem. Biol. Drug Des., 2017, 89, 221–242 CrossRef CAS
.
- Z. Zhu, L. Wei, A. K. Yadav, Z. Fan, A. Kumar, M. Miao, S. Banerjee and H. Huang, Cyanine-functionalized 2,2′-bipyridine compounds for photocatalytic cancer therapy, J. Org. Chem., 2023, 88, 626–631 CrossRef CAS
.
- D. V. Titov, V. Cracan, R. P. Goodman, J. Peng, Z. Grabarek and V. K. Mootha, Complementation of mitochondrial electron transport chain by manipulation of the NAD+/NADH ratio, Science, 2016, 352, 231–235 CrossRef CAS
.
- M. Li, K. H. Gebremedhin, D. Ma, Z. Pu, T. Xiong, Y. Xu, J. S. Kim and X. Peng, Conditionally activatable photoredox catalysis in living systems, J. Am. Chem. Soc., 2022, 144, 163–173 CrossRef CAS
.
- L. E. Navas and A. Carnero, NAD+ metabolism, stemness, the immune response, and cancer, Signal Transduction Targeted Ther., 2021, 6, 2 CrossRef CAS PubMed
.
-
(a) C. Li, Y. Cai, M. Pang, X. Zhou, X. Luo and Z. Xiao, A coumarin-appended cyclometalated iridium(III) complex for visible light driven photoelectrochemical bioanalysis, Biosens. Bioelectron., 2020, 147, 111779 CrossRef CAS PubMed
;
(b) C. Zhang, K. Qiu, C. Liu, H. Huang, T. W. Rees, L. Ji, Q. Zhang and H. Chao, Tracking mitochondrial dynamics during apoptosis with phosphorescent fluorinated iridium(III) complexes, Dalton Trans., 2018, 47, 12907–12913 RSC
;
(c) S. Shaikh, Y. Wang, F. ur Rehman, H. Jiang and X. Wang, Phosphorescent Ir(III) complexes as cellular staining agents for biomedical molecular imaging, Coord. Chem. Rev., 2020, 416, 213344 CrossRef CAS
;
(d) S. Liu, J. Han, W. Wang, Y. Chang, R. Wang, Z. Wang, G. Li, D. Zhu and M. R. Bryce, AIE-active Ir(III) complexes functionalised with a cationic Schiff base ligand: synthesis, photophysical properties and applications in photodynamic therapy, Dalton Trans., 2022, 51, 16119–16125 RSC
.
- A. Sinopoli, C. J. Wood, E. A. Gibson and P. I. P. Elliott, Hybrid Cyclometalated Iridium Coumarin Complex as a Sensitiser of Both n-and p-Type DSSCs, Eur. J. Inorg. Chem., 2016, 2016, 2887–2890 CrossRef CAS
.
- Y. Wu, G. D. Sutton, M. D. S. Halamicek, X. Xing, J. Bao and T. S. Teets, Cyclometalated Iridium-Coumarin Ratiometric Oxygen Sensors: Improved Signal Resolution and Tunable Dynamic Ranges, Chem. Sci., 2022, 13, 8804–8812 RSC
.
- Y. Lu, R. Conway-Kenny, J. Wang, X. Cui, J. Zhao and S. M. Draper, Exploiting coumarin-6 as ancillary ligands in 1,10-phenanthro-line Ir(III) complexes: generating triplet photosensitizers with high upconversion capabilities, Dalton Trans., 2018, 47, 8585–8589 RSC
.
- S. Y. Takizawa, R. Kano, N. Ikuta and S. Murata, An anionic iridium(III) complex as a visible-light absorbing photosensitizer, Dalton Trans., 2018, 47, 11041–11046 RSC
.
- P.-N. Lai, C. H. Brysacz, M. K. Alam, N. A. Ayoub, T. G. Gray, J. Bao and T. S. Teets, Highly efficient red-emitting bis-cyclometalated iridium complexes, J. Am. Chem. Soc., 2018, 140, 10198–10207 CrossRef CAS PubMed
.
- S. Takizawa, N. Ikuta, F. Zeng, S. Komaru, S. Sebata and S. Murata, Impact of substituents on excited-state and photo-sensitizing properties in cationic iridium(III) complexes with ligands of coumarin 6, Inorg. Chem., 2016, 55, 8723–8735 CrossRef CAS
.
- A. Kobayashi, E. Muramatsu, M. Yoshida and M. Kato, Two excited state collaboration of heteroleptic Ir(III)-coumarin complexes for H2 Evolution dye-sensitized photocatalysts, Energies, 2021, 14, 2425 CrossRef CAS
.
- S.-Y. Takizawa, C. Pérez-Bolívar, P. Anzenbacher and S. Murata, Cationic iridium complexes coordinated with coumarin dyes -sensitizers for visible-light-driven hydrogen generation, Eur. J. Inorg. Chem., 2012, 2012, 3975–3979 CrossRef CAS
.
- G. L. Nelson, C. T. Ronayne, L. N. Solano, S. K. Jonnalagadda, S. Jonnalagadda, T. J. Schumacher, Z. S. Gardner, H. Palle, C. Mani, J. Rumbley and V. R. Mereddy, Synthesis and biological evaluation of N, N-dialkylcarboxy coumarin-NO donor conjugates as potential anticancer agents, Bioorg. Med. Chem. Lett., 2021, 52, 126411 CrossRef PubMed
.
- V. Novohradsky, A. Rovira, C. Hally, A. Galindo, G. Vigueras, A. Gandioso, M. Svitelova, R. Bresolí-Obach, H. Kostrhunova, L. Markova, J. Kasparkova, S. Nonell, J. Ruiz, V. Brabec and V. Marchán, Towards Novel Photodynamic Anticancer Agents Generating Superoxide Anion Radicals: A cyclometalated IrIII complex conjugated to a far-red emitting coumarin, Angew. Chem., Int. Ed., 2019, 58, 6311–6315 CrossRef CAS PubMed
.
- R. R. Ye, C. P. Tan, L. N. Ji and Z. W. Mao, Coumarin-appended phosphorescent cyclometalated iridium(III) complexes as mitochondria-targeted theranostic anticancer agents, Dalton Trans., 2016, 54, 13042–13051 RSC
.
- C. Liu, X. Liu, X. Ge, Q. Wang, L. Zhang, W. Shang, Y. Zhang, X. A. Yuan, L. Tian, Z. Liu and J. You, Fluorescent iridium(III) coumarin-salicylaldehyde schiff base compounds as lysosome-targeted antitumor agents, Dalton Trans., 2020, 49, 5988–5998 RSC
.
- R. Xu, Y. Wu, Z. Liu, J. Liu and X. Liu, lysosomal targeted cyclometallic iridium(III) salicylaldehyde-coumarin Schiff Base complexes and anticancer application, Front. Chem., 2022, 10, 906954 CrossRef CAS
.
- A. Rovira, E. Ortega-Forte, C. Hally, M. Jordà-Redondo, D. Abad-Montero, G. Vigueras, J. I. Martínez, M. Bosch, S. Nonell, J. Ruiz and V. Marchán, Exploring sructure–activity relationships in photodynamic therapy anticancer agents based on Ir(III)-COUPYconjugates, J. Med. Chem., 2023, 66, 7849–7867 CrossRef CAS PubMed
.
- C. G. L. Nongpiur, C. Soh, D. F. Diengdoh, A. K. Verma, R. Gogoi, V. Banothu, W. Kaminsky and M. R. Kollipara, 3-acetyl-coumarin-substituted thiosemicarbazones and their ruthenium, rhodium and iridium metal complexes: An investigation of the antibacterial, antioxidant and cytotoxicity activities, J. Organomet. Chem., 2023, 998, 122788 CrossRef CAS
.
- C. G. L. Nongpiur, L. Dkhar, D. K. Tripathi, K. M. Poluri, W. Kaminsky and M. Kollipara, R. Half-sandwich platinum group metal complexes containing coumarin-N-acylhydrazone hybrid ligands: Synthesis and biological evaluation studies, Inorg. Chim. Acta, 2021, 525, 120459 CrossRef CAS
.
- J. Li, C. F. Zhang, S. H. Yang, W. C. Yang and G. F. Yang, A coumarin-based fluorescent probe for selective and sensitive detection of thiophenols and its application, Anal. Chem., 2014, 86, 3037–3042 CrossRef CAS
.
- C. Li, W. Lu, X. Zhou, M. Pang and X. Luo, visible-light driven photoelectrochemical platform based on the cyclometalated iridium-(III) complex with coumarin 6 for detection of MicroRNA, Anal. Chem., 2018, 90, 14239–14246 CrossRef CAS PubMed
.
- Y. Gregoriou, G. Gregoriou, A. Manoli, P. Papageorgis, B. M. Larney, D. Vangeli, S. McColman, V. Yilmaz, H. Hsu, M. Skubal, A. Ogirala, E. Athanasiou, D. T. Cramb, N. Dietis, K. Strati, G. Itskos, A. I. Constantinoub and C. Andreou, Photophysical and biological assessment of coumarin-6 loaded polymeric nanoparticles as a cancer imaging agent, Sens. Diagn., 2023, 2, 1277–1285 RSC
.
- C. G. Arya, R. Gondru, Y. Li and J. Banothu, Coumarin–benzimidazole hybrids: A review of developments in medicinal chemistry, Eur. J. Med. Chem., 2022, 227, 113921 CrossRef PubMed
.
-
(a) M. Negi, T. Dixit and V. Venkatesh, Ligand dictated photosensitization of Ir(III) dithiocarbamate complexes for photodynamic therapy, Inorg. Chem., 2023, 62, 20080–20095 CrossRef CAS PubMed
;
(b) A. K. Pal and A. Datta, First-principles design of heavy-atom-free singlet oxygen photosensitizers for photodynamic therapy, J. Chem. Phys., 2024, 160, 164720 CrossRef CAS PubMed
;
(c) G. Xu, C. Li, C. Chi, L. Wu, Y. Sun, J. Zhao, X. H. Xia and S. Gou, A supramolecular photosensitizer derived from an Arene-Ru(II) complex self-assembly for NIR activated photodynamic
and photothermal therapy, Nat. Commun., 2022, 13, 3064 CrossRef CAS PubMed
.
- B. Yuan, J. Liu, R. Guan, C. Jin, L. Ji and H. Chao, Endoplasmic eticulum Targeted Cyclometalated Iridium(III) Complexes as Efficient Photodynamic Therapy Photosensitizers, Dalton Trans., 2019, 48, 6408–6415 RSC
.
- L. Qiao, J. Liu, S. Kuang, X. Liao, J. Kou, L. Ji and H. Chao, A mitochondrion-targeted BODIPY-Ir(III) conjugate as a photoinduced ROS generator for the oxidative destruction of triple-negative breast cancer cells, Dalton Trans., 2021, 50, 14332–14341 RSC
.
- A. Bera, S. Gautam, S. Sahoo, A. K. Pal, P. Kondaiah and A. R. Chakravarty, Red light active Pt(IV)-BODIPY prodrug as a mitochondria and endoplasmic reticulum targeted chemo-PDT agent, RSC Med. Chem., 2022, 13, 1526–1539 RSC
.
- S. J. Chadwick, D. Salah, P. M. Livesey, M. Brust and M. Volk, Singlet oxygen generation by laser irradiation of gold nanoparticles, J. Phys. Chem. C, 2016, 120, 10647–10657 CrossRef CAS
.
- S. Shamjith, M. M. Joseph, V. P. Murali, G. S. Remya, J. B. Nair, C. H. Suresh and K. K. Maiti, NADH-depletion triggered energy shutting with cyclometalated iridium(III) complex enabled bimodal Luminescence-SERS sensing and photodynamic therapy, Biosens. Bioelectron., 2022, 204, 14087 CrossRef
.
- A. K. Yadav, V. Singh, R. Kushwaha, D. Dolui, R. Rai, P. Dhar, A. Dutta, B. Koch and S. Banerjee, Polypyridyl Co(II)–curcumin complexes as photo–activated anticancer and antibacterial agents, ChemBioChem, 2023, 24, e202300033 CrossRef CAS PubMed
.
- T. Feng, Z. Tang, J. Karges, J. Shen, C. Jin, Y. Chen, Y. Pan, Y. He, L. Ji and H. Chao, Exosome camouflaged coordination-assembled Iridium(III) photosensitizers for apoptosis-autophagy-ferroptosis induced combination therapy against melanoma, Biomaterials, 2023, 301, 122212 CrossRef CAS PubMed
.
- Y. Wang, P. Mesdom, K. Purkait, B. Saubaméa, P. Burckel, P. Arnoux, C. Frochot, K. Cariou, T. Rossel and G. Gasser, Ru(II)/Os(II)-based carbonic anhydrase inhibitors as photodynamic therapy photosensitizers for the treatment of hypoxic tumours, Chem. Sci., 2023, 14, 11749–11760 RSC
.
- J. Liu, Y. Wu, G. Yang, Z. Liu and X. Liu, Mitochondrial targeting half-sandwich iridium(III) and ruthenium(II) dppf complexes and in vitro anticancer assay, J. Inorg. Biochem., 2023, 239, 112069 CrossRef CAS PubMed
.
- G. Sahu, S. A. Patra, S. Lima, S. Das, H. Görls, W. Plass and R. Dinda, Ruthenium(II)-dithiocarbazates as anticancer agents: synthesis, solution behavior, and mitochondria-targeted apoptotic cell death, Chem. – Eur. J., 2023, 29, e202202694 CrossRef CAS PubMed
.
- S. Kuang, F. Wei, J. Karges, L. Ke, K. Xiong, X. Liao, G. Gasser, L. Ji and H. Chao, Photodecaging of a mitochondria-localized Iridium(III) endoperoxide complex for two-photon photoactivated therapy under hypoxia, J. Am. Chem. Soc., 2022, 144, 4091–4101 CrossRef CAS PubMed
.
- S. Kuang, L. Sun, X. Zhang, X. Liao, T. W. Rees, L. Zeng, Y. Chen, X. Zhang, L. Ji and H. Chao, A mitochondrion-localized two-photon photosensitizer generating carbon radicals against hypoxic tumors, Angew. Chem., Int. Ed., 2020, 59, 20697–20703 CrossRef CAS PubMed
.
- E. Balsa, E. A. Perry, C. F. Bennett, M. Jedrychowski, S. P. Gygi, J. G. Doench and P. Puigserver, Defective NADPH production in mitochondrial disease complex
I causes inflammation and cell death, Nat. Commun., 2020, 11, 2714 CrossRef CAS
.
- V. Singh, N. K. Rana, M. Kashif, P. P. Manna, T. S. B. Baul and B. Koch, Aqua-(2-formylbenzoato)triphenyltin(IV) induces cell cycle arrest and apoptosis in hypoxic triple negative breast cancer cells, Toxicol. in Vitro, 2023, 86, 105484 CrossRef CAS PubMed
.
- S. Nikolić, J. Arakelyan, V. Kushnarev, S. M. Alfadul, D. Stanković, Y. I. Kraynik, S. Grgurić-Šipka and M. V. Babak, Coordination of Ru(II)-arene fragments to dipyridophenazine ligands leads to the modulation of their in vitro and in vivo anticancer activity, Inorg. Chem., 2023, 62, 8188–8199 CrossRef
.
- J. Zhao, Y. Gao, R. Huang, C. Chi, Y. Sun, G. Xu, X. H. Xia and S. Gou, Design of Near-Infrared-Triggered Metallo-Photosensitizers via a Self-Assembly-Induced Vibronic Decoupling Strategy, J. Am. Chem. Soc., 2023, 145, 11633–11642 CrossRef CAS PubMed
.
- A. Upadhyay, A. Nepalia, A. Bera, D. K. Saini and A. R. Chakravarty, Platinum(II) boron–dipyrromethene complex for cellular imaging and mitochondria–targeted photodynamic therapy in red light, Chem. – Asian J., 2023, 18, e202300667 CrossRef CAS PubMed
.
Footnote |
† Electronic supplementary information (ESI) available: HPLC spectra, stability curve, 1O2 generation plot, cell viability plots, confocal images, and caspase-3 activity plot. See DOI: https://doi.org/10.1039/d4qi01601g |
|
This journal is © the Partner Organisations 2024 |