DOI:
10.1039/D3QI01902K
(Research Article)
Inorg. Chem. Front., 2024,
11, 172-185
A latest-generation fluoride with excellent structural stiffness for ultra-efficient photoluminescence and specific four-peak emission temperature sensing†
Received
19th September 2023
, Accepted 3rd November 2023
First published on 3rd November 2023
Abstract
Fluorides have garnered tremendous attention in rare-earth-doped fluorescent probes owing to their low phonon energy and excellent optical transparency. However, the latest generation of fluorides, LiYF4, is plagued by extremely complex and uncontrollable synthesis methods, which greatly restricts its further exploration and application. Herein, a straightforward one-step method for the synthesis LiYF4:Ln3+ with micron-sized cones and nano-spheres is reported. Astonishingly, self-sensitized luminescence was achieved under multi-wavelength excitation when Er3+ was singly doped. LiYF4:Yb3+,Er3+ demonstrated superior luminescence intensity to those of commercial green phosphors (NaYF4:Yb3+,Er3+); this ultra-efficient photoluminescence was confirmed from the crystal structure, electronic band properties, morphological analysis and Debye temperature calculations. Further, by constructing cross-relaxation between the Ce3+ and Er3+ ions (4I11/2 + 2F5/2 → 4I13/2 + 2F7/2), the specific four-peak emission (SFPE) intensity of Er3+ in NIR-IIb was significantly increased, further enhancing the relative sensitivity of thermally coupled temperature sensing based on SFPE. Subsequently, non-thermally coupled temperature sensing based on SFPE was also achieved through the construction of phonon-assisted energy transfer between Ho3+ 5I6 and Er3+ 4I13/2. In summary, this paper not only puts forward theoretical and experimental arguments for the use of LiYF4 to replace the conventional NaYF4, but also substantiates the extraordinary prospects of LiYF4 as a temperature-sensitive fluorescent probe in nanomedicine.
1. Introduction
The photoluminescence (PL) efficiency of rare earth ions depends primarily on the crystal field, structure, phonon energy and local positional symmetry of the host material.1 Amongst these characteristics, phonon energy is a critical component affecting the quantum yield. Hosts having low phonon energy can suppress the multi-phonon relaxation process and reduce non-radiative energy losses.2 In particular, phonon energy is essential for the emission of rare earth ions in the NIR, as NIR emission is susceptible to being quenched by high-energy vibrations.3 Therefore, the choice of host determines the optical properties of rare-earth-ion dopants. Rare-earth-ion-doped fluorides have excellent photoluminescence efficiency due to their high photodamage threshold and low phonon energy.4 Among them, NaYF4 is widely used for rare-earth-doped photoluminescence5,6 and bioimaging in the NIR due to its outstanding chemical stability and low phonon energy.7 NaYF4:18%Yb3+,2%Er3+ is even known as a commercial green phosphor owing to its high-intensity green light emission.8 However, LiYF4 has received little attention.
As currently reported, LiYF4:Nd3+ has an amazingly high quantum efficiency in the NIR-II (28%) under 808 nm excitation, with relatively stronger emission and higher quantum yields than NaYF4 NPs with the same doping ratio, much higher than any previously reported fluoride.9 Xiuwen Wang et al. investigated the effect of Li+ ion doping on the morphology, structure and up-conversion luminescence of NaYF4:Yb3+,Er3+ nanocrystals and found a significant enhancement of the up-conversion luminescence by Li+ ion doping,10 but did not investigate the principle in depth. In fact, LiYF4, a representative fluoride, has the advantage of low phonon energy and optimal optical transparency to minimize non-radiative energy losses. Rare-earth-ion-doped LiYF4 crystals demonstrated superior quantum yields under excitation at 1490 nm compared to NaYF4 crystals.11 However, the complex synthesis process of LiYF4 and the high operational and environmental requirements have hindered further research. Among the synthesis methods reported so far, there are thermal decomposition methods that use precise control of the synthesis environment and process,12,13 two-step synthesis methods that use stepwise regulation of the synthesis of YF3 and then LiYF4,14 and improved methods that use special high-pressure steel kettles or rely on high-temperature tube furnaces.15 In general, the synthesis process is more tedious and technically demanding than for other fluorides, limiting its application to rare-earth-doped luminescence and contactless temperature sensing.
In this work, we have successfully synthesized rare-earth-doped LiYF4via a one-step hydrothermal method. When LiYF4 was singly doped with Er3+ ions, it displayed Er3+ ion self-sensitization and high-intensity luminescence with multi-wavelength excitation.16,17 After the introduction of the sensitizer Yb3+, its ultra-efficient photoluminescence was 2.06 times higher than that of commercial green phosphors (NaYF4:Yb3+,Er3+), and the specific four-peak emission (SFPE) in the NIR-IIb was 3.15 times higher than the single-peak emission of commercial green phosphors. Even more surprising is the excellent performance in temperature sensing based on the fluorescence intensity ratio of the two green thermally coupled energy levels (Er3+ 2H11/2, 4S3/2) and SFPE in the NIR-IIb. Subsequently, we co-doped Ce3+ ions to achieve a 3.07-fold increase in emission intensity in the NIR-IIb region while achieving a further increase in relative sensitivity. Finally, temperature sensing based on the Ho3+ and Er3+ energy levels was also achieved. A pathway has been opened for the application of LiYF4 in bioimaging and nanomedicine.
2. Results and discussion
2.1 Structure and composition
The XRD patterns of LiYF4:x%Er3+ phosphors (x = 0.6, 0.8, 1.0, 1.2, 1.4, and 1.6) are shown in Fig. 1c; all the diffraction patterns were well matched with the standard reference, indicating that all the generated samples exhibited favorable single phases. Furthermore, as the concentration of Er3+ doping increased, the locations of the diffraction peaks in the XRD patterns moved toward smaller angle, revealing that the substitution of Er3+ with Y3+ resulted in the substitution of an ion with a greater ionic radius. The General Structure Analysis System (GSAS) was used to apply Rietveld analysis to refine the LiYF4:1%Er3+ phosphors, as shown in Fig. 1d. The low residual factor parameters (Rp = 6.35%, Rwp = 8.77%) demonstrated the high purity of the sample. The simple hydrothermal one-step stable synthesis of rare-earth-doped LiYF4 sets the foundation for our subsequent discussion.
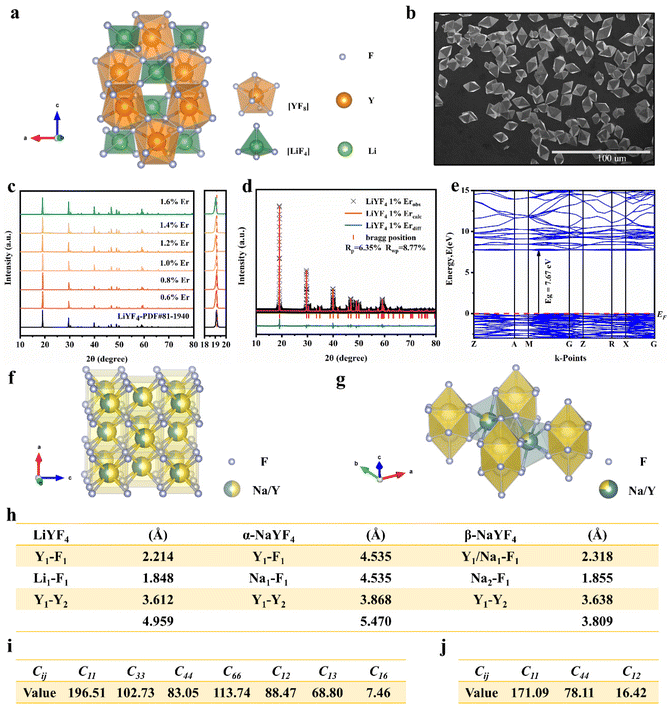 |
| Fig. 1 (a) Structural model of the LiYF4 host and the F, Y, and Li site positions in the structure. (b) SEM image of the LiYF4 host. (c) XRD patterns of the LiYF4:x%Er3+ phosphors, along with the standard XRD data of LiYF4 (PDF 81-1940). (d) XRD Rietveld refinement of LiYF4:1%Er3+ phosphors. (e) Energy band structures of the LiYF4 host; the Fermi energy levels are indicated by dashed lines. Schematic illustrations of unit cell structures of α-NaYF4 (f) and β-NaYF4 (g). (h) Bond lengths (Å) in LiYF4, α-NaYF4 and β-NaYF4. Calculation results of the elastic coefficients Cij of LiYF4 (i) and NaYF4 (j). | |
2.1.1 Structural properties.
The compound LiYF4 crystallizes in a tetragonal system with the space group I41/a; the ordered conformation is shown in Fig. 1a, in which Y3+ and Li+ are located at the center of the octa-coordinated dodecahedron [YF8] and the tetra-coordinated tetrahedron [LiF4], respectively. Nevertheless, as illustrated in Fig. 1f, α-NaYF4 is a disordered crystal, in which Y3+ and Na+ stochastically take up the cationic position. In the disordered β-NaYF4 crystal (Fig. 1g), there are two Ln3+ cation sites; one is occupied by Na+, and the other one is dominated exclusively by Na+ and Y3+ at random. The sensitizer and activator ions Yb3+ (112.5 pm, CN = 8), Er3+ (114.4 pm, CN = 8) and Ho3+ (115.5 pm, CN = 8) tend to assume the position of Y3+ (115.9 pm, CN = 8), forming the luminescence centre in LiYF4 and NaYF4. Therefore, compared to NaYF4:Ln3+, the superior luminescence efficiency for LiYF4:Ln3+ is reasonable based on their ordered cation sites.18 In addition, the Y–Y atomic distance in LiYF4 is shorter compared to those of α-NaYF4 and β-NaYF4, and it is well-known that the closer Ln3+ position in the crystal facilitates efficient Ln3+–Ln3+ energy transfer and thus yields efficient photoluminescence. The bond lengths of Y–F in LiYF4, α-NaYF4 and β-NaYF4 are 2.214, 4.535 and 2.318 Å, respectively, and the Li/Na–F bond lengths are 1.848, 4.535 and 1.855 Å, respectively (Fig. 1h). With the shorter bond lengths in LiYF4, the bonding forces between the bonded atoms are the stronger, which is also consistent with the fact that the lighter the atoms, the greater the interatomic forces. In terms of structural properties, the better luminous efficiency of LiYF4:Ln3+ was justified by its tight lattice structure.18
Fig. 1b presents the SEM image of the LiYF4 host, which exhibited excellent dispersion and micron-sized octahedral cones. Here, we present an analysis of the morphological structure of the octahedral conical LiYF4 and the conventional spherical NaYF4; as shown in Fig. S1,† the octahedral cones have an edge length d, volume (Vc), and surface area (Sc):
| 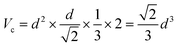 | (1) |
| 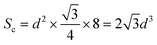 | (2) |
For the traditional spherical NaYF4 with radius r, the volume (Vs), and surface area (Ss) are:
|  | (3) |
when
Vs =
Vc:
| 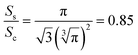 | (5) |
Thus, the octahedral conical LiYF4 has a larger surface area than that of commonly reported sphere-like NaYF4 for an identical volume, which improves the thermal response of their up-conversion behavior,19 and this would further improve the sensitivity of the temperature-sensitive fluorescent probe.
2.1.2 Electronic band properties.
A detailed calculation of the energy band properties of LiYF4 was performed to verify whether its electronic structure was suitable to facilitate Ln3+ emission. The CASTEP software package in the software Materials Studio was used for the calculation. As shown in Fig. 1e, the band gap value of the LiYF4 system was 7.67 eV, which was also very close to the result calculated (7.52 eV) by Yang Xiao et al.20,21 It was further confirmed by the diffuse reflectance spectrum in Fig. S2.† Owing to the photoionization effect, the bandgap is also related to thermal stability and luminous efficiency. Additionally, the photoionization process can be impeded by a wide bandgap. Consequently, the luminescence intensity of phosphors is anticipated to be positively proportional to the bandgap of the host crystal lattice.22 Based on the unique electronic structure of LiYF4, its wide bandgap of >3.3 eV is advantageous for accommodating the electronic states of the rare-earth-ion luminescence centers. The density of states (DOS) and the partial atomic components (PDOS) of LiYF4 are shown in Fig. S3,† which reveals that the conduction band (CB) was mainly contributed by Li and Y ions, with very little PDOS contribution from the F ions, while the valence band (VB) was mainly contributed by F ions, with very little PDOS contribution from the Li and Y ions, and that while the important interactions between the F 2s and Y 4p orbitals are rarely discussed in the literature, they are also important interaction mechanisms.
2.1.3 Structural stiffness.
Crystal structures with higher structural stiffness have weaker phonon energies, which can limit the electron–phonon coupling effect to some extent, implying a lower probability of non-radiative relaxation.2 The Debye temperature and Young's modulus E can be used to describe the structural stiffness.23 The elastic coefficient Cij was calculated using the CASTEP software package, and the calculations for LiYF4 and NaYF4 are displayed in Fig. 1i and j, respectively. Taking LiYF4 as an example, we made continued calculations for its Debye temperature and Young's modulus (E). The stability requirement was determined by measuring the elastic coefficients Cij of the tetragonal phase LiYF4 as follows: C11 > |C12|, 2C132 < C33(C11 + C12), C44 > 0, and 2C162 < C66(C11–C12), indicating the rationale of the calculation results.
According to the Voigt approximation, the shear modulus GV and bulk modulus BV can be expressed in relation to the elastic coefficients Cij of the crystal as:
|  | (6) |
| 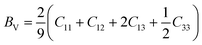 | (7) |
According to the Reuss approximation, the shear modulus GR and bulk modulus BR have the following relationships with the elastic compliance coefficients Sij of the crystal:
|  | (8) |
| 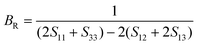 | (9) |
The compliance coefficient Sij is the inverse matrix of the elasticity coefficient Cij, the Voigt and Reuss equations describe the upper and lower limits of the real crystal constant, respectively, and the crystal modulus is simply the arithmetic average given by Voigt and Reuss, which is also called the Voigt–Reuss–Hill approximation. The calculation formula is as follows:
|  | (10) |
|  | (11) |
Young's modulus (E) represents the stiffness of the material and can be further calculated as:
|  | (12) |
The compressional longitudinal wave velocity (VP) and transverse wave velocity (VS) were calculated as:
|  | (13) |
|  | (14) |
The mean sound velocity Vm can be obtained as:
| 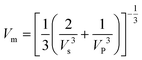 | (15) |
The Debye temperature θD can be obtained from the average sound speed and Debye approximation:
| 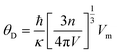 | (16) |
where
ħ is the Bronk constant,
k is the Boltzmann constant,
n is the number of atoms in the protocell,
V is the protocell volume, and
Vm is the average phonon velocity.
Based on the calculation results, the Young's modulus (E) value of LiYF4 (ELiYF4) is 164 N m−2, and the Debye temperature (DLiYF4) is 569 K; this result is very similar to that in the previous literature report (570 K).24 The Young's modulus (E) of NaYF4 (ENaYF4) is 136 N m−2, and the Debye temperature (DNaYF4) is 532 K. ELiYF4 > ENaYF4 and DLiYF4 > DNaYF4; this is also consistent with the fact that the smaller the atomic mass, the greater the interatomic force, and the higher the Debye temperature. This is an important reason for the superior luminescence intensity of LiYF4. It also provided important theoretical support for LiYF4 as a better luminescence host to replace NaYF4.
Furthermore, the Debye temperature is a fixed eigenvalue of a crystal:
| 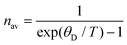 | (17) |
nav is the average phonon number. When exp(
θD/
T) − 1 < 1, that is, exp(
θD/
T) < 2,
T >
θD, then the phonon with the largest energy is excited. Low-frequency phonons can be generated at lower temperatures, and there are also less of them overall. As such, the higher Debye temperature has a higher luminous heat quenching temperature caused by multiphonon relaxation, and in theory, the effective temperature sensing range of LiYF
4 based on the luminous intensity ratio before the luminescence burst is greater than that of NaYF
4.
In parallel, we synthesized α-NaYF4 and β-NaYF4 phosphors using the hydrothermal method. The XRD patterns are shown in Fig. S4.† To experimentally verify our calculations, infrared spectroscopy was carried out. A detailed evaluation of the lattice vibrations of LiYF4, α-NaYF4 and β-NaYF4 is shown in Fig. S5.† The lattice vibrations can be assessed by the absorption positions;20 the absorption positions of LiYF4 (213, 264, 299 and 339 cm−1) were overall lower than those of α-NaYF4 (324, 409 and 517 cm−1) and β-NaYF4 (250, 330 and 400 cm−1). This was confirmation of the better structural stiffness of LiYF4.
2.2 Photoluminescence (PL) properties
Er3+-doped LiYF4 not only achieved self-sensitization, but also exhibited excellent luminescence properties under multiwavelength excitation. Under excitation at 980, 808 and 381 nm, the red emissions centered at 653 and 667 nm were assigned to the 4F9/2 energy level split, and the green emissions centered at 523–529 nm and 542–550 nm were ascribed to the 2H11/2-energy level split and 4S3/2 energy level split; the SFPE in the NIR-IIb under 808 and 980 nm excitation was attributed to the 4I13/2 energy level split. The emission spectra are shown in Fig. 2a and b (at 980 nm), Fig. 2c and d (at 808 nm) and Fig. 2f (at 381 nm). The ideal doping concentrations for the Er3+ emissions at the three excitation wavelengths were 1% for the visible region emission and 1.4% for the NIR. The emission slopes of the LiYF4:1%Er3+ phosphor at 529, 550, 667 and 1545 nm under 980 nm excitation in Fig. S6a and c† were 2.12, 2.04, 1.97 and 0.95, respectively. The green and red emission were two-photon processes and NIR emission was a single-photon process based on the fitted slope value n (I ∝ Pn ), and the energy conversion mechanism at 980 nm excitation is shown in Fig. 3b. Equally, the green and red emission of the Er3+ ions under 808 nm excitation were also two-photon processes (Fig. S6b†), and the NIR emission was a single-photon process (Fig. S6c†), with the energy conversion mechanism shown in Fig. 3c. Subsequently, we also examined the excitation spectra at 552 nm, as shown in Fig. 2e. The excitation spectra show centres at 361, 381, 414, 426 and 452 nm, corresponding to the well-known Er3+ ion 4I15/2 → 4G9/2, 4I15/2 → 4G11/2, 4I15/2 → 2H9/2, 4I15/2 → 4F9/2, 4I15/2 → 4F3/2, 4I15/2 → 4F5/2 and 4I15/2 → 4F7/2 transitions,25 respectively. Here, we used the highest absorption peak wavelength of 381 nm for excitation and obtained the emission spectra shown in Fig. 2f. Different from the excitation at 980 nm and 808 nm, the red and green light emission corresponded to single-photon processes, and the energy conversion mechanism is shown in Fig. 3d. Furthermore, we monitored the diffuse reflectance spectra of LiYF4 and LiYF4:1%Er3+ as shown in Fig. S7,† and the characteristic peaks of Er3+ ions that appeared in comparison to those of the LiYF4 matrix further validated its excellent self-sensitized luminescence properties.
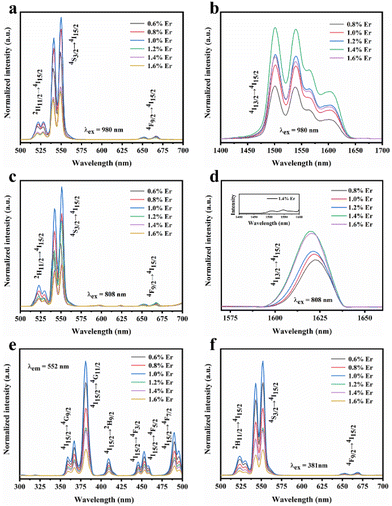 |
| Fig. 2 (a) Visible PL spectra and (b) NIR PL spectra of LiYF4:x%Er3+ under 980 nm excitation. (c) Visible PL spectra and (d) NIR PL spectra of LiYF4:x%Er3+ under 808 nm excitation. (e) PLE (λem = 552 nm) and (f) PL spectra (λex = 381 nm) of LiYF4:x%Er3+. | |
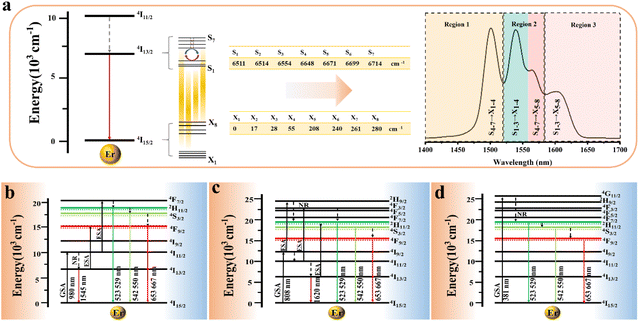 |
| Fig. 3 (a) Stark sublevels of the 4I13/2 and 4I15/2 levels of the Er3+ ions in the LiYF4 host, and the transition of each Stark sublevel corresponding to the SFPE of LiYF4:1.4%Er3+ under 980 nm excitation. Energy transfer mechanism of LiYF4:Er3+ under 980 nm (b), 808 nm (c), and 381 nm (d) excitation. | |
In addition, we further analyzed the SFPE of LiYF4:x%Er3+ in the NIR-IIb; it was mainly due to the splitting of the ground state 4I15/2 and excited state 4I13/2 into multiple Stark sub-levels by the action of the crystal field. The electronic transitions from the 4I13/2 to the 4I15/2 energy levels of Er3+ ions involve intra-configurational transitions within the 4fN configuration, known as 4f–4f transitions. These transitions are not induced by electric dipole interactions because the parity of states within the 4fN configuration is the same, resulting in a zero value for the matrix elements of electric dipole transitions between these states. Consequently, transitions between energy levels within the 4fN configuration are parity-forbidden. However, 4f–5d transitions are allowed, as the matrix elements of electric dipole transitions between these levels are non-zero. Odd-rank crystal field terms can mix configuration states with opposite parity to the 4fN configuration, such as 4fN−15d or 4fN−15g configurations. However, crystallographic point groups with inversion symmetry, like C4h, do not have odd-rank crystal field terms, and according to the selection rules for rare-earth-ion electric dipole transitions in crystals: Δl = ±1, ΔS = 0, |ΔL| ≤ 6, |ΔJ| ≤ 6, when J or J′ = 0, |ΔJ| = 2, 4, 6, |ΔM| = p + q, electric dipole transitions from the 4I13/2 to 4I15/2 energy levels of Er3+ ions are forbidden.
In these 4f–4f transitions, magnetic dipole and electric quadrupole transitions are also significant. Based on the selection rules for magnetic dipole transitions: Δl = 0, ΔS = 0, ΔL = 0, |ΔJ| = 0, ±1, ΔM = 0, ± 1, and electric quadrupole transitions: Δl = 0, ΔS = 0, ΔL ≤ 2, ΔJ ≤ 2, transitions involving magnetic dipole and electric quadrupole moments from the 4I13/2 to 4I15/2 energy levels of Er3+ ions are allowed. Generally, the energy level splitting of rare earth ions can be decomposed based on the irreducible representation of the point group, using the character of the irreducible representation of the point group to approximate the character of the rotational group representation. This decomposition process was exactly the same as that of the energy levels, because the irreducible representation of the point group in the crystal was actually the quantum number that can completely determine the state, and it had the same nature as the angular momentum quantum number in the three-dimensional rotational group, which can characterize the states and energy levels of the rare earth ions in the crystal. The character of the rotational group representation under various symmetry operations can be calculated using the following formula:
| 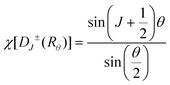 | (18) |
In the formula, + indicates even parity, and − indicates odd parity. Rθ represents the θ angles along the axis of rotation. J is the total angular momentum of the rare earth energy levels. The character represented by any rotation group of J can be computed using this formula. Then, the character of the rotation group is obtained, and the character of the point group irreducible representation is used to decompose the character of the rotation group representation. The number of irreducible representations of the point group contained in the obtained rotation group representation is the number of Stark levels decomposed by the J level in the point group. LiYF4 has the I41/a space group, which belongs to the C4h point group. C4h = C4 × Ci; it can be viewed as direct product of the group C4 and the group Ci. The decomposition of the rotation group in this point group is obtained by the compatibility relation between the irreducible representations of these two groups, and the process can be referred to Table S1.† We eventually found the number of Stark levels of the 4I13/2 (J = 13/2) level and the 4I15/2 (J = 15/2) level for the Er3+ ion to be 7 and 8, respectively (marked as S1 → S7, X1 → X8), which is consistent with the calculation of the Harry Diamond Laboratories using diagonalization of the Hamiltonian.26 Based on these specific calculation results of the Stark levels of 4I13/2 and Stark levels of 4I15/2 (S1 → S7: 6511, 6514, 6554, 6648, 6671, 6699, 6714 cm−1; X1 → X8: 0, 17, 28, 55, 208, 240, 261, 280 cm−1), and according to the difference in wavenumbers between the various Stark energy levels, we have drawn the transition energy level diagram of SFPE, as shown in Fig. 3a, corresponding to the emission spectrum, with the four parts from left to right coming from S4–7 → X1–4, S1–3 → X1–4, S4–7 → X5–8 and S1–3 → X5–8, respectively. The SFPE in NIR-IIb opens the possibility for the following temperature sensing applications at both thermally coupled Stark sub-levels and non-thermally coupled levels.
2.2.2 Luminescence enhancement and ultra-efficient PL.
We further demonstrated the superior luminous intensity of LiYF4 with the introduction of the sensitizer Yb3+ due to its higher structural stiffness. Fig. S8a† presents the XRD images of LiYF4:18%Yb3+,x%Er3+ (x = 1, 2, 3, 4, 5). The emission spectra in the visible range and NIR-IIb are presented in Fig. S8b, c,† and the optimal doping concentration was 18%Yb3+,2%Er3+ in the visible region and 18%Yb3+,3%Er3+ in the NIR-IIb. After the incorporation of the sensitizer, there was a 9.60-fold improvement in the visible region and 5.76-fold enhancement in the NIR-IIb region. The emission intensity of β-NaYF4:18%Yb3+,2%Er3+ (commercial phosphors) and LiYF4:18%Yb3+,2%Er3+ phosphors was compared. It was clear that the ultra-efficient green emission of the LiYF4:18%Yb3+,2%Er3+ phosphors was outstanding, as it was 2.06 times higher than that of commercial β-NaYF4:18%Yb3+,2%Er3+ phosphors (Fig. 4a). The NIR-IIb emission intensity of the LiYF4:18%Yb3+,2%Er3+ phosphor was also excellent, being 3.15 times stronger than that of β-NaYF4:18%Yb3+,2%Er3+ phosphors; that of LiYF4:18%Yb3+,3%Er3+ was 3.75 times that of β-NaYF4:18%Yb3+,2%Er3+ (Fig. 4b). Furthermore, the SFPE of LiYF4:18%Yb3+,3%Er3+ in NIR-IIb made it possible to use for thermally coupled temperature sensing at the Stark level.
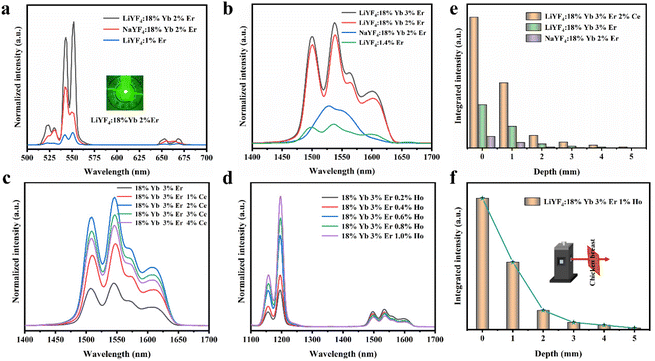 |
| Fig. 4 (a) Up-conversion spectra (visible region) of LiYF4:2%Er3+, LiYF4:18%Yb3+,2%Er3+ and NaYF4:18%Yb3+,2%Er3+ under 980 nm excitation. (b) NIR down-conversion spectra of LiYF4:1.4%Er3+, LiYF4:18%Yb3+,2/3%Er3+ and NaYF4:18%Yb3+,2%Er3+ under 980 nm excitation. (c) NIR down-conversion spectra of LiYF4:18%Yb3+,2%Er 3+,x%Ce3+ under 980 nm excitation. (d) Down-conversion spectra (NIR) of LiYF4:18%Yb3+,2%Er 3+,x%Ho3+ under 980 nm excitation. The emission integrated intensity at 1400–1700 nm (e) and 1100–1700 nm (f) in different depths of chicken breast. | |
To further improve the NIR emission, two separate ion doping systems were constructed. Fig. S9† depicts the XRD results of LiYF4:18%Yb3+,3%Er3+,x%Ce3+ (x = 1, 2, 3 and 4), and Fig. 4c presents the NIR-IIb emission spectrum under 980 nm excitation, with the emission intensity peaking at 2% Ce3+ doping. The intensity of the NIR-IIb emission was increased by a coefficient of 3.07 compared to that of LiYF4:18%Yb3+,3%Er3+. This was attributed to the cross-relaxation between Er3+ and Ce3+ ions (4I11/2 + 2F5/2 → 4I13/2 + 2F7/2), causing a further increase in the 4I13/2 (Er3+) energy level population. However, the cross-relaxation between Ce3+ ions increased as the Ce3+ ion concentration increased, and the optimum doping concentration was found to be 2%. Following this, the depth of penetration of LiYF4:18%Yb3+,3%Er3+,2%Ce3+ in chicken breasts was explored to illustrate their significant potential for bioimaging. As shown in Fig. S10a,† with the increased depth of the chicken breast, the emission intensity diminishes considerably. Compared with the penetration depth of LiYF4:18%Yb3+,3%Er3+ (4 mm) and β-NaYF4:18%Yb3+,2%Er3+(3 mm), LiYF4:18%Yb3+,3%Er3+,2%Ce3+ achieved a depth of 5 mm (Fig. 4e). Subsequently, using co-doping with Ho3+ ions, it was investigated whether the energy transfer (as depicted in Fig. 6g) between the Ho 5I6 and 5I7 energy levels and the Er 4I13/2 energy levels played a role in promoting the NIR-IIb emission of the Er3+ ions. LiYF4:18%Yb3+, 3%Er3+,x%Ho3+ (x = 0.2, 0.4, 0.6, 0.8 and 1.0) were prepared. Their XRD patterns are shown in Fig. S9b,† and their NIR emission spectra under 980 excitation are shown in Fig. 4d. On one hand, the probability of phonon-assisted energy transfer was relatively lower because phonon energy was not excited in large quantities at room temperature, and the energy level difference between Er 4I13/2 and Ho 5I7 (1494 cm−1) was less than that of Ho 5I6 to Er 4I13/2 (2004 cm−1), of which the former energy transfer played a dominant role. On the other hand, there was competition between the Yb–Er and Yb–Ho energy transfer. As a result, the SFPE intensity of the Er3+ ions decreased with increasing doping concentration of Ho3+ ions. Despite this, LiYF4:18%Yb3+,2%Er3+,1%Ho3+ also penetrated to a depth of 5 mm (Fig. 4f) and the PL spectra for different depths of chicken breast are shown in Fig. S10b.† The tremendous promise of LiYF4 in the bioimaging field was demonstrated.
2.3. Temperature sensing performance
2.3.1 Temperature sensing in the visible region.
Temperature sensing based on luminous intensity ratio (LIR) technology27 is one of the most popular non-contact temperature detection methods.28 Fig. S15† presents the experimental equipment schematic for temperature sensing. The two green photothermal thermally coupled levels of Er3+ (2H11/2, 4S3/2) are usually used as the two energy levels for temperature sensing. Here, the LiYF4:18%Yb3+,3%Er3+ phosphors were excited at 980 nm. In the visible region, the ratio of the signals of the 2H11/2 and 4S3/2 levels of Er3+ were chosen for the following temperature sensing experiments. Fig. 5a shows the green emission spectra at various temperatures. The variation of the integral of the emission intensity with temperature for the thermally coupled levels in the LiYF4:18%Yb3+,3%Er3+ phosphor is shown in Fig. 5b; a significant difference can be found in the fact that the average integral intensity of the 4S3/2 → 4I15/2 (550 nm) transition decreased more rapidly than that of the 2H11/2 → 4I15/2 (529 nm) transition. The relative population of thermally coupled levels was compatible with the Boltzmann distribution law, which can be calculated using eqn (19):29 | 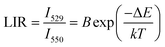 | (19) |
where I529 and I550 represent the integral emission intensities of Er3+ (2H11/2 and 4S3/2), and B is a fitted parameter. ΔE is the energy difference between 2H11/2 and 4S3/2. k is the Boltzmann constant, and T is the absolute temperature. As presented in Fig. 5c, the values of B and ΔE/k were 7.1035 and 1107.2625, respectively.
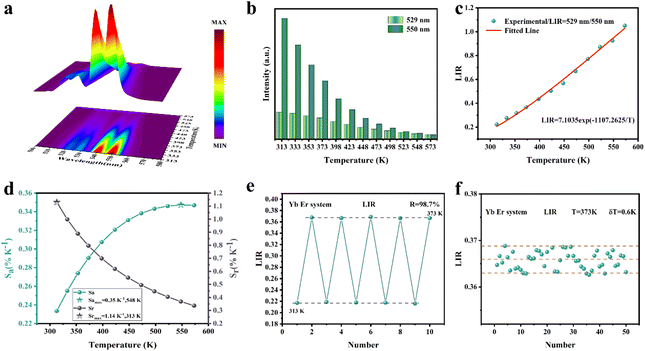 |
| Fig. 5 (a) Temperature-dependent upconversion (UC) emission spectra of LiYF4:18%Yb3+,3%Er3+. (b) Summary of the integrated intensity of the emission of the thermally coupled levels in the LiYF4:18%Yb3+,3%Er3+ phosphors at different temperatures. (c) LIR values related to the temperature. (d) Sr/Sa-temperature curve of LiYF4:18%Yb3+,3%Er3+. (e) LIR repeatability over five heating–cooling cycles. (f) LIR values measured 50 times continuously at 373 K. | |
According to previous reports, in order to better evaluate and compare the temperature measurement capabilities of different thermometers, quantitative comparisons are usually made using absolute sensitivity (Sa) and relative sensitivity (Sr), which can be estimated using the following expressions:
|  | (20) |
| 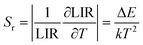 | (21) |
As shown in Fig. 5d, Sa and Sr have a high degree of fitting accuracy. However, regardless of the physical mechanism of the luminous thermometer, it is not reliable to use Sa to assess its temperature measurement performance.30 Instead, Sr should be used for comparison. Nevertheless, the maximum Sa (0.35%) of the phosphor was not very low. The LiYF4:18%Yb3+, 3%Er3+ phosphor exhibits excellent Sr (1107/T2), reaching a maximum of 1.14% K−1 at 313 K, showing excellent accuracy compared to those in other luminescent materials that utilize the Er3+ thermally coupled levels for temperature measurement (Table 1). In addition, we performed five more heating–cooling cycles to investigate the effect on its LIR repeatability, as shown in Fig. 5e. R can be used to describe the ability of a thermometer to provide the same result repeatedly in the same environment and is calculated as:
| 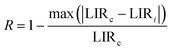 | (22) |
where LIR
c is the standard value extracted from the calibration curve and LIR
i is the value for each measurement.
R was 98.7%, which indicated that our phosphors have good reproducibility. The LiYF
4:18%Yb
3+,3%Er
3+ phosphors were measured 50 times continuously at 373 K to study the temperature uncertainty (
δT), as shown in
Fig. 5f. The temperature uncertainty (
δT) is a critical property for measuring temperature readout performance and can be calculated according to
eqn (23):
|  | (23) |
here
δLIR is the standard deviation of the LIR, and
δLIR/LIR is the relative uncertainty of the LIR, depending on the material used and the experimental testing device. The value of temperature uncertainty (
δT) was calculated to be very small at 0.6 K, which demonstrates the excellent accuracy of the temperature measurement.
Table 1 Comparison of the temperature measurement performance of different materials
Compound |
λ
ex (nm) |
λ
em (nm) |
Temperature (K) |
S
r (%K−1) |
Ref. |
NaYF4:Yb,Er |
980 |
522/553 |
160–320 |
1082/T2 |
31
|
LaF3:Yb,Er |
980 |
523/545 |
150–400 |
844/T2 |
32
|
CaWO4:Yb,Er |
980 |
521/548 |
300–530 |
946/T2 |
33
|
Gd2O3:Yb,Er |
980 |
522/546 |
300–900 |
746/T2 |
34
|
LiYF4:Yb,Er |
980 |
529/550 |
313–573 |
1107/T2 |
This work |
2.3.2 Temperature sensing in the NIR-IIb.
We produced LiYF4:18%Yb3+,3%Er3+, LiYF4:18%Yb3+,3%Er3+, 0.2%Ho3+ and LiYF4:18%Yb3+,3%Er3+,2%Ce3+ nanospheres with a size of about 205 nm by optimizing the synthesis process, which makes it possible to use LiYF4 in nanomedicine. The XRD patterns are shown in Fig. S11a,† and the SEM images are shown in Fig. S11b–d.† We divided the SFPE in the NIR-IIb into three regions (region 1: S4–7 → X1–4, region 2: S1–3 → X1–4 and S4–7 → X5–8, region 3: S1–3 → X5–8) for convenience in the following temperature sensing. Fig. 6a presents the temperature-dependent NIR-IIb emission spectra of LiYF4:18%Yb3+,3%Er3+. The increase in temperature caused spectral broadening and a blue-shift in the position of the emission peak in region 1, as illustrated in Fig. S13.† This was mostly due to the fact that the 4I13/2 Stark sublevels S1–3 and S4–7 follow the Boltzmann thermal distribution, causing the number of S4–7 electrons to rise with temperature. S4 to S7 also satisfy the Boltzmann thermal distribution, so that the electrons continue to be distributed to higher energy levels, causing the blue shift of the peak position and spectral broadening. Then, thermal quenching brought on by an increase in temperature was responsible for the decrease in peak intensity. At the same time, the emission integral intensity of region 1 (S4–7 → X1–4) increased with increasing temperature (Fig. S12a†). This was due to the fact that the Stark sublevels X1–4 and X4–5 of 4I15/2 also conform to the Boltzmann redistribution, resulting in the continuous decrease in the number of X1–4 electrons, but an increasing number of electrons in S4–7, giving a greater chance of a transition from S4–7 to X1–4. On the contrary, the emission integral intensity of region 3 (S1–3 → X5–8) decreased with increasing temperature (Fig. S12a†). This was because S1–3 and S4–7, X1–4 and X5–8 follow the Boltzmann thermal distribution, which led to a continuous decrease in the number of S1–3 electrons and the continuous saturation of X5–8 electrons, decreasing the likelihood of the transition from S1–3 to X5–8. Using eqn (19), the LIR (region 1: S4–7 → X1–4/region 3: S1–3 → X5–8) values can be calculated, and they are given in Fig. 6d. The Sa and Sr values were calculated, as shown in Fig. 6e, f and it was found that Sa max = 0.622% K−1 (T = 313 K), while the Sr value was 450/T2 with Sr max = 0.460% K−1 (T = 313 K); this is higher than that of NaScF4:Yb3+,Er3+ (313/T2), which is one of the few substrates with SFPE in the NIR-IIb region, and even higher than that after increasing Sr (340/T2) by re-doping with Mn2+ ions (NaScF4:Yb3+,Er3+,Mn2+).35
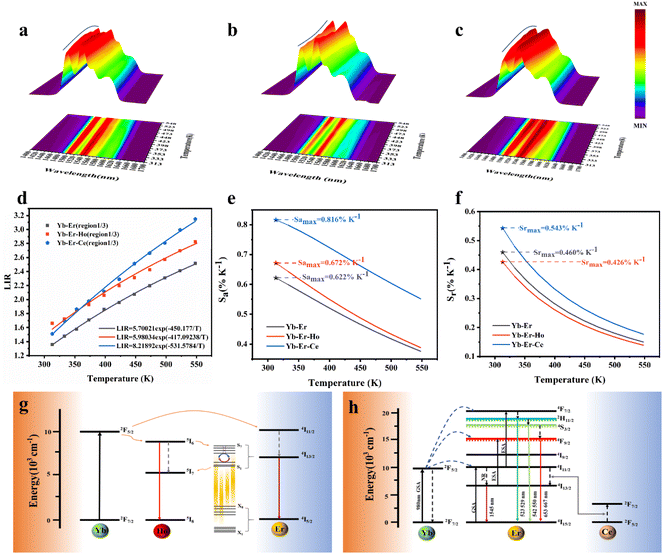 |
| Fig. 6 Temperature-dependent NIR-IIb emission spectra of LiYF4:18%Yb3+,3%Er3+ (a), LiYF4:18%Yb3+,3%Er3+,0.2%Ho3+ (b), and LiYF4:18%Yb3+,3%Er3+,2%Ce3+ (c). (d) LIR values as a function of temperature for Yb–Er, Yb–Er–Ho and Yb–Er–Ce. (e) Sa-temperature curve. (f) Sr-temperature curve. Energy transfer mechanisms of Yb–Er–Ho (g) and Yb–Er–Ce (h) under 980 nm excitation. | |
The SFPE intensity of the Er3+ ions decreased with increasing doping concentration of Ho3+ ions, as shown in Fig. 4d, so Li-YF4:18%Yb3+,3%Er3+,0.2%Ho3+ was selected to continue the temperature sensing study in the NIR-IIb. The temperature-dependent NIR-IIb emission spectra of LiYF4:18%Yb3+,3%Er3+,0.2%Ho3+ are shown in Fig. 6b. With increasing temperature, the emission peak position of region 1 was blue shifted, and the spectrum was broadened. Unlike in the case of LiYF4:18%Yb3+,3%Er3+, the emission peak intensity continued to increase with increasing temperature as a result of the continuous replenishment of the 5I6 level of the Ho3+ ions to the S4–7 level of the Er3+ ions, which cancels out the influence of thermal quenching on it. As shown in Fig. S12b,† region 1 had an increase in comprehensive intensity. Fig. 6g illustrates the energy transfer method. The ground-state 2F7/2 electrons of the Yb3+ ions absorbed the 980 nm photon energy to transition to the excited state 2F5/2 level, and the energy was transferred to the activators so that the ground-state electrons of the Ho3+ ions and Er3+ ions absorbed energy to transition to the Ho3+–5I6 and Er3+–4I11/2 levels. Then, the 1200 nm NIR-IIa emission of the Ho3+ ions was generated. In contrast, the electrons of the Er3+ 4I11/2 level reached the Er3+ 4I13/2 level through non-radiative relaxation, and the 4I13/2 → 4I5/2 electron transition produced the SFPE in the NIR-IIb of the Er3+ ions. However, as shown in Fig. 6g, there was energy transfer between the Ho3+ 5I6 and 5I7 levels and the Stark sublevels S1–3 and S4–7 of the Er3+ 4I13/2 level, which breaks the thermal coupling distribution between the Stark sublevels of the Er3+ ions, and this is a possible cause of the slight decrease in Sr. Fig. 6d shows the LIR (region 1: S4–7 → X1–4/region 3: S1–3 → X5–8) fitting curve. The maximum Sa value of the LiYF4:18%Yb3+,3%Er3+,0.2%Ho3+ nanospheres was 0.672% K−1 (T = 313 K) (Fig. 6e), and the Sr value was 417/T2 with Sr max = 0.426 K−1 (T = 313 K) (Fig. 6f). Although the value of Sr was lower than that without Ho3+ doping, it was still higher than that of NaScF4:Yb3+,Er3+. Furthermore, it provided the possibility for subsequent temperature sensing in the NIR-IIa and NIR-IIb.
LiYF4:18%Yb3+,3%Er3+,2%Ce3+ nanospheres were again selected for temperature sensing research. The temperature-dependent NIR-IIb emission spectra of LiYF4:18%Yb3+,3%Er3+,2%Ce3+ are presented in Fig. 6c, and the energy transfer mechanism is shown in Fig. 6h. Cross relaxation between the Ce3+ ions and Er3+ ions (4I11/2 (Er3+) + 2F5/2 (Ce3+) → 4I13/2 (Er3+) + 2F7/2 (Ce3+)) was brought about by the doping of Ce3+ ions and was primarily responsible for the amplification of the SFPE intensity of the Er3+ ions in the NIR-IIb. Because of this, the number of electrons at the 4I13/2 level was only increased and there was no energy transfer with the thermally coupled S1–7 Stark sublevels of Er3+ as in the case of Ho3+ ions, which led to a more pronounced increase in the integrated intensity of region 1 and a more significant decrease in the integrated intensity of region 3 with increasing temperature (Fig. S13c†). The LIR (region 1: S4–7 → X1–4/region 3: S1–3 → X5–8) values as a function of temperature are shown in Fig. 6d. The Sa and Sr values were calculated, and I was found that Sa max = 0.816% K−1 (T = 313 K), and the Sr was 532/T2 with Sr max = 0.543% K−1 (T = 313 K) (Fig. 6e and f). Sa and Sr were significantly improved compared with those of LiYF4:18%Yb3+,3%Er3+. This showed the excellent accuracy compared to luminescent materials that use thermal coupling levels for temperature measurements in the NIR (Table 2).
Table 2 Comparison of the temperature measurement performance of different materials
Compound |
λ
ex (nm) |
Stark transition |
Temperature (K) |
S
r (%K−1) |
Ref. |
NaScF4:Yb/Er |
980 |
Er3+:4I13/2 → 4I15/2 |
298–333 |
313/T2 |
35
|
NaScF4:Yb/Er/Mn |
980 |
Er3+:4I13/2 → 4I15/2 |
298–333 |
340/T2 |
35
|
BaMoO4:Yb/Er |
980 |
Er3+:4I13/2 → 4I15/2 |
293–553 |
110/T2 |
36
|
TiO2:Yb/Er |
980 |
Er3+:4F9/2 → 4I15/2 |
307–673 |
107/T2 |
37
|
NaYbF4:Tm |
980 |
Tm3+:3H4 → 3H6 |
10–295 |
102/T2 |
38
|
CaF2:Nd/Y |
800 |
Nd3+:4F3/2 → 4I11/2 |
300–335 |
190/T2 |
39
|
LiYF4:Yb/Er |
980 |
Er3+:4I13/2 → 4I15/2 |
313–548 |
450/T2 |
This work |
LiYF4:Yb/Er/Ho |
980 |
Er3+:4I13/2 → 4I15/2 |
313–548 |
417/T2 |
LiYF4:Yb/Er/Ce |
980 |
Er3+:4I13/2 → 4I15/2 |
313–548 |
532/T2 |
In addition, the results of LIR repeatability over five heating–cooling cycles and 50 consecutive measurements of the LIR value at 333 K are shown in Fig. S14a and b.† The R values of LiYF4:18%Yb3+,3%Er3+, LiYF4:18%Yb3+,3%Er3+,0.2%Ho3+ and LiYF4:18%Yb3+,3%Er3+,2%Ce3+ were 99.7%, 99.6% and 99.7%, respectively, and the temperature uncertainty (δT) was 1.4 K, 1.3 K and 0.3 K, respectively. Once again, the SFPE was shown to have an excellent performance as a temperature sensor.
2.3.3 Temperature sensing in the NIR-IIa and NIR-IIb.
We tried to use the SFPE of Er3+ for non-thermal coupling temperature measurement. The emission spectra of the LiYF4:18%Yb3+,3%Er3+,0.2%Ho3+ nanospheres excited at 980 nm with temperature change are shown in Fig. 7a. As the temperature rose, the emission intensity of Ho3+ ion 5I6 level at 1200 nm in the NIR-IIa decreased, while the SFPE intensity of the Er3+ ion 4I13/2 level in the NIR-IIb remained stable and even increased slightly above 448 K. The intensity of the emission integrals is shown in Fig. 7b, and it indicated that as the temperature rose, the phonon vibration became active and participated in the Ho 5I6 → Er 4I13/240 energy transfer process, leading to an increase in the number of electrons in Er 4I13/2 and a decrease in the number of electrons in Ho 5I6. The two-part LIR was fitted using the non-thermal coupling formula: | 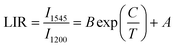 | (24) |
where I1545 and I1200 represent the emission intensity integrals of Er3+ and Ho3+. A, B and C are fitting parameters, as shown in Fig. 7c. A, B and C were 0.89554, 245.30728 and −2554.64363, respectively. As shown in Fig. 7d, the LiYF4:18%Yb3+,3%Er3+,0.2%Ho3+ nanospheres exhibited excellent Sr, reaching a maximum of 0.64% K−1 at 498 K (Table 3). The results of LIR repeatability over five heating–cooling cycles and 50 consecutive measurements of the LIR value at 498 K are shown in Fig. 7e and f, R = 99.8%, δT = 0.9 K. It was further demonstrated that the SFPE in the NIR-IIb offered excellent temperature readout performance in non-thermal coupling temperature measurement.
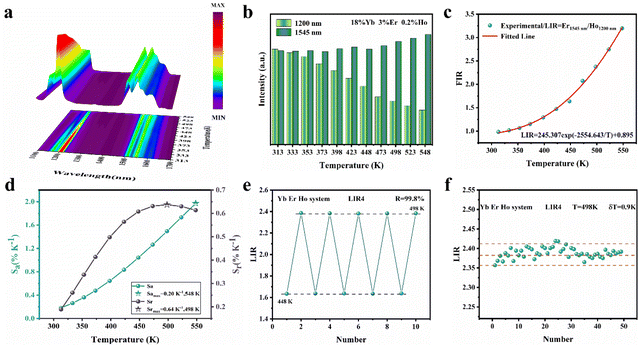 |
| Fig. 7 (a) Temperature-dependent UC emission spectra of LiYF4:18%Yb3+,3%Er3+,0.2%Ho3+ nanospheres under 980 nm excitation. (b) Summary of the integrated intensity of the emission of the thermally coupled levels in the LiYF4:18%Yb3+,3%Er3+,0.2%Ho3+ nanospheres at different temperatures. (c) LIR values as a function of temperature. (d) Sr/Sa-temperature curves of the LiYF4:18%Yb3+,3%Er3+,0.2%Ho3+ nanospheres. (e) LIR repeatability over five heating–cooling cycles. (f) LIR values measured 50 times continuously at 373 K. | |
Table 3 Comparison of the temperature measurement performance of different materials
Compound |
λ
ex (nm) |
λ
em (nm) |
T (K) |
S
r (%K−1) |
Ref. |
LaF3:Yb/Pr |
980 |
978/1302 |
15–105 |
0.525 |
41
|
YVO4:Yb/Er/Ho |
980 |
1194/1545 |
313–573 |
0.166 |
40
|
Y3Al5O12:Yb/Er/Ho |
980 |
1194/1545 |
313–573 |
0.467 |
40
|
BaTiO3:Yb/Er/Ho |
980 |
1194/1545 |
313–573 |
0.4 |
40
|
LiYF4:Yb/Er/Ho |
980 |
1200/1545 |
313–548 |
0.64 |
This work |
3. Conclusions
In summary, we have synthesized LiYF4:Ln3+ with well-dispersed micron octahedral cones and nanosphere morphologies via a one-step hydrothermal synthesis. When LiYF4 was singly doped with Er3+ ions, self-sensitized luminescence was accomplished under 381, 808 and 980 nm excitation. LiYF4:18%Yb3+,2%Er3+ exhibited ultra-efficient photoluminescence in the visible region, showing 2.06 times higher green emission than that of commercial phosphors (NaYF4:18%Yb3+,2%Er3+). The two green thermally coupled energy levels of Er3+ demonstrated high relative sensitivity (1107/T2). The transition of the 4I13/2 split Stark sublevel (S1–7) to the 4I15/2 split Stark sublevel (X1–8) of the Er3+ ion displayed SFPE in the NIR-IIb, and the relative sensitivity based on the thermal coupling Stark sublevel was 450/T2, which is higher than any previously reported relative sensitivity for Er3+ SFPE. Co-doping with Ce3+ ions to give LiYF4:18%Yb3+,2%Er3+,2%Ce3+ increased the NIR-IIb emission intensity by a factor of 3.07, and the relative sensitivity increased to 531/T2. When co-doped with Ho3+ ions (LiYF4:18%Yb3+,2%Er3+,0.2%Ho3+), during non-thermally coupled temperature measurements of the characteristic emission of Ho3+ ions in NIR-II and the SFPE of Er3+ ions in NIR-IIb, the relative sensitivity reached a maximum value of 0.64% K−1 at 498 K, demonstrating its excellent temperature measurement performance at both thermally coupled and non-thermally coupled energy levels and making LiYF4 a significant development in the field of bioimaging and nanomedicine.
4. Experimental section
LiF (A.R., Beijing Chemical Factory), NaF (A.R., Beijing Chemical Factory), LiNO3 (A.R., Beijing Chemical Factory), NH4F (G.R., Sinopharm Chemical Reagent Co., Ltd), C10H14N2O8Na2·2H2O (A.R., Aladdin), Y(NO3)3·6H2O (A.R, Aladdin), Yb(NO3)3·6H2O (A.R, Aladdin), Er(NO3)3·6H2O (A.R, Aladdin), Ho(NO3)3·6H2O (A.R, Aladdin), Ce(NO3)3·6H2O (A.R, Aladdin).
4.1 Synthesis of LiYF4:Ln3+ (Ln = Er, Yb/Er) microcrystals
Normally, Ln(NO3)3·6H2O (1 mmol) and LiF (4 mmol) were added in predetermined molar ratios to an empty beaker. Then, 10 ml deionized water was added dropwise, and the mixture was stirred continuously for 30 minutes to form a clear solution. NH4F (32 mmol) was then dissolved in 20 ml deionised water in another empty beaker and stirred for 30 minutes. The previously clear solution was then slowly added and stirred 30 minutes before the mixture was poured into a Teflon-lined stainless-steel autoclave and stored at 200 °C for 24 hours. Finally, the prepared micron crystals were washed with deionized water and ethanol and precipitated using centrifugation. These samples were dehydrated at 60 °C for 24 h.
4.2 Synthesis of LiYF4:Ln3+ (Ln = Yb/Er, Yb/Er/Ho, Yb/Er/Ce) nanocrystals
The differences were that we replaced the reactant LiF with LiNO3 (1 ml, 1 M), and the reaction time was adjusted to 6 h. Other processes were the same as above.
4.3 Synthesis of α-NaYF4:18%Yb3+,2%Er3+ phosphors
First, C10H14N2O8Na2·2H2O (0.1 g), Yb(NO3)3 (1.8 ml 0.1 M), Y(NO3)3 (8 ml 0.1 M), and Er(NO3)3 (0.2 ml 0.1 M) were added to 10 ml deionized water and stirred for 30 minutes. Subsequently, NaF (2 mmol) and NH4F (0.3 g) were added into the mixed solution, the pH was adjusted to 3, and the mixture was then stirred for 1 h. The mixture was then poured into a Teflon-lined stainless steel autoclave and stored at 120 °C for 24 hours. Finally, the α-NaYF4:18%Yb3+,2%Er3+ phosphors were washed with deionized water and ethanol and precipitated by centrifugation. These samples were dehydrated at 60 °C for 24 h.
4.4 Synthesis of β-NaYF4:18%Yb3+,2%Er3+ phosphors
The difference was that the reaction temperature was adjusted to 180 °C and the reaction time was 24 hours; the rest of the process was the same as that for α-NaYF4:18%Yb3+,2%Er3+.
4.4.1 Characterization.
An X-ray diffractometer (SmartLab SE, Japan) with Cu K radiation (λ = 0.15405 nm) was used for the crystalline X-ray diffraction (XRD) analysis of all samples. A field-emission scanning electron microscope (SEM) (Regulus 8100, Hitachi,) with an operating voltage of 10 kV and a working distance of 12.3 nm was utilized to explore the surface morphology of the synthesized samples. An Andor Shamrock SR-750 spectrometer with a 980 nm/808 nm variable power laser (∼15 W cm−2), 75 W Xe lamp and an SR830 DSP lock-in amplifier and CCD detector (DS3-21312-112, and DS3-51412-0309 BWT Beijing Ltd) was used to capture the emission spectra of the prepared materials. The NIR emission spectra were captured using an Andor SR-500i spectrometer (Andor Technology Co, Belfast, U.K.). A temperature control system (TAP-02, orient-KOJI) and copper-constant thermocouple were used to complete the temperature sensing studies. Fourier transform infrared (FT-IR) spectroscopy was performed using a FT-IR spectrometer (VERTEX 80, Bruker). Diffuse reflectance spectra were obtained using a UV–vis spectrometer (Shimadzu UV-2450 spectrometer).
Author contributions
Kejie Li and Hanyu Xu designed, analysed, and experiments. Kejie Li wrote and revised manuscripts. Zhiying Wang and Mengmeng Dai assisted in performance testing. Zuoling Fu provided revision guidance, suggestions and financial support.
Conflicts of interest
There are no conflicts to declare.
Acknowledgements
This research was supported by the National Science Foundation of China (Grant No. 12374374), the Key Projects of Jilin Province Science and Technology Development Plan (Grant No. 20230201060GX) and the Graduate Innovation Fund of Jilin University (Grant No. 2023CX043). The authors would like to acknowledge the Instrument and Equipment Sharing Platform, college of physics (Jilin University) for testing assistance.
References
- D. J. Naczynski, M. C. Tan, R. E. Riman and P. V. Moghe, Rare Earth Nanoprobes for Functional Biomolecular Imaging and Theranostics, J. Mater. Chem. B, 2014, 2, 2958–2973 RSC
.
- Y. Zhou, C. Li and Y. Wang, Crystal–Field Engineering Control of an Ultraviolet–Visible–Responsive Near–Infrared–Emitting Phosphor and Its Applications in Plant Growth, Night Vision, and NIR Spectroscopy Detection, Adv. Opt. Mater., 2022, 10, 2102246 CrossRef CAS
.
- E. Thimsen, B. Sadtler and M. Y. Berezin, Shortwave-infrared (SWIR) emitters for biological imaging: a review of challenges and opportunities, Nanophotonics, 2017, 6, 1043–1054 CrossRef CAS
.
- S. Yu, D. Tu, W. Lian, J. Xu and X. Chen, Lanthanide-doped near-infrared II luminescent nanoprobes for bioapplications, Sci. China Mater., 2019, 62, 1071–1086 CrossRef CAS
.
- L. Wu, M. Jia, D. Li and G. Chen, Shell Engineering on Thermal Sensitivity of Lifetime-Based NIR Nanothermometers for Accurate Temperature Measurement in Murine Internal Liver Organ, Nano Lett., 2023, 23, 2862–2869 CrossRef CAS PubMed
.
- A. Schroter, S. Märkl, N. Weitzel and T. Hirsch, Upconversion Nanocrystals with High Lanthanide Content: Luminescence Loss by Energy Migration versus Luminescence Enhancement by Increased NIR Absorption, Adv. Funct. Mater., 2022, 32, 2113065 CrossRef CAS
.
- S. Tsuboi, S. Yamada, Y. Nakane, T. Sakata, H. Yasuda and T. Jin, Critical Review—Water-Soluble Near-Infrared Fluorophores Emitting over 1000 nm and Their Application to In Vivo Imaging in the Second Optical Window (1000–1400 nm), ECS J. Solid State Sci. Technol., 2017, 7, R3093–R3101 CrossRef
.
- S. Xu, S. Xiang, Y. Zhang, J. Zhang, X. Li, J. Sun, L. Cheng and B. Chen, 808 nm laser induced photothermal effect on Sm3+/Nd3+ doped NaY(WO4)2 microstructures, Sens. Actuators, B, 2017, 240, 386–391 CrossRef CAS
.
- X. Jiang, C. Cao, W. Feng and F. Li, Nd3+-doped LiYF4 nanocrystals for bio-imaging in the second near-infrared window, J. Mater. Chem. B, 2016, 4, 87–95 RSC
.
- X. Wang, X. Zhang, Y. Wang, H. Li, J. Xie, T. Wei, Q. Huang, X. Xie, L. Huang and W. Huang, Comprehensive studies of the Li+ effect on NaYF4:Yb/Er nanocrystals: morphology, structure, and upconversion luminescence, Dalton Trans., 2017, 46, 8968–8974 RSC
.
- T. Y. O. G. Chen, A. Kachynski, H. Ågren and P. N. Prasad, Intense visible and near-infrared upconversion photoluminescence in colloidal LiYF4:Er3+ nanocrystals under excitation at 1490 nm, ACS Nano, 2011, 5, 4981–4986 CrossRef PubMed
.
- S. L. Maurizio, G. Tessitore, G. A. Mandl and J. A. Capobianco, Luminescence dynamics and enhancement of the UV and visible emissions of Tm3+ in LiYF4:Yb3+,Tm3+ upconverting nanoparticles, Nanoscale Adv., 2019, 1, 4492–4500 RSC
.
- F. Carl, L. Birk, B. Grauel, M. Pons, C. Würth, U. Resch-Genger and M. Haase, LiYF4:Yb/LiYF4 and LiYF4:Yb,Er/LiYF4 core/shell nanocrystals with luminescence decay times similar to YLF laser crystals and the upconversion quantum yield of the Yb,Er doped nanocrystals, Nano Res., 2020, 14, 797–806 CrossRef
.
- W. Li, Q. He, J. Xu, C. Shao, S. Sun, S. Fan and L. Hu, Efficient NIR to NIR up-conversion in LiYF4:Yb3+,Tm3+ micro-octahedrons by modified hydrothermal method, J. Lumin., 2020, 227, 117396 CrossRef CAS
.
- A. B. Andrade, G. F. C. Bispo, Z. S. Macedo and M. E. G. Valerio, Synthesis and characterization of luminescent Ln3+ (Ln = Eu, Tb and Dy)-doped LiYF4 microcrystals produced by a facile microwave-assisted hydrothermal method, J. Lumin., 2020, 219, 116843 CrossRef CAS
.
- Y. Song, R. Sun, G. Sun, Y. Xie and L. Sun, Upconversion/Downshifting Multimode Luminescence of Lanthanide-doped Nanocrystals for Multidimensional Information Encoding Security, Chem. – Asian J., 2022, 17(17), e202200537 CrossRef CAS PubMed
.
- Y. Song, M. Lu, G. Mandl, Y. Xie, G. Sun, J. Chen, X. Liu, J. A. Capobianco and L. N. Sun, Energy Migration Control of Multimodal Emissions in an Er3+ -Doped Nanostructure for Information Encryption and Deep-Learning Decoding, Angew. Chem., Int. Ed., 2021, 60, 23790–23796 CrossRef CAS PubMed
.
- S. Wang, J. Lin, Y. He, J. Chen, C. Yang, F. Huang and D. Chen, Remarkable laser-driven upconverting photothermal effect of Cs3LnF6@glass nanocomposites for anti-counterfeiting, Chem. Eng. J., 2020, 394, 124889 CrossRef CAS
.
- L. Yan, J. Huang, Z. An, Q. Zhang and B. Zhou, Activating Ultrahigh Thermoresponsive Upconversion in an Erbium Sublattice for Nanothermometry and Information Security, Nano Lett., 2022, 22, 7042–7048 CrossRef CAS PubMed
.
- Y. Xiao, X. Kuang, Y. Yeung and M. Ju, Investigation of the Structure and Luminescence Mechanism of Tm3+-Doped LiYF4: New Theoretical Perspectives, Inorg. Chem., 2020, 59, 1211–1217 CrossRef CAS PubMed
.
- Y. Xiao, M. Ju, H. Yuan and Y. Yeung, Unveiling the Local Structure and Luminescence Mechanism of Er3+-Doped LiYF4: A Promising Near-Infrared Laser Crystal, J. Phys. Chem. C, 2021, 125, 18015–18021 CrossRef CAS
.
- K. Zhang, B. Wang, T. Liu, H. An, S. Deng, Z. Hu, Y. Kong and Q. Zeng, Sc dopant induced tailoring of persistent luminescence in Na3YSi3O9:Eu2+ for information recording, J. Mater. Chem. C, 2022, 10, 15967–15980 RSC
.
- K. A. Denault, J. Brgoch, S. D. Kloss, M. W. Gaultois, J. Siewenie, K. Page and R. Seshadri, Average and local structure, debye temperature, and structural rigidity in some oxide compounds related to phosphor hosts, ACS Appl. Mater. Interfaces, 2015, 7(2), 7264–7272 CrossRef CAS PubMed
.
- L. E. Misiak and M. Subotowicz, Temperature EPR study of LiYl-xYbxF4(Gd3+) single crystals, Solid State Commun., 1991, 80, 761–766 CrossRef CAS
.
- S. Stojadinović, N. Tadić and R. Vasilić, Down-conversion photoluminescence of ZrO2:Er3+ coatings formed by plasma electrolytic oxidation, Mater. Lett., 2018, 219, 251–255 CrossRef
.
- N. Karayianis, Theoretical energy levels and g values for the 4I terms of Nd3+ and Er3+ in LiYF4, J. Phys. Chem. Solids, 1971, 32, 2385–2391 CrossRef CAS
.
- M. D. Dramicanin, L. Marciniak, S. Kuzman, W. Piotrowski, Z. Ristic, J. Perisa, I. Evans, J. Mitric, V. Dordevic, N. Romcevic, M. G. Brik and C. G. Ma, Mn5+-activated Ca6Ba(PO4)4O near-infrared phosphor and its application in luminescence thermometry, Light: Sci. Appl., 2022, 11, 279 CrossRef CAS PubMed
.
- R. An, Y. Liang, R. Deng, P. Lei and H. Zhang, Hollow nanoparticles synthesized via Ostwald ripening and their upconversion luminescence-mediated Boltzmann thermometry over a wide temperature range, Light: Sci. Appl., 2022, 11, 217 CrossRef CAS PubMed
.
- K. Zhu, Z. Wang, H. Xu and Z. Fu, Development of Multifunctional Materials Based on Heavy Concentration Er3+–Activated Lead–free Double Perovskite Cs2NaBiCl6, Adv. Opt. Mater., 2022, 10, 2201182 CrossRef CAS
.
- M. Jia, Z. Sun, M. Zhang, H. Xu and Z. Fu, What determines the performance of lanthanide-based ratiometric nanothermometers?, Nanoscale, 2020, 12, 20776–20785 RSC
.
- S. Zhou, K. Deng, X. Wei, G. Jiang, C. Duan, Y. Chen and M. Yin, Upconversion luminescence of NaYF4: Yb3+, Er3+ for temperature sensing, Opt. Commun., 2013, 291, 138–142 CrossRef CAS
.
- H. Zhang, X. Dong, L. Jiang, Y. Yang, X. Cheng and H. Zhao, Comparative analysis of upconversion emission of LaF3:Er/Yb and LaOF:Er/Yb for temperature sensing, J. Mol. Struct., 2020, 1206, 127665 CrossRef CAS
.
- X. Cheng, K. Yang, J. Wang, L. Yang and X. Cheng, Up-conversion luminescence and optical temperature sensing behaviour of Yb3+/Er3+ codoped CaWO4 material, Opt. Mater., 2016, 58, 449–453 CrossRef CAS
.
- S. K. Singh, K. Kumar and S. B. Rai, Er3+/Yb3+ codoped Gd2O3 nano-phosphor for optical thermometry, Sens. Actuators, A, 2009, 149, 16–20 CrossRef CAS
.
- G. Xiang, X. Liu, Q. Xia, X. Liu, S. Xu, S. Jiang, X. Zhou, L. Li, D. Wu, L. Ma, X. Wang and J. Zhang, Design of a bi-functional NaScF4: Yb3+/Er3+ nanoparticles for deep-tissue bioimaging and optical thermometry through Mn2+ doping, Talanta, 2021, 224, 121832 CrossRef CAS PubMed
.
- R. Lei, X. Liu, F. Huang, D. Deng, S. Zhao, H. Xu and S. Xu, Optical thermometry based on anomalous temperature-dependent 1.53 μm infrared luminescence of Er3+ in BaMoO4: Er3+/Yb3+ phosphor, Opt. Mater., 2018, 86, 278–285 CrossRef CAS
.
- B. Cao, J. Wu, X. Wang, Y. He, Z. Feng and B. Dong, Multiple Temperature-Sensing Behavior of Green and Red Upconversion Emissions from Stark Sublevels of Er3+, Sensors, 2015, 15, 30981–30990 CrossRef CAS PubMed
.
- Y. Shang, Q. Han, S. Hao, T. Chen, Y. Zhu, Z. Wang and C. Yang, Dual-Mode Upconversion Nanoprobe Enables Broad-Range Thermometry from Cryogenic to Room Temperature, ACS Appl. Mater. Interfaces, 2019, 11, 42455–42461 CrossRef CAS PubMed
.
- M. Quintanilla, Y. Zhang and L. M. Liz-Marzán, Subtissue Plasmonic Heating Monitored with CaF2:Nd3+,Y3+ Nanothermometers in the Second Biological Window, Chem. Mater., 2018, 30, 2819–2828 CrossRef CAS
.
- M. Jia, Z. Fu, G. Liu, Z. Sun, P. Li, A. Zhang, F. Lin, B. Hou and G. Chen, NIR–II/III Luminescence Ratiometric Nanothermometry with Phonon–Tuned Sensitivity, Adv. Opt. Mater., 2020, 8, 1901173 CrossRef CAS
.
- A. M. Kaczmarek, M. K. Kaczmarek and R. Van Deun, Er3+-to-Yb3+ and Pr3+-to-Yb3+ energy transfer for highly efficient near-infrared cryogenic optical temperature sensing, Nanoscale, 2019, 11, 833–837 RSC
.
|
This journal is © the Partner Organisations 2024 |