DOI:
10.1039/D4PY00380B
(Paper)
Polym. Chem., 2024,
15, 2840-2848
Enhancement in the initiating activity of chalcones via a long-alkyl chain strategy for free radical photopolymerization and 3D printing†
Received
8th April 2024
, Accepted 27th May 2024
First published on 31st May 2024
Abstract
A novel chalcone-based derivative, E-PHMTO, equipped with an alkyl chain and chemically similar to the commercial photoinitiator ITX, was successfully synthesized. Compared to the intermediate reaction products E/Z-HMTO, the introduction of an alkyl chain to the chalcone group shifts the focus of E-PHMTO towards proton transfer rather than isomeric side reactions, resulting in an LED-sensitive photoinitiator with excellent initiating performance through steric hindrance. Furthermore, E-PHMTO demonstrated superior initiating activity compared to ITX under LED@385 nm irradiation and proved to possess outstanding polymerization properties suitable for 3D photo-printing applications. This research might offer a versatile approach for the convenient design and preparation of highly efficient, LED-sensitive chalcone photoinitiators.
1. Introduction
Over the past few decades, photo-induced polymerization, also known as photopolymerization,1–3 has garnered significant interest as an alternative to traditional thermal polymerization, due to its unique advantages such as high efficiency, the absence of solvents, and excellently spatiotemporal control. Consequently, the photopolymerization technique has been extensively applied across various industrial applications, including coatings,4 lithography,5 and 3D/4D printing.6 With increasing emphasis on environmental protection, there has been a shift towards using visible light illumination instead of traditional UV mercury lamps for photopolymerization in recent years, attributed to the former's benefits including low energy consumption, user comfort, safety, and ozone-free nature.7–9 Photoinitiators (PIs) absorb light under irradiation, producing radicals essential for polymer formation, thus playing a pivotal role in photopolymerization systems. Notably, visible light-induced polymerization10–13 necessitates PIs that have long absorption wavelengths in the near-UV and visible light regions. However, traditional UV PIs are incompatible with visible light photopolymerization, making the development of visible light PIs both essential and urgent.14–16
To date, chalcone PIs have been identified as among the most promising candidates for initiating free radical photopolymerization due to their simple synthesis through a one-step aldehyde–ketone condensation reaction.17–21 Furthermore, chalcone PIs can be readily modified with a variety of heterocycles, and electron donors and acceptors, enabling their conjugation degree to align well with the long emission wavelength of visible light sources.22,23 Additionally, the low toxicity and eco-friendliness of chalcones have been confirmed, significantly advancing the application of photopolymerization in biological fields.24,25 However, chalcone PIs are prone to side photochemical reactions, such as reversible isomerization or dimerization,26,27 during polymerization due to their rotatable C
C bonds, which result in a lower initiating efficiency compared to commercial PIs. A universal strategy to mitigate these side reactions and enhance the performance of chalcone PIs is imperative.
Our group initially explored the use of additives to restrict the movement of C
C bonds adjacent to carbonyl groups in chalcones, aiming to enhance their photoinitiating efficiency. We discovered that the co-initiator triethanolamine (TEOA) could form intermolecular interactions with chalcones. For instance, the presence of a charge transfer complex (CTC) between (2E,6E)-2,6-bis(furan-2-ylmethylidene) (BFC)/TEOA was confirmed.28 Additionally, intermolecular hydrogen bonding was observed with (2E,6E)-2,6-bis((1H-pyrrol-2-yl)methylene)cyclohexan-1-one (BPC)/TEOA.29 These weak bonds prevent the chalcone PIs from isomerizing, yet they do not elevate the performance of chalcones to the theoretical maximum. Consequently, there remains an efficiency gap between the chalcone/TEOA initiating systems and commercial PIs.
To further enhance the activity of chalcones for free radical photopolymerization, an alkyl chain from 5-bromopent-1-ene was successfully attached to the chalcone PI (E)-3-((1H-pyrrol-2-yl)methylene)thiochroman-4-one (E-HMTO) to limit its isomerization reaction. Consequently, a novel one-component LED-sensitive chalcone PI, (E)-3-((1-(pent-4-en-1-yl)-1H-pyrrol-2-yl) methylene)thiochroman-4-one (E-PHMTO), was synthesized. To validate the effectiveness of this alkyl chalcone modification, the optical properties, isomerization capabilities, and photoinitiating abilities of both E-HMTO and E-PHMTO were evaluated through absorption spectroscopy, photolysis experiments, theoretical calculations, and polymerization kinetics. Furthermore, the commercial PI ITX was chosen for comparison with E-PHMTO. Photoprinting experiments were also conducted to assess E-PHMTO's potential for additive manufacturing.
2. Experimental section
2.1. Materials
2-Pyrrolidine, 5-bromo-1-pentene and thiochroman-4-one were purchased from Energy Chemical (Shanghai, China). NaOH, NaH and the solvents ethyl alcohol, acetonitrile, DMF, and DMSO-d6 were purchased from Heowns (Tianjin, China). The commercial PI isopropyl thioxanthrone (ITX), coinitiator triethylamine (TEA) and the purified monomer 1,6-hexadiol diacrylate (HDDA) were supplied by Jiangsu Jicui Photosensitive Electronic Materials Research Institute Limited Company (Jiangsu, China). The synthesis route and chemical structures of the monomers and PIs are shown in Scheme 1.
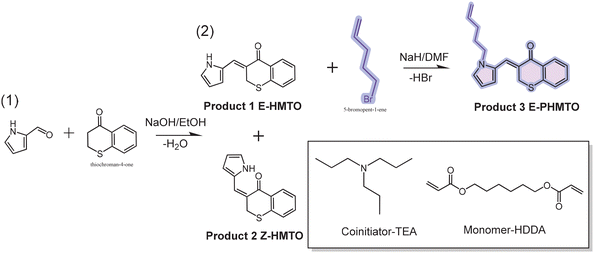 |
| Scheme 1 Synthetic route for compounds E-HMTO, Z-HMTO, and E-PHMTP, and coinitiator TEA and monomer HDDA used in this research. | |
2.2. Synthetic route to chalcone based derivatives
(E)-3-((1H-Pyrrol-2-yl)methylene)thiochroman-4-one (E-HMTO).
2-Pyrrolidine (2 mmol, 0.19 g), sodium hydroxide (2 mmol, 0.08 g), thiosermann-4-one (2 mmol, 0.324 g) and ethanol (10 ml) were added into a 25 mL two-necked round-bottom flask, and the flask was vacuumed and purged with dry nitrogen three times. Then the mixture was stirred at room temperature for about 2–3 h and TLC was used as a tool for the detection of the reaction process. When the raw material point disappeared, stirring was stopped. The organic phase was evaporated under reduced pressure. The crude product was purified by column chromatography on silica gel. 1H NMR (400 MHz, DMSO-d6)δ 11.66 (s, 1H), 8.01–7.99 (d, J = 8 Hz, 1H), 7.62 (s, 1H), 7.49–7.45 (t, J = 8 Hz, 1H), 7.41–7.39 (d, J = 8 Hz, 1H), 7.32–7.28 (t, J = 8 Hz, 1H), 7.15 (s, 1H), 6.82 (s, 1H), 6.32 (s, 1H), 4.29 (s, 1H). HRMS (ESI) calculated for C14H12NOS [M + H]+: 242.0595; found: 242.1518; elemental analysis calculated for C14H11NOS: C, 69.68; H, 4.59; N, 5.80; O, 6.63; S, 13.29; found: C, 70.38; H, 4.81; N, 5.56; O, 6.45; S, 12.78.
(Z)-3-((1H-Pyrrol-2-yl)methylene)thiochroman-4-one (Z-HMTO).
The Z-isomer was also obtained from the synthetic route for (E)-3-((1H-pyrrol-2-yl)methylene)thiochroman-4-one. 1H NMR (400 MHz, DMSO-d6)δ 12.29 (s, 1H), 8.15–8.13 (d, J = 8 Hz, 1H), 7.48–7.39 (m, 2H), 7.32–7.29 (t, J = 4 Hz, 1H), 7.28–7.26 (m, 1H), 7.19–7.12 (d, J = 28 Hz, 2H), 6.74 (s, 1H), 6.30 (s, 1H), 4.05 (s, 1H). HRMS (ESI) calculated for C14H12NOS [M + H]+: 242.0595; found: 242.0623; elemental analysis calculated for C14H11NOS: C, 69.68; H, 4.59; N, 5.80; O, 6.63; S, 13.29; found: C, 69.55; H, 4.71; N, 5.66; O, 6.55; S, 13.52.
(E)-3-((1-(Pent-4-en-1-yl)-1H-pyrrol-2-yl)methylene)thiochroman-4-one (E-PHMTO).
E-HMTO (2 mmol, 0.48 g), sodium hydride (2 mmol, 0.048 g), and 5-bromo-1-pentene (0.02 mol, 0.296 g) were added into a 25 mL two-necked round-bottom flask, and the flask was vacuumed and purged with dry nitrogen three times. Then the mixture was stirred at 0 °C for about 5–8 h and TLC was used as a tool for the detection of the reaction process. The reaction mixture was quenched by H2O and the organic phase was extracted with ethyl acetate (3 × 20 mL). The mixed organic layer was washed with salt water. The organic layer was dried with anhydrous Na2SO4. The combined organic layers were filtered and the solvent was removed under reduced pressure. The crude product was purified by silica gel column chromatography. 1H NMR (400 MHz, DMSO-d6)δ 8.04–8.02 (s, 1H), 7.77–7.55 (m, 2H), 7.44–7.24 (m, 2H), 7.12 (s, 1H), 6.71–6.70 (d, J = 4 Hz, 1H), 6.26–6.24 (d, J = 4 Hz, 1H), 5.97–5.62 (m, 2H), 5.00–4.84 (m, 2H), 4.28 (s, 1H), 4.06–4.03 (t, J = 4 Hz, 1H), 3.87–3.72 (m, 1H), 1.97–1.58 (m, 2H). HRMS (ESI) calculated for C19H19NOS [M + H]+: 310.1221; found: 310.1262; elemental analysis calculated for C19H18NOS: C, 73.75; H, 6.19; N, 4.53; O, 5.17; S, 10.36; found: C, 73.55; H, 6.28; N, 4.33; O, 5.18; S, 10.65.
2.3. Computational studies
All the calculations were performed using the Gaussian16 package. The geometries and HOMO–LUMO orbitals were optimized by the B3LYP functional with the 6-311G** basis set for all atoms. Vibration frequency analysis at the same level was carried out to check the stationary points or transition states as minima or saddle points, and to obtain the thermodynamic correction to Gibbs free energies.30,31
2.4. Characterization
UV–vis absorption spectra were recorded using a GENESYS150 (Thermo Fisher Scientific, American) (1 cm path length). 1H-NMR spectra were collected with an AVANCE III spectrometer (Bruker, Germany) operating at 400 MHz using DMSO-d6 as the solvent and tetramethyl silane as an internal reference.
2.5. Steady state photolysis study
Steady state photolysis studies were performed in the solvent of acetonitrile upon the irradiation with LED@405 nm combined with LED@465 nm at a light intensity of 25 mW cm−2 without air (room temperature). To obtain the same absorbance for the control experiment, the concentrations of E-HMTO, Z-HMTO, and E-PHMTO in acetonitrile were set as 6.2 × 10−5 mol L−1, 6.2 × 10−4 mol L−1, and 1.3 × 10−4 mol L−1, respectively.
2.6. Light sources for photolysis and photopolymerization
LED lamps with the emission wavelength centered at 385 nm, 405 nm, and 465 nm were purchased from LAMPLIC (Shenzhen, China). The incident light intensity was recorded by radiometers (Photoelectric Instrument Factory of the Beijing Normal University).
2.7. Photopolymerization kinetics
A real-time Fourier transform IR (FT-IR) spectrometer (Nicolet 5700) equipped with an MCT/A KBr detector–beam splitter combination (650–4000 cm−1, 4 scans per s) was used to monitor the photopolymerization kinetics. Mixtures of photoinitiating systems stirred for 10 min including E-PHMTO (1 wt%, 0.5 wt%, 0.1 wt%, 0.05 wt%), E,Z-HMTO (0.1 wt%)/TEA (1 wt%), commercial photoinitiator ITX (1 wt%)/TEA (1 wt%) and monomer HDDA were dropped onto a piece of KBr crystal with a thickness of 2 ± 0.1 mm, covered by another clear piece of KBr crystal and then exposed to the light source LED@385 nm or LED@405 nm with a light intensity of 70 mW cm−2 in the absence of air. The double bond conversion of the monomer HDDA was calculated based on the decrease in the area of the acrylate absorption peak at 800–850 cm−1 using the following equation:
where At corresponds to the area of the absorbance peak area of acrylate at 800–850 cm−1 at time t during the polymerization and A0 corresponds to the initial area of the absorbance peak before the polymerization.24
2.8. 3D printing
The nanoArch P150 DLP 3D printing machine fabricated by BOSTON MICRO FABRICATION was used. The print recipe comprised the monomer HDDA with the PI E-PHMTO (0.1 wt%). The resolution was 1080 DPI. The intensity of irradiation light power was 15 mW cm−2, recorded by radiometers. Meanwhile, the printing speed was achieved by printing each 20 μm thick layer in 10 s considering E-PHMTO's polymerization kinetics. The post-curing was conducted with mercury lamp irradiation for 1 h in alcohol.
3. Results and discussion
3.1. Absorption properties
In this study, three compounds were synthesized. E-HMTO and Z-HMTO were isolated from the aldehyde–ketone condensation products, while E-PHMTO was synthesized via a secondary substitution reaction of E-HMTO with 5-bromo-1-pentene. Owing to the isomeric side reactions caused by the double bond adjacent to the carbonyl group and the photochemical inertness of the cis-isomer, as indicated in related chalcone studies, E/Z-HMTO are generally regarded as less efficient photoinitiators. Conversely, E-PHMTO, designed as a one-component photoinitiator, demonstrates enhanced initiating efficiency. This improvement is attributed to the steric effect of its long alkyl chain, which mitigates cis–trans isomerism.
To verify the aforementioned hypothesis, the absorption properties of E-PHMTO and E/Z-HMTO were initially examined and are presented Fig. 1. Both E-PHMTO and E-HMTO exhibit a narrow absorption band due to the π–π* transition, spanning from 300 to 450 nm in the near-UV and blue light region, which corresponds to their close energy gaps of 3.69 eV and 3.66 eV (Fig. 2). This suggests their suitability as LED-sensitive photoinitiators. Furthermore, the addition of an alkyl chain has indeed reduced the absorptivity of the chalcone's primary molecular skeleton. Nonetheless, the molar extinction coefficients of E-HMTO at ε365 nm, ε385 nm, and ε405 nm remain high, recorded at 7415 M−1 cm−1, 8328 M−1 cm−1, and 7729 M−1 cm−1 respectively, surpassing even that of isopropyl thioxanthrone (ITX) at these wavelengths. Additionally, the cis-isomer Z-HMTO showed a red-shifted absorption, with its maximum εmax being less than 3000 M−1 cm−1.
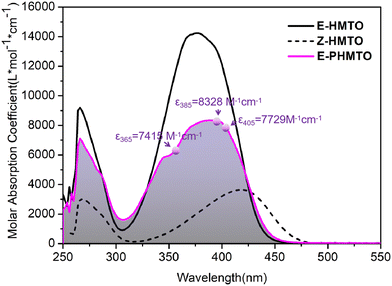 |
| Fig. 1 UV-vis absorption spectra of compounds E-HMTO, Z-HMTO and E-PHMTO in acetonitrile solutions. | |
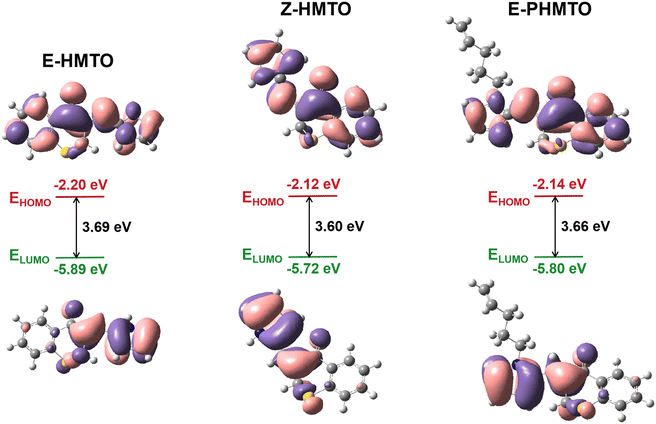 |
| Fig. 2 Illustration of the frontier molecular orbitals (LUMOs and HOMOs) determined at the B3LYP/6-311G** level of theory. | |
3.2. Photochemical properties
To further elucidate the initiating mechanism of these three compounds in free radical photopolymerization, steady-state photolysis experiments were conducted. The photolysis of E-HMTO, both in the presence and absence of the common co-initiator triethylamine (TEA), was investigated. In the absence of TEA, E-HMTO exhibited photostability, undergoing photo-isomerization rather than photolysis under irradiation. As observed in Fig. 3(a), the maximum absorption peak of E-HMTO shifted from 375 nm to 410 nm with increasing irradiation time under LED@405 nm irradiation at room temperature. This shift was reversed to some extent under subsequent LED@465 nm irradiation, indicating trans → cis and cis → trans photo-isomerization of E/Z-HMTO, respectively, consistent with real-time 1H-NMR photolysis results. As illustrated in Fig. 4(a), the H1–5 signals, with chemical shifts of 11.65 ppm, 7.66 ppm, 7.15 ppm, 6.80 ppm, and 8.00 ppm, respectively, diminished while new H signals H1′–5′, with chemical shifts of 12.19 ppm, 7.19 ppm, 7.12 ppm, 6.75 ppm, and 8.15 ppm, respectively, emerged after 10 minutes of LED@405 nm irradiation of E-HMTO alone. These H1′–5′ signals disappeared, and the H1–5 signals reappeared after subsequent LED@465 nm irradiation. As TEA was added to E-HMTO's initiating system, photolysis was evident, yet E-HMTO still underwent trans → cis isomerization within the first 10 s of LED@405 nm irradiation. Additionally, the absorbance at 373 nm significantly increased, nearly returning to its initial state under LED@465 nm irradiation, suggesting incomplete decomposition of E-HMTO and its predominant conversion to Z-HMTO. This conversion adversely affected the hydrogen abstraction reaction with TEA, leading to low initiating efficiency. Photolysis results of Z-HMTO alone and Z-HMTO/TEA are displayed in Fig. S8 and S9,† respectively, revealing larger absorption shifts but less photolysis than shown by the E-HMTO and E-HMTO/TEA initiating systems. Conversely, the photolysis behavior of E-PHMTO, both with and without TEOA, showed improvement. There was virtually no shift in the absorption band of E-PHMTO (Fig. 3(c)) and E-PHMTO/TEA (Fig. 3(d)) in any direction. This highlighted the advantageous properties of E-PHMTO concentrating on proton transfer during the photopolymerization, corresponding to E-PHMTO showing significant degradation instead of converting into Z-PHMTO over an irradiation period of 60 s.
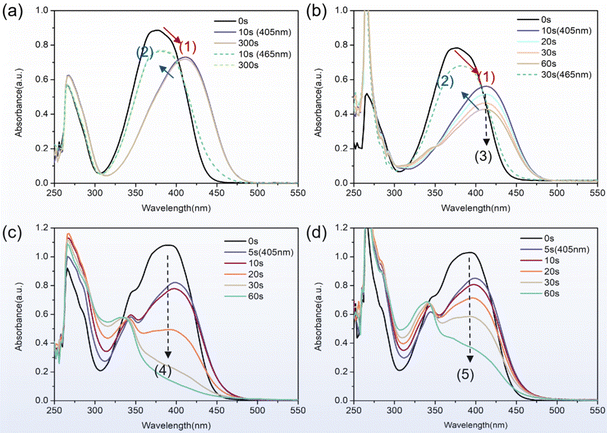 |
| Fig. 3 Steady state photolysis experiments of E-HMTO (6.2 × 10−5 mol L−1) in the (a) absence and (b) presence of the coinitiator TEA; steady state photolysis experiments of E-PHMTO (1.3 × 10−4 mol L−1) in the (c) absence and (d) presence of the coinitiator TEA. | |
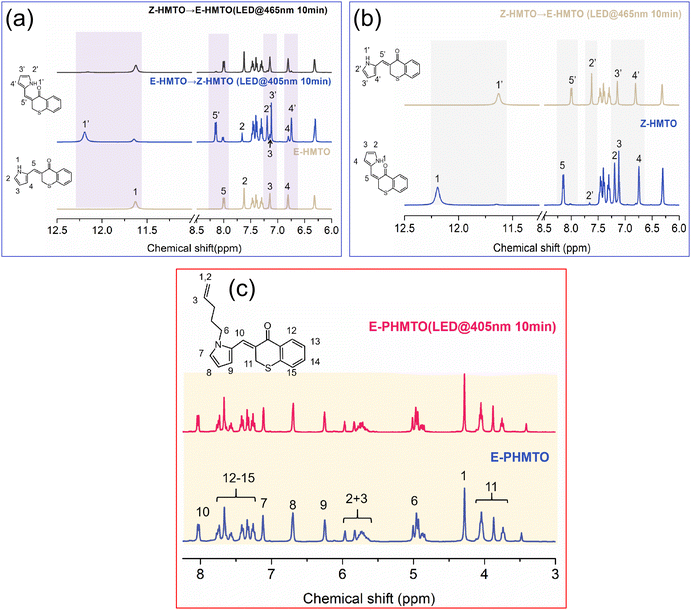 |
| Fig. 4 (a) 1H-NMR spectra of E-HMTO under LED@405 nm and LED@465 nm irradiation (100 mW cm−2) in the solvent of DMSO-d6 at different time points; (b) 1H-NMR spectrum of E-HMTO under LED@465 nm irradiation; (c) 1H-NMR spectrum of E-PHMTO under LED@405 nm irradiation within 10 min. | |
Real-time 1H-NMR spectrum results (Fig. 4(c)) for E-PHMTP before and after LED@405 nm irradiation for 10 min indicated that no trans → cis photo-isomerization occurred due to the absence of new hydrogen signals. Importantly, this finding revealed that the alkyl chain substituted on the pyrrole ring effectively acts as a donor, accelerating hydrogen abstraction and thus endowing E-PHMTO with significant potential as a one-component LED-sensitive photoinitiator. The proposed photochemical mechanism of E-HMTO and E-PHMTO in the presence and absence of TEA is shown in Scheme 2.
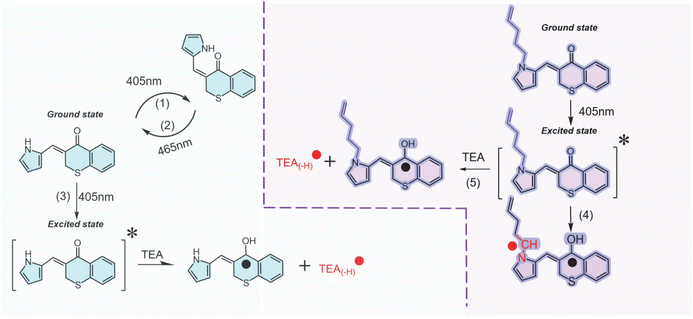 |
| Scheme 2 Proposed photochemical mechanism of E-HMTO and E-PHMTO in the presence and absence of TEA coresponding to photolysis results in Fig. 3. | |
Furthermore, all photolysis results indicated that introducing an alkyl chain onto the pyrrole ring generates a steric effect that restricts photo-isomerism, thereby promoting the photo-degradation reaction. To better understand the steric effect induced by alkyl chains, density functional theory (DFT) was utilized to assess the impact of incorporating a long alkyl chain on the rotation of the double bond near the carbonyl group. As illustrated in Fig. 5, the intrinsic reaction coordinate (IRC) calculation of the transition state (TS) smoothly transitions to the reactants with or without the addition of a long alkyl chain. It demonstrated that the energy barrier increases from 59.0 kcal mol−1 to 65.1 kcal mol−1 with the introduction of a long alkyl chain, indicating that long alkyl chains can inhibit the movement of the double bond,32,33 ultimately contributing to E-PHMTO exhibiting a reduced trans → cis isomerism conversion.
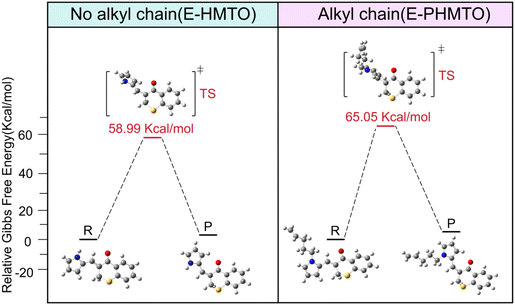 |
| Fig. 5 Energy profiles for the photochemical trans → cis isomerization of E-HMTO and E-PHMTO. | |
3.3. Photopolymerization kinetics
Considering the initiating performance of E/Z-HMTO and E-PHMTO for free radical photopolymerization, the photopolymerization kinetics of HDDA used as a monomer with these compounds serving as PIs under LED@405 nm (70 mW cm−2) irradiation were analyzed by FT-IR. Specifically taking E-PHMTO as an example, the final double bond conversion rates of HDDA during the polymerization were 85%, 90%, 95%, and 80%, respectively, as E-PHMTO's concentration decreased from 1 wt% to 0.05 wt% (Fig. 6(a)). This demonstrates that reducing the concentration of E-PHMTO prior to irradiation could enhance its initiating ability. Therefore, the optimal double bond conversion and initiation rate for E-PHMTO were observed at a concentration condition of 0.1 wt%, suggesting that at this concentration, the optical shielding effect of E-PHMTO is sufficiently minimized to facilitate its photochemical reaction. However, at a concentration of 0.05 wt%, the generation of free radicals by E-PHMTO was inadequate, leading to a slight decrease in the polymerization rate. As shown in Fig. 6(b), TEA (1 wt%) was utilized as a hydrogen donor alongside the PIs (0.1 wt%). The final double bond conversions of HDDA initiated by E-PHMTO/TEA, E-HMTO/TEA, and Z-HMTO/TEA were 73%, 70%, and 5%, respectively. On the one hand, the initiating performance of E-PHMTO/TEA was less effective compared to E-PHMTO alone under identical illumination conditions, demonstrating E-PHMTO's high efficiency as an LED-sensitive PI and its poor compatibility with the co-initiator TEA. On the other hand, the initiating efficiency of E-HMTO/TEA and Z-HMTO/TEA corresponded with their inconspicuous photolysis experiment outcomes. This seemed to demonstrate that the photo-initiating ability of E-PHMTO/TEA was lower than that of E-PHMTO alone, in agreement with the photolysis results. This phenomenon might be a result of TEA inhibiting the polymerization.34
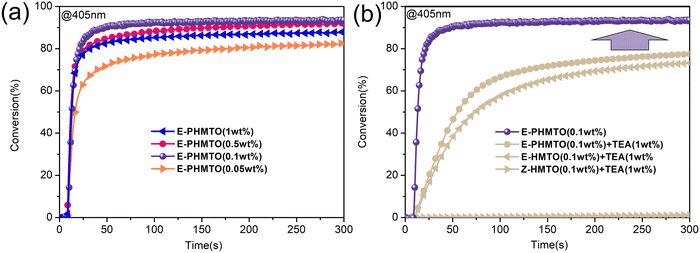 |
| Fig. 6 (a) Kinetics profiles of monomer HDDA initiated by E-PHMTO under various concentration conditions without co-initiators upon LED@405 nm (70 mW cm−2) irradiation; (b) kinetics profiles of monomer HDDA initiated by different initiating systems: E-PHMTO, E-PHMTO/TEA, E-HMTO/TEA, and Z-HMTO/TEA upon LED@405 nm (70 mW cm−2) irradiation. | |
Finally, the commercial PI ITX was chosen for comparison with our designed PI, E-PHMTO, due to their similar chemical structures involving thioxanthone and absorption wavelengths at 385 nm and 405 nm. To our knowledge, ITX typically forms a two-component system with the additive TEA to initiate polymerization under irradiation from the optimally matched light source LED@385 nm. Notably, the final double bond conversion of HDDA initiated by E-PHMTO alone is significantly higher than that achieved by ITX in the presence of TEA at various concentrations (1 wt%, 0.5 wt%, 0.1 wt%) under LED@385 nm illumination (Fig. 7). Therefore, our designed PI, E-PHMTO, indeed possesses superior initiating capability compared to the commercial PI ITX under this illumination condition.
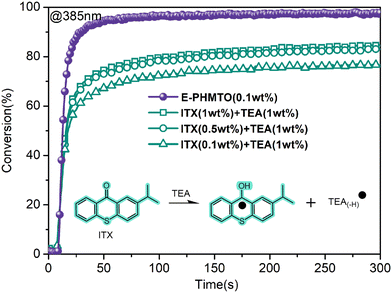 |
| Fig. 7 Kinetics profiles of monomer HDDA initiated by E-PHMTO and initiation system ITX/TEA followed by FT-IR. | |
3.4. 3D printing
Based on E-PHMTO's high efficiency in free radical photopolymerization, a DLP 3D printer with LED@405 nm (15 mW cm−2) irradiation was utilized to fabricate a 3D model of a bird's nest within a brief irradiation period. Optical microscopy was employed to assess the model's resolution ratio. The 3D printing test, conducted in the presence of air using the E-PHMTO/HDDA system, demonstrated that the model exhibits excellent photobleaching properties and high spatial resolution, with a minimum thickness of 0.5 mm. This underscores the high photo-sensitivity of the proposed printing system (Fig. 8).
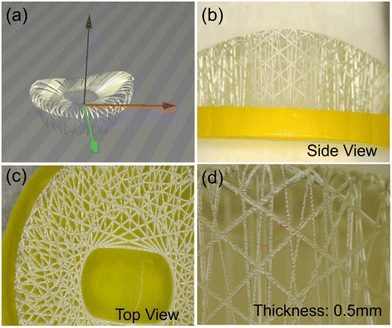 |
| Fig. 8 (a) STL file of a bird's nest for 3D printing; (b)–(d) the bird's nest model produced using the E-PHMTO/HDDA system. | |
4. Conclusion
In summary, the innovative addition of an alkyl chain to the electron donor segment of the chalcone PI E-HMTO, which features a D–π-A chemical structure, successfully inhibits its trans → cis photoisomerization. This modification results in the creation of a novel one-component chalcone PI, E-PHMTO, characterized by high initiating efficiency, that demonstrates significantly enhanced photolysis properties and superior initiating performance under LED@405 nm irradiation, even in the absence of a co-initiator and compared to E-HMTO/TEA. Additionally, E-PHMTO exhibits exceptional initiating activity compared to the commercial PI ITX under LED@385 nm irradiation and proves to be highly effective in DLP 3D printing applications. The strategy of introducing an alkyl chain to induce a steric effect that mitigates side isomerism reactions in chalcone PIs is both universal and sustainable, offering a valuable approach for designing highly effective chalcone PIs.
Author contributions
Jingfang Li: Conceptualization, methodology, software, data curation, writing – original draft, funding acquisition; Qilu Deng: Methodology, investigation; Xianju Zhou: Data curation; Wenjie Li: Methodology, investigation; Xiaoqun Zhu: Supervision; Jun Nie: Supervision.
Conflicts of interest
The authors declare that they have no known competing financial interests or personal relationships that could have appeared to influence the work reported in this paper.
Acknowledgements
This work was supported by the Science and Technology Research Program of Chongqing Municipal Education Commission (Grant No. KJQN202200631). The authors also appreciate the support from Jiangsu Jicui Photosensitive Electronic Materials Research Institute Limited Company.
References
- N. Corrigan, J. Yeow, P. Judzewitsch, J. Xu and C. Boyer, Angew. Chem., Int. Ed., 2019, 58, 5170–5189 CrossRef CAS PubMed.
- C. Dietlin, S. Schweizer, P. Xiao, J. Zhang, F. Morlet-Savary, B. Graff, J.-P. Fouassier and J. Lalevée, Polym. Chem., 2015, 6, 3895–3912 RSC.
- H.-W. Pei, K. Ye, Y. Shao, D. Chen, Z.-Y. Sun, T. Gong, D. Liu and K. Sun, Polym. Chem., 2024, 15, 248–268 RSC.
- Y. Zhu, L. Li, Y. Zhang, Y. Ou, J. Zhang, Y. Yagci and R. Liu, Prog. Org. Coat., 2023, 174, 107272 CrossRef CAS.
- N. D. Dolinski, Z. A. Page, E. B. Callaway, F. Eisenreich, R. V. Garcia, R. Chavez, D. P. Bothman, S. Hecht, F. W. Zok and C. J. Hawker, Adv. Mater., 2018, 30, 1800364 CrossRef PubMed.
- V. Hahn, T. Messer, N. M. Bojanowski, E. R. Curticean, I. Wacker, R. R. Schröder, E. Blasco and M. Wegener, Nat. Photonics, 2021, 15, 932–938 CrossRef CAS.
- Z. Li, X. Zou, F. Shi, R. Liu and Y. Yagci, Nat. Commun., 2019, 10, 3560 CrossRef PubMed.
- Y. Zhu, D. Xu, Y. Zhang, Y. Zhou, Y. Yagci and R. Liu, Angew. Chem., Int. Ed., 2021, 60, 16917–16921 CrossRef CAS PubMed.
- C. Wang, X. Meng, Z. Li, M. Li, M. Jin, R. Liu and Y. Yagci, ACS Macro Lett., 2020, 9, 471–475 CrossRef CAS PubMed.
- L. Deng and J. Qu, Prog. Org. Coat., 2023, 183, 107766 CrossRef CAS.
- J. P. Fouassier, X. Allonas and D. Burget, Prog. Org. Coat., 2003, 47, 16–36 CrossRef CAS.
- K. Kaya, J. Kreutzer and Y. Yagci, ChemPhotoChem, 2019, 3, 1187–1192 CrossRef CAS.
- A. Gallastegui, A. Dominguez-Alfaro, L. Lezama, N. Alegret, M. Prato, M. L. Gómez and D. Mecerreyes, ACS Macro Lett., 2022, 11, 303–309 CrossRef CAS PubMed.
- M. Abdallah, A. Hijazi, P. G. Cozzi, A. Gualandi, F. Dumur and J. Lalevée, Macromol. Chem. Phys., 2021, 222, 2000404 CrossRef CAS.
- M. A. Tasdelen, J. Lalevée and Y. Yagci, Polym. Chem., 2020, 11, 1111–1121 RSC.
- D. K. Balta, N. Arsu, Y. Yagci, A. K. Sundaresan, S. Jockusch and N. J. Turro, Macromolecules, 2011, 44, 2531–2535 CrossRef CAS.
- N. Giacoletto and F. Dumur, Molecules, 2021, 26, 3192 CrossRef CAS PubMed.
- M. Ibrahim-Ouali and F. Dumur, Eur. Polym. J., 2021, 158, 110688 CrossRef CAS.
- L. Deng, L. Tang and J. Qu, Prog. Org. Coat., 2022, 167, 106859 CrossRef CAS.
- W. Lu, G. Ma and J. Qu, Prog. Org. Coat., 2024, 187, 108102 CrossRef CAS.
- S. Gong, X. Wu, Q. Liao, S. Deng, J. Hou, K. Tang, Y. Xiong, Z. Li and H. Tang, Green Chem., 2023, 25, 2730–2744 RSC.
- S. Liu, Y. Zhang, K. Sun, B. Graff, P. Xiao, F. Dumur and J. Lalevée, Eur. Polym. J., 2021, 147, 110300 CrossRef CAS.
- Y. Zhang, H. Chen, L. Vidal, G. Schrodj, F. Morlet-Savary, F. Dumur, A. Simon-Masseron and J. Lalevée, Adv. Mater. Technol., 2022, 7, 2200074 CrossRef CAS.
- L. Tang, J. Nie and X. Zhu, Polym. Chem., 2020, 11, 2855–2863 RSC.
- H. Chen, D. Zhu, T. Kavalli, P. Xiao, M. Schmitt and J. Lalevée, Polym. Chem., 2023, 14, 3543–3568 RSC.
- J. Li, H. Wu, Y. Chen, K. Cao, Y. Li, Q. Ding, X. Zhou, Z. Fu, J. Nie and X. Zhu, Prog. Org. Coat., 2023, 178, 107455 CrossRef CAS.
- J. Li, W. Li, Z. Zhang, Q. Deng, X. Luo, J. Yang, X. Zhu and J. Nie, Prog. Org. Coat., 2024, 187, 108198 CrossRef CAS.
- J. Li, X. Zhang, J. Nie and X. Zhu, J. Photochem. Photobiol., A, 2020, 402, 112803 CrossRef CAS.
- J. Li, J. Nie and X. Zhu, Prog. Org. Coat., 2021, 151, 106099 CrossRef CAS.
- T. Lu and F. Chen, J. Comput. Chem., 2012, 33, 580–592 CrossRef CAS.
- X. Guo, W. Wang, D. Wan and M. Jin, Eur. Polym. J., 2021, 156, 110617 CrossRef CAS.
- M. Menzinger and R. Wolfgang, Angew. Chem., Int. Ed. Engl., 1969, 8, 438–444 CrossRef CAS.
- C. A. Grambow, L. Pattanaik and W. H. Green, J. Phys. Chem. Lett., 2020, 11, 2992–2997 CrossRef CAS.
- A. Valdebenito and M. V. Encinas, J. Polym. Sci., Part A: Polym. Chem., 2003, 41, 2368–2373 CrossRef CAS.
|
This journal is © The Royal Society of Chemistry 2024 |