DOI:
10.1039/D4PM00089G
(Review Article)
RSC Pharm., 2024,
1, 151-160
Enhancement of drug permeation across skin through stratum corneum ablation
Received
21st March 2024
, Accepted 7th April 2024
First published on 19th April 2024
Abstract
The presence of the uppermost layer of the skin, referred to as the stratum corneum (SC), restricts the therapeutic efficacy of many drugs by acting as a barrier for drug molecules. Consequently, only a small number of molecules are likely to reach the intended target region. To overcome this impediment, transdermal drug delivery (TDD) that ablates the SC was developed, resulting in the formation of micropores that develop in a defined region of the skin's outer layer, which facilitates the delivery of extremely hydrophilic medications and macromolecules throughout the skin. The process of SC ablation involves the use of a range of physical techniques, which may be categorized as element-based heating, radiofrequency, laser, and suction ablation. Lately, there has been an increasing fascination with using physical ablative methods for skin treatment. Studies have shown that using ablative methods to improve drug delivery has many benefits, such as higher bioavailability, shorter treatment duration, and rapid recovery of the skin barrier. This review presents a comprehensive overview of the principles underlying a variety of methods for SC ablation, focusing on their potential for dramatically increasing skin absorption of drug molecules, delivering vaccines as a non-invasive alternative to injections, facilitating the delivery of macromolecules, and their application in drug delivery for chronic diseases like Alzheimer's disease or diabetes mellitus. In addition, we summarize some previous studies that compared the effectiveness of various SC ablation methods.
1. Introduction
TDD has gained significant popularity for administering medications for a considerable period of time, commencing with the introduction of the scopolamine patch to the market in 1979.1 Nitroglycerine received authorization as the second transdermal patch in 1981.2 Presently, it is estimated that over a billion transdermal patches are manufactured each year.3 For example, clonidine and nitroglycerin, which are used to treat cardiovascular disorders, fentanyl, which is used to treat chronic pain, and nicotine, which is used to treat addiction to smoking, are all now available through TDD.4 Hormone replacement therapies and contraceptive patches are also available.5 TDD provides numerous advantages compared to oral and other invasive drug delivery methods because it reduces hepatic first-pass metabolism, enhances therapeutic efficacy, increases patient compliance, and reduces systemic side effects.6 However, the SC, the initial protective layer of the skin, presents a considerable obstacle to the effective transport of therapeutics via the transdermal route; consequently, therapeutic agents are less efficiently delivered, and only certain classes of medications can be given transdermally.7 In order to address these constraints, numerous studies have been carried out on SC ablation methods, which use localized heat to selectively damage the structure of the SC.8 These methods enhance the delivery of water-soluble medicines and macromolecules by forming microchannels in the skin.9
1.1. Skin structure
The skin is the body's first defensive mechanism against harmful environmental factors, and it is the largest organ in the body, covering about 1.5–2 m2 of surface area.10 The skin's protective function is reflected in its multilayered architecture.11Fig. 1 depicts the three main layers of human skin: the uppermost epidermis (most pharmacological processes, including the binding of drugs, their metabolism, and their active transport, all take place in this layer), the deeper dermis (which provides structural support for the skin), and the deepest hypodermis (which contains a large amount of fat for the purpose of regulating internal body temperature in cold weather and protecting against external damage).7 The SC, which consists of a lipid matrix and corneocytes that are packed tightly together like bricks and mortar, plays the primary role as the skin barrier.10 Researchers have developed chemical and physical methods to change the SC structure, which can improve transdermal drug absorption.12 The process of chemical enhancement is an example of a passive method that uses organic solvents, fatty acids, glycols, and surfactants,13 while physical methods use outside energy as a primary driving force in order to damage the SC.14 Examples include thermal ablation (thermophoresis), suction ablation, microneedles, iontophoresis, needleless injection, and ultrasound.15
 |
| Fig. 1 The diagram displays drug delivery sites of action and the basic skin layers, which consist of the epidermis, dermis, and hypodermis. The SC, which is the component of the skin that is present at the epidermis’ top layer, is accountable for its barrier function. Additional constituents of the skin comprise nerves and blood vessels. This figure has been adapted from ref. 11 with permission from Bentham Science Publishers, Ltd, copyright © 2019. | |
1.2. Routes of drug penetration
There are potentially three routes that a medicated molecule uses to go through the intact SC.16 The first method is the appendageal route, which allows medications to go via hair follicles and other appendages.13 However, both hair follicles and sweat ducts have a small surface area, which limits the area that the drug may directly come into contact when administered.17 Drugs that are hydrophilic may bypass the corneocytes and enter the body through the second route, which is called the transcellular or intracellular route.16 The last path is intercellular or paracellular, where the medication diffuses across the continuous lipid matrix, therefore being the best way to transport lipophilic substances.18 Although it is true that there are paths for pharmaceuticals to do so in order to enter systemic circulation, the majority of medications are unable to pass the skin barrier.8 For instance, standard TDD is unable to carry ionic medications or macromolecules such as proteins and peptides.19
Fig. 2 shows how TDD has evolved through many developmental generations in an effort to overcome restrictions by focusing on the mechanics of different approaches to improving drug penetration through the skin while minimizing any potential risks to the skin.20 The first generation comprises conventional patches with hydrophilic medicines of low molecular weights.10 Second-generation delivery systems used innovative methods, including iontophoresis, ultrasound, and chemical enhancers, to control the rate of distribution and enhance the percutaneous penetration and absorption of various therapeutic drugs.21 Nevertheless, it might present a challenge to disturb the SC barrier without inducing irritation, particularly when employing chemical enhancers.20 In order to drive or force a drug molecule past the SC barrier and into the epidermis, the primary goal of the third generation is to disrupt or eliminate the SC using direct physical techniques, which include thermal ablation, microneedles, electroporation, and microdermabrasion methods, to increase skin penetration while simultaneously safeguarding underlying tissues.22 This review mostly focuses on SC ablation methods.
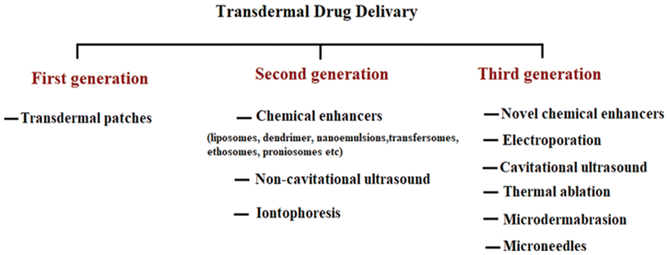 |
| Fig. 2 Illustration of the three distinct TDD generations, each representing advancements made at that particular age. Lipophilic small drugs were delivered using transdermal patches in the first generation. The introduction of the second generation involved implementing patches that incorporated alterations to enhance permeability and maximize drug delivery. Advancements during the third generation enhanced the ability of macromolecules and vaccinations to permeate the outer corneum layer, resulting in improved effectiveness of TDD. This figure has been adapted from ref. 7 with permission from Elsevier B.V., copyright © 2020. | |
2. Thermal ablation
Thermophoresis, another term for thermal ablation, is a physical method used to selectively destroy the SC structure through localized heat, typically above 100 °C.9 The SC will be destroyed to some degree, which will result in the following: disrupting the structure of lipid accumulation, destroying the structure responsible for the keratin network, and the breakdown and vaporization of a portion of the SC, which will result in the production of micropores and an increase in the permeability of the skin.23 This process aims to create micron-scale channels (diameter between 50 and 100 μm) similar to those produced using a microneedle array.11 The SC can be removed by exposing the area to high temperatures for a brief period (usually on the order of microseconds) without causing any damage to the underlying epidermis.24 This temperature differential across the SC allows the skin's surface to remain hot while preserving the deeper layers of live tissue, keeping them much cooler and structurally unchanged.25 For safety concerns, it is generally not recommended to ablate large sections of the skin surface; therefore, heating sources with diameters of just a few microns are often utilized.26 This method offers superior control and repeatability compared to alternative techniques like mechanical abrasion and tape-stripping.9 Moreover, it enables the efficient distribution of molecules with hydrophilic properties and macromolecular compounds.27 Thermal ablation may be conducted using many methods, including element-based heating,28 radiofrequency,29 and lasers.30
2.1. Element-based heating
Thermoporation, also known as element-based heating, has drawn attention as a technique for enhancing the rate of skin penetration by producing small openings in the SC.31 Various technologies have been developed for creating these pores in the skin through thermal ablation.32 One such technology is PassPort™, developed by Altea Therapeutics, depicted in Fig. 3, which combines thermal microporation and patch technology within a single device.33 PassPort™ generates aqueous micropores in the skin via thermal ablation, enabling TDD.34 In this technology, the porator, which is contained inside the applicator, is applied to the skin.31 Subsequently, the porator transfers heat energy by supplying electrical energy to an array of metallic filaments for a few microseconds, activated by pressing a button.29 This process leads to the localized disintegration and vaporization of the SC.35 After applying the patch containing medication, a variety of drug molecules can penetrate deeper skin layers through these temporary micropores.31 This method enhances the absorption of active substances via the skin, allowing for either a local or systemic effect.29 According to previous studies, micropores have a depth ranging from 30 to 50 μm and a width between 50 and 200 μm.36 The PassPort™ System enables the delivery of a diverse array of therapeutics and vaccines that cannot be delivered using standard patches.35 It is known for its cost-effectiveness, affordability, and precise drug delivery capabilities across diverse drug classes.34 In contrast to microneedles, this innovative technology eliminates the need for needles, pumps, or complex devices, potentially making it a safer and more advantageous choice.31
 |
| Fig. 3 The PassPort™ system induces micropores in the SC, facilitating the passage of medications from a transdermal patch. This may result in either a local or systemic effect, with the medication being released in a sustained or immediate manner. This figure has been adapted from ref. 8 with permission from Controlled Release Society, Copyright © 2020. | |
The PassPort™ device was among the first skin patches designed for transdermal insulin delivery.37 The insulin skin patch device aims to improve patient compliance compared to insulin injection analogs while enhancing and maintaining basal insulin levels to lessen the likelihood of hypoglycemia.38 Ono et al.31 showed that PassPort™-induced pores improved the delivery of insulin and fluorescein isothiocyanate–dextran (FD-4) into the skin. After 8 hours, there was a notable rise in the permeability of FD-4 after it was applied to the stripped skin, with roughly a 4500-fold greater cumulative quantity seen. However, the penetration increased by 930-fold after PassPort™ was applied. On the other hand, the PassPort™ system resulted in a steady increase in the concentration of insulin in the blood, and the bioavailability was found to be 21.1% in vivo when compared to subcutaneous injection, and the skin's resistance had almost returned to its original level after 12 hours. Moreover, Badkar et al.28 examined the TDD of interferon alpha-2b (INFa2b) using the PassPort™ device, with or without the use of iontophoresis. The molecular weight of INFa2b is about 19.271 kDa, which makes its delivery using iontophoresis unfeasible. The PassPort™ device effectively delivered INFa2b in vivo to hairless rats, giving a cumulative dose of 397 ± 67 ng over a period of 6 hours. Microporation and iontophoresis, when used together, significantly improved the efficacy of this delivery approach, resulting in a cumulative delivery of 722 ± 169 ng over the same time frame. Furthermore, Hata et al.39 used the PassPort™ device in order to treat Alzheimer's disease. They examine the impact of p3-Alcb9-19 on mitochondrial activity in monkeys, as mitochondrial dysfunction is prevalent in the brains of Alzheimer's disease patients and is known to decrease p3-Alcb37 levels. In vivo tests have verified that the transdermal application of p3-Alcb9-19 (at a dosage of 1.0 mg kg−1) on monkeys effectively transports the peptide into their brains, which increases mitochondrial activity in monkey brains. It is possible that this therapy holds great promise for reviving, safeguarding, and boosting mental abilities in Alzheimer's patients.
2.2. Radiofrequency (RF)
To perform RF thermal ablation, an electrode with the shape of a tiny needle is placed into the patient's skin.40 When skin cells are exposed to high frequencies (between 100 and 500 kHz), they vibrate due to the ions near the electrodes.40 These vibrations generate heat, causing water to evaporate and cells to ablate.20 Consequently, small microchannels with a depth of 50 μm are formed in the top layers of the skin, resulting in drug transport through the skin, as shown in Fig. 4.9 RF microporation successfully delivered hydrophilic, lipophilic, and macromolecules transdermally.41 Ahn et al.42 proved that the RF microporation device effectively created micropores on both porcine skin and human skin, resulting in increased permeability for delivering fluorescein isothiocyanate-dextrans (FITC-dextrans) transdermally. Nevertheless, the lower molecular weight of FITC-dextran (4 K) facilitated enhanced penetration compared to higher molecular weights (10 K and 20 K). Additionally, porcine skin has a higher propensity for penetration in comparison with human skin. Following a 24-hour duration, the cumulative quantity of 4K FITC-dextran that permeated human skin was 10.61 ± 1.68, whereas in porcine skin it was 13.44 ± 0.18 μM. In contrast, the total permeability of human skin was determined to be 4.82 ± 0.32 μM at 10 K and 2.53 ± 0.48 μM at 20 K, whereas the permeability of porcine skin was 5.92 ± 0.46 μM at 10 K and 3.34 ± 0.19 μM at 20 K.
 |
| Fig. 4 An illustration of the process of TDD following the use of the RF microporation device. It works by creating microchannels in the SC, allowing TDD of different compounds. This figure has been adapted from the open access article from ref. 42, the https://www.ncbi.nlm.nih.gov/pmc/articles/PMC7724873/figure/Fig1/ is licensed under https://creativecommons.org/licenses/by/4.0/. | |
Most recently, RF and microneedles (MNs), known as RFMN, have been used together to improve TDD by combining thermal treatment and mechanical effects to facilitate the crossing of hydrophilic macromolecules through the SC.43 RFMN is useful in the treatment of a broad variety of dermatological diseases, such as acne vulgaris, melasma, skin rejuvenation and rosacea.44 Yogya et al.43 evaluated the efficacy of RFMN for reducing wrinkles in a sample of 29 individuals, as well as the combined effect of applying topical polynucleotides (PNs) after RFMN therapy. Every participant received RFMN over the course of three sessions, spaced out by two weeks. 34% of patients had a significant improvement in their condition, ranging from 51% to 75% after two months of getting treatment. In contrast, just 27% of the individuals in the control group achieved comparable outcomes. These findings indicate that RFMN technology is a secure and efficient option for addressing periorbital wrinkles, since neither significant side effects nor patient discomfort were reported.43 Furthermore, Yu et al.45 found that a combination of RFMN and topical minoxidil (5%) was a successful treatment method for male pattern hair loss in 19 individuals. The average hair count and hair thickness increased from 44.12 ± 21.58 and 53 ± 13 μm to 73.14 ± 25.45 and 71 ± 15 μm, respectively, after 5 months of treatment on the side of the patient receiving combination therapy. While on the side receiving monotherapy, the average hair count and hair thickness rose from 46.22 ± 18.77 and 52 ± 16 μm to 63.21 ± 19.22 and 66 ± 14 μm, respectively.
2.3. Laser ablation
As mentioned earlier, the primary obstacle to TDD is the SC. However, this barrier can be effectively controlled and removed using lasers.46 Laser ablation works by creating a micropore structure in the skin and increasing the skin temperature, which in turn enhances skin diffusivity.9 A number of earlier investigations have revealed that the use of the laser ablation method enables the penetration and absorption of macromolecules,47 hydrophilic compounds,48 vitamin C, and growth factors through the skin.49 In the beginning, fully-ablative lasers were used to make TDD more effective.30 However, the use of a fully ablative laser may cause skin sensitivity, prolonged erythema, and an extended postoperative recovery time.50 To mitigate these risks, ablative fractional lasers (AFLs) represent a unique technology that offers improved safety compared to fully-ablative laser methods.51 Two notable examples include the ablative fractional erbium-doped yttrium aluminum garnet laser (AFL–Er:YAG) and the ablative fractional carbon dioxide laser (AFL–CO2).52 Both of these lasers have excellent tolerability and safety in humans and promote faster tissue recovery, resulting in a quick onset of action, as shown in Fig. 5.30 For instance, they can effectively and safely deliver 5-fluorouracil cream to treat hypertrophic scars,53 triamcinolone cream for areata alopecia,54 or epidermal growth factor and AFL together to treat acne scars.55Table 1 summarizes the key distinctions between these two lasers.
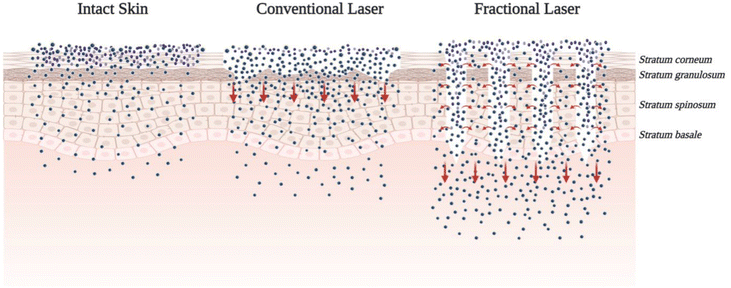 |
| Fig. 5 Illustration of the fundamental distinctions between conventional lasers and AFLs. The use of AFLs offers a variety of benefits to the patient. For example, avoiding the SC barrier makes it possible for drugs to be delivered rapidly into deeper layers of tissue. In addition, they demonstrate rapid and thorough recovery. This figure has been adapted from the open access article from ref. 59, the https://europepmc.org/articles/PMC8401127/figure/pharmaceuticals-14-00772-f005/ is licensed under https://creativecommons.org/licenses/by/4.0/. | |
Table 1 Comparison between AFL–Er:YAG and AFL–CO2
|
AFL–Er:YAG |
AFL–CO2 |
Ref. |
Mechanism of action |
Both fractional lasers are intensely absorbed by water, resulting in the creation of vertical laser channels, so they are referred to as microscopic thermal zones (MTZs), which act as microchannels with diameters of 50–300 μm and depths of 50–1850 μm in order to improve how drugs are absorbed through the skin |
|
30
|
Light wavelength |
2940 nm |
10 600 nm |
52
|
Uses |
They are used in the management of melasma, alopecia, vitiligo, vaccine delivery, and analgesics, and treatment of viral warts. In addition, they have cosmetic uses |
|
56
|
The penetration depth |
Less |
More |
56
|
Side effects |
AFL–Er:YAG lasers cause less thermal damage to the adjacent tissue, resulting in faster dermal repair and less dyspigmentation than AFL–CO2 lasers |
|
57
|
Coagulation zone |
The AFL–Er:YAG laser has a lower coagulation effect compared to the AFL–CO2 laser |
|
58
|
The use of AFLs in the fields of dermatology and pharmacology has increased dramatically in recent years, resulting in a significant increase in research on this method.60 For instance, Lee et al.61 used an AFL–CO2 to deliver small interfering RNA (siRNA) to the skin with the aim of treating psoriasis. This was achieved by creating microchannels to silence the gene responsible for psoriasiform lesions. AFL–CO2 greatly improved the removal of plaque, which increased the siRNA flux in mouse skin by 33-fold and in pig skin by 14-fold, respectively, in comparison with the control. Additionally, it led to a 64% reduction in IL-6 expression. In another study conducted by Disphanurat et al.62 on twenty-four individuals with striae alba, they received either AFL–CO2 and the topical aloe vera gel or AFL–CO2 and human epidermal growth factor. Both combinations produced high patient satisfaction and greatly enhanced the texture of the striae surface. However, those who received treatment with AFL–CO2 and human epidermal growth factor showed 50% improvement compared to people who were treated with AFL–CO2 and the topical aloe vera gel, which showed 25% improvement six months after their last treatment. Moreover, in 95.8% of patients, there was evidence of post-inflammatory hyperpigmentation, which improved within six months.
Alternatively, Sun et al.63 conducted a study of the management of thirty cases of Deep Infantile Hemangioma (DIH) using AFL–Er:YAG. They delivered the laser every two weeks for a duration of twenty-four weeks, followed by applying 0.5% timolol maleate topically under occlusion for thirty minutes, four times daily. Improvement was excellent in 76.7% of patients, fair in 13.3%, and moderate in 10%. Furthermore, Abd El-Dayem et al.64 evaluated the therapeutic effectiveness of using two treatment methods for keloid scars in thirty patients. The first method is an AFL–Er:YAG, followed by a steroid cream. The second method is to use a steroid injection directly into the affected area. Keloids had an average pre-treatment score of 6.9 ± 1.9 on the Vancouver Scar Scale (VSS). After the treatment, the average keloid VSS on the side that received the injection was 2.63 ± 2.09, while it was 2.07 ± 2.02 on the side that received AFL–Er:YAG. So, using AFL–Er:YAG is an effective and safe way to treat cosmetic problems.
While the use of laser ablation methods is both costly and mostly limited to hospitals, the SC may now be painlessly ablated using portable technology.8 The most recent device, the P.L.E.A.S.E.® laser, also known as the Precise Laser Epidermal System, was created by Pantec Biosolutions AG, a company in Liechtenstein.65 It relies on an Er:YAG laser designed to quickly form micropores that can be set between 100 μm and 150 μm with minimal damage to the skin.27 The P.L.E.A.S.E.® device works by causing an abrupt loss of water molecules, leading to the disruption of the epidermal layer and the subsequent formation of micropores.66 This process method makes it possible for small molecules and macromolecules to be delivered into the bloodstream through the skin.67,68 To achieve ablation that is minimally invasive and completely painless, the pore depth must be limited so that it avoids dermal nerve endings.65 This causes little or negligible heat injury to the adjacent skin, as in the case of a CO2 laser.69 Joshi et al.70 effectively vaccinated mice with measles vaccinations by encapsulating them in crosslinked bovine serum albumin microparticles. They then topically applied these microparticles to the skin treated with a P.L.E.A.S.E.® laser. It was shown that P.L.E.A.S.E.® laser immunization may create immune responses comparable to those induced by subcutaneous injection. Additionally, Bauer et al.71 used a P.L.E.A.S.E.® laser for plaque psoriasis treatment in combination with biological therapy on eight patients. The combination of AFL and etanercept had a positive safety profile and resulted in a considerable enhancement in the lesion-specific score by 1.75 points compared to the baseline. When used individually, etanercept or AFL resulted in a score improvement of just 0.75 points. Furthermore, Vora et al.72 used a P.L.E.A.S.E.® laser to deliver heparin through the skin of a pig's ear, utilizing Franz Cell systems. The TDD values of the heparin loaded poloxamer gel and heparin solution were 11.28 ± 5.32 μg cm−2 and 26.07 ± 1.82 μg cm−2, respectively. Nevertheless, the heparin gel is highly probable to have sustained delivery for a longer period of time, similar to a maintenance dosage given by continuous intravenous infusion. This proves that TDD of hydrophilic macromolecules is possible after implementing the P.L.E.A.S.E.® system.
3. Suction ablation
This method involves the formation of a very tiny blister by the application of a vacuum, which then has its top surface removed to provide a gateway through which drugs can enter dermal circulation.73 Cellpatch® is a commercially available device developed by a Swedish company,74 which incorporates a reservoir for the medication, an epidermatome for removing the blister surface, and a suction device.75 This method works by creating small skin erosions (typically 5–6 mm in diameter) by suctioning the epidermal barrier, which allows the medicine to be absorbed through the skin.76 Researchers employed this method to deliver morphine for the treatment of postoperative pain.77 Additionally, it was reported that the antidiuretic peptide vasopressin was used on healthy human individuals, achieving approximately 100% bioavailability.78 On the other hand, this approach is used to determine whether or not topical formulations are bioequivalent.79 As shown in Fig. 6, when interstitial fluid and serum fill a blister, this fluid provides a pharmacokinetic compartment in which a topical drug can be measured.80 If several blisters appear, the drug's concentration can be measured over time in the skin.80 This method is simple to use, and there is little risk that the obtained results will be rejected.81
 |
| Fig. 6 Suction blisters are formed by applying negative pressure to the skin, which causes a disruption in the skin layers that ultimately leads to the appearance of blisters filled with interstitial fluid. This figure has been adapted from ref. 82 with permission from The International Society of Dermatology, Copyright © 2011. | |
Nevertheless, the suction method has certain drawbacks, such as the lengthy amount of time it takes for a blister to form (about 2.5 hours).74 However, this may be decreased to 15–70 minutes by increasing the surface temperature up to 38 °C.83 Although the possible adverse effects of the suction method may be less severe than those connected with the use of intravenous catheters, there is still a chance of epidermal infection.84 Moreover, since a skin blister only affects a small portion of the skin's surface, a visible scar may develop.85 As a result, this method is considered invasive for practical use.81 Given these negative aspects, suction ablation did not gain popularity and it is not expected to be the method of choice.75
4. Evaluation of the efficacy of different SC ablation methods
Several previous studies compared the effectiveness of various skin treatment methods utilizing different technologies to improve TDD.86 Nieboer et al. performed a comparison study of the efficacy of AFL, microneedle, and RF methods for delivering hydrophilic compounds such as indocyanine green, a fluorescent agent. The results indicate that the use of AFL–Er:YAG, with a 300 μm ablation depth, exhibited the greatest fluorescence intensity, with a mean value of 38.89 AU. This creates deeper microchannels on the skin and may enhance the efficacy of delivering hydrophilic medication. While microneedles with a needle length of 500 μm had an average fluorescence intensity of 33.02 AU, they demonstrated an efficient alternative, followed by AFL–CO2, which had an average fluorescence intensity of 26.25 AU at an ablation depth of 300 μm. However, RF, in the depth range of ±80–90 μm, had an average fluorescence intensity of 15.27 AU, and it was not effective like AFLs and microneedles.86 Furthermore, Elsaid et al.87 conducted a comparison of 60 patients suffering from hypertrophic scars. 30 patients received treatment with the AFL–Er:YAG, while another 30 received treatment with AFL–CO2. They discovered that the AFL–Er:YAG was most effective at improving both pliability and scar vascularity, whereas the effects of AFL–CO2 were mostly observed in terms of improving pliability and reducing scar height. Moreover, Tuan et al.88 demonstrated that the utilization of AFL–CO2 yielded better results in 39 patients suffering from xanthelasma palpebrarum lesions compared to AFL–Er:YAG. The rate of significant enhancement in the third and fourth sessions for AFL–CO2 and AFL–Er:YAG was 60.98% compared to 39.02% and 90.24% compared to 63.41%, respectively. The recurrence rates for AFL–CO2 and AFL–Er:YAG were 22% and 24%, respectively, indicating no significant differences between the two. Additionally, Makwana et al.89 showed that AFL–CO2 had superior efficacy compared to microdermabrasion in reducing the visibility of acne scars in 100 volunteers. The average degree of acne scars before treatment was 3.26 ± 0.60. Results for microdermabrasion after 6 months of treatment were 2.62 ± 0.80, whereas those for AFL–CO2 were 1.78 ± 0.71. Lastly, Kale et al.90 evaluated the efficacy of a P.L.E.A.S.E.® laser, microneedles, and iontophoresis in facilitating the TDD of hydrophilic drug donepezil over pig skin using Franz Cell. Following the passive delivery of the drug, its permeability was 26.87 ± 3.97 μg sq.−1 cm−1. Permeability was greatly enhanced after applying a P.L.E.A.S.E.® laser (1562 ± 231.8 μg sq.−1 cm−1), followed by iontophoresis (623.4–52.1 μg sq.−1 cm−1), and finally microneedles (282.23 ± 8.28 μg sq.−1 cm−1).
5. Conclusion
The TDD method enables patients to self-administer medication safely and conveniently. In spite of these benefits, the barrier qualities of the SC impose limitations on the use of many medications, including proteins and biotherapeutics. Nevertheless, developments in TDD based on diverse technologies that use SC physical ablative techniques have made this route more attractive. These SC ablation methods will generate microchannels on the skin surface. Consequently, this will facilitate and augment the absorption of various pharmaceutical molecules. Several SC ablation methods have been developed to an advanced level. Among them, the PassPort™ technology employs a resistor array as a source of targeted heating, while the RF microporation device uses microelectrodes to generate microchannels. Er:YAG and CO2 lasers are two of the fractional lasers that are used most often for aiding in drug delivery, as they are highly absorbed by water. This results in the creation of vertical laser channels that function as microchannels, which can enhance TDD. Moreover, these three technologies improve bioavailability and allow for quick recovery of the SC without causing underlying dermal damage. However, suction ablation is an invasive method with some drawbacks, making it less likely to be a method of choice. Therefore, it is crucial to understand the differences in skin permeation characteristics among various devices to select equipment that enables greater permeation of specific medications in accordance with the intended therapeutic effect.
Author contributions
Ayyah Abdoh: writing original draft, revising, and editing; David Liu: reviewing and editing; and Yousuf Mohammed: conceptualization, revising, editing, and supervision. All authors agreed with the final version of the submitted manuscript.
Conflicts of interest
The authors declare no conflicts of interest.
Acknowledgements
Ayyah Abdoh is thankful and acknowledges the support of the RTP (Research Training Program) scholarship that is funded by the Commonwealth Government of Australia and the University of Queensland. This work was carried out at the Translational Research Institute, Woolloongabba, QLD 4102, Australia, which is supported by a grant from the Australian Government.
References
- N. Matharoo, H. Mohd and B. Michniak-Kohn, Wiley Interdiscip. Rev.: Nanomed. Nanobiotechnol., 2023, 16, e1918 Search PubMed.
- P. P. Deshmukh, V. Changediya and V. Rajurkar, World J. Pharm. Res., 2022, 11, 561–577 CAS.
- H. Lindley-Hatcher, J. Wang, A. I. Hernandez-Serrano, J. Hardwicke, G. Nurumbetov, D. M. Haddleton and E. Pickwell-MacPherson, Pharmaceutics, 2021, 13, 2052 CrossRef CAS PubMed.
- P. Raut and S. Mali, International Research Journal of Modernization in Engineering Technology and Science, 2023, 05, 1276–1285 Search PubMed.
- K. Purushotham and K. A. Vijetha, GSC Biol. Pharm. Sci., 2023, 22, 245–255 CrossRef CAS.
- L. C. Ng and M. Gupta, Asian J. Pharm. Sci., 2020, 15, 13–25 CrossRef PubMed.
- K. A. S. Al-Japairai, S. Mahmood, S. H. Almurisi, J. R. Venugopal, A. R. Hilles, M. Azmana and S. Raman, Int. J. Pharm., 2020, 587, 119673 CrossRef PubMed.
- R. Parhi and A. Mandru, Drug Delivery Transl. Res., 2021, 11, 817–841 CrossRef PubMed.
- W. Y. Jeong, M. Kwon, H. E. Choi and K. S. Kim, Biomater. Res., 2021, 25, 1–15 CrossRef PubMed.
- S. Sadab, S. Sahu, S. Patel, R. Khan, B. Khare, B. S. Thakur, A. Jain and P. K. Jain, Asian J. Dent. Health Sci., 2022, 2, 40–47 CrossRef.
- H. A. Benson, J. E. Grice, Y. Mohammed, S. Namjoshi and M. S. Roberts, Curr. Drug Delivery, 2019, 16, 444–460 CrossRef CAS PubMed.
- D. Ramadon, M. T. McCrudden, A. J. Courtenay and R. F. Donnelly, Drug Delivery Transl. Res., 2021, 12, 1–34 Search PubMed.
- M. Hasan, A. Khatun and K. Kogure, Pharmaceutics, 2022, 14, 525 CrossRef CAS PubMed.
- A. Z. Alkilani, J. Nasereddin, R. Hamed, S. Nimrawi, G. Hussein, H. Abo-Zour and R. F. Donnelly, Pharmaceutics, 2022, 14, 1152 CrossRef CAS PubMed.
- D. Dhamecha, V. Rajendra, A. Rathi, S. Ghadlinge, M. Saifee and M. Dehghan, Int. J. Health Res., 2010, 3, 57–70 CAS.
- M. Elmowafy, Colloids Surf., B, 2021, 203, 111748 CrossRef CAS PubMed.
- S. Malvey, J. V. Rao and K. M. Arumugam, Pharma Innovation, 2019, 8, 181–197 CAS.
- M. Emanet and G. Ciofani, Adv. NanoBiomed Res., 2023, 3, 2300020 CrossRef CAS.
- N. Akhtar, V. Singh, M. Yusuf and R. A. Khan, Biomed. Eng., 2020, 65, 243–272 CrossRef CAS PubMed.
- P. Bala, S. Jathar, S. Kale and K. Pal, J. Pharm. Res., 2014, 8, 1805–1835 CAS.
- A. Soni, J. Dua and D. Prasad, J. Drug Delivery Ther., 2022, 12, 176–180 CrossRef.
- D. Gohil, D. Panchal, V. Saggu, C. Sajan, K. Joshi, K. Patel, R. Maheshwari and Dr. R. Srivastava, Transdermal Drug Delivery System (TDDS): An Overview, Bhumi Publishing, 2023, 1, 45–52 Search PubMed.
- L.-Y. Long, J. Zhang, Z. Yang, Y. Guo, X. Hu and Y. Wang, J. Drug Delivery Sci. Technol., 2020, 60, 102007 CrossRef CAS.
- A.-M. Neculai and L.-M. Cima, Eximia, 2022, 4, 144–151 Search PubMed.
- J. W. Lee, P. Gadiraju, J.-H. Park, M. G. Allen and M. R. Prausnitz, J. Controlled Release, 2011, 154, 58–68 CrossRef CAS PubMed.
- A. Arora, M. R. Prausnitz and S. Mitragotri, Int. J. Pharm., 2008, 364, 227–236 CrossRef CAS PubMed.
- Y. Li, L. Guo and W. Lu, Photonics Lasers Med., 2013, 2, 315–322 Search PubMed.
- A. V. Badkar, A. M. Smith, J. A. Eppstein and A. K. Banga, Pharm. Res., 2007, 24, 1389–1395 CrossRef CAS PubMed.
-
G. Levin, in Percutaneous Penetration Enhancers Physical Methods in Penetration Enhancement, Springer, 2017, pp. 233–241. DOI:10.1007/978-3-662-53273-7_15.
- Y. Zhao, J. Voyer, Y. Li, X. Kang and X. Chen, Expert Opin. Drug Delivery, 2023, 20, 31–54 CrossRef CAS PubMed.
- N. Ono, T. Iibuchi, H. Todo, S. Itakura, H. Adachi and K. Sugibayashi, Eur. J. Pharm. Sci., 2022, 170, 106096 CrossRef CAS PubMed.
- M. I. Irianti, R. Rahmasari, A. E. Arifianti and R. Iswandana, J. Appl. Pharm. Sci., 2020, 10, 166–179 CAS.
-
Y. Zhou, V. Kumar, A. Herwadkar and A. K. Banga, Percutaneous penetration enhancers physical methods in penetration enhancement, 2017, pp. 423–437. DOI:10.1007/978-3-662-53273-7_27.
- Y. Liu, L. Yang and Y. Cui, Sens. Actuators Rep., 2023, 5, 100150 CrossRef.
- G. G. Dukare, P. A. Shelke and G. S. Sanap, World J. Pharm. Res., 2023, 12, 640–658 CAS.
- H. Kalluri and A. K. Banga, AAPS PharmSciTech, 2011, 12, 431–441 CrossRef CAS PubMed.
- R. Primavera, B. D. Kevadiya, G. Swaminathan, R. J. Wilson, A. De Pascale, P. Decuzzi and A. S. Thakor, Nanomaterials, 2020, 10, 789 CrossRef CAS PubMed.
- E. Mathers, E. Saso, D. Syahmi, B. Chan, S. Qu, M. Wang, D. Li and P. Zhao, Pharm. Chron., 2021, 29, 3 Search PubMed.
- S. Hata, H. Saito, T. Kakiuchi, D. Fukumoto, S. Yamamoto, K. Kasuga, A. Kimura, K. Moteki, R. Abe and S. Adachi, EMBO Mol. Med., 2023, 15, e17052 CrossRef CAS PubMed.
- A. Zaid Alkilani, M. T. McCrudden and R. F. Donnelly, Pharmaceutics, 2015, 7, 438–470 CrossRef PubMed.
- J. Kim, J.-H. Jang, J. H. Lee, J. K. Choi, W.-R. Park, I.-H. Bae, J. Bae and J. W. Park, Pharm. Res., 2012, 29, 2017–2029 CrossRef CAS PubMed.
- G. Y. Ahn, H.-S. Eo, D. Kim and S.-W. Choi, Biomater. Res., 2020, 24, 1–7 CrossRef PubMed.
- Y. Yogya, R. Wanitphakdeedecha, S. Wongdama, Y. Nanchaipruek, C. Yan and S. Rakchart, Dermatol. Ther., 2022, 12, 1133–1145 CrossRef PubMed.
- I. Magro, A. Kochhar, D. Arnaoutakis and K. Karimi, Facial Plast. Surg. Aesthetic Med., 2022, 24, S-3–S-10 CrossRef PubMed.
- A. J. Yu, Y. J. Luo, X. G. Xu, L. L. Bao, T. Tian, Z. X. Li, Y. X. Dong and Y. H. Li, Clin. Exp. Dermatol., 2018, 43, 775–781 CrossRef PubMed.
- R. Pal, P. Pandey, S. Waheed, S. K. Thakur, V. Sharma, A. Chanana and R. P. Singh, World J. Pharm. Res., 2022, 12, 688–711 Search PubMed.
- S. Scheiblhofer, S. Drothler, W. Braun, R. Braun, M. Boesch and R. Weiss, Vaccine, 2021, 39, 5259–5264 CrossRef CAS PubMed.
- S. Gou, S. del Rio-Sancho, H.-J. Laubach and Y. N. Kalia, Int. J. Pharm., 2022, 628, 122259 CrossRef CAS PubMed.
- B. H. B. Machado, J. Frame, J. Zhang and M. Najlah, Aesthetic Plast. Surg., 2021, 45, 1020–1032 CrossRef PubMed.
- C.-Y. Hsiao, S.-C. Yang, A. Alalaiwe and J.-Y. Fang, Expert Opin. Drug Delivery, 2019, 16, 937–952 CrossRef CAS PubMed.
- C.-H. Lin, I. A. Aljuffali and J.-Y. Fang, Expert Opin. Drug Delivery, 2014, 11, 599–614 CrossRef CAS PubMed.
- J. Schoppink and D. F. Rivas, Adv. Drug Delivery Rev., 2022, 182, 114109 CrossRef CAS PubMed.
- A. A. Tawfik, M. Fathy, A. Badawi, N. Abdallah and H. Shokeir, Clin., Cosmet. Invest. Dermatol., 2019, 12, 173–180 CrossRef CAS PubMed.
- M. C. A. Issa, M. Pires, P. Silveira, E. Xavier de Brito and C. Sasajima, J. Cosmet. Laser Ther., 2015, 17, 37–40 CrossRef PubMed.
- Y. Ratanapokasatit and P. Sirithanabadeekul, J. Clin. Aesthetic Dermatol., 2022, 15, 44 Search PubMed.
- T. Searle, F. R. Ali and F. Al-Niaimi, Dermatol. Ther., 2021, 11, 93–104 CrossRef PubMed.
- H. G. F. Siqueira, M. dos Santos Pereira, P. R. Teixeira, P. R. de Gusmão, V. V.-B. F. Jeovani and A. de Almeida Flippo, Surg. Cosmet. Dermatol., 2023, 15, e20230111 Search PubMed.
-
N. Verma, S. Yumeen and B. S. Raggio, Ablative laser resurfacing, StatPearls Publishing [Internet], 2020 Search PubMed.
- I. Portugal, S. Jain, P. Severino and R. Priefer, Pharmaceuticals, 2021, 14, 772 CrossRef CAS PubMed.
- E. Wenande, R. R. Anderson and M. Haedersdal, Adv. Drug Delivery Rev., 2020, 153, 169–184 CrossRef CAS PubMed.
- W.-R. Lee, Y.-K. Lin, A. Alalaiwe, P.-W. Wang, P.-Y. Liu and J.-Y. Fang, Mol. Ther.–Nucleic Acids, 2020, 19, 240–251 CrossRef CAS PubMed.
- W. Disphanurat, A. Kaewkes and W. Suthiwartnarueput, Lasers Surg. Med., 2020, 52, 166–175 CrossRef PubMed.
- L. Sun, C. Wang, Y. Cao, X. Lv, L. Tian, D. Liu, L. Li and W. Zhao, J. Dermatol. Treat., 2021, 32, 1053–1059 CrossRef CAS PubMed.
- D. H. Abd El-Dayem, H. A. Nada, N. S. Hanafy and M. L. Elsaie, J. Cosmet. Dermatol., 2021, 20, 138–142 CrossRef PubMed.
- A. R. Darade, M. Lapteva, T. Hoffmann, M. Mandler, A. Schneeberger and Y. N. Kalia, Pharmaceutics, 2022, 14, 151 CrossRef CAS PubMed.
- C. H. Tripp, H. Voit, A. An, M. Seidl-Philipp, J. Krapf, S. Sigl, N. Romani, B. Del Frari and P. Stoitzner, Exp. Dermatol., 2021, 30, 1279–1289 CrossRef CAS PubMed.
- S. del Rio-Sancho, M. Lapteva, K. Sonaje, C. Böhler, V. Ling, W.-H. Boehncke and Y. N. Kalia, Int. J. Pharm., 2020, 580, 119234 CrossRef CAS PubMed.
- E. H. Taudorf, C. Lerche, A. Erlendsson, P. Philipsen, S. H. Hansen, C. Janfelt, U. Paasch, R. Anderson and M. Hædersdal, Lasers Surg. Med., 2016, 48, 519–529 CrossRef CAS PubMed.
- D. Joshi, M. Uddin, M. D’Souza and R. Gala, Technology Update: Laser Ablation Technology for Transdermal Immunization, Journal of Vaccines & Vaccination, 2021, 15, 1–4 Search PubMed.
- D. Joshi, R. P. Gala, M. N. Uddin and M. J. D'Souza, Int. J. Pharm., 2021, 606, 120882 CrossRef CAS PubMed.
- M. Bauer, E. Lackner, P. Matzneller, V. Al Jalali, S. Pajenda, V. Ling, C. Bohler, W. Braun, R. Braun, M. Boesch, P. M. Brunner and M. Zeitlinger, Front. Med., 2021, 8, 712511 CrossRef PubMed.
- D. Vora, Y. Kim and A. K. Banga, Ther. Delivery, 2021, 12, 133–144 CrossRef CAS PubMed.
- P. R. Nivrutti, P. A. Nath and J. H. Anantrao, J. Pharm. Res. Int., 2021, 33, 46–61 Search PubMed.
- R. S. Vaseem, A. D’cruz, S. Shetty, A. Vardhan, S. Shenoy, S. M. Marques, L. Kumar and R. Verma, Advanced Pharmaceutical Bulletin, 2024, 14, 67–85 Search PubMed.
- Y. B. Schuetz, A. Naik, R. H. Guy and Y. N. Kalia, Expert Opin. Drug Delivery, 2005, 2, 533–548 CrossRef CAS PubMed.
- P. Svedman, S. Lundin, P. Höglund, C. Hammarlund, C. Malmros and N. Pantzar, Pharm. Res., 1996, 13, 1354–1359 CrossRef CAS PubMed.
- D. Westerling, P. Hoglund, S. Lundin and P. Svedman, Br. J. Clin. Pharmacol., 1994, 37, 571–576 CrossRef CAS PubMed.
- P. Svedman, C. Svedman and S. Lundin, Lancet, 1991, 337, 1506–1509 CrossRef CAS PubMed.
-
T. R. Patel, University of Georgia, 2020.
- C. Herkenne, I. Alberti, A. Naik, Y. N. Kalia, F.-X. Mathy, V. Préat and R. H. Guy, Pharm. Res., 2008, 25, 87–103 CrossRef CAS PubMed.
- S. Supe and P. Takudage, Sking Res. Technol., 2021, 27, 299–308 CrossRef PubMed.
- R. Czajkowski, The International Society of Dermatology, 2011, 50, 180–183 CrossRef PubMed.
- M. B. Brown, G. P. Martin, S. A. Jones and F. K. Akomeah, Drug Delivery, 2006, 13, 175–187 CrossRef CAS PubMed.
- J. A. Down and N. G. Harvey, Transdermal Drug Delivery, 2002, 123, 327–359 Search PubMed.
- F. Erdő, N. Hashimoto, G. Karvaly, N. Nakamichi and Y. Kato, J. Controlled Release, 2016, 233, 147–161 CrossRef PubMed.
- M. J. Nieboer, A. A. Meesters, M. Almasian, G. Georgiou, M. A. de Rie, R. M. Verdaasdonk and A. Wolkerstorfer, Lasers Med. Sci., 2020, 35, 1357–1365 CrossRef PubMed.
- D. Elsaid, A.-A. Al-Tawel, H. Sabry and E. Hasby, Benha J. Appl. Sci., 2023, 8, 65–79 CrossRef.
- H. Tuan, Y. Chen, S. Yang, D. Liu, D. Chen and Y. Zhao, Photobiomodulation, Photomed., Laser Surg., 2021, 39, 131–136 CrossRef CAS PubMed.
- J. Makwana, D. Vora and V. Soyal, International Journal of Research and Review, 2022, 9, 34–38 CAS.
- M. Kale, T. Kipping and A. K. Banga, Int. J. Pharm., 2020, 589, 119853 CrossRef CAS PubMed.
|
This journal is © The Royal Society of Chemistry 2024 |
Click here to see how this site uses Cookies. View our privacy policy here.