DOI:
10.1039/D4OB00327F
(Review Article)
Org. Biomol. Chem., 2024,
22, 3559-3583
Recent developments in the enzymatic modifications of steroid scaffolds
Received
29th February 2024
, Accepted 15th April 2024
First published on 16th April 2024
Abstract
Steroids are an important family of bioactive compounds. Steroid drugs are renowned for their multifaceted pharmacological activities and are the second-largest category in the global pharmaceutical market. Recent developments in biocatalysis and biosynthesis have led to the increased use of enzymes to enhance the selectivity, efficiency, and sustainability for diverse modifications of steroids. This review discusses the advancements achieved over the past five years in the enzymatic modifications of steroid scaffolds, focusing on enzymatic hydroxylation, reduction, dehydrogenation, cascade reactions, and other modifications for future research on the synthesis of novel steroid compounds and related drugs, and new therapeutic possibilities.
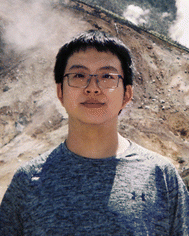 Huibin Wang | Huibin Wang received his B.S. degree from Shenyang Pharmaceutical University in 2021 and M.S. degree from the University of Tokyo in 2023. He is currently a Ph.D. candidate in the University of Tokyo under the supervision of Prof. Ikuro Abe. His research interest focuses on understanding and reprogramming nature's biosynthetic machinery based on structural enzymology. |
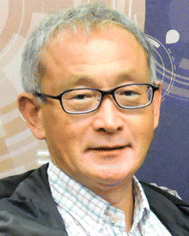 Ikuro Abe | Ikuro Abe received his B.S. (1984) and Ph.D. (1989) from The University of Tokyo, where he studied chemistry and biochemistry of natural products biosynthesis. He did his postdoctoral research with Professors Guy Ourisson and Michel Rohmer mostly at the Ecole Nationale Supérieure de Chimie de Mulhouse (1989–1991), and with Professor Glenn D. Prestwich at the State University of New York at Stony Brook (1991–1996) and then at the University of Utah (1996–1998). In 1998, he moved back to Japan to join the faculty at University of Shizuoka (1998–2009), and then was appointed as Professor at the Graduate School of Pharmaceutical Sciences, The University of Tokyo (2009–). His research interests mostly focus on exploring and engineering the natural products biosynthesis. He received the Japanese Society of Pharmacognosy Award (2017), Sumiki Umezawa Memorial Award (2017), The Pharmaceutical Society of Japan Award (2019), Prizes for Science and Technology by the Minister of Education, Culture, Sports, Science and Technology, Japan (2019), and The Society for Actinomycetes Japan, Omura Award (2023). He is a Fellow of The Royal Society of Chemistry (FRSC) and a former President of The Japanese Society of Pharmacognosy. |
1. Introduction
Thousands of steroids have been discovered in microorganisms, plants, and animals, and these compounds display a variety of pharmacological activities, including anti-inflammatory, antitumor, antimicrobial, antiandrogenic, and immunosuppressive effects.1–3 The diverse biological activities attributed to the steroid nucleus stem from its array of functionalities, through the presence of hydroxyl, carbonyl, halide, and glycosyl groups at various positions.4,5
Classical steroids are characterized by the 6/6/6/5- tetracyclic ring system, with methyl groups at C-10 and C-13 and a substituent group at C-17. Many bioinspired total syntheses of biologically active steroids have been reported.6,7 Meanwhile, the use of enzymes in biocatalytic processes offers high selectivity, efficiency, and sustainability.8–11 Enzymatic modifications of steroids are being pursued, and efforts toward the identification of novel enzymes and protein engineering are crucial for enhancing enzyme selectivity and activity.12,13 Besides, the chemoenzymatic method presents an effective and concise approach for the synthesis of steroidal products and has been recently reviewed by Qu and coworkers.14
In this review, we highlight the developments during the past five years in the enzymatic modifications of steroid scaffolds. We provide a comprehensive overview of advancements in enzymatic hydroxylation, epoxidation, ketoreduction, dehydrogenation, halogenation, glycosylation, side-chain cleavage, methylation and demethylation, ring system reconstruction, acylation, amination, amidation, isomerization, sulfonation, novel biosynthetic pathways, and artificial multi-enzyme cascades and biotransformations.
2. Hydroxylation
Hydroxylation of steroids stands as one of the most pivotal reactions in modifications of the steroid scaffold.15–17 The addition of hydroxy groups not only influences physiological functions, polarity, solubility, and toxicity but also serves as a synthetic starting point for the preparation of valuable steroid drugs.18,19 In this section, we discuss recent advances in the discovery and engineering of cytochrome P450 oxygenases (P450s), non-heme iron- and α-ketoglutarate-dependent oxygenases (αKG OXs), Rieske oxygenases, and other enzymes for site-specific hydroxylations. For a comparative summary elucidating the preference sites for enzymatic hydroxylation of steroids, readers are directed to the 2020 review by Zhang et al., the 2022 review by Zhu et al. and the 2023 review by Abas et al.15–17
2.1 P450s
C-14 functionalized steroids, encompassing 14α-OH and 14β-OH substituted steroids, are widely distributed in nature and exhibit significant biological activities, as evidenced by drugs such as the cardiac glycosides digoxin (1), digitoxigenin (2), and bufotalin (3), and the veterinary drug proligestone (4) (Fig. 1A).20
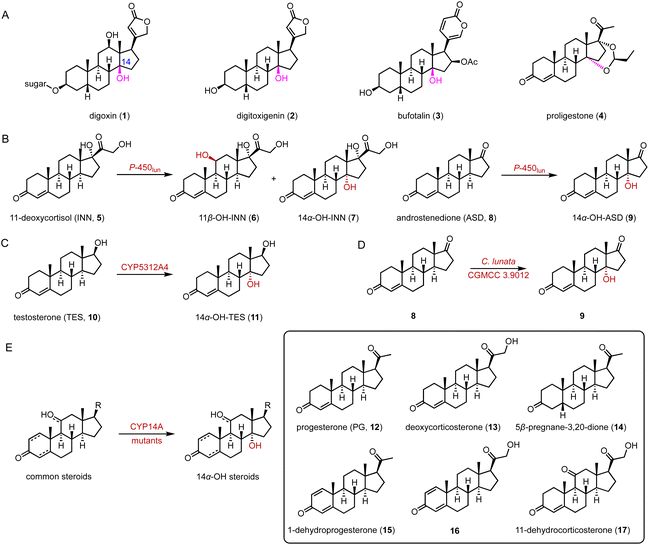 |
| Fig. 1 C-14 hydroxylation mediated by P450s. (A) Structures of C-14 functionalized steroid drugs. Hydroxylation reactions catalyzed by (B) P-450lun, (C) CYP5312A4, (D) C. lunata, and (E) CYP14A enzyme variants. | |
In 2019, Zhang and coworkers utilized the fungal P-450lun from Cochliobolus lunatus for C-14α hydroxylation.21 P-450lun catalyzed the C-11β and C-14α hydroxylation of 11-deoxycortisol (INN, 5), producing hydrocortisone (6) and 14α-OH-INN (7), respectively (the final 6/7 product ratio is about 3
:
2), while it mainly hydroxylated androstenedione (ASD, 8) to yield 14α-OH-ASD (9) (regiospecificity over 99%) (Fig. 1B). Additionally, in 2019, Ichinose and coworkers characterized CYP5312A4 for the C-14α hydroxylation of testosterone (TES, 10), to generate 14α-OH-TES (11) (95% substrate conversion) (Fig. 1C).22
In 2022, Ge and coworkers employed metabolomic and transcriptomic analyses to identify the C-14α hydroxylase CYP11411 from the plant Calotropis gigantea and CYP44476 from the toad Bufo gargarizans.23 They conducted a C-14α hydroxylation reaction towards ASD (8) and obtained over 5 g of 14α-OH-ASD (9) through culture optimization of C. lunata CGMCC 3.9012 (70% isolation rate of 8) (Fig. 1D). Recently, Qu and coworkers identified the highly efficient and promiscuous 14α hydroxylase CYP14A from Cochliobolus lunatus.24 Through semi-rational engineering, the enzyme variants I111L/M115K and I111L/V124W displayed improved C-14 specificity and activity towards various steroids (12–17) (90–95% site selectivity, 72–78% conversion) (Fig. 1E).
The hydroxylation of C-19 in the steroid nucleus is crucial for the preparation of bioactive C-19 hydroxyl steroids, such as ouabagenin (18) and 19-nor steroidal drugs (Fig. 2A).25–27 However, this process presents a significant challenge due to the steric hindrance from the proximity of the C-19 methyl group between the A and B rings of steroids.26 In 2019, Zhu and coworkers identified the P450 enzyme STH10 from Thanatephorus cucumeris NBRC 6298, which catalyzed 19- and 11β-hydroxylations of INN (5) (Fig. 2B).28 Subsequently, Zhou and coworkers improved the C-19 hydroxylation activity of T. cucumeris towards 5 and 17-acetyl-INN (17) by optimizing the fermentation conditions (80% conversion at the multi-gram scale) (Fig. 2C).29
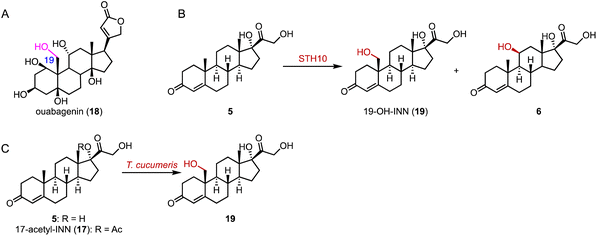 |
| Fig. 2 C-19 hydroxylation mediated by P450s. (A) The structure of ouabagenin (18). Hydroxylation reactions catalyzed by (B) STH10 and (C) T. cucumeris. | |
The valuable bile acid, ursodeoxycholic acid (UDCA, 22), is utilized for the treatment of primary biliary cholangitis.30 It features a unique C-7β hydroxy group. The only difference between UDCA and chenodeoxycholic acid (CDCA) is the configuration of the C-7 hydroxy group, which imparts hydrophilicity to UDCA.31 Direct hydroxylation at C-7 through chemical synthesis is challenging, due to the spatial distance of the C-7 position from any functional group facilitating C–H activation.32
The current synthetic approach for UDCA (22) involves a chemo-enzymatic pathway employing bile acids as initial materials.33 In contrast, lithocholic acid (LCA, 20), a cost-effective byproduct, could be directly converted to 22. Bornscheuer and coworkers engineered CYP107D1 (OleP) to catalyze the C-7β hydroxylation of 20 to produce 22, and the triple enzyme variant (F84Q/S240A/V291G) showed nearly perfect regio- and stereo-selectivity (over 95% selectivity), while the wild-type OleP catalyzed C-6β hydroxylation towards 20 to generate murideoxycholic acid (MDCA, 21) (0.04 mmol L−1 h−1space time yield) (Fig. 3A).34,35
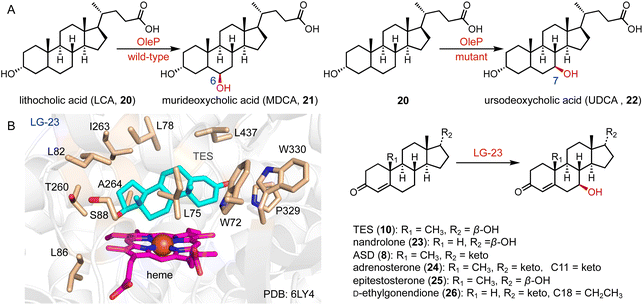 |
| Fig. 3 C-7 hydroxylation mediated by P450s. Hydroxylation reactions catalyzed by (A) OleP and (B) LG-23 and the binding mode of TES in the active site of LG-23. The TES, heme, and residues in the TES-binding site are colored cyan, magenta, and wheat, respectively. | |
Reetz and coworkers performed the directed evolution of P450 BM3 and identified the enzyme variant LG-23, containing 14 mutations compared with the wild-type enzyme.36 LG-23 efficiently catalyzed the C-7β hydroxylation of different steroid substrates, including TES (10), nandrolone (23), ASD (8), adrenosterone (24), epitestosterone (25), and D-ethylgonendione (26) (75–95% selectivity, 55–99% conversion, 32–82% yield). The co-crystal structure of LG-23 and 10 showed that TES binds to various residues in the active site via van der Waals and hydrophobic interactions, revealing the binding mode for selective C-7β hydroxylation (Fig. 3B).
Protein engineering of P450s aims to improve the activity, thermostability, and/or alter the selectivity to conduct new-to-nature reactions.37–42 Schallmey and coworkers engineered the C-16α hydroxylase CYP154C5 based on its crystal structure, and found that the CYP154C5 F92A variant catalyzed the hydroxylation of progesterone (PG, 12), generating the 21-hydroxylated product 11-deoxycorticosterone (28) in addition to 16α-OH PG (27) (Fig. 4A).43 Bernhardt and coworkers conducted the semi-rational protein engineering of the C-1α hydroxylase CYP260A1.44 The S276N variant catalyzed the 1α-hydroxylation of PG (12), while the S276I variant led to the C-17α hydroxylation of PG (12) due to substrate orientation (Fig. 4B).
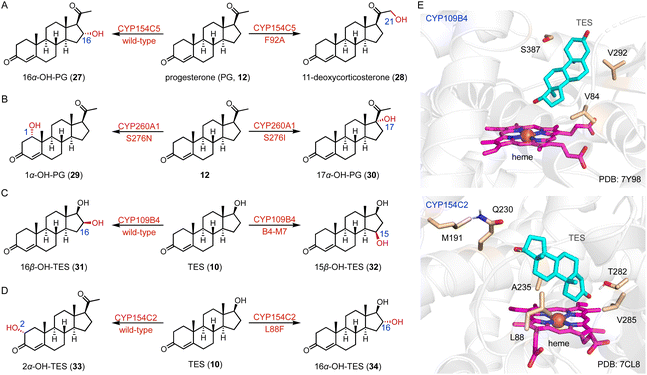 |
| Fig. 4 Protein engineering of P450s for steroid synthesis. The protein engineering of (A) CYP154C5, (B) CYP260A1, (C) CYP109B4, and (D) CYP154C2. (E) The binding mode of TES in the active sites of CYP109B4 and CYP154C2. The TES, heme, and hot-spot residues are colored cyan, magenta, and wheat, respectively. | |
Li and coworkers engineered the C-16β steroid-hydroxylase CYP109B4 based on its crystal structure, and identified three crucial residues (V84, V292, and S387) involved in regio-selectivity control.45 The B4-M7 variant (L240V/S387F/V84L/V292S/I291T/M290F/F294I) was characterized for its ability to switch the regioselectivity to C-15β hydroxylation (Fig. 4C and E). Li and coworkers mutated the arginine residues around the substrate entrance and active site of the C-16β hydroxylase CYP105D7, and the conversion rate of the CYP105D7 R70A/R190A variant increased almost 9-fold towards TES (10) compared with the wild-type.46 Recently, they utilized a structure-guided rational design strategy to improve the C-2α hydroxylation activity of CYP154C2.46 The double variants L88F/M191F and M191F/V285L improved the activities towards TES (10) and ASD (8), respectively, while all enzyme variants containing L88F generated the 16α-hydroxylation product (Fig. 4D and E).
The dependence of the P450 catalytic function on the cofactor NAD(P)H and redox partner proteins limits its practical applications.47 One useful strategy is to engineer P450 monooxygenases into the peroxizyme forms, peroxygenase and peroxidase.48 Using this approach, Bell and coworkers altered the C-16α hydroxylase CYP154C8 to a peroxygenase (over 100-fold peroxygenase activity) by the T258E variant.49
Fungal organisms harbor numerous P450 enzymes capable of the highly selective production of valuable hydroxysteroids.50–53 For example, CYP5150AP3 and CYP5150AN1 from Thanatephorus cucumeris NBRC 6298 catalyzed the C-7β hydroxylation of INN (5) and TES (10), and the C-2β hydroxylation of 11-deoxycortisol (5), respectively.54 P450cur from Curvularia sp. VKM F-3040 possessed C-7β hydroxylase activity toward ASD (8), dehydroepiandrosterone (DHEA), and androstenediol, and C-7α hydroxylase activity toward 1-dehydrotestosterone.55 CYP68J5_Fusarium graminearum (CYP68J5_fg) showed C-12β hydroxylation (31% yield), 15α-hydroxylation (48% yield) and C-12β and C-15α di-hydroxylation (21% yield) activities towards PG (12).56 CYP68J5 from Aspergillus ochraceus TCCC41060 catalyzed the C-11α hydroxylation of both 16,17α-epoxyprogesterone and D-ethylgonendione.57 The promiscuous CYP68BE1 from Beauveria bassiana accomplished mono-hydroxylation on C-11α, C-1α, and C-6β and di-hydroxylation on C-1β, C-11α and C-6β,11α of six steroids, including PG (12), TES (10), ASD (8), estrone, estra-4,9-diene-3,17-dione and 1,4-androstadiene-3,17-dione with more than 70% conversion rate.58 CYP68JX from Colletotrichum lini ST-1 catalyzed the hydroxylation of DHEA to generate 3β,7α,15α-trihydroxy-5-androstene-17-one, a key intermediate for the synthesis of drospirenone, a progestin and antiandrogen medication.59
Novel steroid hydroxylases were also characterized from bacteria, animals, and other kingdoms.60 For example, CYP154C2 from Streptomyces avermitilis MA4680 catalyzed the C-2α hydroxylation of TES (10).61 The novel CYP17A2 from Mastacembelus armatus (MA_CYP17A2), identified by genome mining, efficiently performed the C-17α hydroxylation of PG (12) (120.9 ± 4.2 mg L−1 in 7 days).62 CYP109A2 from Bacillus megaterium DSM319 catalyzed the 16β-hydroxylation of TES (10),63 while the human P450 11B2 catalyzed the C-11β hydroxylation of 11-deoxycorticosterone (28) and PG (12).64
2.2 αKG OXs
α-Ketoglutarate-dependent oxygenases (αKG OXs) are metalloenzymes with a non-heme Fe2+ ion in the active center, and are widely distributed in both primary and secondary metabolic pathways.65,66 They perform various reactions, including hydroxylation, epoxidation, desaturation, epimerization, halogenation, cyclization, demethylation, and aziridination.67,68 Industrially, αKG OXs are used for the regioselective hydroxylation of amino acids, such as proline derivatives, and for the biosynthesis of antibiotics.69,70
Mizutani and coworkers reported that 22,26-oxycholesterol 16α-hydroxylase (16DOX) stereoselectively catalyzed the C-16α hydroxylation of (22S)-22,26-dihydroxy-cholesterol (35) (Fig. 5A).71 Moreover, our group discovered the multifunctional αKG OX SptF, involved in the fungal meroterpenoid emervaridone biosynthesis pathway.72–74 It promiscuously catalyzed α-hydroxylation at the C-5 and C-9 positions with conversion rates of 34%–53% over 24 h, demonstrating the biocatalytic potential of αKG OXs for modifications of the steroid nucleus (Fig. 5B).
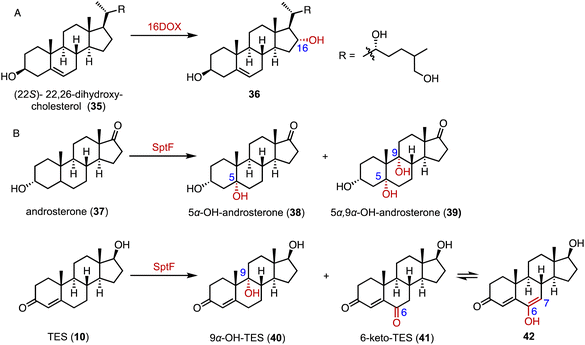 |
| Fig. 5 Hydroxylation reactions mediated by αKG OXs. Hydroxylation reactions catalyzed by (A) 16DOX and (B) SptF. | |
2.3 Rieske oxygenases
3-Ketosteroid 9α-hydroxylase (KSH), an essential enzyme in bacterial steroid degradation, consists of two components: a Rieske oxygenase (KshA) and a ferredoxin reductase (KshB).75–78 It catalyzes the 9α-hydroxylation of ASD (8) to produce 9α-OH-ASD (43). Qin and coworkers reconstituted the NADH regeneration system, utilizing the fusion expression of toluene 2,3-oxidoreductase TDO-R from Pseudomonas putida F17 instead of KshB, along with a Rieske [2Fe–2S] iron-oxidoreductant protein, TDO-F.79 The reconstitution significantly enhanced the electron transfer efficiency by 176.3%. Optimization led to the final production of 5.24 g L−1 of 9α-OH-ASD (35) from ASD (8), with a yield of 99.3% (Fig. 6).
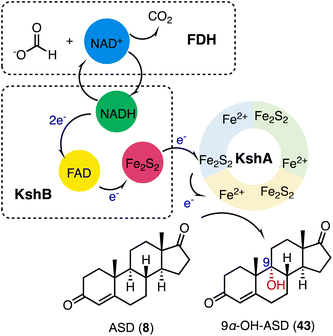 |
| Fig. 6 C-9 hydroxylation mediated by KSH. | |
Recently, Liu and coworkers employed KSH as an efficient biocatalyst to cleave the C9–C10 bond and aromatize the A-ring of steroids, by C-9α hydroxylation and a fragmentation cascade.80 Investigation of the substrate scope revealed the acceptance of a broad range of dienone steroids with variable C-17 side chains to generate the corresponding 9,10-secosteroid products (44 to 49) (87–97% yield). In addition, KSH mainly converted the non-dienone substrates that lack the C1–C2 double bond to the corresponding C-9α hydroxylated products (50 to 52) (64–94% yield) (Fig. 7).
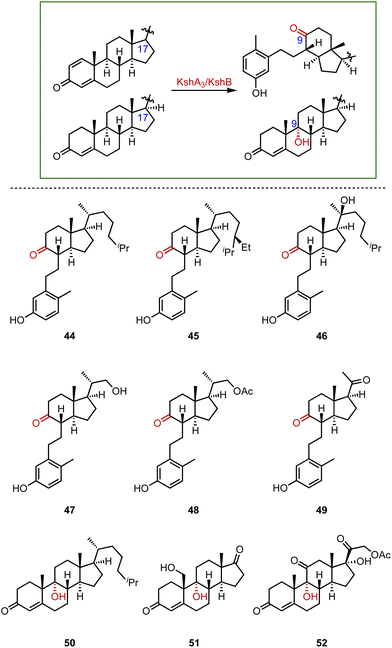 |
| Fig. 7 The substrate scope of C-9α hydroxylation and the fragmentation cascade catalyzed by KshA3/KshB. | |
2.4 Other oxygenases
Fungal unspecific peroxygenases (UPOs) offer significant advantages in the stereo- and regio-selective oxidation of aliphatic C–H bonds, such as hydroxylation, epoxidation, and sulfoxidation.81–83 Kiebist and coworkers characterized CglUPO, from the ascomycetous fungus Chaetomium globosum, which converted TES (10) to 4,5 epoxy-TES (53) and 16α-OH-TES (34) with a total turnover number (TTN) of up to 7000 (Fig. 8).84
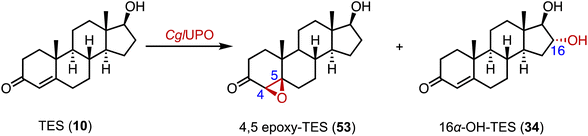 |
| Fig. 8 Hydroxylation and epoxidation mediated by CglUPO. | |
3. Epoxidation
Epoxides are valuable organic intermediates with a reactive oxirane group capable of nucleophilic opening, elimination, reduction, or rearrangement.85,86 In terms of steroid epoxidation, Auchus and coworkers reported that CYP17A1 catalyzed the novel C-16α,17 epoxidation of 16,17-dehydroprogesterone (54) in addition to the minor C-21 hydroxylation to generate 55 and 56, respectively (Fig. 9A).87 Besides, CYP17A1 A105L variant gave a 1
:
5 ratio of 55
:
56. Wang and coworkers performed the directed evolution of P450 BM3 and identified the variant P450 BM-3 5-B10 (A221V/H940Q based on the P450 BM3 enzyme variant 139-3), which catalyzed the 16α,17-epoxidation of PG (12) (Fig. 9B).88 As mentioned previously, CglUPO also catalyzed the 4,5-epoxidation of TES (10) (Fig. 8).84
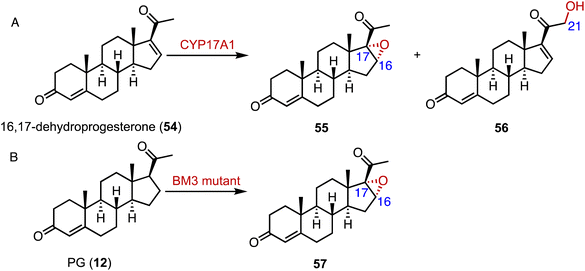 |
| Fig. 9 Epoxidation mediated by P450s. Epoxidation reactions catalyzed by (A) CYP17A1 and (B) the P450 BM3 enzyme variant. | |
4. Ketoreduction
The biocatalytic reduction of prochiral ketones into the corresponding chiral alcohols represents a valuable strategy in asymmetric synthesis.89–91 Hydroxysteroid dehydrogenase (HSDH) plays a pivotal role in the metabolic processes and synthetic pathways for the ketoreductions of steroid hormones.90–93 Typical HSDHs are C-7α hydroxysteroid dehydrogenase (7α-HSDH) and C-7β hydroxysteroid dehydrogenase (7β-HSDH), used for the synthesis of UDCA (22). 7α-HSDH catalyzes the C-7 oxidation of chenodeoxycholic acid (CDCA, 58) to 7-oxo LCA (59), and then 7β-HSDH converts 59 to UDCA (22) (Fig. 10).94–96 Considering the atom economy of the HSDH cofactor system, Xu and coworkers switched the cofactor preference of 7β-HSDH from NADPH to NADH for cost-effective biotechnical applications.97 In addition, these researchers recently characterized a novel NADH-dependent Rs7β-HSDH from Roseococcus sp. that showed high specific activity towards 59 (63.3 U per mgprotein).98
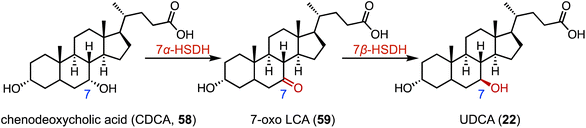 |
| Fig. 10 The synthesis of UDCA (22) through C-7 hydroxylation mediated by 7α-HSDH and 7β-HSDH. | |
The C-3 hydroxyl substituent is an important moiety in various steroid drugs, such as allopregnanolone (brand name: Zulresso, treatment of postpartum depression) (60), dehydroepiandrosterone (brand name: Intrarosa, treatment of moderate to severe dyspareunia) (61), and alfaxalone (brand name: Alfaxan, a neurosteroid anesthetic) (62) (Fig. 11A).99 Recently, Xu and coworkers developed an efficient platform for the stereo-complementary synthesis of 3-hydroxy-5-hydrogen steroids.100 They utilized Ct3α-HSDH and Ss3β-HSDH, which demonstrated exclusive chemo-, regio-, and enantio-selectivities for the reduction of 3-ketones across 25 examples (up to 99% conversion and >99% ee) (Fig. 11B). In a separate effort, Qin and coworkers identified LfSDR1 E141L/I192V variant by screening and engineering ketoreductases for the synthesis of allopregnanolone (60) with >97% conversion and a 90% isolated yield (Fig. 11C).101
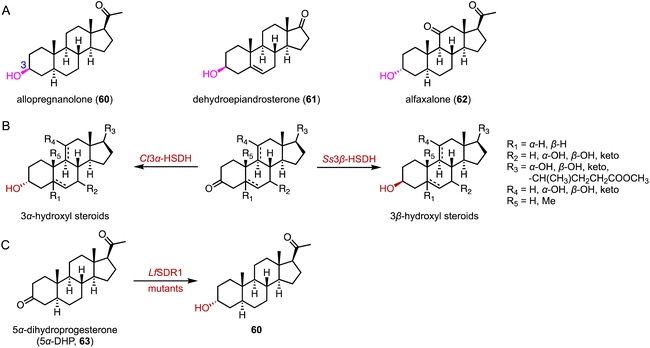 |
| Fig. 11 C-3 reduction mediated by reductases. (A) The structure of C-3 hydroxy steroid drugs. (B) The stereo-complementary synthesis of C-3 hydroxy steroids by Ct3α-HSDH and Ss3β-HSDH. (C) Reduction reaction catalyzed by LfSDR1. | |
An unusual dual-role reductase, mnOpccR, involved in phytosterol catabolism, was discovered by Qu and coworkers in 2020.102 It reduced 3-oxo-4-pregnene-20-carboxyl-CoA (3-OPC-CoA, 64) in either a four-electron manner to form 20-hydroxymethyl pregn-4-ene-3-one (4-HBC, 65) or a two-electron manner to 3-oxo-4-pregnene-20-carbaldehyde (3-OPA, 66) to form 65. The strain mJTU8 (ΔkstD-Δhsd4A and pG13-mnOpccR) harboring mnOpccR from Mycobacterium neoaurum resulted in the exclusive generation of 65 from phytosterols (Fig. 12).
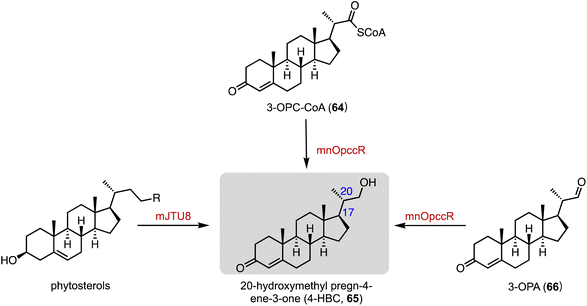 |
| Fig. 12 Reduction mediated by mnOpccR. | |
5. Dehydrogenation
The flavin adenine dinucleotide (FAD)-dependent 3-ketosteroid Δ1-dehydrogenase (KstD) introduces a double bond between the C-1 and C-2 atoms in the A ring of the C-3 ketosteroids, a key feature in several glucocorticoids such as dexamethasone (67), betamethasone dipropionate (68), clobetasol propionate (69), and beclomethasone dipropionate (70) (Fig. 13A).103 In recent years, various KstDs with high activities have been discovered and applied.104–112 For instance, Guo and coworkers used Nocardioides for the Δ1,2-dehydrogenation of 71 to produce 72, which is an important intermediate for the synthesis of fluocinolone acetonide (Fig. 13B).113 In 2022, Chen and coworkers adopted a Focused Site-directed Iterative Saturation Mutagenesis (FSISM) strategy for the engineering of MsKstD1, and the quadruple enzyme variant MK4 (H132M/L113F/V419W/M51L) exhibited 10-fold higher activity towards hydrocortisone than wild-type MsKstD1.114 Furthermore, MK4 catalyzed the desaturation of 73 to generate 74, a key intermediate in the synthesis of 16β-methylcorticoids (Fig. 13C).115
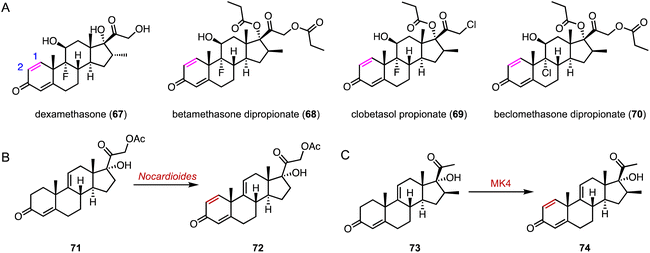 |
| Fig. 13 Dehydrogenation mediated by KstDs. (A) Structures of glucocorticoids. Dehydrogenation reactions catalyzed by (B) Nocardioides and (C) MK4. | |
6. Halogenation
The incorporation of halogens significantly alters the physicochemical properties of compounds and may affect their biological activities, metabolic pathways, and pharmacokinetic profiles.116 In addition, carbon–halogen bonds are a prominent class of synthetic building blocks in organic synthesis, which can be used for subsequent metal-catalyzed cross-coupling reactions and nucleophilic substitution reactions.117,118 In terms of the enzymatic halogenation of steroids, Lewis and coworkers performed family-wide activity profiling of flavin-dependent halogenases (FDHs) and identified 1-F11, which catalyzed the bromination of β-estradiol 17-(β-D-glucuronide) (75) at the C-4 position in 57% yield (Fig. 14A).119 In addition, Ayala used a fungal chloroperoxidase for the di-halogenation of estradiol (76) at the C-2 and C-4 positions to generate 78 (Fig. 14B).120
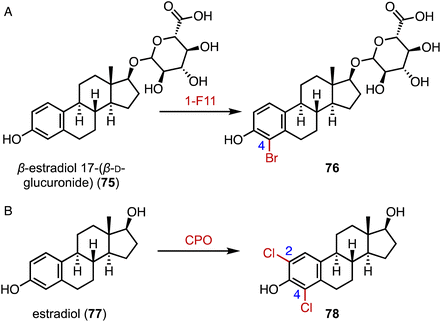 |
| Fig. 14 Halogenation mediated by halogenases. Halogenations catalyzed by (A) 1-F11 and (B) CPO. | |
7. Glycosylation
Steroid glycosylation plays a multifaceted role in biology and medicine, influencing the solubility, bioactivity, and therapeutic potential of steroid drugs.121–123 A notable example is cardiotonic steroids, which feature glycosylation at the C-3 position, leading to lower cardiotoxicity.124 Chemical glycosylation of steroids faces limitations due to the protection and deprotection processes, poor regio- and stereo-selectivities, and low yields.125,126 An alternative solution is enzymatic glycosylation, by enzymes such as PpUGT6, UGT80A40, UGT80A41, UGT73CR1, UGT80A33, and UGT80A34 from the medicinal plant Paris polyphylla, and TfS3GT2 from the pharmacologically important herb fenugreek, which are involved in the biosynthesis of steroidal saponins.121,127–130
Feng and coworkers reported the structures of the first sterol glycosyltransferase, UGT51 from Saccharomyces cerevisiae and its complex with uridine diphosphate glucose (UDPG, 79).131 The UDPG binding pocket features hydrogen and nonpolar bonds (Fig. 15A). UGT51 catalyzed the glycosylation of ergosterol to generate ergosteryl-glucoside.132 Guided by semi-rational protein engineering, the enzyme variant M7_1 (S801A/L802A/V804A/K812A/E816K/S849A/N892D) was identified and converted protopanaxadiol to the ginsenoside Rh2.133
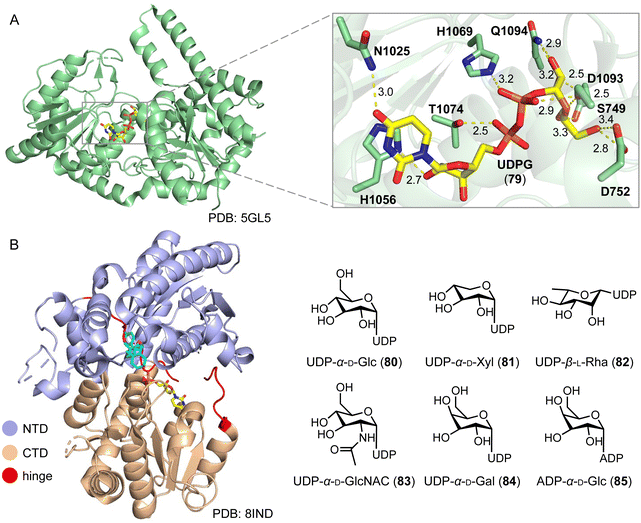 |
| Fig. 15 Glycosylation mediated by glycosyltransferases. (A) Overall structure and active site of UGT51. The protein and the ligand UDPG are shown in pale green and yellow, respectively. (B) Overall structure of UGT74AN3 and sugar donor promiscuity of UGT74AN3. The NTD domain, CTD domain, and hinge motif are colored light blue, wheat, and red, respectively. | |
Long and coworkers conducted a structure–function analysis of the plant steroid 3-O-glycosyltransferase UGT74AN3, from the medicinal plant Calotropis gigantea.134–136 It mainly catalyzes the C-3 glycosylation of steroids. The overall structure displays a classic GT-B fold structure, characterized by two Rossmann-like β/α/β domains connected by a hinge linker. The N-terminal domain (NTD) and the C-terminal domain (CTD) play primary roles in recognizing and binding the sugar acceptor and donor, respectively. UGT74AN3 showed broad substrate promiscuity towards 78 acceptors and 6 sugar donors (80–85).135,136 UGT74AN3 also exhibited N-/S-glycosylation activities towards aromatic compounds, expanding the biocatalytic toolbox for producing valuable glycosides (Fig. 15B).
8. Side-chain cleavage
The side-chain cleavage products, androstenedione (ASD, 8) and androst-1,4-diene-3,17-dione (ADD), derived from cholesterol (86) or phytosterols through biological fermentation, serve as key intermediates in the industrial production of steroid drugs.137–139 In humans, cholesterol (86) undergoes a series of three hydroxylation steps catalyzed by CYP11A1, leading to the cleavage of the C-20, 22 bond and the formation of pregnenolone (87) (Fig. 16A).140,141
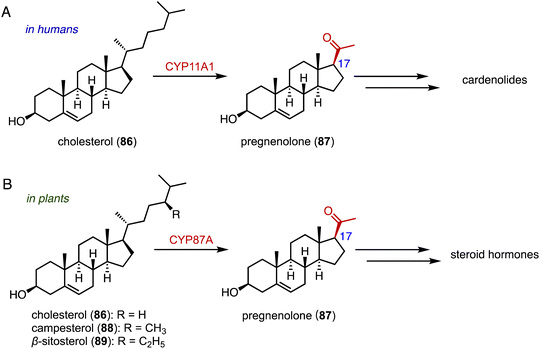 |
| Fig. 16 Side-chain cleavage mediated by P450s in humans (A) and in plants (B). | |
Recently, Wang and coworkers reported that CYP87A4 is involved in sterol side-chain cleavage in digoxin (1) biosynthesis.142 Sonawane and coworkers also found that CYP87A exhibited side-chain cleavage activity on both cholesterol (86) and phytosterols (campesterol 88 and β-sitosterol 89), resulting in the formation of pregnenolone (87).143 This marks the initial committed step in cardenolide biosynthesis (Fig. 16B).
In the biosynthetic pathway of the furanosteroid demethoxyviridin, Gao and coworkers identified a novel pregnane side-chain cleavage pathway mediated by a Baeyer–Villiger monooxygenase (VidF), an esterase (VidP), and a dehydrogenase (VidH).144 These three enzymes work together to cleave the C-17 and C-20 side chain of 3-dihydrovirone (90) to generate 91 (Fig. 17).
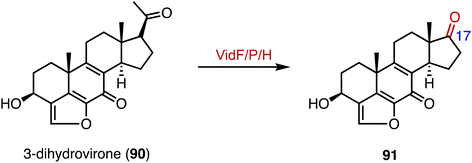 |
| Fig. 17 Side-chain cleavage mediated by VidF/P/H. | |
9. Methylation and demethylation
Methyltransferases (MTs) are ubiquitous in nature and serve in a wide range of vital life processes, including signal transduction, transcriptional regulation, and natural product biosynthesis.145 Recently, Qu and coworkers identified the novel carboxyl methyltransferase (CbMT) OPCMT, from Mycobacteria.146 It exhibited broad substrate specificity (92 to 104), converting carboxylic acids to the corresponding carboxylic acid methyl esters (Fig. 18A). Haslinger and coworkers characterized StrAOMT, an O-methyltransferase from Streptomyces avermitilis, which catalyzed the methylation of 2-hydroxyestradiol (105) to generate 2-methoxyestradiol (106) and 107 (Fig. 18B).147
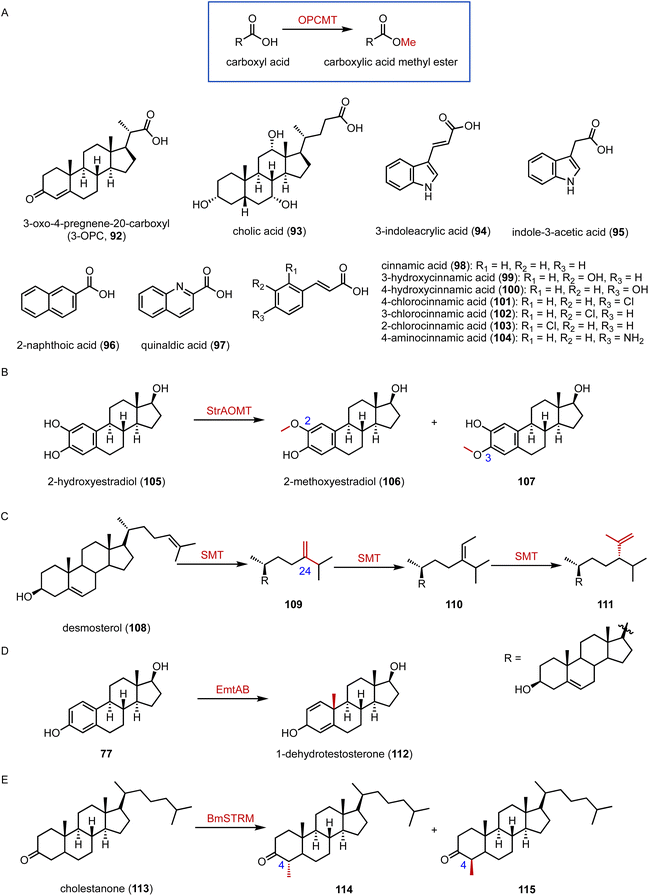 |
| Fig. 18 Methylation reactions. (A) The substrate specificity of OPCMT. Methylations catalyzed by (B) StrAOMT, (C) SMT, (D) the EmtAB system, and (E) BmSTRM. | |
Welander and coworkers reported sterol C-24 methyltransferases (SMTs) from both sponges and yet-uncultured bacteria that catalyzed the methylation of desmosterol (108) to generate C-24 methyl sterols, including 24-methylenecholesterol and (epi)codisterol (109 to 111) (Fig. 18C).148,149 Furthermore, Liebeke and coworkers found that gutless marine annelids predominantly synthesized sitosterol, a plant sterol, by a noncanonical SMT, indicating the broader occurrence of plant-like sterol biosynthesis in animals.150,151
Chiang and coworkers reported a B12-dependent methyltransferase system, EmtAB, from an anaerobic β-proteobacterium, Denitratisoma sp. strain DHT3, which converted estradiol (77) to 1-dehydrotestosterone (112) (Fig. 18D).152,153 These results suggested a novel metabolic connection between cobalamin and steroid metabolism. The ratio of 4α/4β-methyl sterane is used as an important indicator of sediment maturity in crude oil and natural gas exploration.154 The 4β-methyl steranes have long been considered to be formed from 4α-methyl steroids during diagenesis.155 However, Lu and coworkers discovered that BmSTRM, a sterol A-ring methylase (STRM) from B. minutum, catalyzed the biosynthesis of both C-4α and C-4β methyl sterols from cholestanone (113) (Fig. 18E).
The C-14α demethylation catalyzed by P450 51 is a crucial step in eukaryotic sterol biosynthesis, such as lanosterol conversion in mammals.156,157 Guengerich and coworkers solved the crystal structure of the human P450 51A1, and the application of those data in isotopic experiments supported the major contribution of Compound 0 (ferric peroxide anion, FeO2−) in 24,25-dihydrolanosterol (116) C–C cleavage (Fig. 19A).158 In addition, a cytochrome P450 aromatase (CYP19A1) is responsible for the biosynthesis of estrogens from androgens, by C-10 demethylation and A-ring aromatization.159–161 Similarly, in the biosynthetic pathway of demethoxyviridin, the cytochrome P450 monooxygenase VidA catalyzes the C-13 demethylation and C-ring aromatization processes.144
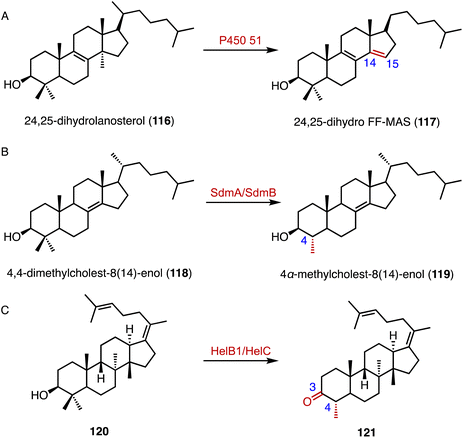 |
| Fig. 19 Demethylation reactions catalyzed by (A) P450 51, (B) SdmA/SdmB, and (C) HelB1/HelC. | |
Welander and coworkers highlighted bacterial sterol biosynthesis as distinct from eukaryotes, and identified the C-4 demethylation reaction in Methylococcus capsulatus.162 In detail, the Rieske-type oxygenase SdmA catalyzed the oxidation of the C-4β methyl group of 4,4-dimethylcholest-8(14)-enol (118), and the NAD(P)H-dependent enzyme SdmB catalyzed the dehydrogenation to generate 4α-methylcholest-8(14)-enol (119) (Fig. 19B). Yao and coworkers identified an unusual C-4 demethylation process in the biosynthesis of helvolic acid.163 The cytochrome P450 enzyme HelB1 oxidized the C-4β methyl group of 120, and the multifunctional SDR HelC removed the methyl group via a β-keto carboxylic acid intermediate to produce 121 (Fig. 19C).
10. Ring system reconstruction
Reconstruction of ring systems plays vital roles in natural product biosynthesis, particularly in the production of complex molecules like steroids.164 These processes enable the generation of structural diversity, which is crucial for the adaptation and survival of organisms in their environments. For example, in the biosynthesis of demethoxyviridin, three P450 enzymes (VidE, VidG, VidR), a short chain oxidoreductase (VidO), a hypothetical protein (VidN), and the glyoxalase VidQ are responsible for the installation of the typical furan ring (Fig. 20A).144 In diosgenin biosynthesis, CYP90 catalyzes the dihydroxylation of cholesterol (86), allowing the further oxidative cyclization to generate the spiroketal moiety (Fig. 20B).165 In addition, the flavin-containing Baeyer–Villiger monooxygenase (BVMO) PockeMO, from T. thermophila, catalyzes the insertion of a single oxygen atom into the D ring system of ASD (8) to generate 125 (Fig. 20C).166
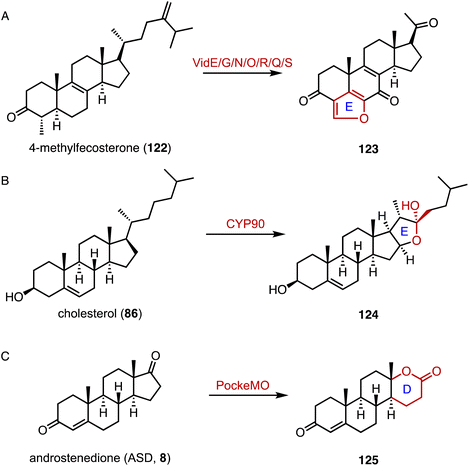 |
| Fig. 20 Ring system reconstruction. Ring contractions catalyzed by (A) VidE/G/N/O/R/Q/S and (B) CYP90. (C) Ring expansion catalyzed by PockeMO. | |
Chiang and coworkers identified the aed (actinobacterial oestrogen degradation) gene cluster, encoding estrogen-degrading genes, in the actinobacterium Rhodococcus sp. Strain B50.167 In the degradation pathway, the cytochrome P450 monooxygenase AedA is responsible for estrone (126) C-4 hydroxylation, and the 4-hydroxyestrone 4,5-dioxygenase AedB subsequently catalyzed the meta-cleavage of the estrogen A ring to generate pyridinestrone acid (PEA, 129) (Fig. 21).
 |
| Fig. 21 Ring reconstruction mediated by AedA/B. | |
11. Acylation
Acylation serves as a crucial strategy for enhancing the biological activities of steroid drugs, and stands as a significant avenue for drug modification.168 Achieving site-specific acylation through chemical methods remains challenging, due to the similar chemical environments of the hydroxyl groups in steroids.169,170 In contrast, enzymatic acylation provides a sustainable and alternative approach. For instance, Qiao and coworkers discovered a highly regio- and stereo-selective acetyltransferase, AmAT19, from Astragalus membranaceus.171 It catalyzed the 6α-OH acetylation of various steroids, producing the hyodeoxycholic acid 6-O-acetates 130, 131, and 132 (Fig. 22). Zhou and coworkers reported the structure of a human diacylglycerol O-acyltransferase 1 (DGAT1), which facilitates the conversion of cholesterol to cholesteryl ester and is crucial for cholesterol storage and transport.172 The cryogenic electron microscopy (cryo-EM) structure of human ACAT1 revealed a dimeric structure with nine transmembrane segments, forming a cytosolic and transmembrane tunnel for acyl-coenzyme A and cholesterol entry, respectively.173
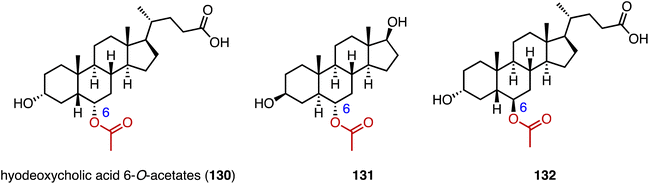 |
| Fig. 22 Structures of acylated steroids mediated by AmAT19. | |
Bacteria utilize aminoacyl-transfer RNAs (tRNAs) to aminoacylate membrane lipids, thus enhancing antimicrobial resistance and virulence, in reactions that have not yet been observed in eukaryotes.174–176 Becker and coworkers discovered that most fungi, including pathogens like Aspergillus fumigatus, utilize tRNA-dependent mechanisms to attach aspartate to ergosterol (133), forming ergosteryl-3β-O-L-aspartate (Erg-Asp, 134), a major sterol in fungal membranes.177 Aspartylation is catalyzed by ergosteryl-3β-O-L-aspartate synthase (ErdS), which transfers aspartate from Asp-tRNAAsp onto 133, and the removal of the Asp modifier from 134 is catalyzed by a dedicated hydrolase, ErdH (Fig. 23). The discovery of tRNA-dependent sterol aspartylation highlights a previously unrecognized aspect of fungal biology and offers potential targets for combating fungal infections.
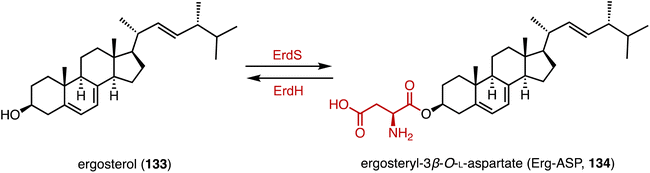 |
| Fig. 23 Aspartylation mediated by ErdS/ErdH. | |
12. Amination and amidation
Chiral amines are important building blocks in many pharmaceuticals and fine chemicals.178 Aminotransferases utilize the pyridoxal-5′-phosphate (PLP) cofactor to transfer an amino group from the amino donor to the targeted amino acceptor in a stereoselective fashion.179–181 For the efficient synthesis of 17α-amino steroids, Hailes and coworkers employed the ω-transaminase ArRMut1 for the transamination of 135 to 137 (88–90% yield, 83–89 isolated yield) (Fig. 24A).182 Prodanovic and coworkers also used this ω-transaminase for the conversion of dihydrotestosterone (138) to generate 139 (50% yield) (Fig. 24B).183 In the biosynthetic pathway of steroidal glycoalkaloids (SGAs), Mizutani and coworkers characterized PGA4, a C-26 aminotransferase, which catalyzes the transamination of 140 to produce 141 by introducing the nitrogen into the cholesterol backbone (Fig. 24C).184
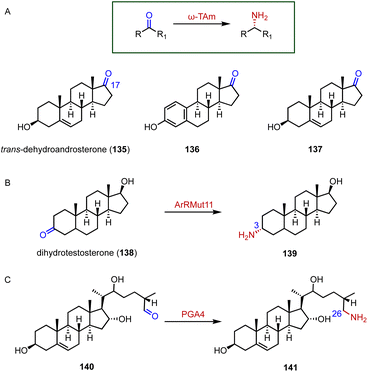 |
| Fig. 24 Aminations mediated by aminotransferases. (A) The substrate specificity of ω-TAm. Amination reactions catalyzed by (B) ArRMut1 and (C) PGA4. | |
Bacteria in the gut produce amino acid bile acid amidates that are important in host-mediated metabolic processes.185 Recently, Patterson's and Quinn's groups reported that bile salt hydrolase (BSH) not only plays a role in bile acid metabolism but also acts as an amine N-acyltransferase, forming bacterial bile acid amidates (BBAAs).186,187 BSH belongs to the N-terminal nucleophile superfamily of enzymes and catalyzes the hydrolysis of glycocholic acid (142) and taurocholic acid (143) to generate BBAAs (Fig. 25).
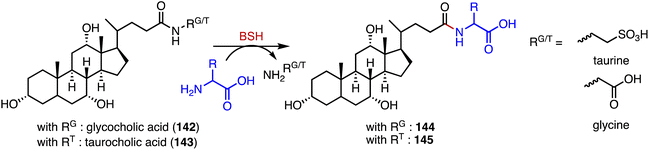 |
| Fig. 25 Amidation mediated by bile salt hydrolase (BSH). | |
13. Isomerization
Ketosteroid isomerase (KSI) catalyzes the rearrangement of the double bond at C-5 and C-6 in ketosteroids through an enolate intermediate, and a typical KSI reaction is shown in Fig. 26.188–190 Understanding how enzymes adapt to temperature is crucial for grasping molecular evolution and enzyme function. Herschlag and coworkers found that the temperature adaptation of KSI primarily stems from one residue change, indicating widespread parallel adaptation across organisms.191,192 Boxer and colleagues reported that an electric field in the active site of KSI aligns its substrate in the ground state, minimizing structural changes during activation to the transition state. These results support the proposal that the active site electric field in KSI is preorganized to enhance catalysis, offering a model for measuring electrostatic preorganization in enzymatic systems.193 Alexandrova and coworkers analyzed the electrostatic preorganization of KSI, and found that topologically similar local electric fields had similar reaction barriers.194
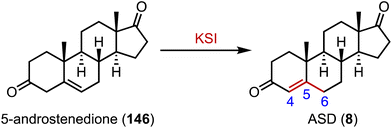 |
| Fig. 26 Isomerization mediated by ketosteroid isomerase (KSI). | |
14. Sulfonation
The human gut flora performs a variety of chemical modifications on endogenous and exogenous substances entering the gut, including hydrolysis, chemical bond breaking, and reductive reactions.195–197 The host-derived enzymes typically modify molecules through binding and oxidation reactions. An important example is sulfonation, the conversion of alcohols to sulfates via sulfotransferases (SULTs), which help organisms remove toxic substances and produce signaling molecules.198,199 Recently, Devlin's and Johnson's groups reported cholesterol (86) sulfonation by gut bacteria (Fig. 27).200,201 Johnson's group determined that cholesterol sulfate entered the circulation and assisted in regulating cholesterol levels,201 while Devlin's group found an immunoregulatory function of bacterially-produced cholesterol sulfate and the SULT-dependent inhibition of T cell migration.200
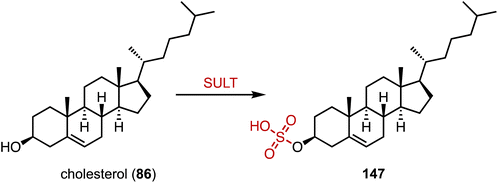 |
| Fig. 27 Sulfonation mediated by sulfotransferase (SULT). | |
15. Novel biosynthetic pathways
Natural products refer to the molecules created by microorganisms, plants, and animals through secondary metabolism. Compared with primary metabolites, they possess structural complexity and diversity, as well as attractive biological activities. Recently, novel biosynthetic pathways of steroid-related natural products, including furanosteroids, steroidal glycoalkaloids (SGAs), and diosgenin, have been clarified.
15.1 Furanosteroids
Furanosteroids are a class of fungal secondary metabolites represented by demethoxyviridin (148), demethoxyviridiol (149), viridin, and wortmannin,202,203 which are nanomolar-potency inhibitors of phosphatidylinositol 3-kinase (PI3K).204 Gao and coworkers characterized the biosynthetic pathway of 148 and 149.144 It shares a similar early step in the biosynthetic pathway to ergosterol (133). VidE, VidG, VidR, VidO, and VidN are responsible for converting 122 or 153 to 154 or 159. Subsequently, VidQ oxidizes the C-7 position to produce 154 or 158. VidS mediates the 1,4-dehydration reaction to form the typical furan ring. Afterwards, a Baeyer–Villiger monooxygenase (VidF), an esterase (VidP) and a dehydrogenase (VidH) cleave the C-17, 20 side chain to produce the intermediate 91 or 164 with a carbonyl group at C-17. Two P450 oxidases (VidD and VidA) catalyze the aromatization of the C-ring, and finally a P450 oxidase (VidK) catalyzes the 1β-hydroxylation of 166 or 162 to generate demethoxyviridin (148) or demethoxyviridiol (149), respectively. The oxidase (VidJ) and the dehydrogenase (VidM) are responsible for the mutual conversion between the 3-OH and 3-keto groups in the pathway (Fig. 28). The biosynthetic pathway of 148 and 149 is widespread and conserved in fungi.
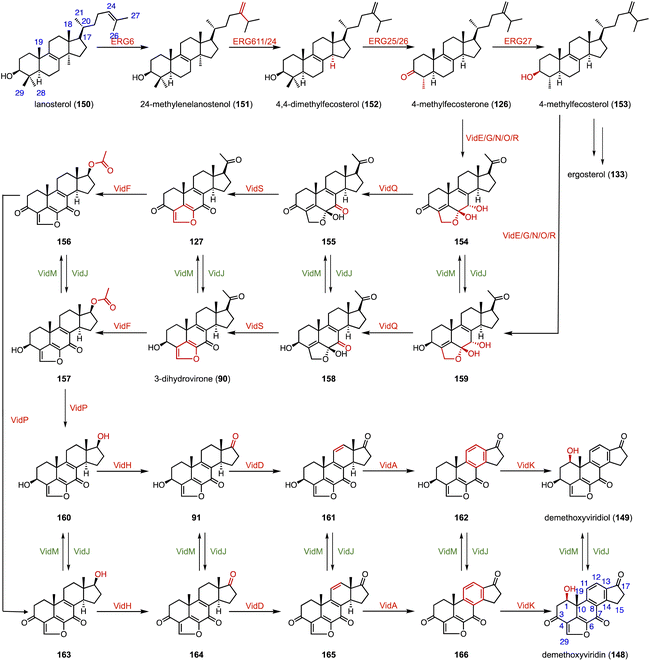 |
| Fig. 28 Biosynthetic pathway of demethoxyviridin and demethoxyviridiol. | |
15.2 Steroidal glycoalkaloids (SGAs)
SGAs are toxic specialized metabolites found in members of the Solanaceae, such as tomato, potato, and eggplant.205–209 They are classified into solanidane and spirosolane, based on their skeleton derived from the cholesterol side chain.210,211 Recently, Mizutani's, Aharoni's, and other research groups identified the biosynthetic pathway of SGAs.184,212–218 As shown in Fig. 29, two P450 enzymes (PGA2, PGA1) and the αKG OX 16DOX catalyze the hydroxylation of cholesterol (86) to generate 167, which is then converted to 168 by a series of enzymes, including PGA3 and PGA4. In addition, diverse uridine diphosphate-dependent glycosyltransferases (UGTs) are responsible for the glycosylation of SGAs to produce α-tomatine (170), dehydrotomatine (171), α-solamarine (172), and β-solamarine (173). Notably, the spirosolane moiety is tailored by diverse enzymes for branching the biosynthetic pathways of SGAs. DPS (dioxygenase for potato solanidane synthesis) catalyzes the C-16 hydroxylation of spirosolane-type SGAs, resulting in desaturation via E/F ring arrangement to generate the solanidane scaffold. GAME34 (glycoalkaloid metabolism34), an αKG OX, catalyzes the conversion of α-tomatine (170) to habrochaitoside (178). GAME31 catalyzes the C-23 hydroxylation of α-tomatine (170), and the product spontaneously isomerized to 179. The intermediate 162 was then converted to 180 by a putative acetyltransferase. GAME40 functions as a C-27 hydroxylase for the efficient detoxification of α-tomatine (170) during tomato fruit ripening.
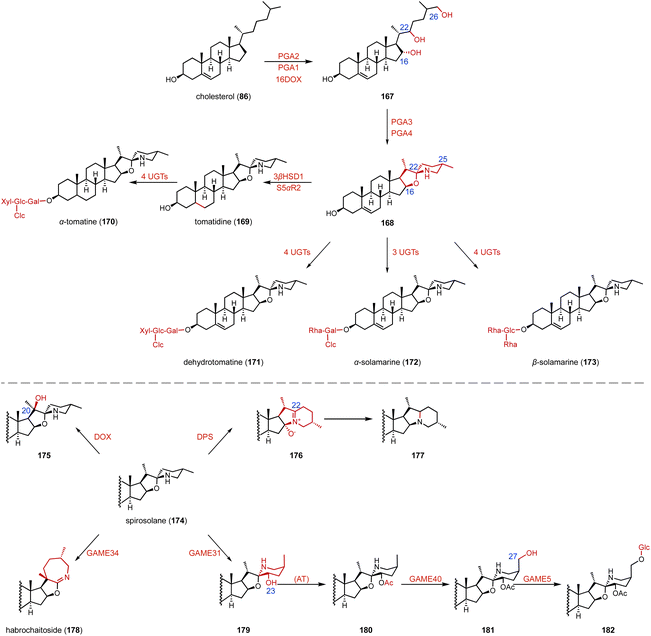 |
| Fig. 29 Biosynthetic pathway of steroidal glycoalkaloids (SGAs). | |
15.3 Diosgenin
Diosgenin (185), a vital precursor in the steroid hormone industry, is derived from plants.219 Weng and coworkers clarified the biosynthetic pathway of 185.165 Pairs of P450 enzymes, such as CYP90, CYP94, CYP72, and CYP82, are recruited for the formation of the characteristic 5,6-spiroketal moiety. In brassinosteroid biosynthesis, CYP90 mainly catalyzes the C-22β hydroxylation of 86 to generate 183 (Fig. 30).
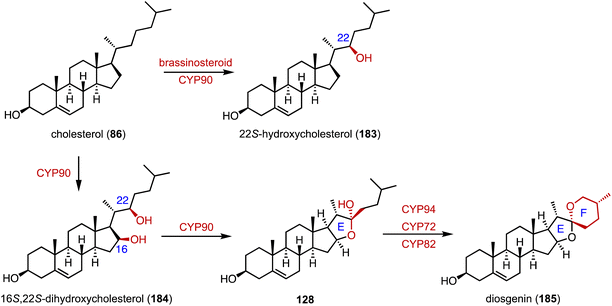 |
| Fig. 30 Biosynthetic pathway of diosgenin. | |
16. Artificial multi-enzyme cascades and biotransformations
Drawing inspiration from nature, multi-enzymatic cascades provide a cost-effective alternative to single-step reactions.220,221 These cascades integrate multiple catalytic steps within a single reaction vessel, streamlining the production of a diverse array of chemicals.222 The numbers of reports on enzymatic cascade reactions in the synthesis of steroid drugs continue to grow.223
Kong and coworkers discovered and functionally characterized a steroidal glycosyltransferase (SGT) from Ornithogalum saundersiae (OsSGT1) and a steroidal glycoside acyltransferase (SGA) from Escherichia coli (EcSGA1) for the biosynthesis of acylated steroidal glycosides, and demonstrated their improved cytotoxic activities against human tumor cell lines (Fig. 31A).224 Chang and coworkers developed a one-pot artificial cascade reaction involving three different P450 modules (17AM, 21M, and 11BM) for the biosynthesis of hydrocortisone (6).225 The optimized three-P450-module co-expression system, named hyg4, achieved the direct production of hydrocortisone (6) from PG (12) with a total yield of 16.82 μmol L−1 within 24 hours (Fig. 31B). Recently, Zhou and coworkers performed the protein engineering of P450 BM3 and a series of P450 BM3 enzyme variants were identified for C-16β, C-17α, C-21, and C-17α/21 hydroxylation of PG (12) and C-11α oxidation of 5.226 The developed P450 variants were utilized in the one pot reaction with 1 g L−112 as the starting material, resulting in C-11α/β-hydrocortisone production at a molar conversion rate of 81 and 84%, respectively (Fig. 31C).
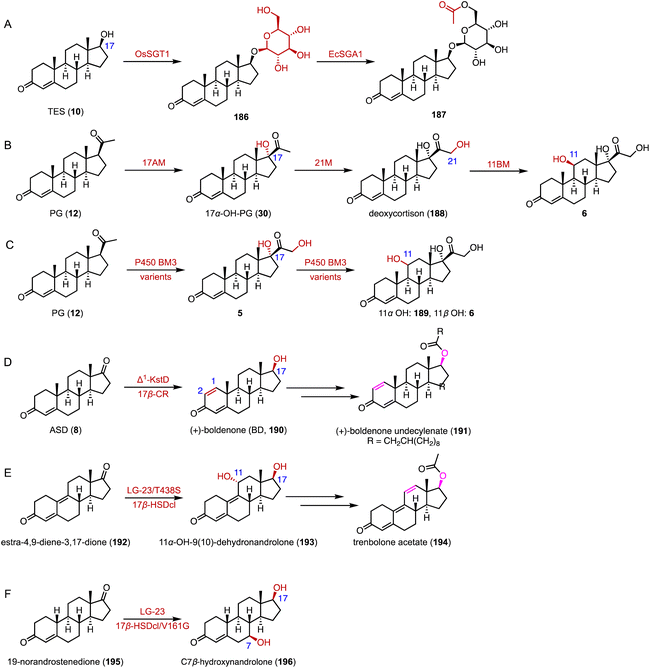 |
| Fig. 31 Enzymatic cascade reactions for the synthesis of steroid drugs. Enzymatic cascades for the synthesis of (A) the acylated steroidal glycoside 187, (B) hydrocortisone (6), (C) 6 and 189, (D) (+)-boldenone (190), (E) 11α-OH-9(10)-dehydronandrolone (193), and (F) 7β-hydroxynandrolone (196). | |
Chen and coworkers utilized the engineered KstD ReM2 (I51L/I350T) and the newly discovered carbonyl reductase 17β-CR to synthesize (+)-boldenone (BD, 190) from ASD (8), which is a key intermediate for the synthesis of (+)-boldenone undecylenate (191) (Fig. 31D).227
Li and coworkers performed the biocatalytic one-pot C-11α hydroxylation (LG-23/T438S) and 17β-ketoreduction (17β-HSDcl) of estra-4,9-diene-3,17-dione (192).228 The product 193 is a key intermediate for the synthesis of the veterinary medicine trenbolone acetate (194) (Fig. 31E). Meanwhile, Li and coworkers established a biocatalytic cascade reaction including the C-7β hydroxylation (LG-23) and C-17β ketoreduction (17β-HSDcl/V161G) of 19-norandrostenedione (195) to synthesize 7β-hydroxynandrolone (196), which is an important precursor for the synthesis of C-7 functionalized steroid drugs (Fig. 31F).229
Finally, microbiological transformation is a powerful and green tool in the manufacturing of steroids.230 Recently, Zhu and coworkers knocked out of a β-hydroxyacyl-CoA dehydrogenase gene and introduced a sterol aldolase gene in the genetically modified strains of Mycobacterium fortuitum N13, resulting in the strain N13Δhsd4AΩthl.231 It transformed phytosterols into 3-((1R,3aS,4S,7aR)-1-((S)-1-hydroxypropan-2-yl)-7a-methyl-5-oxooctahydro-1H-inden-4-yl) propanoic acid (HIP-IPA, 197) in 62% isolated yield, which is a key intermediate for the synthesis of dydrogesterone (Fig. 32).
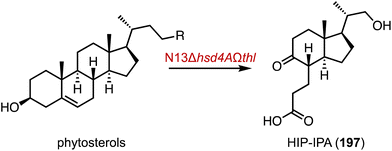 |
| Fig. 32 Biotransformation of phytosterols into 197. | |
17. Conclusions
Over the past five years, diverse enzymatic modifications of steroids have attracted keen interest, significantly advancing our understanding of biocatalytic steroid drug synthesis. These enzymatic transformations offer precise control of stereochemistry and regioselectivity, particularly the enzymatic hydroxylation, ketoreduction, glycosylation and acylation of steroids. For example, the recently discovered C-14α hydroxylase could be utilized for the preparation of the important intermediates for the synthesis of cardiac glycosides. Meanwhile, the substrate promiscuity of hydroxylases, such as the αKG OX and UPO, provides an alternative strategy for the site-specific hydroxylation of steroids.
Synthetic biology is revolutionizing the industrial production of steroid drugs through concerted efforts in metabolic engineering, enzyme discovery, and enzyme engineering that are new to both biology and chemistry. The enzymatic synthesis of steroids has not only broadened the substrate scope but also opened avenues for the generation of novel derivatives with improved therapeutic properties. Future research on steroid biosynthesis will characterize the intriguing biochemical reactions, the cryptic enzymatic mechanisms, and the biological significance of diverse steroid modifications, such as the sterol aspartylation in fungi and sulfonation in gut bacteria.
In summary, harnessing the power of enzymes is paving the way toward streamlining and enhancing the synthetic routes of steroids, ultimately accelerating the discovery and development of unique steroid drugs.
Conflicts of interest
There are no conflicts to declare.
Acknowledgements
This work was supported in part by a Grant-in-Aid for Scientific Research from the Ministry of Education, Culture, Sports, Science and Technology, Japan (JSPS KAKENHI Grant Number JP20KK0173, JP21K18246, and JP23H00393), the New Energy and Industrial Technology Development Organization (NEDO, Grant Number JPNP20011), AMED (Grant Number JP21ak0101164) from Japan Science and Technology Agency.
References
- N. Sultana, Steroids, 2018, 136, 76–92 CrossRef CAS PubMed
.
- W. Ericson-Neilsen and A. D. Kaye, Ochsner J., 2014, 14, 203–207 Search PubMed
.
- F. Mao, W. Ni, X. Xu, H. Wang, J. Wang, M. Ji and J. Li, Molecules, 2016, 21, 75 CrossRef PubMed
.
- D. E. Yerien, S. Bonesi and A. Postigo, Org. Biomol. Chem., 2016, 14, 8398–8427 RSC
.
- S. Gorog, Anal. Sci., 2004, 20, 767–782 CrossRef PubMed
.
- Z. Zhang, X. Qian, Y. Gu and J. Gui, Nat. Prod. Rep., 2024, 41, 251–272 RSC
.
- Y. Wang and J. Gui, Acc. Chem. Res., 2024, 57, 568–579 CAS
.
- J. B. Pyser, S. Chakrabarty, E. O. Romero and A. R. H. Narayan, ACS Cent. Sci., 2021, 7, 1105–1116 CrossRef CAS PubMed
.
- W. Y. Tong and X. Dong, Recent Pat. Biotechnol., 2009, 3, 141–153 CrossRef CAS PubMed
.
- S. K. Wu, R. Snajdrova, J. C. Moore, K. Baldenius and U. T. Bornscheuer, Angew. Chem., Int. Ed., 2021, 60, 88–119 CrossRef CAS PubMed
.
- E. Romero, B. S. Jones, B. N. Hogg, A. Rue Casamajo, M. A. Hayes, S. L. Flitsch, N. J. Turner and C. Schnepel, Angew. Chem., Int. Ed., 2021, 60, 16824–16855 CrossRef CAS PubMed
.
- J. Chen, F. Fan, G. Qu, J. Tang, Y. Xi, C. Bi, Z. Sun and X. Zhang, Metab. Eng., 2020, 57, 31–42 CrossRef CAS PubMed
.
- W. J. Sun, L. Wang, H. H. Liu, Y. J. Liu, Y. H. Ren, F. Q. Wang and D. Z. Wei, Metab. Eng., 2019, 56, 97–110 CrossRef CAS PubMed
.
- M. M. Zheng, Z. Lin, S. J. Lin and X. D. Qu, Eur. J. Org. Chem., 2024, 27, e202301066 CrossRef CAS
.
- R. Zhu, Y. Liu, Y. Y. Yang, Q. Min, H. Li and L. X. Chen, Adv. Synth. Catal., 2022, 364, 2701–2719 CrossRef CAS
.
- H. Abas, P. Blencowe, J. L. Brookfield and L. A. Harwood, Chem. – Eur. J., 2023, 29, e202301066 CrossRef CAS PubMed
.
- X. Zhang, Y. Peng, J. Zhao, Q. Li, X. Yu, C. G. Acevedo-Rocha and A. Li, Bioresour. Bioprocess., 2020, 7, 2 CrossRef
.
- J. Cramer, C. P. Sager and B. Ernst, J. Med. Chem., 2019, 62, 8915–8930 CrossRef CAS PubMed
.
- J. Münch, P. Püllmann, W. Y. Zhang and M. J. Weissenborn, ACS Catal., 2021, 11, 9168–9203 CrossRef
.
- L. P. Zhao, Z. Y. Bo and Y. Yang, Nat. Synth., 2023, 2, 699–700 CrossRef PubMed
.
- J. Chen, J. Tang, Y. Xi, Z. Dai, C. Bi, X. Chen, F. Fan and X. Zhang, Appl. Microbiol. Biotechnol., 2019, 103, 8363–8374 CrossRef CAS PubMed
.
- D. Permana, K. Niesel, M. J. Ford and H. Ichinose, ACS Omega, 2022, 7, 13932–13941 CrossRef CAS PubMed
.
- Y. Zhao, B. Zhang, Z. Q. Sun, H. Zhang, W. Wang, Z. R. Wang, Z. K. Guo, S. Y. Yu, R. X. Tan and H. M. Ge, ACS Catal., 2022, 12, 9839–9845 CrossRef CAS
.
- F. Z. Song, M. M. Zheng, J. L. Wang, H. H. Liu, Z. Lin, B. B. Liu, Z. X. Deng, H. J. Cong, Q. H. Zhou and X. D. Qu, Nat. Synth., 2023, 2, 729–739 CrossRef
.
- T. Terasawa and T. Okada, Tetrahedron, 1986, 42, 537–545 CrossRef CAS
.
- H. Renata, Q. H. Zhou, G. Dünstl, J. Felding, R. R. Merchant, C. H. Yeh and P. S. Baran, J. Am. Chem. Soc., 2015, 137, 1330–1340 CrossRef CAS PubMed
.
- J. Lia, A. Amatuni and H. Renata, Curr. Opin. Chem. Biol., 2020, 55, 111–118 CrossRef PubMed
.
- W. Lu, X. Chen, J. Feng, Y. J. Bao, Y. Wang, Q. Wu and D. Zhu, Appl. Environ. Microbiol., 2018, 84, e00503-18 CrossRef PubMed
.
- J. L. Wang, Y. N. Zhang, H. H. Liu, Y. Shang, L. J. Zhou, P. L. Wei, W. B. Yin, Z. X. Deng, X. D. Qu and Q. H. Zhou, Nat. Commun., 2019, 10, 3378 CrossRef PubMed
.
- D. Suraweera, H. Rahal, M. Jimenez, M. Viramontes, G. Choi and S. Saab, Liver Int., 2017, 37, 1877–1886 CrossRef PubMed
.
- S. Fiorucci and E. Distrutti, Handb. Exp. Pharmacol., 2019, 256, 265–282 CAS
.
- Z. Wang and C. Hui, Org. Biomol. Chem., 2021, 19, 3791–3812 RSC
.
- F. Tonin and I. W. C. E. Arends, Beilstein J. Org. Chem., 2018, 14, 470–483 CrossRef CAS PubMed
.
- S. Grobe, C. P. S. Badenhorst, T. Bayer, E. Hamnevik, S. Wu, C. W. Grathwol, A. Link, S. Koban, H. Brundiek, B. Grossjohann and U. T. Bornscheuer, Angew. Chem., Int. Ed., 2021, 60, 753–757 CrossRef CAS PubMed
.
- S. Grobe, A. Wszolek, H. Brundiek, M. Fekete and U. T. Bornscheuer, Biotechnol. Lett., 2020, 42, 819–824 CrossRef CAS PubMed
.
- A. Li, C. G. Acevedo-Rocha, L. D'Amore, J. Chen, Y. Peng, M. Garcia-Borras, C. Gao, J. Zhu, H. Rickerby, S. Osuna, J. Zhou and M. T. Reetz, Angew. Chem., Int. Ed., 2020, 59, 12499–12505 CrossRef CAS PubMed
.
- B. Ma, Q. Wang, H. Ikeda, C. Zhang and L. H. Xu, Appl. Environ. Microbiol., 2019, 85, e01530-19 CrossRef PubMed
.
- C. G. Acevedo-Rocha, C. G. Gamble, R. Lonsdale, A. T. Li, N. Nett, S. Hoebenreich, J. B. Lingnau, C. Wirtz, C. Fares, H. Hinrichs, A. Deege, A. J. Mulholland, Y. Nov, D. Leys, K. J. McLean, A. W. Munro and M. T. Reetz, ACS Catal., 2018, 8, 3395–3410 CrossRef CAS
.
- X. D. Zhang, Y. Hu, W. Peng, C. H. Gao, Q. Xing, B. J. Wang and A. T. Li, Front. Chem., 2021, 9, 649000 CrossRef CAS PubMed
.
- W. Y. Chen, M. J. Fisher, A. Leung, Y. Cao and L. L. Wong, ACS Catal., 2020, 10, 8334–8343 CrossRef CAS
.
- J. Yang, Y. Liu, D. Zhong, L. Xu, H. Gao, J. D. Keasling, X. Luo and H. H. Chou, Metab. Eng., 2023, 80, 119–129 CrossRef CAS PubMed
.
- Y. Yang and F. H. Arnold, Acc. Chem. Res., 2021, 54, 1209–1225 CrossRef CAS PubMed
.
- P. Bracco, H. J. Wijma, B. Nicolai, J. A. R. Buitrago, T. Klünemann, A. Vila, P. Schrepfer, W. Blankenfeldt, D. B. Janssen and A. Schallmey, ChemBioChem, 2021, 22, 1099–1110 CrossRef CAS PubMed
.
- Y. Khatri, I. K. Jozwik, M. Ringle, I. A. Ionescu, M. Litzenburger, M. C. Hutter, A. M. W. H. Thunnissen and R. Bernhardt, ACS Chem. Biol., 2018, 13, 1021–1028 CrossRef CAS PubMed
.
- X. D. Zhang, P. P. Shen, J. Zhao, Y. Y. Chen, X. Li, J. W. Huang, L. L. Zhang, Q. Li, C. H. Gao, Q. Xing, C. C. Chen, R. T. Guo and A. T. Li, ACS Catal., 2023, 13, 1280–1289 CrossRef CAS
.
- Q. Gao, B. Ma, Q. Wang, H. Zhang, S. Fushinobu, J. Yang, S. Lin, K. Sun, B. N. Han and L. H. Xu, Appl. Environ. Microbiol., 2023, 89, e0218622 CrossRef PubMed
.
- N. Kumar, J. He and J. F. Rusling, Chem. Soc. Rev., 2023, 52, 5135–5171 RSC
.
- S. Fan and Z. Cong, Acc. Chem. Res., 2024, 57, 613–624 CAS
.
- J. Akter, E. F. Hayball and S. G. Bell, Catal. Sci. Technol., 2023, 13, 6355–6359 RSC
.
- X. W. Zhang, J. W. Guo, F. Y. Cheng and S. Y. Li, Nat. Prod. Rep., 2021, 38, 1072–1099 RSC
.
- S. L. Li, Y. W. Chang, Y. N. Liu, W. Tian and Z. X. Chang, J. Steroid Biochem. Mol. Biol., 2023, 227, 106236 CrossRef CAS PubMed
.
- G. Yi, H. Zou, T. Long, T. Osire, L. Wang, X. Wei, M. Long, Z. Rao and G. Liao, Bioresour. Technol., 2023, 394, 130244 CrossRef PubMed
.
- S. Mao, X. Wang, Z. Zhang, S. Wang, K. Li, F. Lu and H. Qin, Biochem. Eng. J., 2020, 164, 107781 CrossRef CAS
.
- W. Lu, J. H. Feng, X. Chen, Y. J. Bao, Y. Wang, Q. Q. Wu, Y. H. Ma and D. M. Zhu, Appl. Environ. Microbiol., 2019, 85, e01182-19 CrossRef PubMed
.
- V. Kollerov, S. Tarlachkov, A. Shutov, A. Kazantsev and M. Donova, Int. J. Mol. Sci., 2023, 24, 17256 CrossRef CAS PubMed
.
- L. Wang, X. Wu, C. Gao, L. Wei, Q. Li and A. Li, Appl. Environ. Microbiol., 2023, 89, e0196322 CrossRef PubMed
.
- X. Wang, X. Yang, X. Jia, P. Jin, Z. Wang, F. Lu and X. Liu, Ann. Microbiol., 2020, 70, 45 CrossRef CAS
.
- Y. Peng, Y. Wang, T.-J. Chen, J.-J. Chen, J.-L. Yang, T. Gong and P. Zhu, Chin. Chem. Lett., 2024, 35, 108818 CrossRef CAS
.
- P. He, H. Li, J. Sun, X. M. Zhang, J. S. Gong, J. S. Shi and Z. H. Xu, J. Steroid Biochem. Mol. Biol., 2022, 220, 106096 CrossRef CAS PubMed
.
- P. Subedi, K. H. Kim, Y. S. Hong, J. H. Lee and T. J. Oh, J. Microbiol. Biotechnol., 2021, 31, 464–474 CrossRef CAS PubMed
.
- Q. Wang, B. Ma, S. Fushinobu, C. Zhang and L. H. Xu, Biochem. Biophys. Res. Commun., 2020, 522, 355–361 CrossRef CAS PubMed
.
- C. Liu, K. Chen, Y. Wang, M. Shao, Z. Xu and Z. Rao, Biochem. Eng. J., 2022, 177, 108264 CrossRef CAS
.
- I. K. Jozwik, E. Bombino, A. Abdulmughni, P. Hartz, H. J. Rozeboom, H. J. Wijma, R. Kappl, D. B. Janssen, R. Bernhardt and A. W. H. Thunnissen, FEBS J., 2023, 290, 5016–5035 CrossRef CAS PubMed
.
- S. M. Glass, M. J. Reddish, S. A. Child, C. J. Wilkey, D. F. Stec and F. P. Guengerich, J. Steroid Biochem. Mol. Biol., 2021, 208, 105787 CrossRef CAS PubMed
.
- S. S. Gao, N. Naowarojna, R. Cheng, X. Liu and P. Liu, Nat. Prod. Rep., 2018, 35, 792–837 RSC
.
- C. Q. Herr and R. P. Hausinger, Trends Biochem. Sci., 2018, 43, 517–532 CrossRef CAS PubMed
.
- R. Ushimaru and I. Abe, ACS Catal., 2022, 1045–1076, DOI:10.1021/acscatal.2c05247
.
- C. R. Zwick and H. Renata, ACS Catal., 2023, 13, 4853–4865 CrossRef
.
- A. Amatuni and H. Renata, Org. Biomol. Chem., 2019, 17, 1736–1739 RSC
.
- W. L. Cheung-Lee, J. N. Kolev, J. A. McIntosh, A. A. Gil, W. Pan, L. Xiao, J. E. Velasquez, R. Gangam, M. S. Winston, S. Li, K. Abe, E. Alwedi, Z. E. X. Dance, H. Fan, K. Hiraga, J. Kim, B. Kosjek, D. N. Le, N. Salehi Marzijarani, K. Mattern, J. P. McMullen, K. Narsimhan, A. Vikram, W. Wang, J. X. Yan, R. S. Yang, V. Zhang, W. Zhong, D. A. DiRocco, W. J. Morris, G. S. Murphy and K. M. Maloney, Angew. Chem., Int. Ed., 2024, e202316133, DOI:10.1002/anie.202316133
.
- M. Nakayasu, N. Umemoto, K. Ohyama, Y. Fujimoto, H. J. Lee, B. Watanabe, T. Muranaka, K. Saito, Y. Sugimoto and M. Mizutani, Plant Physiol., 2017, 175, 120–133 CrossRef CAS PubMed
.
- H. Tao, T. Mori, H. Chen, S. Lyu, A. Nonoyama, S. Lee and I. Abe, Nat. Commun., 2022, 13, 95 CrossRef CAS PubMed
.
- T. Bai, Y. Matsuda, H. Tao, T. Mori, Y. Zhang and I. Abe, Org. Lett., 2020, 22, 4311–4315 CrossRef CAS PubMed
.
- T. Mori, Z. Yu, H. Tao and I. Abe, Org. Lett., 2022, 24, 1737–1741 CrossRef CAS PubMed
.
- M. Petrusma, R. van der Geize and L. Dijkhuizen, Antonie Van Leeuwenhoek, 2014, 106, 157–172 CrossRef CAS PubMed
.
- H. H. Liu, L. Q. Xu, K. Yao, L. B. Xiong, X. Y. Tao, M. Liu, F. Q. Wang and D. Z. Wei, Appl. Environ. Microbiol., 2018, 84, e02777-17 CrossRef PubMed
.
- H. Sun, J. Yang, K. He, Y.-P. Wang and H. Song, Chem. Eng. Sci., 2021, 230, 116195 CrossRef CAS
.
- S. Baldanta, J. M. Navarro Llorens and G. Guevara, Microorganisms, 2021, 9, 1171 CrossRef CAS PubMed
.
- Z. L. Zhu, X. Gao, Z. Song, C. Li, F. P. Lu, M. Tanokura and H. M. Qin, ACS Sustainable Chem. Eng., 2020, 8, 16720–16730 CrossRef CAS
.
- H. Song, Z. Zhang, C. Cao, Z. Tang, J. Gui and W. Liu, Angew. Chem., Int. Ed., 2024, e202319624, DOI:10.1002/anie.202319624
.
- C. Aranda, J. Carro, A. Gonzalez-Benjumea, E. D. Babot, A. Olmedo, D. Linde, A. T. Martinez and A. Gutierrez, Biotechnol. Adv., 2021, 51, 107703 CrossRef CAS PubMed
.
- A. Kinner, K. Rosenthal and S. Lutz, Front. Bioeng. Biotechnol., 2021, 9, 705630 CrossRef PubMed
.
- A. Beltran-Nogal, I. Sanchez-Moreno, D. Mendez-Sanchez, P. Gomez de Santos, F. Hollmann and M. Alcalde, Curr. Opin. Struct. Biol., 2022, 73, 102342 CrossRef CAS PubMed
.
- J. Kiebist, K. U. Schmidtke, J. Zimmermann, H. Kellner, N. Jehmlich, R. Ullrich, D. Zander, M. Hofrichter and K. Scheibner, ChemBioChem, 2017, 18, 563–569 CrossRef CAS PubMed
.
- E. J. de Vries and D. B. Janssen, Curr. Opin. Biotechnol, 2003, 14, 414–420 CrossRef CAS PubMed
.
- S. Meninno and A. Lattanzi, ACS Org. Inorg. Au, 2022, 2, 289–305 CrossRef CAS PubMed
.
- F. K. Yoshimoto, H. M. Peng, H. Zhang, S. M. Anderson and R. J. Auchus, Biochemistry, 2014, 53, 7531–7540 CrossRef CAS PubMed
.
- D. Jiang, R. Tu, P. Bai and Q. Wang, Biotechnol. Lett., 2013, 35, 1663–1668 CrossRef CAS PubMed
.
- G. W. Huisman, J. Liang and A. Krebber, Curr. Opin. Chem. Biol., 2010, 14, 122–129 CrossRef CAS PubMed
.
- E. E. Ferrandi, S. Bertuletti, D. Monti and S. Riva, Eur. J. Org. Chem., 2020, 2020, 4463–4473 CrossRef CAS
.
- M. J. Badran, N. Bertoletti, A. Keils, A. Heine, G. Klebe and S. Marchais-Oberwinkler, J. Steroid Biochem. Mol. Biol., 2019, 189, 135–144 CrossRef CAS PubMed
.
- Z. Y. Liu, R. Z. Zhang, W. C. Zhang and Y. Xu, Crit. Rev. Biotechnol., 2023, 43, 770–786 CrossRef CAS PubMed
.
- S. C. Shi, Z. N. You, K. Zhou, Q. Chen, J. Pan, X. L. Qian, J. H. Xu and C. X. Li, Adv. Synth. Catal., 2019, 361, 4661–4668 CrossRef CAS
.
- Y. Q. Zhao, Y. J. Liu, W. T. Ji, K. Liu, B. Gao, X. Y. Tao, M. Zhao, F. Q. Wang and D. Z. Wei, Microb. Cell Fact., 2022, 21, 59 CrossRef CAS PubMed
.
- W. L. Liu, W. Shen, Y. Y. Xia, X. Z. Chen and H. Q. Yang, ACS Sustainable Chem. Eng., 2024, 12, 1333–1342 CrossRef CAS
.
- F. Tonin, L. G. Otten and I. Arends, ChemSusChem, 2019, 12, 3192–3203 CrossRef CAS PubMed
.
- Z. N. You, Q. Chen, S. C. Shi, M. M. Zheng, J. Pan, X. L. Qian, C. X. Li and J. H. Xu, ACS Catal., 2019, 9, 466–473 CrossRef CAS
.
- B.-Y. Yang, Z.-N. You, J.-T. Xue, J. Pan, C.-X. Li and J.-H. Xu, Mol. Catal., 2023, 537, 112946 CrossRef CAS
.
- K. A. Scott, M. H. Qureshi, P. B. Cox, C. M. Marshall, B. C. Bellaire, M. Wilcox, B. A. R. Stuart and J. T. Njardarson, J. Med. Chem., 2020, 63, 15449–15482 CrossRef CAS PubMed
.
- C. Zeng, S. Xu, J. Shen, S. Zhao, X. Xu and L. Peng, Org. Lett., 2024, 26, 127–131 CrossRef CAS PubMed
.
- Y. Hou, J. Lv, Y. Guo, J. Fang, C. Huang, W. Zhang, X. Jia, S. You and B. Qin, Mol. Catal., 2023, 548, 113433 CrossRef CAS
.
- H. Peng, Y. Wang, K. Jiang, X. Chen, W. Zhang, Y. Zhang, Z. Deng and X. Qu, Angew. Chem., Int. Ed., 2021, 60, 5414–5420 CrossRef CAS PubMed
.
- B. Zhang, D.-F. Zhou, M.-J. Li, J.-H. Lan, H. Li, M.-L. Shao, Z.-Q. Liu and Y.-G. Zheng, Syst. Microbiol. Biomanuf., 2024, 74, 631–660 CrossRef
.
- S. Mao, J. Sun, L. Wang, X. Gao, X. Liu, F. Lu and H.-M. Qin, Biochem. Eng. J., 2022, 181, 108383 CrossRef CAS
.
- Y. Wang, R. Zhang, J. Feng, Q. Wu, D. Zhu and Y. Ma, Microorganisms, 2022, 10, 508 CrossRef CAS PubMed
.
- R. Zhang, X. Xu, H. Cao, C. Yuan, Y. Yuminaga, S. Zhao, J. Shi and B. Zhang, Appl. Microbiol. Biotechnol., 2019, 103, 6605–6616 CrossRef CAS PubMed
.
- J. M. Luo, H. L. Cui, H. C. Jia, F. Li, H. J. Cheng, Y. B. Shen and M. Wang, J. Agric. Food Chem., 2020, 68, 9496–9512 CrossRef CAS PubMed
.
- S. Mao, Y. Chen, J. Sun, C. Wei, Z. Song, F. Lu and H. M. Qin, Enzyme Microb. Technol., 2021, 146, 109777 CrossRef CAS PubMed
.
- X. C. Liu, R. J. Zhang, Z. W. Bao, C. Y. Yuan, H. J. Cao, J. P. Shi, J. S. Sun and B. G. Zhang, Microb. Cell Fact., 2023, 22, 53 CrossRef CAS PubMed
.
- M. Tataruch, P. Wójcik, A. M. Wojtkiewicz, K. Zaczyk, K. Szymanska and M. Szaleniec, Catalysts, 2020, 10, 1460 CrossRef CAS
.
- B. M. D'Arcy, M. R. Swingle, L. Schambeau, L. Pannell, A. Prakash and R. E. Honkanen, Sci. Rep., 2019, 9, 5969 CrossRef PubMed
.
- X. Li, T. Chen, F. Peng, S. Song, J. Yu, D. N. Sidoine, X. Cheng, Y. Huang, Y. He and Z. Su, Microb. Cell Fact., 2021, 20, 158 CrossRef CAS PubMed
.
- J. Tang, C. L. Zeng, L. Y. Xie, J. H. Wang, M. Tian and C. C. Guo, Chem. Lett., 2018, 47, 110–112 CrossRef CAS
.
- Y. Zhang, M. Liu, H. Wang, J. Lin and F. Chen, Mol. Catal., 2022, 531, 112661 CrossRef CAS
.
- J. H. Wei, Y. J. Zhang, M. J. Liu, Y. T. Ning, Y. R. Cao and F. E. Chen, Angew. Chem., Int. Ed., 2024, 63, e2023139 Search PubMed
.
- R. Wilcken, M. O. Zimmermann, A. Lange, A. C. Joerger and F. M. Boeckler, J. Med. Chem., 2013, 56, 1363–1388 CrossRef CAS PubMed
.
- W. J. Chung and C. D. Vanderwal, Angew. Chem., Int. Ed., 2016, 55, 4396–4434 CrossRef CAS PubMed
.
- D. C. Blakemore, L. Castro, I. Churcher, D. C. Rees, A. W. Thomas, D. M. Wilson and A. Wood, Nat. Chem., 2018, 10, 383–394 CrossRef CAS PubMed
.
- B. F. Fisher, H. M. Snodgrass, K. A. Jones, M. C. Andorfer and J. C. Lewis, ACS Cent. Sci., 2019, 5, 1844–1856 CrossRef CAS PubMed
.
- E. Undiano, R. Roman, A. Miranda-Molina and M. Ayala, Nat. Prod. Res., 2022, 36, 5353–5357 CrossRef CAS PubMed
.
- C. Wen, W. Huang, M. M. He, W. L. Deng and H. H. Yu, Biotechnol. Lett., 2020, 42, 135–142 CrossRef CAS PubMed
.
- C. Wen, W. Huang, X. L. Zhu, F. Zhang and R. W. Jiang, Org. Lett., 2018, 20, 534–537 CrossRef CAS PubMed
.
- P. Chaturvedi, P. Misra and R. Tuli, Appl. Biochem. Biotechnol., 2011, 165, 47–68 CrossRef CAS PubMed
.
- P. Mladenka, L. Applova, J. Patocka, V. M. Costa, F. Remiao, J. Pourova, A. Mladenka, J. Karlickova, L. Jahodar, M. Voprsalova, K. J. Varner, M. Sterba, O. E. R. Tox, C. H. K. Researchers and Collaborators, Med. Res. Rev., 2018, 38, 1332–1403 CrossRef CAS PubMed
.
- G. Bati, J. X. He, K. B. Pal and X. W. Liu, Chem. Soc. Rev., 2019, 48, 4006–4018 RSC
.
- B. He, X. Bai, Y. Tan, W. Xie, Y. Feng and G. Y. Yang, Synth. Syst. Biotechnol., 2022, 7, 602–620 CrossRef CAS PubMed
.
- W. Song, C. C. Zhang, J. L. Wu, J. Z. Qi, X. Hua, L. P. Kang, Q. Yuan, J. F. Yuan and Z. Y. Xue, ACS Synth. Biol., 2022, 11, 1669–1680 CrossRef CAS PubMed
.
- J. Gao, Y. Xu, C. Hua, C. Li and Y. Zhang, Front. Plant Sci., 2021, 12, 809579 CrossRef PubMed
.
- M. He, S. Guo, Y. Yin, C. Zhang and X. Zhang, Mol. Biol. Rep., 2023, 50, 2137–2146 CrossRef CAS PubMed
.
- S. Upadhyay, G. S. Jeena, Shikha and R. K. Shukla, Planta, 2018, 248, 519–544 CrossRef CAS PubMed
.
- L. Chen, Y. Zhang and Y. Feng, J. Struct. Biol., 2018, 204, 371–379 CrossRef CAS PubMed
.
- D. Warnecke, R. Erdmann, A. Fahl, B. Hube, F. Muller, T. Zank, U. Zahringer and E. Heinz, J. Biol. Chem., 1999, 274, 13048–13059 CrossRef CAS PubMed
.
- Y. Zhuang, G. Y. Yang, X. Chen, Q. Liu, X. Zhang, Z. Deng and Y. Feng, Metab. Eng., 2017, 42, 25–32 CrossRef CAS PubMed
.
- W. Huang, Y. He, R. W. Jiang, Z. X. Deng and F. Long, ACS Catal., 2022, 12, 2927–2937 CrossRef CAS
.
- W. Huang, X. L. Zhang, J. H. Li, J. X. Lv, Y. H. Wang, Y. He, J. Song, H. Ågren, R. W. Jiang, Z. X. Deng and F. Long, ACS Catal., 2023, 14, 475–488 CrossRef
.
- W. Huang, C. Wen, Z. R. Zhou, Z. H. Fu, A. Katz, A. Plotnikov, S. J. D. Karlish and R. W. Jiang, Adv. Synth. Catal., 2019, 361, 3114–3119 CrossRef CAS
.
- A. Zhao, X. Zhang, Y. Li, Z. Wang, Y. Lv, J. Liu, M. A. Alam, W. Xiong and J. Xu, Biotechnol. Adv., 2021, 53, 107860 CrossRef CAS PubMed
.
- R. Ullrich, M. Poraj-Kobielska, S. Scholze, C. Halbout, M. Sandvoss, M. J. Pecyna, K. Scheibner and M. Hofrichter, J. Inorg. Biochem., 2018, 183, 84–93 CrossRef CAS PubMed
.
- T. N. Yuan, M. Yang, K. Gehring and N. S. Sampson, Biochemistry, 2019, 58, 4224–4235 CrossRef CAS PubMed
.
- E. R. Simpson and G. S. Boyd, Eur. J. Biochem., 1967, 2, 275–285 CrossRef CAS PubMed
.
- C. Groschel, S. Tennakoon and E. Kallay, Adv. Pharmacol., 2015, 74, 413–458 Search PubMed
.
- E. Carroll, B. Ravi Gopal, I. Raghavan, M. Mukherjee and Z. Q. Wang, Nat. Commun., 2023, 14, 4042 CrossRef CAS PubMed
.
- M. Kunert, C. Langley, R. Lucier, K. Ploss, C. E. Rodriguez Lopez, D. A. Serna Guerrero, E. Rothe, S. E. O'Connor and P. D. Sonawane, Nat. Plants, 2023, 9, 1607–1617 CrossRef CAS PubMed
.
- G. Q. Wang, G. D. Chen, S. Y. Qin, D. Hu, T. Awakawa, S. Y. Li, J. M. Lv, C. X. Wang, X. S. Yao, I. Abe and H. Gao, Nat. Commun., 2018, 9, 1838 CrossRef PubMed
.
- E. Abdelraheem, B. Thair, R. F. Varela, E. Jockmann, D. Popadic, H. C. Hailes, J. M. Ward, A. M. Iribarren, E. S. Lewkowicz, J. N. Andexer, P. L. Hagedoorn and U. Hanefeld, ChemBioChem, 2022, 23, e202200212 CrossRef CAS PubMed
.
- Z. Lin, Z. Hu, L. Zhou, B. Liu, X. Huang, Z. Deng and X. Qu, Proc. Natl. Acad. Sci. U. S. A., 2023, 120, e2301389120 CrossRef CAS PubMed
.
- N. Sokolova, L. Zhang, S. Deravi, R. Oerlemans, M. R. Groves and K. Haslinger, ChemBioChem, 2023, 24, e202300076 CrossRef CAS PubMed
.
- M. O. Brown, B. O. Olagunju, J. L. Giner and P. V. Welander, Nat. Commun., 2023, 14, 1859 CrossRef CAS PubMed
.
- A. K. Lee, J. H. Wei and P. V. Welander, Nat. Commun., 2023, 14, 2904 CrossRef CAS PubMed
.
- D. Michellod, T. Bien, D. Birgel, M. Violette, M. Kleiner, S. Fearn, C. Zeidler, H. R. Gruber-Vodicka, N. Dubilier and M. Liebeke, Science, 2023, 380, 520–526 CrossRef CAS PubMed
.
- J. Brocks and I. Bobrovskiy, Science, 2023, 380, 455–456 CrossRef CAS PubMed
.
- P. H. Wang, Y. L. Chen, S. T. Wei, K. Wu, T. H. Lee, T. Y. Wu and Y. R. Chiang, Proc. Natl. Acad. Sci. U. S. A., 2020, 117, 1395–1403 CrossRef CAS PubMed
.
- M. Elias-Arnanz, Proc. Natl. Acad. Sci. U. S. A., 2020, 117, 1833–1835 CrossRef CAS PubMed
.
- G. A. Wolff, N. A. Lamb and J. R. Maxwell, Org. Geochem., 1986, 10, 965–974 CrossRef CAS
.
- N. Robinson, G. Eglinton, S. C. Brassell and P. A. Cranwell, Nature, 1984, 308, 439–442 CrossRef CAS
.
- K. D. McCarty, M. E. Sullivan, Y. Tateishi, T. Y. Hargrove, G. I. Lepesheva and F. P. Guengerich, J. Biol. Chem., 2023, 299, 104841 CrossRef CAS PubMed
.
- S. Kalita, S. Shaik and K. D. Dubey, ACS Catal., 2022, 12, 5673–5683 CrossRef CAS
.
- K. D. McCarty, Y. Tateishi, T. Y. Hargrove, G. I. Lepesheva and F. P. Guengerich, Angew. Chem., Int. Ed., 2024, e202317711, DOI:10.1002/anie.202317711
.
- D. Hu, Y. H. Gao, X. S. Yao and H. Gao, Curr. Opin. Chem. Biol., 2020, 59, 47–53 CrossRef CAS PubMed
.
- G. Di Nardo, C. Zhang, A. G. Marcelli and G. Gilardi, Int. J. Mol. Sci., 2021, 22, 631 CrossRef CAS PubMed
.
- F. K. Yoshimoto and F. P. Guengerich, J. Am. Chem. Soc., 2014, 136, 15016–15025 CrossRef CAS PubMed
.
- A. K. Lee, A. B. Banta, J. H. Wei, D. J. Kiemle, J. Feng, J. L. Giner and P. V. Welander, Proc. Natl. Acad. Sci. U. S. A., 2018, 115, 5884–5889 CrossRef CAS PubMed
.
- J. M. Lv, D. Hu, H. Gao, T. Kushiro, T. Awakawa, G. D. Chen, C. X. Wang, I. Abe and X. S. Yao, Nat. Commun., 2017, 8, 1644 CrossRef PubMed
.
- M. C. Tang, Y. Zou, K. Watanabe, C. T. Walsh and Y. Tang, Chem. Rev., 2017, 117, 5226–5333 CrossRef CAS PubMed
.
- B. Christ, C. Xu, M. Xu, F. S. Li, N. Wada, A. J. Mitchell, X. L. Han, M. L. Wen, M. Fujita and J. K. Weng, Nat. Commun., 2019, 10, 3206 CrossRef PubMed
.
- M. J. Furst, S. Savino, H. M. Dudek, J. R. Gomez Castellanos, C. Gutierrez de
Souza, S. Rovida, M. W. Fraaije and A. Mattevi, J. Am. Chem. Soc., 2017, 139, 627–630 CrossRef PubMed
.
- T. H. Hsiao, Y. L. Chen, M. Meng, M. R. Chuang, M. Horinouchi, T. Hayashi, P. H. Wang and Y. R. Chiang, Microb. Biotechnol., 2021, 14, 1212–1227 CrossRef CAS PubMed
.
- L. L. Wang, Z. H. Jiang, J. H. Zhang, K. Chen, M. Zhang, Z. L. Wang, B. J. Wang, M. Ye and X. Qiao, Nat. Commun., 2023, 14, 5969 CrossRef CAS PubMed
.
- Z. Chang, Y. Li, Y. H. Lu and H. Xiao, Enzyme Microb. Technol., 2023, 162, 110148 CrossRef CAS PubMed
.
- J. A. Lara, A. Burciaga-Monge, A. Chávez, M. Revés, R. Lavilla, M. Arró, A. Boronat, T. Altabella and A. Ferrer, Front. Plant Sci., 2018, 9, 588 CrossRef PubMed
.
- L. L. Wang, K. Chen, Z. L. Wang, Y. Yi, M. Zhang, A. Hasan, Y. Kuang, S. Shaker, R. Yu, H. T. Wang, H. Y. Liu, M. Ye and X. Qiao, Org. Biomol. Chem., 2021, 19, 7186–7189 RSC
.
- L. Wang, H. Qian, Y. Nian, Y. Han, Z. Ren, H. Zhang, L. Hu, B. V. V. Prasad, A. Laganowsky, N. Yan and M. Zhou, Nature, 2020, 581, 329–332 CrossRef CAS PubMed
.
- T. Long, E. W. Debler and X. Li, Curr. Opin. Struct. Biol., 2022, 74, 102369 CrossRef CAS PubMed
.
- E. M. Fozo and E. A. Rucks, Adv. Microb. Physiol., 2016, 69, 51–155 CrossRef CAS PubMed
.
- R. N. Fields and H. Roy, RNA Biol., 2018, 15, 480–491 CrossRef PubMed
.
- A. M. Smith, J. S. Harrison, C. D. Grube, A. E. Sheppe, N. Sahara, R. Ishii, O. Nureki and H. Roy, Mol. Microbiol., 2015, 98, 681–693 CrossRef CAS PubMed
.
- N. Yakobov, F. Fischer, N. Mahmoudi, Y. Saga, C. D. Grube, H. Roy, B. Senger, G. Grob, S. Tatematsu, D. Yokokawa, I. Mouyna, J. P. Latge, H. Nakajima, T. Kushiro and H. D. Becker, Proc. Natl. Acad. Sci. U. S. A., 2020, 117, 14948–14957 CrossRef CAS PubMed
.
- L. Banoth, N. S. Thakur, J. Bhaumik and U. C. Banerjee, Chirality, 2015, 27, 382–391 CrossRef CAS PubMed
.
- S. A. Kelly, S. Pohle, S. Wharry, S. Mix, C. C. R. Allen, T. S. Moody and B. F. Gilmore, Chem. Rev., 2018, 118, 349–367 CrossRef CAS PubMed
.
- M. Fuchs, J. E. Farnberger and W. Kroutil, Eur. J. Org. Chem., 2015, 6965–6982 CrossRef CAS PubMed
.
- K. Koper, S. W. Han, D. C. Pastor, Y. Yoshikuni and H. A. Maeda, J. Biol. Chem., 2022, 298, 102122 CrossRef CAS PubMed
.
- N. Richter, R. C. Simon, W. Kroutil, J. M. Ward and H. C. Hailes, Chem. Commun., 2014, 50, 6098–6100 RSC
.
- N. Kaličanin, G. Kovačević, M. Spasojević, O. Prodanović, S. Jovanović-Šanta, D. Škorić, D. Opsenica and R. Prodanović, Process Biochem., 2022, 121, 674–680 CrossRef
.
- M. Nakayasu, N. Umemoto, R. Akiyama, K. Ohyama, H. J. Lee, H. Miyachi, B. Watanabe, T. Muranaka, K. Saito, Y. Sugimoto and M. Mizutani, Plant J., 2021, 108, 81–92 CrossRef CAS PubMed
.
- R. A. Quinn, A. V. Melnik, A. Vrbanac, T. Fu, K. A. Patras, M. P. Christy, Z. Bodai, P. Belda-Ferre, A. Tripathi, L. K. Chung, M. Downes, R. D. Welch, M. Quinn, G. Humphrey, M. Panitchpakdi, K. C. Weldon, A. Aksenov, R. da Silva, J. Avila-Pacheco, C. Clish, S. Bae, H. Mallick, E. A. Franzosa, J. Lloyd-Price, R. Bussell, T. Thron, A. T. Nelson, M. Wang, E. Leszczynski, F. Vargas, J. M. Gauglitz, M. J. Meehan, E. Gentry, T. D. Arthur, A. C. Komor, O. Poulsen, B. S. Boland, J. T. Chang, W. J. Sandborn, M. Lim, N. Garg, J. C. Lumeng, R. J. Xavier, B. I. Kazmierczak, R. Jain, M. Egan, K. E. Rhee, D. Ferguson, M. Raffatellu, H. Vlamakis, G. G. Haddad, D. Siegel, C. Huttenhower, S. K. Mazmanian, R. M. Evans, V. Nizet, R. Knight and P. C. Dorrestein, Nature, 2020, 579, 123–129 CrossRef CAS PubMed
.
- B. Rimal, S. L. Collins, C. E. Tanes, E. R. Rocha, M. A. Granda, S. Solanki, N. J. Hoque, E. C. Gentry, I. Koo, E. R. Reilly, F. Hao, D. Paudel, V. Singh, T. Yan, M. S. Kim, K. Bittinger, J. P. Zackular, K. W. Krausz, D. Desai, S. Amin, J. P. Coleman, Y. M. Shah, J. E. Bisanz, F. J. Gonzalez, J. P. Vanden Heuvel, G. D. Wu, B. S. Zemel, P. C. Dorrestein, E. E. Weinert and A. D. Patterson, Nature, 2024, 626, 859–863 CrossRef CAS PubMed
.
- D. V. Guzior, M. Okros, M. Shivel, B. Armwald, C. Bridges, Y. Fu, C. Martin, A. L. Schilmiller, W. M. Miller, K. M. Ziegler, M. D. Sims, M. E. Maddens, S. F. Graham, R. P. Hausinger and R. A. Quinn, Nature, 2024, 626, 852–858 CrossRef CAS PubMed
.
- J. Skerlova, H. Lindstrom, E. Gonis, B. Sjodin, F. Neiers, P. Stenmark and B. Mannervik, FEBS Lett., 2020, 594, 1187–1195 CrossRef CAS PubMed
.
- B. Mannervik, A. Ismail, H. Lindstrom, B. Sjodin and N. H. Ing, Front. Mol. Biosci., 2021, 8, 765970 CrossRef CAS PubMed
.
- Y. Musdal, A. Ismail, B. Sjodin and B. Mannervik, Biomolecules, 2023, 13, 976 CrossRef CAS PubMed
.
- M. M. Pinney, D. A. Mokhtari, E. Akiva, F. Yabukarski, D. M. Sanchez, R. Liang, T. Doukov, T. J. Martinez, P. C. Babbitt and D. Herschlag, Science, 2021, 371, 1010 CrossRef PubMed
.
- F. Yabukarski, T. Doukov, M. M. Pinney, J. T. Biel, J. S. Fraser and D. Herschlag, Sci. Adv., 2022, 8, eabn7738 CrossRef CAS PubMed
.
- Y. Wu, S. D. Fried and S. G. Boxer, J. Am. Chem. Soc., 2020, 142, 9993–9998 CrossRef CAS PubMed
.
- M. R. Hennefarth and A. N. Alexandrova, ACS Catal., 2020, 10, 9915–9924 CrossRef CAS
.
- A. Pant, T. K. Maiti, D. Mahajan and B. Das, Microb. Ecol., 2023, 86, 97–111 CrossRef PubMed
.
- A. Y. M. Woo, M. A. Aguilar Ramos, R. Narayan, K. C. Richards-Corke, M. L. Wang, W. J. Sandoval-Espinola and E. P. Balskus, Nat. Rev. Chem., 2023, 7, 319–339 CrossRef CAS PubMed
.
- M. Funabashi, T. L. Grove, M. Wang, Y. Varma, M. E. McFadden, L. C. Brown, C. Guo, S. Higginbottom, S. C. Almo and M. A. Fischbach, Nature, 2020, 582, 566–570 CrossRef CAS PubMed
.
- W. J. Massey and J. M. Brown, Nat. Microbiol., 2022, 7, 1327–1328 CrossRef CAS PubMed
.
- G. D. D’Agostino, S. N. Chaudhari and A. S. Devlin, Nat. Chem. Biol., 2024, 20, 410–421 CrossRef PubMed
.
- L. Yao, G. D. D'Agostino, J. Park, S. Hang, A. A. Adhikari, Y. Zhang, W. Li, J. Avila-Pacheco, S. Bae, C. B. Clish, E. A. Franzosa, C. Huttenhower, J. R. Huh and A. S. Devlin, Nat. Microbiol., 2022, 7, 1404–1418 CrossRef CAS PubMed
.
- H. H. Le, M. T. Lee, K. R. Besler, J. M. C. Comrie and E. L. Johnson, Nat. Microbiol., 2022, 7, 1390–1403 CrossRef CAS PubMed
.
- B. K. Senapati, Org. Chem. Front., 2021, 8, 2608–2642 RSC
.
- P. Luo, J. H. Huang, J. M. Lv, G. Q. Wang, D. Hu and H. Gao, Nat. Prod. Rep., 2024 10.1039/d3np00052d
.
- G. Powis, R. Bonjouklian, M. M. Berggren, A. Gallegos, R. Abraham, C. Ashendel, L. Zalkow, W. F. Matter, J. Dodge and G. Grindey,
et al.
, Cancer Res., 1994, 54, 2419–2423 CAS
.
- G. Bianco, P. Schmitt-Kopplin, A. Crescenzi, S. Comes, A. Kettrup and T. R. Cataldi, Anal. Bioanal. Chem., 2003, 375, 799–804 CrossRef CAS PubMed
.
- C. Bailly, Steroids, 2021, 176, 108933 CrossRef CAS PubMed
.
- A. A. Egorova, N. A. Chalaya, I. N. Fomin, A. I. Barchuk and S. V. Gerasimova, Agronomy, 2022, 12, 462 CrossRef CAS
.
- P. D. Sonawane, A. Jozwiak, S. Panda and A. Aharoni, Curr. Opin. Plant Biol., 2020, 55, 118–128 CrossRef CAS PubMed
.
- D. K. Zhao, Y. Zhao, S. Y. Chen and E. J. Kennelly, Nat. Prod. Rep., 2021, 38, 1423–1444 RSC
.
- S. E. Milner, N. P. Brunton, P. W. Jones, N. M. O'Brien, S. G. Collins and A. R. Maguire, J. Agric. Food Chem., 2011, 59, 3454–3484 CrossRef CAS PubMed
.
- J. A. Delbrouck, M. Desgagne, C. Comeau, K. Bouarab, F. Malouin and P. L. Boudreault, Molecules, 2023, 28, 4957 CrossRef CAS PubMed
.
- P. D. Sonawane, U. Heinig, S. Panda, N. S. Gilboa, M. Yona, S. P. Kumar, N. Alkan, T. Unger, S. Bocobza, M. Pliner, S. Malitsky, M. Tkachev, S. Meir, I. Rogachev and A. Aharoni, Proc. Natl. Acad. Sci. U. S. A., 2018, 115, E5419–E5428 CrossRef CAS PubMed
.
- P. D. Cardenas, P. D. Sonawane, U. Heinig, A. Jozwiak, S. Panda, B. Abebie, Y. Kazachkova, M. Pliner, T. Unger, D. Wolf, I. Ofner, E. Vilaprinyo, S. Meir, O. Davydov, A. Gal-On, S. Burdman, A. Giri, D. Zamir, T. Scherf, J. Szymanski, I. Rogachev and A. Aharoni, Nat. Commun., 2019, 10, 5169 CrossRef PubMed
.
- R. Akiyama, M. Nakayasu, N. Umemoto, J. Kato, M. Kobayashi, H. J. Lee, Y. Sugimoto, Y. Iijima, K. Saito, T. Muranaka and M. Mizutani, Plant Cell Physiol., 2021, 62, 775–783 CrossRef CAS PubMed
.
- R. Akiyama, B. Watanabe, M. Nakayasu, H. J. Lee, J. Kato, N. Umemoto, T. Muranaka, K. Saito, Y. Sugimoto and M. Mizutani, Nat. Commun., 2021, 12, 1300 CrossRef CAS PubMed
.
- R. Akiyama, B. Watanabe, J. Kato, M. Nakayasu, H. J. Lee, N. Umemoto, T. Muranaka, K. Saito, Y. Sugimoto and M. Mizutani, Plant Cell Physiol., 2022, 63, 981–990 CrossRef CAS PubMed
.
- P. D. Sonawane, A. Jozwiak, R. Barbole, S. Panda, B. Abebie, Y. Kazachkova, S. A. Gharat, O. Ramot, T. Unger, G. Wizler, S. Meir, I. Rogachev, A. Doron-Faigenboim, M. Petreikov, A. Schaffer, A. P. Giri, T. Scherf and A. Aharoni, New Phytol., 2022, 234, 1394–1410 CrossRef CAS PubMed
.
- R. Akiyama, N. Umemoto and M. Mizutani, Plant Biotechnol., 2023, 40, 185–191 CrossRef CAS PubMed
.
- M. Jesus, A. P. Martins, E. Gallardo and S. Silvestre, J. Anal. Methods Chem., 2016, 2016, 4156293 Search PubMed
.
- D. Yi, T. Bayer, C. P. S. Badenhorst, S. Wu, M. Doerr, M. Hohne and U. T. Bornscheuer, Chem. Soc. Rev., 2021, 50, 8003–8049 RSC
.
- E. Ricca, B. Brucher and J. H. Schrittwieser, Adv. Synth. Catal., 2011, 353, 2239–2262 CrossRef CAS
.
- Y. Zhou, S. K. Wu and U. T. Bornscheuer, Chem. Commun., 2021, 57, 10661–10674 RSC
.
- X. Yin, J. Liu, C. X. Kou, J. J. Lu, H. Zhang, W. Song, Y. H. Li, Z. Y. Xue and X. Hua, Metab. Eng., 2023, 76, 232–246 CrossRef CAS PubMed
.
- M. Liu and J. Q. Kong, Acta Pharm. Sin. B, 2018, 8, 981–994 CrossRef PubMed
.
- H. Pan, S. Chang, Y. Qu, M. Liu, W. Tian and Z. Chang, Biochem. Eng. J., 2023, 198, 109023 CrossRef CAS
.
- Q. Chen, Z. Chao, K. Wang, X. Wang, H. Meng, X. Liu, X. Shan and J. Zhou, ACS Catal., 2024, 14, 4117–4129 CrossRef CAS
.
- Y. J. Zhang, M. J. Liu, Z. X. Yang, J. Lin, Z. D. Huang and F. E. Chen, Green Chem., 2023, 25, 3223–3235 RSC
.
- Y. Q. Peng, C. H. Gao, Z. L. Zhang, S. J. Wu, J. Zhao and A. T. Li, ACS Catal., 2022, 12, 2907–2914 CrossRef CAS
.
- Z. L. Zhang, C. H. Gao, J. Zhao, X. G. Peng, Q. Li and A. T. Li, ACS Catal., 2023, 13, 13111–13116 CrossRef CAS
.
- J. Feng, Q. Wu, D. Zhu and Y. Ma, ChemSusChem, 2022, 15, e202102399 CrossRef CAS PubMed
.
- X. Li, R. Zhang, J. Li, N. Liu, X. Chen, Y. Liu, G. Zhao, K. Ding, P. Yao, J. Feng, Q. Wu, D. Zhu and Y. Ma, JACS Au, 2024 DOI:10.1021/jacsau.3c00688
.
|
This journal is © The Royal Society of Chemistry 2024 |