Gram-scale chemical synthesis of galactosyllactoses and their impact on infant gut microbiota in vitro†
Received
19th December 2023
, Accepted 14th February 2024
First published on 14th February 2024
Abstract
Galactooligosaccharides (GOS) are widely used as a supplement in infant nutrition to mimic the beneficial effects found in prebiotic human milk oligosaccharides (HMOs). However, the complexity of the GOS mixture makes it challenging to ascertain which of the GOS components contribute most to their health benefits. Galactosyllactoses (GLs) are lactose-based trisaccharides containing a β-galactopyranosyl residue at the 3′-position (3′galactosyllactose, 3′-GL), 4′-position (4′-galactosyllactose, 4′-GL), or the 6′-position (6′-galactosyllactose, 6′-GL). These GLs are of particular interest as they are present in both GOS mixtures and human milk at early stages of lactation. However, research on the potential health benefits of these individual GLs has been limited. Gram quantities are needed to assess their health benefits but these GLs are not readily available at this scale. In this study, we report the gram-scale chemical synthesis of 3′-GL, 4′-GL, and 6′-GL. All three galactosyllactoses were obtained on a gram scale in good purity from cheap and commercially available lactose. Furthermore, in vitro incubation of GLs with infant faecal microbiota demonstrates that the GLs were able to increase the abundance of Bifidobacterium and stimulate short chain fatty acid production.
Introduction
The establishment of a healthy gut microbiome in early life contributes to healthy immune-development, metabolism and brain development of the infant.1,2 As a major component in breastmilk, human milk oligosaccharides (HMOs) have been found to drive the development of a healthy gut microbiota by specifically stimulating the growth of beneficial gut bacteria, such as bifidobacteria.3 The oligosaccharide content in standard infant formula is typically low, as it is based on bovine milk, which has a much lower oligosaccharide content (∼0.05 g L−1) compared to human milk (∼20 g L−1).4,5 Therefore, in the last decades, it has become common practice to supplement infant formula products with functional oligosaccharides, such as β-galactooligosaccharides (GOS). GOS has been shown to promote the growth of benign micro-organisms such as bifidobacteria.6,7 Upon fermentation by the colonic microbiota, metabolites like short-chain fatty acids (SCFAs) are formed,7,8 which acidify the intestinal environment. This creates a favorable environment for bifidobacteria to grow and outcompete the growth of potentially harmful bacteria, such as Enterobacteriaceae or Clostridium perfringens.9 Next to the role of GOS in modulating the gut microbiome,10 GOS may also reduce the adhesion of pathogenic bacteria to the intestinal epithelium,11 improve mineral absorption,12,13 enhance natural defenses,14 and improve stool consistency and frequency.6,15,16 Commercially available GOS are produced enzymatically using a β-galactosidase enzyme, which normally hydrolyses lactose to form galactose and glucose. However, at high lactose concentrations, kinetically controlled transglycosylation takes place resulting in the intermolecular transfer of the cleaved galactose moiety to another lactose molecule to provide a complex mixture GOS.7,17,18 The most widely used GOS in infant formula, Vivinal® GOS, is a heterogeneous mixture of >100 linear and branched oligosaccharides.19,20 The complexity of the GOS mixture makes it challenging to establish which of the GOS components contribute most to the development of a healthy infant microbiome. Nevertheless, three galactosyllactoses (GLs) present in GOS, 3′-galactosyllactose (3′-GL), 4′-galactosyllactose (4′-GL), and 6′-galactosyllactose (6′-GL), are of particular interest (Fig. 1) as they are also found in human milk and can hence be classified as a human milk oligosaccharides (HMOs).21 GLs are found in higher concentrations in colostrum compared to mature milk and have been linked to anti-inflammatory properties in the intestine in vitro/ex vivo.21 To further dissect the role of specific GLs in shaping the microbiome, chemically pure samples are needed. Purification of GLs from GOS mixtures is highly challenging due to the micro-heterogeneous nature of these samples. Chemical GL synthesis can generate pure material, but needs to be scaled to produce the gram scale quantities needed for research into their potential health benefits22,23 Chemical procedures to prepare GLs at this scale are currently unavailable to the best of our knowledge.
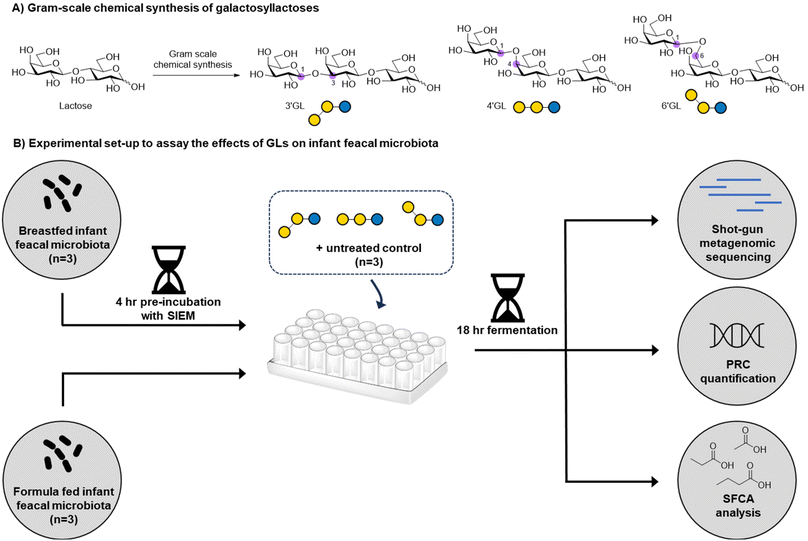 |
| Fig. 1 (A) The structure of the three galactosyllactose trisaccharides, and (B) work flow of their in vitro fermentation by infant faecal microbiota and impact on microbiota composition and metabolite production. | |
Herein, we report the development of a gram-scale chemical synthesis of 3′-GL, 4′-GL, and 6′-GL starting from readily available lactose. The general synthetic strategy for the GL trisaccharides 13–15 employs the synthesis of three different lactose-derived acceptors (4, 7 and 8), which are extended on the non-reducing end with either a β1,3-, β1,4-, or β1,6,-galactopyranosyl residue using galactosyl donor 9. Next, we investigated the impact of 3′-GL, 4′-GL and 6′-GL on infant gut microbiota in vitro by fermentation experiments using infant faecal samples. We found that GLs are important components in GOS as they clearly contribute to its effects on infant microbiota.
Results and discussion
Synthesis of 3′-, 4′- and 6′-GL
The synthesis of 3′-GL, 4′-GL, and 6′-GL started with the regioselective protection of lactose to afford the disaccharide acceptors (please see ESI S3–13† for experimental details). C-3′ hydroxyl lactose-derived acceptor 4 needed to construct 3′-GL was achieved in three steps from lactose (Scheme 1). First, lactose was protected with a 3′,4′-O-isopropylidene followed by benzylation of the remaining hydroxy groups with benzyl bromide and sodium hydride to obtain 2 in 27% overall yield.24,25 The low yield was attributed to the formation of multiple unidentified byproducts during the 3′,4′-O-isopropylidene protection. Potential byproducts include the kinetically favored 4′,6′-O-isopropylidene.25 Moreover, ring opening of the glucose residue can also result in the formation of additional byproducts.26 The 3′,4′-O-isopropylidene was cleaved by heating 2 in 80% aqueous acetic acid to afford the corresponding cis-diol 3 in 78% yield. A small sample of 3 was acetylated using Ac2O in pyridine to confirm the correct regioselectivity of this sequence by NMR. A downfield shift of the C-3′ and C-4′ protons was observed in the 1H-NMR compared to 3 confirming the correct regiochemistry (Fig. S11–13†).
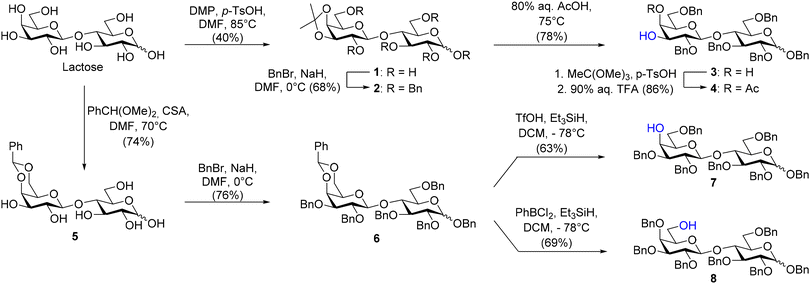 |
| Scheme 1 Gram scale synthesis of lactose acceptor building blocks (4, 7 and 8). | |
The synthesis of the disaccharide acceptors needed to prepare 4′-GL and 6′-GL was achieved from central benzylidene intermediate 5 (Scheme 1). A 4′,6′-O-benzylidene acetal was installed on lactose, followed by benzylation of the remaining hydroxy group using benzyl bromide and sodium hydride to afford 6 in a moderate yield of 56% over two steps.27 The 4′,6′-O-benzylidene was reductively opened to afford either the C-4′ hydroxyl or C-6′ hydroxyl derivate 7 and 8, respectively. The regioselectivity of the reductive ring opening depends on the (Lewis) acid used during the reaction. Triflic acid (TfOH) in combination with triethyl silane as the reducing agent provided the C-4′ hydroxyl 7 in 63% yield. In contrast, the use of dichlorophenylborane in combination with the same reducing agent provided the C-6′ hydroxyl 8 in 69% yield (Scheme 1).28 A small sample of the compound 7 and 8 was acetylated to determine the regioselectivity. As expected, a downfield shift of the C-4′ and C-6′ protons was observed in the 1H-NMR compared to 7 and 8, respectively (Fig. S31–33 and Fig. S45–47†).
With gram quantities of the disaccharides available, the synthesis of galactose-derived thioglycoside donor 9 was achieved in one step from the commercially available β-D-galactose pentaacetate using p-thiocresol and boron trifluoride etherate (BF3·OEt2).29 Regioselective glycosylation of acceptor 3 was attempted by treating cis-diol 3 with thiogalactoside donor 9 using the NIS/TfOH promotor system similar to the report of Craft and Townsend.30,31 Unfortunately, thin layer chromatography (TLC) analysis indicated the formation of numerous side products and it was therefore not possible to isolate the protected 3′-GL (16) (Scheme S1†) in good yield and purity. Since glycosylation of diol 3 was unsuccessful, another strategy was pursued. The cis-diol was subjected to a simple two-step, one-pot procedure to selectively protect the axial C-4′ hydroxyl with an acetyl ester.30 In the first step, 3 was protected with trimethylorthoacetate, followed by an orthoester rearrangement using 90% aqueous TFA to afford 4 in a yield of 86% (Scheme 2). With this acceptor (4), TfOH/NIS promoted glycosylation with glycosyl donor 9 resulted in the successful formation of 10 in a yield of 83% with full β-selectivity (Fig. S22†). Glycosylation of the C-4′ acceptor 7 was also achieved using donor 9via a TfOH/NIS promoted glycosylation resulting in the formation of 11 in a good yield of 74% with full β-selectivity (Fig. S38†). Finally, glycosylation of acceptor 8 with donor 9 under the same conditions afforded 12 in a moderate yield of 52% with full β-selectivity (Fig. S53†). This moderate yield is the result of byproduct formation during the glycosylation reaction. This byproduct was identified as the acetylated acceptor which is presumably formed by orthoester formation with the activated donor followed by rearrangement. Since acetylated acceptor (19) was already synthesized to prove the correct regioselectivity of 8 (vide supra), the 1H-NMR spectra of 19 and the byproduct obtained in the glycosylation of 6′-GL were compared (Fig. S48†). This indeed indicated that the obtained byproduct was the acetylated acceptor 19. All three molecules were deprotected in a two-step process. Deacetylation was achieved by treatment with potassium carbonate in methanol, followed by hydrogenolysis using Pd/C and hydrogen gas to afford 3′-GL (13), 4′-GL (14) and 6′-GL (15) in 75%, 83% and 76% over two steps, respectively (Scheme 2). Overall, 1.42 g of 3′-GL (11% yield, seven steps, 95.4% purity), 2.18 g of 4′-GL (22% yield, six steps, 95.0% purity) and 2.25 g of 6′-GL (15% yield, six steps, 98.1% purity) were obtained. The GLs were subsequently used to evaluate their effect on infant microbiota composition by performing in vitro fermentations in infant faecal microbiota.
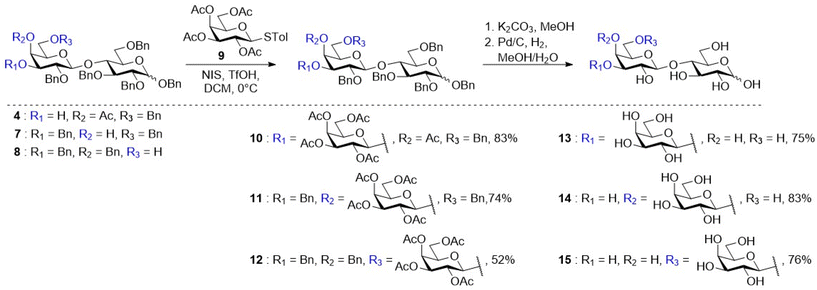 |
| Scheme 2 The glycosylation and deprotection scheme used for the synthesis of 3′-, 4′-, and 6′-GL. | |
The effects of 3′-GL, 4′-GL and 6′-GL on infant faecal microbiota in vitro
To determine the effect of the galactosyllactoses on healthy human infant intestinal microbiota, the TNO i-screen model was used.32 To this end, the faecal microbiota from formula- or breastfed infants were incubated with pure samples of 3′-GL, 4′-GL and 6′-GL (18 h) and compared to an untreated control. At the end of the experiment, the effects of the GLs on the composition of the microbiota was established (see ESI, S15 and 16† for details). An overview of the impact of the different GLs on the top 25 most abundant microbiota taxa can be found in Fig. S1.† Overall, the GLs appear to enhance the abundance of Bifidobacterium species and reduce Bacteroides. DESeq analysis of the data shows that each GL stimulates a certain pattern of bifidobacterial species, with 4′-GL stimulating the most broad pattern of Bifidobacterium (Fig. 2 and 3). In the formula-fed infant microbiota, 4′-GL was most bifidogenic; enhancing levels of B. longum, B. breve, B. pseudocatenulatum and B. kashiwanohense. 6′-GL increased the abundance of B. longum and B. breve. 3′-GL was the only GL significantly stimulating the growth of B. bifidum, in addition to increasing B. longum and B. breve. 4′-GL showed the highest overall bifidogenic potential, followed by 6′-GL and 3′-GL. All GLs significantly reduced Clostridium butyricum levels and selectively decreased certain members of the genus Bacteroides (Fig. 2).
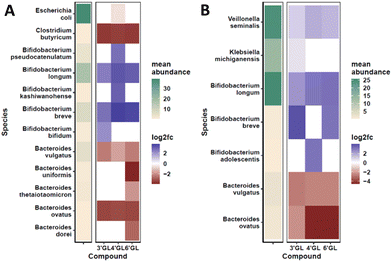 |
| Fig. 2 Visual representation of DESeq2 analysis on the effects of 3′-GL, 4′-GL and 6′-GL on the composition of the infant microbiota (panel A: formula fed infants, panel B: breastfed infants). The microbial taxa represented here are the ones that significantly changed in abundance after exposure to the selected compounds compared to the untreated control. | |
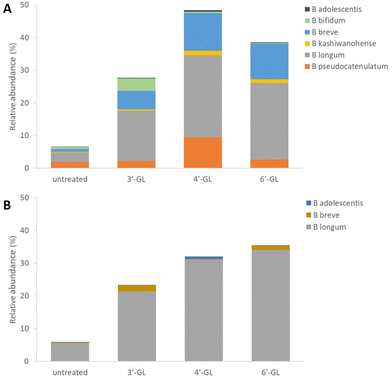 |
| Fig. 3 Bar graphs showing the relative abundances of the different Bifidobacterium species in infant microbiota after exposure to 3′-GL, 4′-GL or 6′-GL (panel A: formula fed infants, panel B: breastfed infants). | |
In the breastfed infant microbiota, 6′-GL and 4′-GL were slightly more bifidogenic compared to 3′-GL. All GLs clearly stimulated the growth of B. longum. 3′-GL and 6′-GL enhanced B. breve, whereas 4′-GL showed a minor increase in B. adolescentis. Similar to the results for the formula-fed infant microbiota, the GLs decreased the abundance of certain members of the genus Bacteroides (Fig. 2 and 3).
A bifidogenic effect for some of the GLs has been described before. Li et al. found that 3′-GL and 6′-GL purified from GOS could enhance Bifidobacterium growth in vitro in adult faecal microbiota samples.33 In that study, microbiota changes were identified at genus level, using fluorescent in situ hybridization (FISH). With that technique, effects at bacterial strain level could not be detected. To our knowledge, our study is the first to investigate the impact of all individual galactosyllactoses on infant microbiota at bacterial strain level.
To characterize the B. longum species into even more detail, qPCR analyses were performed quantifying the levels of its subspecies B. longum subsp. longum and B. longum subsp. infantis in the microbiota samples. The different GLs all stimulated B. longum subsp. longum and B. longum subsp. infantis to a similar extent in the formula-fed infant microbiota (the relative abundances of both subspecies had roughly a 1
:
1 ratio). In the breastfed infant microbiota, B. longum subsp. longum was enhanced by the GLs slightly more than B. longum subsp. infantis (data not shown).
The slight differences we found in bifidogenicity of the GLs between microbiota from infants that were previously formula-fed or breastfed, could in part also be driven by the nutrition received by the infants. In human milk, the levels of 6′-GL are higher early in lactation as compared to 3′-GL and 4′-GL.34,35 On the other hand, infant formula in The Netherlands is often supplemented with galacto-oligosaccharides (GOS). GOS are a complex mixture of oligosaccharides also containing GLs,19 with 4′-GL being the most abundant.36 Thus, the breastfed infant microbiota has seen more 6′-GL as compared to the other GLs and the formula-fed microbiota has seen relatively more 4′-GL. In response, the microbiota may have adapted its composition and/or metabolism to efficiently ferment these respective GLs.
Upon fermentation of substrates, the microbiota can produce different metabolites, such as short-chain fatty acids (SCFA). Fig. 4 shows the levels of SCFA produced by the infant microbiota during fermentation of the GLs. All GLs increased the total concentration of SCFA in the supernatant of the microbiota fermentations, with 4′-GL and 6′-GL showing a significant increase as compared to the control (Fig. 4). This increase was mainly driven by higher levels of acetic acid produced upon fermentation of the GLs (Fig. 4). This is consistent with other studies reporting that galacto-oligosaccharides can enhance acetic acid production by microbiota in vitro,37 as well as in clinical trials in infants38 and adults.39 The increased levels of acetic acid found upon fermentation of the GLs probably reflects their enhancement of Bifidobacterium species growth, as these are known producers of acetic acid.40 SCFA have been found to play a role in intestinal development (serving as energy source for intestinal cells), but also to influence immune function, energy metabolism and gut-brain communication.41 Acetic acid production in particular has been shown to be important for cross-feeding between Bifidobacterium and butyrate-producing bacteria; and potentially also for reducing overproduction of gas in the infant intestine.40 Thus, SCFA are an important factor in infant gut- and overall health.
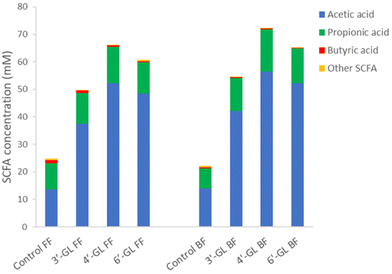 |
| Fig. 4 SCFA concentrations in the supernatant of infant microbiota fermentations. “Other SCFA” include lactic acid, succinic acid, isobutyric acid, 2-methylbutanoic acid, isovaleric acid and valeric acid. FF = formula fed infant microbiota, BF = breastfed infant microbiota. | |
Overall, our results show a clear bifidogenic effect of all the GLs, with 4′-GL and 6′-GL having a slightly stronger effect as compared to 3′-GL. This indicates that the GLs play an important role in the microbiota-shaping effect of GOS. Future development of GOS ingredients which are further enriched in GL-content may hold potential for enhanced bifidogenicity.
Conclusion
A chemical synthetic approach was developed for the gram-scale chemical synthesis of 3′-, 4′- and 6′-GL. These GLs were obtained in moderate to good overall yields and with very high purity. The effects of these compounds on the composition of infant microbiota was investigated. These GLs were found to enhance Bifidobacterium abundance in infant faecal microbiota as tested by in vitro fermentation and supported SCFA production. We expect that the gram-scale availability of GLs enables further investigation of their functionality in other, potentially more physiologically relevant, in vitro model systems and/or in in vivo studies.
Conflicts of interest
There are no conflicts to declare.
Acknowledgements
This work was supported by an NWO-LIFT grant (ENPPS.LIFT.019.015) awarded to T. J. B.
References
- G. R. Gibson and M. B. Roberfroid, Dietary Modulation of the human colonic microbiota: introducing the concept of prebiotics, J. Nutr., 1995, 125(6), 1401–1412 CrossRef CAS PubMed.
- P. A. M. J. Scholtens,
et al., The Early Settlers: Intestinal Microbiology in Early Life, Annu. Rev. Food Sci. Technol., 2012, 3(1), 425–447 CrossRef CAS PubMed.
- L. Bode and E. Jantscher-Krenn, Structure-Function Relationships of Human Milk Oligosaccharides, Adv. Nutr., 2012, 3(3), 383S–391S CrossRef CAS PubMed.
- A. Martinez-Ferez, A. Guadix and E. M. Guadix, Recovery of caprine milk oligosaccharides with ceramic membranes, J. Membr. Sci., 2006, 276(1), 23–30 CrossRef CAS.
- L. Bode, Human milk oligosaccharides: Every baby needs a sugar mama, Glycobiology, 2012, 22(9), 1147–1162 CrossRef CAS PubMed.
- X.-M. Ben,
et al., Supplementation of milk formula with galacto-oligosaccharides improves intestinal micro-flora and fermentation in term infants, Chin. Med. J., 2004, 117(06), 927–931 CAS.
- B. P. Lamsal, Production, health aspects and potential food uses of dairy prebiotic galactooligosaccharides, J. Sci. Food Agric., 2012, 92(10), 2020–2028 CrossRef CAS PubMed.
- M. B. Engfer,
et al., Human milk oligosaccharides are resistant to enzymatic hydrolysis in the upper gastrointestinal tract123, Am. J. Clin. Nutr., 2000, 71(6), 1589–1596 CrossRef CAS PubMed.
- D. J. M. Barnett,
et al., Human milk oligosaccharides, antimicrobial drugs, and the gut microbiota of term neonates: observations from the KOALA birth cohort study, Gut Microbes, 2023, 15(1), 2164152 CrossRef CAS PubMed.
- S. Fanaro,
et al., Galacto-oligosaccharides are bifidogenic and safe at weaning: a double-blind randomized multicenter study, J. Pediatr. Gastroenterol. Nutr., 2009, 48(1), 82–88 CrossRef CAS PubMed.
- V. Ambrogi,
et al., Bifidobacterial β-Galactosidase-Mediated Production of Galacto-Oligosaccharides: Structural and Preliminary Functional Assessments, Front. Microbiol., 2021, 12, 750635 CrossRef PubMed.
- C. M. Weaver,
et al., Galactooligosaccharides improve mineral absorption and bone properties in growing rats through gut fermentation, J. Agric. Food Chem., 2011, 59(12), 6501–6510 CrossRef CAS PubMed.
- D. Paganini,
et al., Consumption of galacto-oligosaccharides increases iron absorption from a micronutrient powder containing ferrous fumarate and sodium iron EDTA: a stable-isotope study in Kenyan infants, Am. J. Clin. Nutr., 2017, 106(4), 1020–1031 CrossRef CAS PubMed.
- S. Arslanoglu, G. E. Moro and G. Boehm, Early supplementation of prebiotic oligosaccharides protects formula-fed infants against infections during the first 6 months of life, J. Nutr., 2007, 137(11), 2420–2424 CrossRef CAS PubMed.
- X. M. Ben,
et al., Low level of galacto-oligosaccharide in infant formula stimulates growth of intestinal Bifidobacteria and Lactobacilli, World J. Gastroenterol., 2008, 14(42), 6564–6568 CrossRef PubMed.
- T. Williams,
et al., Tolerance of formulas containing prebiotics in healthy, term infants, J. Pediatr. Gastroenterol. Nutr., 2014, 59(5), 653–658 CrossRef CAS PubMed.
-
D. Charalampopoulos and R. A. Rastall, Prebiotics and probiotics science and technology, Springer Science & Business Media, 2009, vol. 1 Search PubMed.
- C. Vera,
et al., Synthesis and purification of galacto-oligosaccharides: state of the art, World J. Microbiol. Biotechnol., 2016, 32(12), 197 CrossRef PubMed.
- S. S. van Leeuwen,
et al., 1H NMR analysis of the lactose/β-galactosidase-derived galacto-oligosaccharide components of Vivinal® GOS up to DP5, Carbohydr. Res., 2014, 400, 59–73 CrossRef CAS PubMed.
- M. J. Logtenberg,
et al., Touching the High Complexity of Prebiotic Vivinal Galacto-oligosaccharides Using Porous Graphitic Carbon Ultra-High-Performance Liquid Chromatography Coupled to Mass Spectrometry, J. Agric. Food Chem., 2020, 68(29), 7800–7808 CrossRef CAS PubMed.
- Y. He,
et al., Human colostrum oligosaccharides modulate major immunologic pathways of immature human intestine, Mucosal Immunol., 2014, 7(6), 1326–1339 CrossRef CAS PubMed.
- D. Beith-Halahmi, H. M. Flowers and D. Shapiro, Synthesis of O-β-d-galactopyranosyl-(1→3)-O-β-d-galactopyranosyl-(1→4)-d-glucose, Carbohydr. Res., 1967, 5(1), 25–30 CrossRef CAS.
- V. Perrin,
et al., Identification and synthesis of a trisaccharide produced from lactose by transgalactosylation, Carbohydr. Res., 2000, 325(3), 202–210 CrossRef CAS PubMed.
- D. B. Sarney,
et al., Chemo-enzymatic synthesis of disaccharide fatty acid esters, J. Am. Oil Chem. Soc., 1994, 71(7), 711–714 CrossRef CAS.
- H. H. Baer and S. A. Abbas, Synthesis of O-α l-fucopyranosyl-(1→3)-O-β-d-galactopyranosyl-(1→4)-d-glucose (3′-O-α-l-fucopyranosyllactose), and an Improved Route to its β-(1′′→3′)-linked Isomer, Carbohydr. Res., 1980, 84(1), 53–60 CrossRef CAS.
- K. Agoston,
et al., Kilogram scale chemical synthesis of 2′-fucosyllactose, Carbohydr. Res., 2019, 476, 71–77 CrossRef CAS PubMed.
- D. Qiu and R. R. Schmidt, Glycosyl Imidates, 52. Synthesis of Globotriaosylceramide (Gb3) and Isoglobotriaosylceramide (isoGb3), Liebigs Ann. Chem., 1992, 1992(3), 217–224 CrossRef.
- M. Sakagami and H. Hamana, A selective ring opening reaction of 4,6-O-benzylidene acetals in carbohydrates using trialkylsilane derivatives, Tetrahedron Lett., 2000, 41(29), 5547–5551 CrossRef CAS.
- C.-H. Wu,
et al., Simple and Practical Real-Time Analysis of Solid-Phase Reactions by Thin-Layer Chromatography, Synlett, 2018,(11), 1430–1436 CAS.
- K. M. Craft and S. D. Townsend, Synthesis of lacto-N-tetraose, Carbohydr. Res., 2017, 440–441, 43–50 CrossRef CAS PubMed.
- G. H. Veeneman, S. H. van Leeuwen and J. H. van Boom, Iodonium ion promoted reactions at the anomeric centre. II An efficient thioglycoside mediated approach toward the formation of 1,2-trans linked glycosides and glycosidic esters, Tetrahedron Lett., 1990, 31(9), 1331–1334 CrossRef CAS.
- F. Schuren,
et al., The i-screen: A Versatile Preclinical Platform for Gut Microbiota Studies, J. Probiotics Health, 2019, 7, 7–10 Search PubMed.
- W. Li,
et al., Influences of structures of galactooligosaccharides and fructooligosaccharides on the fermentation in vitro by human intestinal microbiota, J. Funct. Foods, 2015, 13, 158–168 CrossRef CAS.
- W. Sumiyoshi,
et al., Galactosyllactoses in the Milk of Japanese Women: Changes in Concentration during the Course of Lactation, J. Appl. Glycosci., 2004, 51(4), 341–344 CrossRef CAS.
- D. S. Newburg and Y. He, Neonatal Gut Microbiota and Human Milk Glycans Cooperate to Attenuate Infection and Inflammation, Clin. Obstet. Gynecol., 2015, 58(4), 814–826 CrossRef PubMed.
- L. Coulier,
et al., In-depth characterization of prebiotic galacto-oligosaccharides by a combination of analytical techniques, J. Agric. Food Chem., 2009, 57(18), 8488–8495 CrossRef CAS PubMed.
- J. Stiverson,
et al., Prebiotic Oligosaccharides: Comparative Evaluation Using In Vitro Cultures of Infants’ Fecal Microbiomes, Appl. Environ. Microbiol., 2014, 80(23), 7388–7397 CrossRef CAS PubMed.
- X.-M. Ben,
et al., Low level of galacto-oligosaccharide in infant formula stimulates growth of intestinal Bifidobacteria and Lactobacilli, World J. Gastroenterol., 2008, 14(42), 6564 CrossRef PubMed.
- W. Van Dokkum,
et al., Effect of nondigestible oligosaccharides on large-bowel functions, blood lipid concentrations and glucose absorption in young healthy male subjects, Eur. J. Clin. Nutr., 1999, 53(1), 1–7 CrossRef CAS PubMed.
- G. A. Stuivenberg,
et al., Why are bifidobacteria important for infants?, Microorganisms, 2022, 10(2), 278 CrossRef PubMed.
- E. Blaak,
et al., Short chain fatty acids in human gut and metabolic health, Benefic. Microbes, 2020, 11(5), 411–455 CrossRef CAS PubMed.
|
This journal is © The Royal Society of Chemistry 2024 |
Click here to see how this site uses Cookies. View our privacy policy here.