DOI:
10.1039/D3NR06264C
(Review Article)
Nanoscale, 2024,
16, 8236-8255
Cell membrane coated nanoparticles: cutting-edge drug delivery systems for osteoporosis therapy
Received
8th December 2023
, Accepted 21st March 2024
First published on 1st April 2024
Abstract
Osteoporosis, characterized by a reduction in bone mineral density, represents a prevalent skeletal disorder with substantial global health implications. Conventional therapeutic strategies, exemplified by bisphosphonates and hormone replacement regimens, though effective, encounter inherent limitations and challenges. Recent years have witnessed the surge of cell-membrane-coated nanoparticles (CMNPs) as a promising intervention for osteoporosis, leveraging their distinct attributes including refined biocompatibility, heightened pharmaceutical payload capacity, as well as targeted drug release kinetics. However, a comprehensive review consolidating the application of CMNPs-based therapy for osteoporosis remains absent within the existing literature. In this review, we provide a concise overview of the distinctive pathogenesis associated with osteoporosis, alongside an in-depth exploration of the physicochemical attributes intrinsic to CMNPs derived from varied cellular sources. Subsequently, we explore the potential utility of CMNPs, elucidating emerging trends in their deployment for osteoporosis treatment through multifaceted therapeutic approaches. By linking the notable attributes of CMNPs with their roles in mitigating osteoporosis, this review serves as a catalyst for further advances in the design of advanced CMNPs tailored for osteoporosis management. Ultimately, such progress is promising for enhancing outcomes in anti-bone loss interventions, paving the way for clinical translation in the near future.
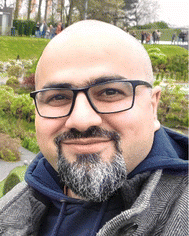 Mohammad-Ali Shahbazi | Dr Shahbazi received his Ph.D. in 2015 from the University of Helsinki, Finland. He is currently an assistant professor at the University Medical Center Groningen. He has over 180 publications and several competitive prizes in his portfolio, including the best Ph.D. thesis in pharmaceutical Sciences in Finland in 2015 and the highly prestigious USERN laureate award in 2020. His current research interest lies in photoactive hydrogels and cell-mimicking nanomedicines for tissue regeneration and cancer immunotherapy to shape the future of multifunctional therapeutics through the combination of material science, biology, and nanotechnology. |
1. Introduction
Osteoporosis, a chronic skeletal disorder, is characterized by diminished bone mass, degradation of bone tissue, and an elevated vulnerability to fractures.1,2 While it affects both genders, its prevalence is more pronounced in women, particularly postmenopausal ones.3 Studies indicate that osteoporosis afflicts approximately 23% of women and 7% of men aged 50 and above.4 Referred to as a “silent disease”, its insidious progression often remains asymptomatic until fractures manifest, primarily within the spine, hip, and wrist. Such fractures can lead to pain, disability, compromised independence, and a diminished quality of life. In severe instances, fracture complications may even prove life-threatening, notably among the elderly.5
Conventional anti-osteoporosis treatments, encompassing bisphosphonates, selective estrogen receptor modulators (SERMs), and calcitonin, might not confer universal efficacy. While these agents can decelerate bone loss or augment bone density, they may not comprehensively reinstate bone health or forestall fractures in all patients.6,7 Moreover, their effectiveness could wane over prolonged use. These traditional medications can also precipitate adverse effects. For instance, bisphosphonates may induce gastrointestinal distress, including nausea and abdominal discomfort, and could lead to rare yet grave outcomes like osteonecrosis of the jaw and atypical fractures.8 SERMs might elevate the risk of thrombotic events and other cardiovascular complications.9 Calcitonin administration may induce nasal irritation and flushing.10 These untoward effects impede the tolerability and enduring usage of these therapeutic agents.
Fortunately, nanotechnology presents inherent advantages such as proficient drug delivery and controlled release mechanisms that hold the potential to effectively address the aforementioned limitations.11–14 Moreover, a particularly promising development emerges: the encapsulation of nanoparticles within cellular membranes has witnessed significant progress, imparting the nanoparticles with an inherent capability for active targeting. This innovation facilitates the accurate and targeted delivery of encapsulated therapeutic agents, particularly in the context of tissue remodeling and regeneration (Fig. 1).15–27 In recent decades, pioneering approaches revolving around the utilization of cell-membrane-coated nanoparticles (CMNPs) have rapidly emerged, aiming to enhance the bone microenvironment and thereby improving the outcome of anti-osteoprotic therapy. These specialized nanoparticles can be functionally engineered to selectively engage specific cells or tissues, including bone tissue, facilitating the controlled release of therapeutic payloads with exquisite accuracy. Notably, CMNPs exhibit remarkable versatility in accommodating diverse therapeutic agents, thereby amplifying their potential in bone regeneration and repair. Importantly, the capacity of CMNPs to precisely target bone cells holds the promise of mitigating the systemic side effects typically associated with conventional osteoporosis medications. This precision would enable the effective and efficient delivery of therapeutic agents to the intended site of action. Despite the comprehensive investigations conducted into CMNPs, it is noteworthy that the utilization of these specialized nanoparticles in the context of osteoporosis treatment remains relatively underexplored, according to our current understanding.
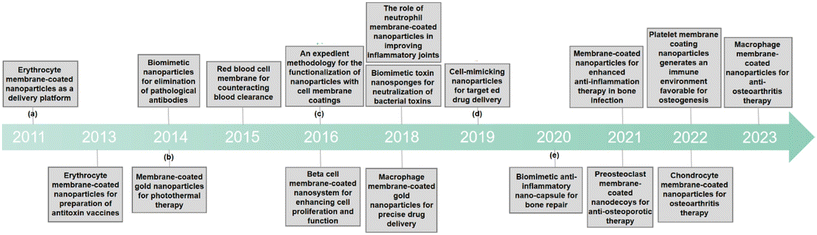 |
| Fig. 1 A chronological representation of the pivotal developmental milestones of CMNPs in biomedicine. (a) The role of polymeric nanoparticles camouflaged with erythrocyte membranes in acting as a biomimetic delivery platform.15 (b) Erythrocyte membrane as an alternative coating to polyethylene glycol for extending the circulation lifespan of gold Nanocages in photothermal therapy.18 (c) Introduction of an easy method to add functionality to nanoparticles coated with cell membranes.20 (d) Precise assembly of cell-mimicking nanoparticles through molecular affinity strategy for targeted drug delivery.25 (e) The role of biomimetic nano-capsule in functioning as a cytokine blocker and inducer of M2 polarization for bone regeneration.26 | |
Herein, we summarize the emerging advances of CMNPs in osteoporosis treatment, including their fabrication methods, physicochemical properties, and therapeutic applications. Simultaneously, we explore the potential utility of CMNPs, elucidating emerging trends in their deployment for osteoporosis treatment through multifaceted therapeutic approaches. Finally, advanced anti-osteoporosis therapies with their potential for clinical translation and emerging CMNPs-based strategies for osteoporosis treatment are discussed.
2. Characteristic pathogenesis of osteoporosis
Osteoporosis, a prevalent skeletal disorder of significant clinical import, is hallmarked by a notable reduction in bone mass and concomitant degradation of microarchitectural integrity. This intricate cascade of bone degeneration culminates in an augmented vulnerability to fractures, an outcome that underscores the gravity of this condition within the realm of musculoskeletal health.28
The central hallmark of osteoporosis lies in the loss of bone mass, which results from an intricate interplay between enhanced bone resorption and compromised bone formation. These processes, normally regulated in a balanced bone remodeling cycle, become dysregulated in osteoporosis, precipitating the characteristic pathological changes that set the stage for increased fracture susceptibility.29,30 At the cellular level, the pivotal players in bone remodeling, osteoclasts, and osteoblasts, assume central roles in driving osteoporotic changes. Enhanced osteoclast activity, triggered by an altered balance of signaling molecules, leads to the breakdown of bone tissue, contributing to the decline in bone mass. Simultaneously, compromised osteoblast function, driven by intricate molecular perturbations, impedes the synthesis of new bone, thereby exacerbating the imbalance between bone resorption and formation (Fig. 2).31,32
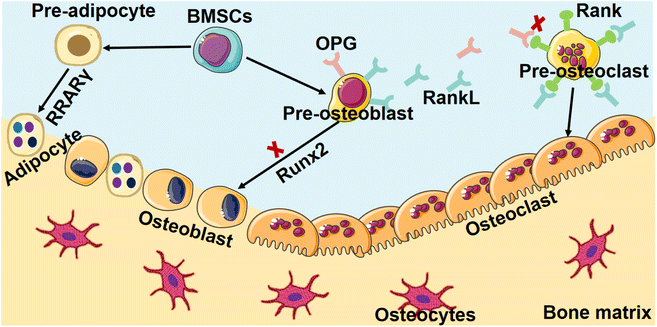 |
| Fig. 2 Dynamic interplay between heightened bone resorption and impaired bone formation in osteoporosis. The roles of osteoclasts and osteoblasts stand pivotal, steering the course of osteoporotic alterations through their synchronized activities. Osteoclasts emerge as protagonists of heightened activity—a manifestation resulting from the intricate disruption of signaling molecule equilibrium. This dysregulated balance serves as the catalyst, propelling osteoclasts into a state of heightened bone resorption. Simultaneously, the functional integrity of osteoblasts, the architects of bone synthesis, experiences compromise. These intricate disruptions intricately converge, obstructing the synthesis of new bone material and further amplifying the prevailing imbalance, initiated by escalated bone resorption. Created with Servier Medical Art (https://smart.servier.com/). | |
2.1 Increased bone resorption
The intricate physiological balance governing bone homeostasis is profoundly disrupted in osteoporosis, resulting in a profound escalation of bone resorption processes. This aberrant phenomenon is mainly attributed to the hyperactivity of osteoclasts, the specialized cellular entities responsible for the degradation of bone tissue.33 The augmented efficacy of these osteoclasts is rooted in the perturbed equilibrium between bone resorption and formation, a fundamental aspect of the bone remodeling cycle.34 The unbridled proliferation of osteoclast populations precipitates an accelerated pace of bone tissue breakdown, thereby engendering a distressing compromise to the fundamental structural integrity of bone.35
The core of this intricate orchestration of osteoclast activity is the dynamic interplay between two pivotal molecular players: the receptor activator of nuclear factor kappa-B ligand (RANKL) and its regulatory counterpart, osteoprotegerin (OPG).36 These factors intricately regulate the differentiation and functioning of osteoclasts, thereby wielding profound influence over the course of bone remodeling.37 However, in the context of osteoporosis, this finely tuned ballet is disrupted, leading to dire consequences for bone health.
A pioneering investigation into the molecular underpinnings of osteoporotic bone resorption reveals the emergence of a complex interplay involving inflammatory cytokines and RANKL. The pathogenesis of osteoporosis hinges on the convergence of a network of inflammatory signaling cascades with the RANKL signaling pathway. At the epicenter of this interplay are pro-inflammatory cytokines, notably interleukin-6 (IL-6) and tumor necrosis factor-alpha (TNF-α), which exhibit a synergistic relationship with RANKL.38,39 These cytokines, in their heightened levels characteristic of osteoporosis, exert a profound stimulatory effect on osteoclastogenesis. This augmented cytokine milieu instigates an upsurge in the expression of RANKL, thereby potentiating the signaling cascade that drives osteoclast differentiation and activity.
The state of RANKL-mediated osteoclastogenesis assumes a pathological disposition, marked by the disturbance of the synchronized molecular cues that typically govern this intricate biological process.37 This disruption of molecular signals culminates in a cascade of heightened osteoclast activity, setting the stage for accelerated bone resorption. The consequence of this augmented bone resorption is manifest in the clinical hallmarks of osteoporosis – diminished bone density and compromised structural integrity.
2.2 Decreased bone formation
In consonance with the pronounced elevation of bone resorption that typifies the osteoporotic context, a feature of significant distinction emerges within its pathogenesis—a marked attenuation in the intricate process of bone formation.40 This deficiency in bone generation arises at its core from an intricate compromise in the functionality of osteoblasts, the key cellular regulators responsible for the elaborate orchestration of bone matrix synthesis and mineralization. The interplay of numerous factors, including hormonal imbalances and genetic predisposition, impairs the proliferation and differentiation of osteoblasts. Consequently, the insufficient deposition of new bone material undermines bone strength and structure.41
In the context of bone research, the Wnt signaling pathway emerges as a crucial regulator, well-documented for its pivotal role in orchestrating bone metastasis and being a central component of molecular coordination.39 This signaling involves in mediating the complex processes of osteoblastogenesis—the differentiation of precursor cells into mature bone-forming cells—and the intricate network that governs bone formation, a foundation of skeletal integrity.40 The canonical arm of the Wnt pathway, renowned for its indispensability in shaping the course of osteoblast differentiation, the facilitation of mineralization processes, and the holistic orchestration of osteoblast function, becomes vulnerable to the influence of an array of multifaceted factors. Within the intricate milieu of osteoporosis, this pathway succumbs to alterations that intricately cause an outcome of diminished osteoblast activity. The canonical Wnt signaling axis, which bears paramount importance for sustaining osteoblast differentiation, the process of mineralization crucial for bone strength, and the overall functional capacity of osteoblasts, confronts a compromised state.41 This compromise is primarily a result of alterations in various factors, notably including mutations in the low-density lipoprotein receptor-related protein 5 (LRP5), a critical entity that intricately interfaces with canonical Wnt signaling.42
The implications of this impeded canonical Wnt signaling affect profoundly within the process of osteoblastogenesis. The proliferation and differentiation of osteoblasts become hindered, thereby leading to an impairment in bone-forming activities. This, in turn, contributes to the exacerbation of the inherent imbalance between the processes of bone resorption and bone formation—a hallmark characteristic of osteoporosis.43 Heightened bone resorption, facilitated by the escalated activity of osteoclasts driven by inflammatory cytokines and RANKL, coincides with diminished bone formation attributable to compromised osteoblast function and the intricate disruption of Wnt signaling. These intricate interplays coalesce to form the complex pathophysiology that defines osteoporosis, a multifaceted ailment that unravels the intricate skeletally dependent equilibrium.
3. Characteristics of CMNPs for osteoporosis
CMNPs represent a novel class of nanoparticles that feature a delicately layered cell membrane derived from select cell types, notably stem cells. This distinctive membrane coating endows CMNPs with tailored properties and functionalities, thus rendering them a highly promising approach in diverse biomedical applications. The compelling advantages of CMNPs reside in their unique attributes encompassing biocompatibility, precision-targeted delivery, controlled release of therapeutic agents, heightened stability, efficient cellular internalization, and multifaceted versatility. These attributes collectively amplify CMNPs’ potential for a wide spectrum of biomedical applications, notably including their application in the complex realm of osteoporosis treatment.
3.1 Biocompatibility and reduced immune response
CMNPs have garnered substantial attention due to their unique capacity to bridge the gap between synthetic nanoparticles and the complex milieu of living organisms. A defining attribute of CMNPs is their inherent biocompatibility and the consequent reduction in immune response, rendering them particularly intriguing for diverse biomedical applications.44 Biocompatibility stands as a foundational tenet in the realm of biomedical nanotechnology. It encapsulates the fundamental requirement that engineered materials and nanoparticles should integrate into biological systems without eliciting adverse effects. Various strategies have been used to enhance the biocompatibilty of CMNPs. Surface modification techniques like PEGylation can prolong circulation time and minimize immune recognition.45 Incorporating biomimetic peptides or proteins onto CMNPs surfaces can also improve their interaction with biological systems.46 Additionally, developing novel coatings or functionalization methods that facilitate specific cell targeting while minimizing off-target effects holds promise for improving the biocompatibility of CMNPs in biomedical applications.47
3.2 Targeted delivery and enhanced tissue specificity
Traditional drug delivery strategies often suffer from limitations associated with non-specific biodistribution, resulting in off-target effects and diminished therapeutic efficacy.48 CMNPs, by virtue of their unique cell membrane coating, present an innovative solution to this challenge. The cell membrane, replete with an array of surface receptors and proteins, plays a pivotal role in cell recognition and binding. When incorporated into CMNPs, this cell membrane coating confers the ability to selectively bind to specific cell types or tissues.49
This targeted binding capability offers transformative potential in therapeutic delivery.50 By functionalizing CMNPs with ligands or antibodies that recognize cell-specific markers, researchers can orchestrate a homing effect, directing CMNPs precisely to the intended site of action. This precision minimizes systemic exposure, reduces potential side effects, and maximizes the therapeutic payload's impact, thus advancing the concept of personalized medicine.
Furthermore, with their cell membrane coating, CMNPs inherently possess a biological affinity that aligns with the targeted tissue's characteristics. This affinity is a consequence of the biomimetic nature of the cell membrane, which mirrors the interactions between cells and tissues within the body.51 As a result, CMNPs exhibit heightened tissue specificity, enhancing their ability to selectively accumulate in the desired anatomical locations.
For instance, in the context of osteoporosis treatment, CMNPs can be engineered to mimic the cell membrane of osteoblasts, the bone-forming cells.52 This affinity allows CMNPs to preferentially accumulate within bone tissue, bypassing other organs and tissues. The enhanced tissue specificity translates to an augmented therapeutic effect precisely at the site of bone degeneration, minimizing systemic exposure and conferring therapeutic benefits with reduced adverse events.
3.3 Controlled drug release for optimized therapeutic efficacy
Controlled drug release is a critical aspect of modern medical treatment, and in this context, CMNPs stand out due to their distinctive structural characteristics. These nanoparticles hold a unique advantage in controlled drug delivery, primarily owing to their versatile structure. The incorporation of a cell membrane coating onto the nanoparticle surface provides a sophisticated platform for the integration of responsive elements, which in turn enables the precise modulation of drug release kinetics.53
The concept of responsive drug release revolves around the ability of CMNPs to be engineered in a way that they respond to specific triggers or stimuli present in their surrounding environment.54 These triggers can encompass a range of factors, including variations in pH, temperature, enzyme activity, or the presence of particular molecules unique to the target area.55 This responsiveness is delicately tailored to ensure that the release of therapeutic agents occurs in a controlled and predetermined manner, in direct response to the cues encountered in the local environment.56
In the context of osteoporosis treatment, CMNPs can be precisely designed to release bone-strengthening agents solely in response to specific biochemical cues indicative of ongoing bone degradation.52 This strategic approach guarantees that the therapeutic payload is released exclusively at the site requiring intervention, thereby fostering bone regeneration.57 Importantly, this localized release strategy minimizes systemic exposure to the therapeutic agents, thus mitigating the potential for systemic side effects.
The key to achieving this level of precision lies in the engineering of CMNPs to intricately interact with the cues specific to the target tissue or disease environment. By intelligently incorporating these responsive elements, researchers are empowered to design CMNPs to dispense therapeutic agents at a rate that harmonizes optimally with the pace of disease progression or the physiological demands of the targeted tissue. This orchestration of drug release profiles is a significant advance markedly enhancing therapeutic efficacy.
3.4 Enhanced stability and prolonged circulation time
The lipid bilayer constituting the cellular membrane furnishes a protective barrier enveloping the synthetic nanoparticle nucleus. This barrier serves to avert direct interaction between the nanoparticle nucleus and the ambient milieu, thereby diminishing the propensity for degradation consequent to enzymatic or chemical processes.58 Additionally, the lipid bilayer functions as an impediment, forestalling the aggregation or agglomeration of nanoparticles, thereby further amplifying their stability within assorted biological fluids.59
A conspicuous challenge characterizing conventional nanoparticles pertains to their prompt clearance from the bloodstream by the immune system's mononuclear phagocyte system (MPS).60 This expeditious elimination curtails their period of circulation and, consequently, their efficacy in accessing targeted sites. CMNPs redress this challenge by manifesting inherent “self” attributes that deter internalization by MPS cells.61 Furthermore, the presence of surface markers derived from the cellular membrane, notably CD47 (a signal connoting “non-phagocytosis”), on the encapsulated nanoparticles, obstructs the process of identification and engulfment by macrophages within the MPS.62 This property engenders an elongated span of circulation within the bloodstream, thereby affording the nanoparticles an augmented opportunity to attain their designated target sites.
CMNPs present an innovative avenue to surmount the constraints associated with conventional synthetic nanoparticles, accomplishing this by amalgamating the merits of cellular membranes with synthetic nanotechnology.63 Augmented stability and extended circulation duration stand as pivotal attributes that synergistically contribute to their capacity for precision-targeted drug conveyance, imaging, and sundry therapeutic applications.
3.5 Facilitated cellular uptake and intracellular delivery
CMNPs, characterized by their distinctive cell membrane coatings and versatile attributes, offer an innovative modality to surmount the challenges pertaining to cellular uptake and intracellular transport.64 The cell membrane envelopment of CMNPs, sourced from diverse cell types, assumes a pivotal role in expediting their engagement with target cells.65 This biointerfacing layer conserves the inherent bioactive constituents and surface markers of the originating cell, thereby facilitating effective recognition and internalization by specific cell subsets intricately involved in bone remodeling and regenerative processes.52,57 Within the osteoporotic context, this signifies the potential customization of CMNPs to encapsulate cell membrane coatings derived from osteoblasts, the bone-forming entities, or osteoclasts, the regulatory agents of bone resorption. These functional coatings serve to heighten the cellular acknowledgement of CMNPs by bone cells, thereby efficaciously instigating their uptake and subsequent intracellular translocation.
Furthermore, the integration of targeting ligands onto the surface of CMNPs serves to further enhance their cellular internalization proficiency and intracellular delivery efficacy.64 These ligands can be selected to exhibit specific binding affinities towards receptors or markers overexpressed on the surface of bone cells. For instance, by targeting receptors such as RANKL or osteocalcin receptors, the interaction of CMNPs with bone-resorbing osteoclasts or bone-forming osteoblasts can be potentiated correspondingly.52 This ligand-facilitated targeting mechanism augments the selectivity of CMNPs towards the pertinent cellular populations, thereby ensuring a heightened concentration of therapeutic agents attains the desired cellular destinations.
Moreover, the internalization of nanoparticles, including CMNPs, frequently occurs via diverse endocytic routes such as clathrin-mediated endocytosis, caveolae-mediated endocytosis, and macropinocytosis.66 Researchers can adeptly harness these pathways to expedite the cellular internalization of CMNPs by bone cells. The manipulation of CMNP surface properties and dimensions can exert influence over the predilection for specific endocytic pathways, thus enabling precise modulation of cellular uptake efficiency.
4. Selection of cell sources for cellular membrane coating
The decision regarding the optimal cell source for cell membrane coating is dependent on the application and intended attributes of the coated nanoparticles. Each cell source offers distinctive merits and constraints, necessitating the assessment of factors encompassing biocompatibility, immunogenicity, isolation feasibility, scalability, and the functional attributes of the resultant coated nanoparticles. In this section, we perform a brief overview of emerging cell membrane coating choices for CMNP.
4.1 Erythrocytes membrane-coated nanoparticles
The utilization of erythrocytes as a cell source for CMNPs constitutes a noteworthy avenue in nanomedicine. Erythrocytes, readily procurable from blood donations or alternative sources such as stored blood units or stem cell-derived erythrocytes, offer an intriguing platform for the coating of nanoparticles with their distinctive membrane.15,61 This natural and biocompatible coating intricately mirrors the inherent attributes of erythrocytes, thereby conferring a myriad of advantages to CMNPs.
First, the erythrocytes’ innate biocompatibility and minimal immunogenicity render them an ideal choice for therapeutic applications.15 This inherent compatibility significantly mitigates the risk of adverse immune responses when CMNPs are employed in therapeutic interventions. More importantly, an additional hallmark of erythrocytes is their extended circulation half-life.18,19 This attribute translates into CMNPs possessing an elongated presence in the bloodstream, a phenomenon that augments their utility in drug delivery applications. The protracted circulation bolsters the prospect of prolonged interactions between CMNPs and target cells, thereby enhancing the efficacy of drug delivery strategies. In a pioneering the Zhang et al. group presented a biomimetic approach, employing a top-down methodology for particle functionalization.15 This involves encapsulating biodegradable polymeric nanoparticles with intact erythrocyte membranes, encompassing both the lipid bilayer and the affiliated membrane proteins. The in vivo experiments involving the administration of nanoparticles loaded with fluorophores to mice unveiled a notably prolonged circulation half-life exhibited by the erythrocyte-mimicking nanoparticles. Furthermore, an investigation into the biodistribution revealed substantial retention of particles within the bloodstream even 72 hours post-injection. The transfer of intact cellular membranes, along with their associated proteins and corresponding functionalities, onto the surface of synthetic particles, presents an innovative and distinctive avenue in nanoparticle functionalization. This approach has the potential to change the field by harnessing the innate attributes of natural cellular components to enhance nanoparticle behavior within biological systems.
Moreover, erythrocytes boast an exceptional discoid morphology, underscored by a slim and pliable membrane.67 These features engender CMNPs with distinctive structural and mechanical attributes. The adaptability and deformability inherent in the RBC membrane confer novel mechanical dimensions to CMNPs, allowing them to maneuver through narrow capillaries and navigate intricate physiological landscapes.68 This property provides possibilities for enhanced tissue penetration and interaction. For instance, Du et al. presents a novel nanoparticle platform, CDC20@ZIF-8@eM-Apt, utilizing zeolitic imidazolate framework-8 (ZIF-8) encapsulated within an erythrocyte membrane cloak and coupled with a targeting aptamer, addressing challenges in bone regeneration by enhancing osteogenic differentiation of bone marrow mesenchymal stem cells (BMSCs), thereby promoting bone regeneration and addressing the bone density loss characteristic of the condition (Fig. 3).69
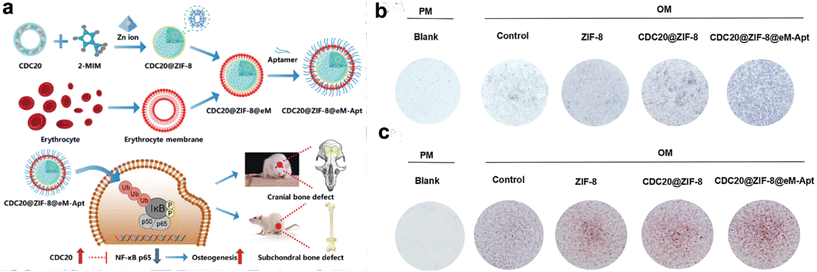 |
| Fig. 3 CDC20-loaded ZIF-8 nanoparticles coated with erythrocyte membranes (eM) promotes osteogenic differentiation of BMSCs. (a) Schematic representation detailing the procedural steps involved in the preparation of CDC20@ZIF-8@eM-Apt nanoparticle. (b and c) Alkaline phosphatase (ALP) staining and Alizarin red S (ARS) staining after cells treated with specific agents. Reproduced with permission.69 Copyright 2023, Wiley-VCH GmbH. | |
4.2 MSCs membrane-coated nanoparticles
MSCs, characterized by their multipotency, exemplify a remarkable versatility. Their adeptness at immunomodulation engenders significant potential for mitigating immune responses, curbing inflammation, and fostering tissue regeneration. Within the context of CMNPs, this attribute translates into the capacity to confer immunomodulatory benefits to the nanoparticles.70 This immunomodulatory potential holds the potential to enhance the therapeutic efficacy of CMNPs, particularly in contexts where immune responses and inflammation need to be managed.
MSCs’ innate self-renewal capabilities and the capacity to differentiate into diverse cell lineages underscore their regenerative potential. In the milieu of CMNPs, these attributes contribute to a multifaceted therapeutic repertoire.71 The potential of MSCs to differentiate into various cell types enhances the versatility of CMNPs, offering tailored regenerative interventions based on the specific cell types required for tissue repair. Furthermore, the feasibility of isolating and expanding MSCs in culture positions them as an attractive cell source for large-scale CMNP production. This scalability aligns with the requisites of mass production, potentially paving the way for widespread therapeutic applications of CMNPs.72
Moreover, MSCs can be engineered or genetically modified to express proteins, receptors, or other functionalities of interest.70,72 This unique attribute allows for the transference of these bioactive components onto CMNPs through the membrane coating, facilitating nuanced customization of CMNPs for specific therapeutic applications. For instance, engineered cell-membrane-coated nanogels, PNG@mR&C, derived from bone mesenchymal stem cell membranes and capable of bone-targeted delivery of therapeutic agents, effectively restore bone metabolic homeostasis by scavenging RANKL, promoting osteogenesis through responsive PTH 1-34 release, and reducing side effects, offering a promising treatment strategy for postmenopausal osteoporosis(Fig. 4).73
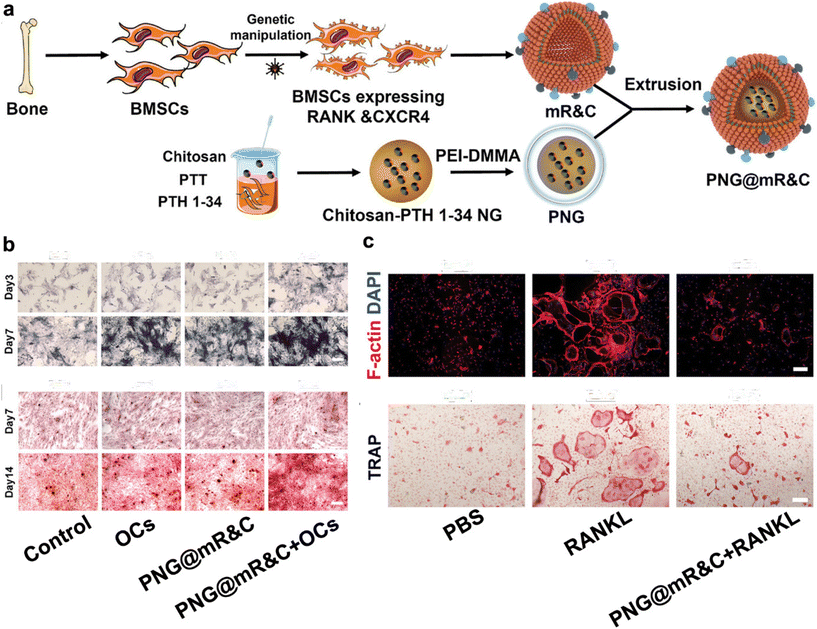 |
| Fig. 4 Engineering bone-targeting PNG@mR&C for multitarget therapy in postmenopausal osteoporosis. (a) Illustration of the PNG@mR&C synthesis process. (b) PNG@mR&C combined with osteoclasts (Ocs) promote ALP activity and mineralized nodule formation. (c) PNG@mR&C combined with RANKL suppress osteoclast differentiation of bone marrow macrophages (BMMs). Reproduced with permission.73 Copyright 2023, Wiley-VCH GmbH. | |
4.3 Macrophage membrane-coated nanoparticles
Macrophages, immune cells pivotal in orchestrating immune responses, inflammation management, tissue repair, and foreign particle clearance, offer an enticing platform for the coating of nanoparticles with their distinctive membrane. Central to the concept of macrophage membrane-coated nanoparticles is the embodiment of macrophages’ inherent attributes.74 These immune cells are adept phagocytes, exerting the activity to eliminate foreign particles. The coating of nanoparticles with macrophage membranes translates into a sophisticated strategy that capitalizes on macrophages’ proficiency in foreign particle clearance. Additionally, macrophages are central players in tissue regeneration and repair, a facet that further augments their utility in the context of CMNPs.74,75
Moreover, a prominent attribute underlying macrophage membrane-coated nanoparticles is their potential for precise and targeted delivery to specific tissues or organs.76,77 Macrophages are ubiquitously distributed, spanning tissues such as the liver, spleen, and bone marrow. This wide-ranging presence translates into an avenue for CMNPs to achieve effective targeting of these tissues.78 The strategic integration of macrophage-coated nanoparticles with microenvironments abundant in macrophages culminates in refined targeting accuracy. In a preceding investigation, the Wang et al. group introduced a biomimetic drug delivery system derived from macrophage membranes enveloping nanoparticles that respond to reactive oxygen species (ROS).78 This incorporation of macrophage membranes serves a dual purpose. Firstly, it effectively prevents the clearance of nanoparticles by evading the reticuloendothelial system. Secondly, it guides the nanoparticles towards sites of inflammation. Additionally, the macrophage membrane actively counteracts proinflammatory cytokines, thereby exerting a localized inhibitory influence on inflammation.79 A comparative analysis of this approach with the utilization of macrophages as carriers for ROS-responsive nanoparticles highlights the suitability of the strategy involving cell membrane-coated drug delivery systems for the management of inflammatory diseases (Fig. 5). Recently, the macrophage membrane-coated nanoparticles have emerged as a promising avenue for bone regeneration and the treatment of osteoporosis.52 For instance, Yin et al. developed a biomimetic anti-inflammatory nano-capsule (BANC) that utilizes lipopolysaccharide-treated macrophage cell membranes with cytokine receptors to block pro-inflammatory cytokines and promote M2 macrophage polarization, enhancing bone tissue repair through controlled release triggered by near-infrared laser irradiation, demonstrating potential therapeutic strategies for bone regeneration.26
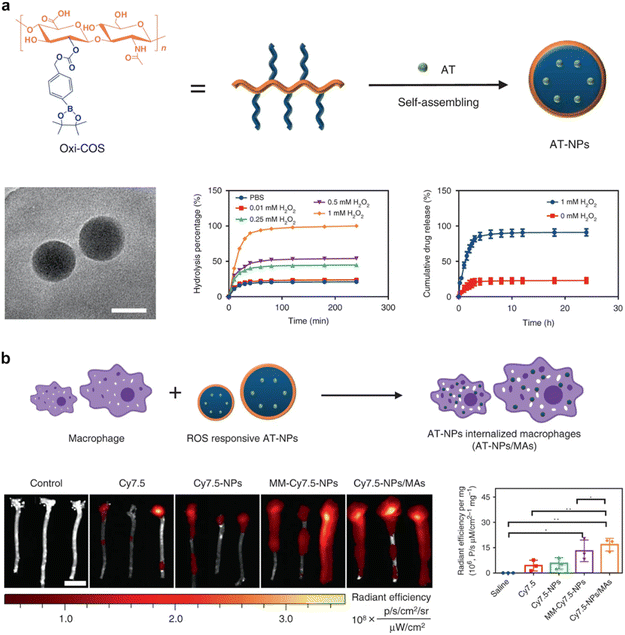 |
| Fig. 5 Macrophage membrane-coated nanoparticles for targeted therapy. (a) Formulation and characterization of ROS-responsive nanoparticles and macrophage membrane-coated nanoparticles. (b) Diagrammatic representation delineates the process of preparing these nanoparticles, the extracorporeal fluorescence bio-imaging, and subsequent quantitative evaluation of the Cy7.5 fluorescent signal within aortic tissues. Reproduced under the terms of a Creative Commons Attribution 4.0 International License.78 Copyright 2020, The Authors. | |
4.4 Neutrophil membrane-coated nanoparticles
As a prominent subset of leukocytes, neutrophils assume a pivotal role in the immune defense against bacterial and fungal infections. Embedded within the framework of neutrophil membrane-coated nanoparticles lies a complex interplay of functionalities intrinsic to neutrophils. Owing to their discernible prevalence within the bloodstream, these immune cells promptly mobilize towards sites marked by infection or inflammation. This choreographed recruitment engenders a pronounced amplification of the targeting efficacy intrinsic to neutrophil membrane-coated nanoparticles, culminating in a deliberate maneuver that navigates towards regions infiltrated by pathogenic incursions.80
The neutrophil membrane, acting as an organic and biocompatible covering, preserves the intrinsic attributes of neutrophils. This facet begets an unparalleled opportunity for CMNPs, imparting various advantages such as enhanced biocompatibility, escalated phagocytic activity, and the potential for precise targeting of infection or inflammation.81 The replication of neutrophil functionalities encapsulated within CMNPs harmonizes with the potential to enhance therapeutic interventions by leveraging innate immunological responses.80
In addition, the paradigm of neutrophil membrane-coated nanoparticles is distinguished by the manipulation of neutrophils to exhibit customized receptors, enzymes, or other functional entities. This progressive stride in bioengineering converges with CMNPs, where such attributes can be conveyed through the membrane coating. This intricate process engenders a novel strategy for tailoring and enhancing the functional attributes of CMNPs, aligning with the unique demands of diverse therapeutic scenarios.80,82 For instance, Liu et al. introduced a novel neutrophil-membrane-coated zeolitic imidazolate framework-8 (ZIF-8) nanodelivery platform (AM@ZIF@NM) designed for targeted transportation of anti-microRNA-155 to endothelial cells.82 The incorporation of neutrophil membrane enhances the specificity of plaque endothelial cell targeting, facilitated by the interaction between the neutrophil membrane protein CD18 and the endothelial cell membrane protein intercellular adhesion molecule-1 (ICAM-1). The ZIF-8 “core” contributes to substantial loading capacity and efficacious escape from endolysosomes. Successful delivery of anti-miR-155 results in effective downregulation of miR-155 expression and preservation of its target gene BCL6. This delivery approach also correspondingly diminishes the expression of RELA and its downstream target genes, namely CCL2 and ICAM-1. Consequently, this anti-miR-155 nanotherapeutic approach exhibits the ability to suppress inflammation within atherosclerotic lesions, thereby mitigating the progression of atherosclerosis (Fig. 6).
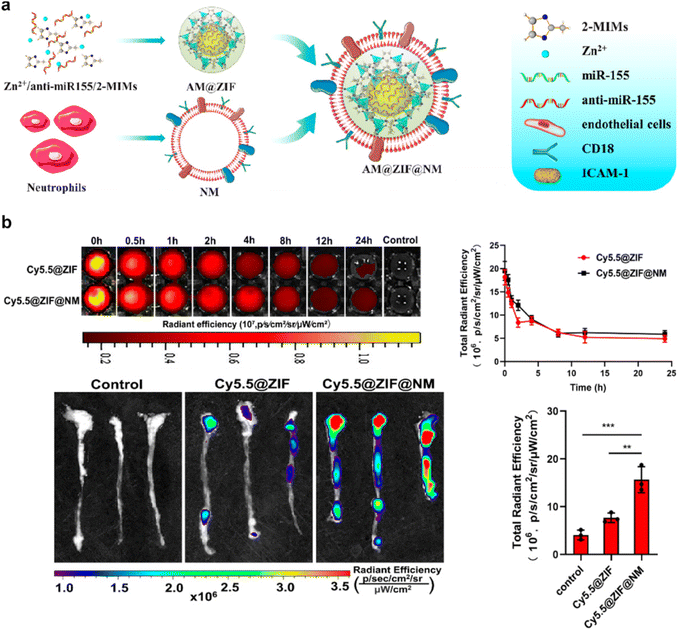 |
| Fig. 6 An example of neutrophil membrane-coated nanoparticles for biomedical application. (a) A schematic depiction elucidating the targeted antiatherosclerosis treatment utilizing neutrophil-membrane-coated ZIF-8 nanoparticles loaded with anti-microRNA-155. (b) Evaluation of the in vivo circulation and targeting potential of Cy5.5-labeled neutrophil-membrane-coated ZIF-8 nanoparticles in mice after intravenous administration. Reproduced with permission.82 Copyright 2023, American Chemical Society. | |
Neutrophils are key players in the inflammatory response associated with osteoporosis. They release pro-inflammatory cytokines and reactive oxygen species, contributing to bone resorption and tissue damage. In addition, NMNPs leverage the inherent homing ability of neutrophils to inflamed tissues, potentially enabling targeted delivery of anti-inflammatory or bone-strengthening agents to osteoporotic lesions. Although direct evidences of using NMNPs for osteoporosis is limited, it is still hold promise for targeted delivery of anti-inflammatory or bone-strengthening agents to osteoporotic lesions by leveraging the innate homing ability of neutrophils to inflamed tissues. For instance, neutrophil-cell-membrane-based biomimetic nanoparticles (NM-LANPs@Ru) could be directly targeting inflamed joints, acting as a nitric oxide (NO) delivery system and exhibiting excellent fluorescence/photoacoustic imaging properties.79
4.5 T cell membrane-coated nanoparticles
T cells, vital defenders within the immune system, assume an extraordinarily specialized role in detecting infected cells. The distinguishing hallmark of T cell functionality is their exceptional ability to identify specific antigens via specialized surface receptors, prominently represented by T cell receptors (TCRs) and co-receptors. This distinctive recognition of antigens constitutes the foundational basis for the design and functionality of CMNPs.83 The emulation of this intricate mechanism within CMNPs orchestrates tailored interventions, thereby enhancing their precision in targeting, proficiency in immunomodulation, and customized functionalities.84
Furthermore, T cells demonstrate an extended capacity for enduring presence, targeted migration to specific tissues, and orchestration of immune responses. These attributes bestow unique advantages upon CMNPs, presenting prospects for sustained drug delivery, immunomodulation, and target therapy.85,86 Moreover, T cells harbor the potential for specific activation and expansion upon encountering their cognate antigens, thus further amplifying the therapeutic impacts of CMNPs.87 In a previous study, Li et al. Developed a Treg cell membrane-coated nanoparticles, which offer a biomimetic strategy for immunosuppression by directly interacting with hyperactive immune cells, effectively inhibiting immuno-inflammatory processes and reducing inflammation and bone resorption in periodontitis models, suggesting their potential as a therapeutic approach for such diseases.88 Nonetheless, a series of challenges should not be neglected. These encompass the possibility of immune recognition directed at T cell-derived coatings, susceptibility to immune rejection in certain contexts, and the intricate task of procuring an ample supply of functional T cells for the extensive production of CMNPs. Each of these challenges needs thorough consideration and effective resolution strategies.
4.6 Osteoblast/osteoclast cell membrane-coated nanoparticles
Osteoblasts and osteoclasts, key players in osteoporosis, exhibit distinct functionalities essential for bone remodeling and homeostasis. Osteoblasts contribute to bone formation by synthesizing bone matrix and minerals. Coating nanoparticles with osteoblast cell membranes could enable them to interact effectively with bone tissue, potentially promoting bone regeneration. For instance, coating nanoparticles with preosteoblastic cells like MC3T3-E1 cell membranes presents a promising strategy for certain bone diseases, as it demonstrates potential to overcome limitations in drug delivery, while optimizing drug loading and delivery features for enhanced efficacy.89 Conversely, osteoclasts are responsible for breaking down bone tissue, which is essential for maintaining bone turnover. Nanoparticles coated with osteoclast membranes pose abundant RANK receptor, thereby neutralized RANKL to prevent osteoclastogenesis.52 However, obtaining a reliable and abundant source of these cells are challenging. For example, isolating osteoclasts from bone marrow can be technically challenging and may yield low quantities of cells. Similarly, obtaining osteoblasts from bone tissue requires invasive procedures and may not always result in pure populations of cells. Additionally, culturing osteoblasts from mesenchymal stem cells can be time-consuming and may involve complex differentiation protocols. Therefore, the development of strategies to overcome these challenges in obtaining these cells for nanoparticle coating is essential for advancing research and potential therapeutic applications in osteoporosis and other bone diseases.
5. Therapeutic applications of CMNPs for bone remodeling
5.1 Enhancement of osteogenic differentiation by CMNPs
The therapeutic potential of CMNPs has unveiled a promising strategy for advancing bone regeneration through the augmentation of osteogenic differentiation and mineralization within MSCs.90 Upon envelopment with membranes derived from MSCs, CMNPs assume the form of biomimetic constructs, closely mirroring the surface characteristics of their parent MSCs. This emulation provides an environment favorable for cell adhesion, proliferation, and, notably, differentiation. The incorporation of cell membrane proteins, encompassing integrins and growth factor receptors, onto CMNPs facilitates the initiation of signaling cascades that underlie osteogenic differentiation.90 This cascade, in turn, culminates in an upsurge of osteogenic marker expression, concomitant with the mineralization process within MSCs. This amalgamation marks a pivotal advance toward the establishment of resilient bone tissue.91,92
Intricately, CMNPs also encapsulate an array of cell membrane-derived factors pivotal for mineralization, including matrix vesicles and calcium-binding proteins.70 Their presence establishes a designated site for the initiation of calcium phosphate deposition, leading to an amplified effect of mineralization. This ultimately results in the crystallization of hydroxyapatite, a fundamental mineral component of bone, thereby substantially enhancing the biomechanical integrity of the regenerated tissue.71
Furthermore, the potential of CMNPs extends beyond mere replication. The deliberate incorporation of distinct osteogenic factors, such as bone morphogenetic proteins (BMPs) and other growth factors, augments their capacity for osteogenesis.72 These factors can be functionally integrated into the CMNP's cell membrane coating or anchored onto their surface. This modification allows for the sustained release and localized delivery of these potent agents, inducing a localized surge in osteogenesis, thus enhancing bone rejuvenation at the precise target site.
For instance, the Cai et al. group introduced a composite ECM-mimetic hydrogel composed of chitosan and collagen, engineered with the integration of MSCs membrane-coated black phosphorus (BP) nanosystem.92 The MSCs membrane-coated BP nanosystem exhibits a mild photothermal effect upon exposure to near-infrared (NIR) light. This effect serves to induce the recruitment of osteoblasts by activating the heat shock proteins (HSPs)-mediated matrix metalloproteinase (MMP) cascade and the ERK-Wnt/β-catenin-RUNX2 signaling axis (Fig. 7a). Concurrently, the thermal decomposition of BP leads to the release of phosphate ions into the surrounding milieu. These released ions act as chemoattractants for calcium ions, facilitating the in situ formation of hydroxyapatite within the ECM. This phenomenon is particularly advantageous for promoting osteoblast migration and fostering osteogenic differentiation (Fig. 7b and c). In vivo, the treatment substantially augments local bone density and fosters the generation of new bone tissue. These findings underscore the promising potential of the innovative MSCs membrane-coated nanosystem in augmenting osteogenic differentiation and facilitating mineralization deposition.
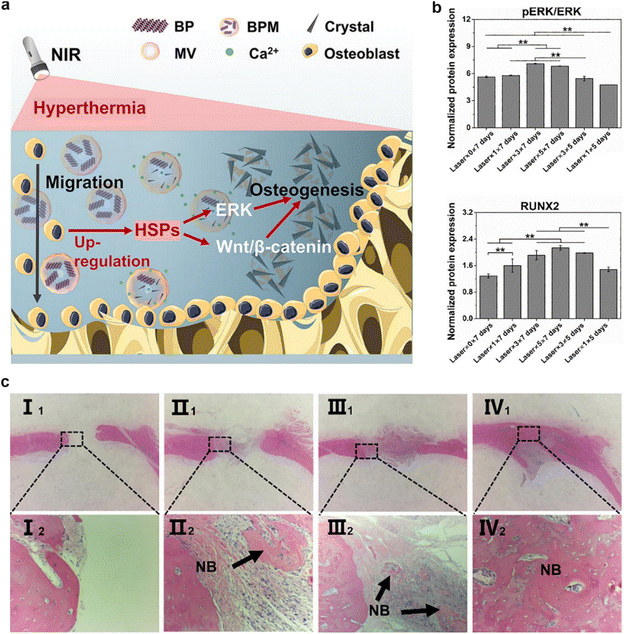 |
| Fig. 7 An example of the enhancement of osteogenic differentiation by CMNPs. (a and b) This CMNPs-based composite effectively imparts a photothermal effect and replicates the physiological matrix vesicle-mediated mineralization process in a biomimetic fashion, thereby fostering bone regeneration. (c) Histological sections were subjected to hematoxylin and eosin (H&E) staining after 8 weeks following implantation. The experimental groups were categorized as follows: Group I consisted of samples (I1, I2) without near-infrared (NIR) laser treatment. Group II included samples (II1, II2) treated once with NIR laser within the 8-week timeframe. Group III encompassed samples (III1, III2) subjected to three separate NIR laser treatments over the 8 weeks. Group IV comprised samples (IV1, IV2) exposed to five distinct NIR laser treatments throughout the 8-week duration. Reproduced with permission.92 Copyright 2022, Elsevier. | |
5.2 Inhibition of osteoclast activity by CMNPs
Osteoclastogenesis, a pivotal process catalyzed by a complex signaling cascade, is mediated by pathways like RANKL.93 In the context of CMNPs, the cell membrane coating emerges as a pioneering approach, orchestrating the disruption of these signaling pathways. Consequently, this interference orchestrates a reduction in the differentiation of osteoclast precursor cells into mature osteoclasts.52 Aiming to impede the progression of bone loss, this intervention seeks to reconstruct the balance in favor of bone preservation.
Notably, CMNPs show their ability to impede bone resorption by effectively inhibiting the attachment and activity of osteoclasts on bone surfaces. This modulation encompasses the intricate interplay of enzymatic secretion and factor release by osteoclasts, culminating in an environment that hampers their proficiency in engaging with bone tissue resorption.52 In a targeted effort to enhance this function, the deliberate incorporation of distinct inhibitors of osteoclast activity, such as bisphosphonates or other anti-resorptive agents, into the cell membrane coating of CMNPs or their surface becomes crucial. This strategic integration capitalizes on their capability for sustained release and localized delivery, orchestrating a highly precise intervention precisely at the site of interest. This effect, catalyzed by CMNPs, stands as a potential strategy to effectively regulate the excessive activity of osteoclasts and mitigate the subsequent cascade of bone resorption.
In a pioneering study, the Yin et al. group developed nanodecoys with the ability to scavenge RANKL and TNF-α. These nanodecoys are created from poly(lactic-co-glycolic acid) (PLGA) nanoparticles coated with membranes derived from preosteoclasts. The utilization of these nanodecoys has proven effective in inhibiting osteoporosis and preserving bone integrity (Fig. 8a).52 The high abundance of RANK and TNF-α receptors (TNF-αR) on the cell membranes effectively neutralizes RANKL and TNF-α, respectively. This dual action prevents the formation of osteoclasts and promotes the development of osteoblasts. The in vivo results indicated the reversal of the osteoporosis progression in ovariectomized mouse models (Fig. 8b and c). The development of these biomimetic nanodecoys offers a promising strategy for restoring the balance between osteoclasts and osteoblasts, representing a significant advance in the clinical management of postmenopausal osteoporosis.
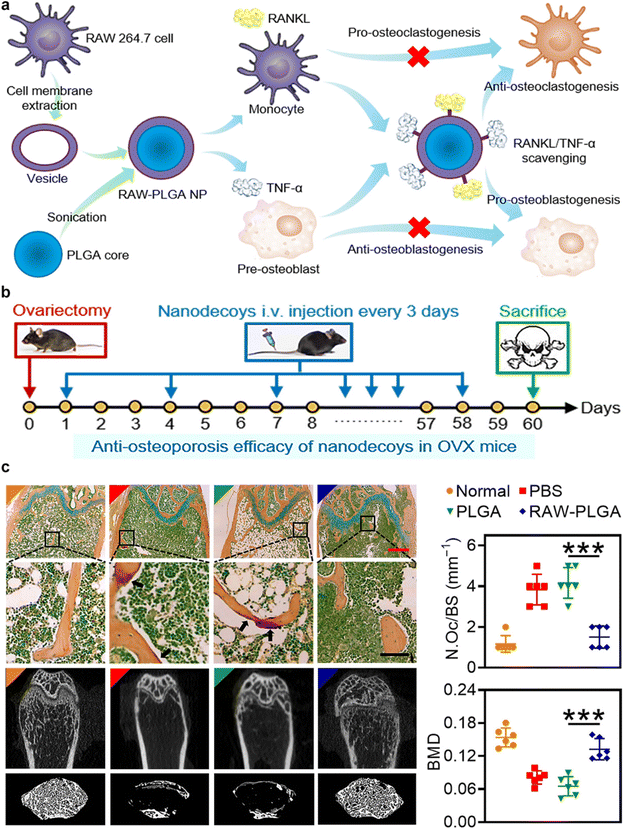 |
| Fig. 8 Preosteoclasts CMNPs for restoring the osteoclast/osteoblast balance in the treatment of postmenopausal osteoporosis. (a) Schematic representation of Preosteoclast CMNPs for Anti-Osteoporosis Management. (b) In vivo study timeline: female C57/BL6 mice underwent bilateral ovariectomy. Over the subsequent 60 days, intravenous injections of PBS, PLGA nanoparticles, or RAW-PLGA nanodecoys were administered at a dosage of 10 mg PLGA per kg every 3 days. On day 60, mice were euthanized for analysis. A group of mice that did not undergo ovariectomy or receive NP/nanodecoy treatment was included as the normal control. (c) Representative photomicrographs of femoral sections stained with tartrate-resistant acid phosphatase, and the representative micro-CT (computed tomography) images of femoral and trabecular bones. Reproduced under the terms of a Creative Commons Attribution 4.0 International License.52 Copyright 2021, The Authors. | |
5.3 Reprogramming bone immune microenvironment
Recent advances in nanomedicine have led to the development of innovative therapeutic approaches aimed at reprogramming the bone immune microenvironment to mitigate osteoporosis.94,95 The bone microenvironment is a dynamic niche with a complex immune component. Immune cells, including macrophages and T cells, play pivotal roles in regulating bone homeostasis.96 Dysregulation of these immune responses, as seen in osteoporosis, leads to increased osteoclast activity and reduced osteoblast function. An understanding of these immune interactions forms the basis for therapeutic interventions.
CMNPs, an emerging nanomedicine platform, have garnered substantial attention due to their unique properties. These nanoparticles are typically derived from cell membranes, such as macrophages or MSCs, and maintain the surface characteristics and functional proteins of their source cells.11 This preservation of native cell properties allows for targeted drug delivery, immune modulation, and improved biocompatibility. CMNPs can be engineered to target specific immune cells within the bone microenvironment. By mimicking the surface markers of immune cells involved in bone regulation, these nanoparticles can modulate immune responses.57 In a prior study, Ding et al. introduced the development of macrophage-mimetic porous SiO2-coated ultra-small Se particles, referred to as porous Se@SiO2 nanospheres, as a potential approach for managing osteolysis.97 Serving as macrophage membrane decoys, these nanoparticles reduced endotoxin levels and neutralized pro-inflammatory cytokines. Furthermore, the release of Se induced macrophage polarization towards the anti-inflammatory M2 phenotype. These effects were mediated through the inhibition of p65, p38, and ERK signaling pathways (Fig. 9a). Additionally, the immune microenvironment created by M-Se@SiO2 alleviated the inhibitory effects of pro-inflammatory cytokines on osteogenic differentiation, as corroborated by both in vitro and in vivo experiments (Fig. 9b and c). These findings underscore the immunomodulatory role of M-Se@SiO2 in LPS-induced inflammation and bone remodeling.
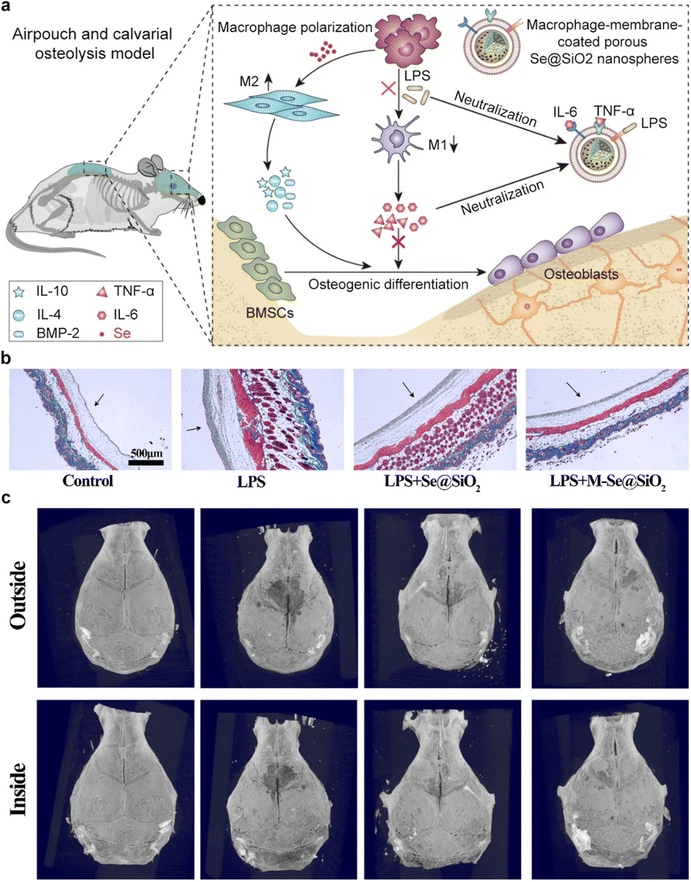 |
| Fig. 9 An example of CMNPs-mediated regulation of bone immune environment. (a) Schematic representation of CMNPs for modulating bone immune microenvironment and enhancing bone remodeling. (b) Masson trichrome staining was employed to examine skin tissue derived from air pouches. Notably, arrows have been employed to demarcate the fibrous layer within the tissue. (c) Three-dimensional reconstructed images of the calvaria were generated for each experimental group. In these images, white arrows are employed to denote areas of osteolysis. Reproduced under the terms of a Creative Commons Attribution 4.0 International License.97Copyright 2021, The Authors. | |
5.4 Combination therapy using CMNPs for osteoporosis
For osteoporosis treatment, the utilization of combination therapy involving CMNPs has arisen as a highly promising strategy.52 The inherent versatility of CMNPs, which can be functionally tailored with a spectrum of drugs and biomolecules, has enabled the achievement of synergistic effects, ultimately translating into markedly enhanced therapeutic outcomes.98 For instance, the Shi et al. group proposed an electroporation-based membrane coating strategy to enhance both anti-inflammatory and osteoconductive therapeutic efficacy.27 The membrane coating achieved through electroporation preserved the structural integrity and orientation of the cell membrane, while also retaining a higher concentration of functional proteins. This led to enhanced properties in terms of the recognition and adsorption of bacteria, toxins, and inflammatory cytokines (Fig. 10a). Leveraging the advantages of electroporation, CMNPs exhibited significantly improved antibacterial and anti-inflammatory capabilities, thereby enhancing the tissue repair process (Fig. 10b). This study introduces a novel self-assembly strategy for cell membrane coating utilizing electroporation, enabling the construction of multifunctional CMNPs tailored for bone remodeling. This approach not only enhances the functional attributes of the coated membrane but also proves to be universally applicable to a variety of nanoparticles or cells. As a result, it holds substantial potential for future applications in the field of bioengineering.
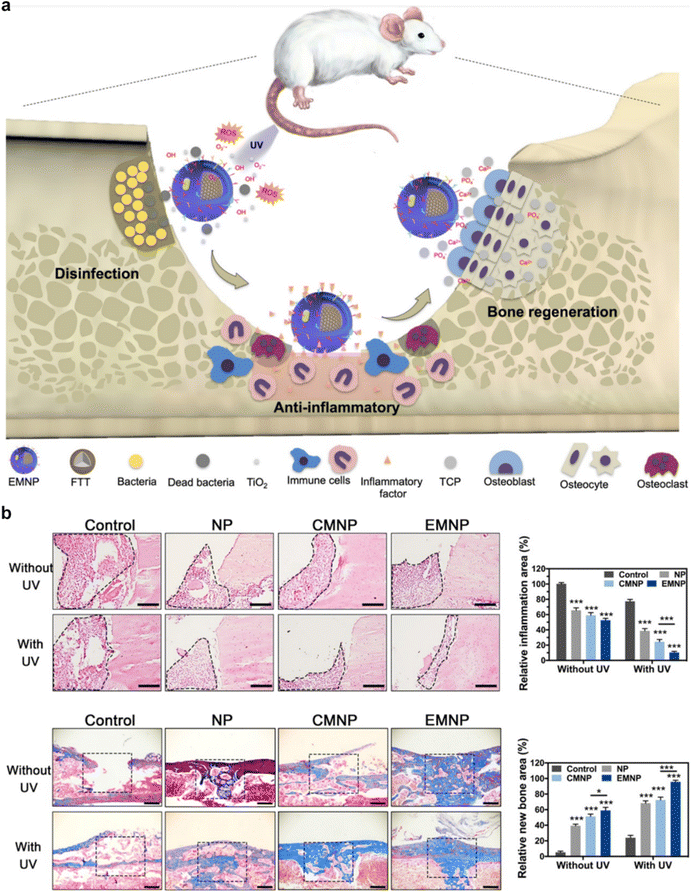 |
| Fig. 10 An example of combination therapy using CMNPs for osteoporosis. (a) Schematic representation of CMNPs for modulating bone immune microenvironment and enhancing bone remodeling. (b) H&E staining was performed on the inflammatory area of the femur after a 5-day treatment period, and Masson staining was performed on the bone repair area after 4 weeks. Reproduced under the terms of a Creative Commons Attribution 4.0 International License.27 Copyright 2021, The Authors. | |
Within the etiology of osteoporosis, the influence of chronic inflammation is well-recognized. In response to this, CMNPs can be configured to carry anti-inflammatory agents such as nonsteroidal anti-inflammatory drugs (NSAIDs) or glucocorticoids.27 These agents, when transported via CMNPs to the bone tissue, have the capacity to mitigate inflammation, thereby creating an environment conducive to effective bone formation. This dual-pronged strategy not only addresses the underlying inflammatory component of osteoporosis but also primes the bone tissue for regeneration.96
Moreover, augmenting CMNPs with bone-targeting moieties like calcium-binding fragments, phosphoserine peptides, or hydroxyapatite-binding peptides results in their preferential accumulation within bone tissue.90 This localization optimizes therapeutic efficacy by ensuring a higher concentration of active agents precisely where they are needed. This strategy aligns with the principle of precision medicine, maximizing the therapeutic impact while minimizing off-target effects.
Additionally, the potential of CMNPs extends to multifaceted therapeutic loading. By encapsulating an array of bioactive substances including drugs, growth factors (BMPs, IGF-1, TGF-β), peptides (osteopontin, osteocalcin, RGD peptides), and bone-targeting agents, a synergistic therapeutic milieu is established. This orchestrated combination capitalizes on diverse mechanisms, engendering a holistic and optimized approach to combatting osteoporosis.
6. Conclusion
While CMNPs hold considerable promise for osteoporosis treatment, the field still faces several challenges that must be addressed to fully realize their potential. In this section, we delve into these challenges and present viable solutions while also exploring future applications of CMNPs in osteoporosis therapy.
6.1 Fabrication challenges and scalability
The fabrication methods for CMNPs can be intricate and reliant on specialized equipment and expertise, potentially limiting scalability and reproducibility. To overcome this challenge, researchers are actively exploring novel fabrication techniques that are cost-effective, scalable, and suitable for large-scale production. These efforts aim to streamline the manufacturing process of CMNPs, making them more accessible for clinical translation.
6.2 Safety and biocompatibility Assurance
While CMNPs are derived from natural cell membranes, ensuring their safety and biocompatibility is paramount. Rigorous evaluation protocols are necessary to assess potential immune responses, toxicity, and long-term effects on the host organism. Collaborative efforts among multidisciplinary teams comprising biologists, chemists, and clinicians are essential to develop standardized safety assessment protocols.
6.3 Regulatory approval pathway
The regulatory pathway for CMNPs as a novel therapeutic intervention for osteoporosis is complex, requiring extensive preclinical and clinical investigations. To expedite this process, collaboration among regulatory agencies, academic institutions, and industry partners is crucial. Establishing clear guidelines and streamlining regulatory pathways for CMNP-based therapies can accelerate their translation from bench to bedside.
6.4 Cost-effectiveness and affordability
Production costs associated with CMNPs, including isolating and characterizing cell membranes, fabricating CMNPs, and functionalizing them with bioactive compounds, may hinder their cost-effectiveness compared to alternative osteoporosis treatment options. Researchers are exploring cost-effective fabrication techniques and alternative sources of cell membranes to reduce production costs without compromising quality and efficacy. Economic modeling studies can provide valuable insights into the cost-effectiveness of CMNP-based therapies, guiding healthcare decision-making and resource allocation.
6.5 Future applications and opportunities
Despite these challenges, CMNPs hold vast potential in osteoporosis therapy. Their unique attributes, such as biocompatibility, biodegradability, and customizable functionality, enable targeted drug delivery, efficient combination therapy, and personalized treatment approaches. Future research directions may include exploring novel applications of CMNPs in regenerative medicine, immunotherapy, and targeted drug delivery to other disease sites beyond osteoporosis. Collaborative efforts among academia, industry, and regulatory agencies are essential to drive innovation in CMNP research and translate scientific discoveries into clinical practice.
In summary, addressing the challenges outlined above is essential to unlock the full potential of CMNPs in osteoporosis therapy. By overcoming fabrication hurdles, ensuring safety and regulatory compliance, and enhancing cost-effectiveness, CMNPs can emerge as a transformative approach for treating osteoporosis and other diseases. Moreover, exploring novel applications and expanding the horizons of CMNP research hold promise for changing healthcare delivery and improving patient outcomes.
Author contributions
J. L., L. L., and X. C. wrote the manuscript. Y. X., W. Z., F. C., and P. C. drew the illustrations. M.-A. S., G. L., and B. M. made substantial contributions to the conception, design of the work and editing of the manuscript. All authors read and approved the final manuscript.
Conflicts of interest
The authors declare no conflict of interest.
Acknowledgements
This work was supported by Wuhan Municipal Bureau of Science and Technology (2022020801020463). J. L., L. L., and X. C. contributed equally to this work. Dr M.-A. Shahbazi acknowledges financial support of the Incentive Fund and Starterbeurs grant from the University of Groningen and University Medical Center Groningen.
References
- H. Lyu, S. S. Zhao, L. Zhang, J. Wei, X. Li and H. Li,
et al., Denosumab and incidence of type 2 diabetes among adults with osteoporosis: population based cohort study, Br. Med. J., 2023, 381, e073435 CrossRef PubMed.
- T. D. Rachner, S. Khosla and L. C. Hofbauer, Osteoporosis: now and the future, Lancet, 2011, 377(9773), 1276–1287 CrossRef CAS PubMed.
- D. M. Black and C. J. Rosen, Clinical Practice. Postmenopausal Osteoporosis, N. Engl. J. Med., 2016, 374(3), 254–262 CrossRef CAS PubMed.
- T. Vilaca, R. Eastell and M. Schini, Osteoporosis in men, Lancet Diabetes Endocrinol., 2022, 10(4), 273–283 CrossRef PubMed.
- K. G. Saag, J. Petersen, M. L. Brandi, A. C. Karaplis, M. Lorentzon and T. Thomas,
et al., Romosozumab or Alendronate for Fracture Prevention in Women with Osteoporosis, N. Engl. J. Med., 2017, 377(15), 1417–1427 CrossRef CAS PubMed.
- A. Sheu, J. R. Greenfield, C. P. White and J. R. Center, Assessment and treatment of osteoporosis and fractures in type 2 diabetes, Trends Endocrinol. Metab., 2022, 33(5), 333–344 CrossRef CAS PubMed.
- S. Starling, New anti-osteoporosis drug target identified, Nat. Rev. Endocrinol., 2021, 17(1), 4–5 CrossRef PubMed.
- M. Shiraki, Y. Yamazaki, T. Kuroda, S. Tanaka and K. Miyata, Serum level of pepsinogen significantly associated with gastric distress induced by amino-bisphosphonates, Osteoporosis Int., 2011, 22(6), 1717–1723 CrossRef CAS PubMed.
- T. J. de Villiers, Clinical issues regarding cardiovascular disease and selective estrogen receptor modulators in postmenopausal women, Climacteric, 2009, 12(Suppl 1), 108–111 CrossRef CAS PubMed.
- T. Matsuyama, T. Morita, Y. Horikiri, H. Yamahara and H. Yoshino, Improved nasal absorption of salmon calcitonin by powdery formulation with N-acetyl-L-cysteine as a mucolytic agent, J. Controlled Release, 2006, 115(2), 183–188 CrossRef CAS PubMed.
- S. Liu, J. M. Yu, Y. C. Gan, X. Z. Qiu, Z. C. Gao and H. Wang,
et al., Biomimetic natural biomaterials for tissue engineering and regenerative medicine: new biosynthesis methods, recent advances, and emerging applications, Mil. Med. Res., 2023, 10(1), 16 Search PubMed.
- Y. Xiong, B. B. Mi, Z. Lin, Y. Q. Hu, L. Yu and K. K. Zha,
et al., The role of the immune microenvironment in bone, cartilage, and soft tissue regeneration: from mechanism to therapeutic opportunity, Mil. Med. Res., 2022, 9(1), 65 CAS.
- Y. Zhao, S. Song, D. Wang, H. Liu, J. Zhang and Z. Li,
et al., Nanozyme-reinforced hydrogel as a H(2)O(2)-driven oxygenerator for enhancing prosthetic interface osseointegration in rheumatoid arthritis therapy, Nat. Commun., 2022, 13(1), 6758 CrossRef CAS PubMed.
- Y. Zhao, D. Wang, T. Qian, J. Zhang, Z. Li and Q. Gong,
et al., Biomimetic Nanozyme-Decorated Hydrogels with H(2)O(2)-Activated Oxygenation for Modulating Immune Microenvironment in Diabetic Wound, ACS Nano, 2023, 17(17), 16854–16869 CrossRef CAS PubMed.
- C. M. Hu, L. Zhang, S. Aryal, C. Cheung, R. H. Fang and L. Zhang, Erythrocyte membrane-camouflaged polymeric nanoparticles as a biomimetic delivery platform, Proc. Natl. Acad. Sci. U. S. A., 2011, 108(27), 10980–10985 CrossRef CAS PubMed.
- C. M. Hu, R. H. Fang, B. T. Luk and L. Zhang, Nanoparticle-detained toxins for safe and effective vaccination, Nat. Nanotechnol., 2013, 8(12), 933–938 CrossRef CAS PubMed.
- J. A. Copp, R. H. Fang, B. T. Luk, C. M. Hu, W. Gao and K. Zhang,
et al., Clearance of pathological antibodies using biomimetic nanoparticles, Proc. Natl. Acad. Sci. U. S. A., 2014, 111(37), 13481–13486 CrossRef CAS PubMed.
- J. G. Piao, L. Wang, F. Gao, Y. Z. You, Y. Xiong and L. Yang, Erythrocyte membrane is an alternative coating to polyethylene glycol for prolonging the circulation lifetime of gold nanocages for photothermal therapy, ACS Nano, 2014, 8(10), 10414–10425 CrossRef CAS PubMed.
- L. Rao, L. L. Bu, J. H. Xu, B. Cai, G. T. Yu and X. Yu,
et al., Red Blood Cell Membrane as a Biomimetic Nanocoating for Prolonged Circulation Time and Reduced Accelerated Blood Clearance, Small, 2015, 11(46), 6225–6236 CrossRef CAS PubMed.
- H. Zhou, Z. Fan, P. K. Lemons and H. Cheng, A Facile Approach to Functionalize Cell Membrane-Coated Nanoparticles, Theranostics, 2016, 6(7), 1012–1022 CrossRef CAS PubMed.
- W. Chen, Q. Zhang, B. T. Luk, R. H. Fang, Y. Liu and W. Gao,
et al., Coating nanofiber scaffolds with beta cell membrane to promote cell proliferation and function, Nanoscale, 2016, 8(19), 10364–10370 RSC.
- Y. Chen, M. Chen, Y. Zhang, J. H. Lee, T. Escajadillo and H. Gong,
et al., Broad-Spectrum Neutralization of Pore-Forming Toxins with Human Erythrocyte Membrane-Coated Nanosponges, Adv. Healthcare Mater., 2018, 7(13), e1701366 CrossRef PubMed.
- Q. Zhang, D. Dehaini, Y. Zhang, J. Zhou, X. Chen and L. Zhang,
et al., Neutrophil membrane-coated nanoparticles inhibit synovial inflammation and alleviate joint damage in inflammatory arthritis, Nat. Nanotechnol., 2018, 13(12), 1182–1190 CrossRef CAS PubMed.
- C. Wang, Y. Wang, L. Zhang, R. J. Miron, J. Liang and M. Shi,
et al., Pretreated Macrophage-Membrane-Coated Gold Nanocages for Precise Drug Delivery for Treatment of Bacterial Infections, Adv. Mater., 2018, 30(46), e1804023 CrossRef PubMed.
- J. Xie, Q. Shen, K. Huang, T. Zheng, L. Cheng and Z. Zhang,
et al., Oriented Assembly of Cell-Mimicking Nanoparticles via a Molecular Affinity Strategy for Targeted Drug Delivery, ACS Nano, 2019, 13(5), 5268–5277 CrossRef CAS PubMed.
- C. Yin, Q. Zhao, W. Li, Z. Zhao, J. Wang and T. Deng,
et al., Biomimetic anti-inflammatory nano-capsule serves as a cytokine blocker and M2 polarization inducer for bone tissue repair, Acta Biomater., 2020, 102, 416–426 CrossRef CAS PubMed.
- M. Shi, K. Shen, B. Yang, P. Zhang, K. Lv and H. Qi,
et al., An electroporation strategy to synthesize the membrane-coated nanoparticles for enhanced anti-inflammation therapy in bone infection, Theranostics, 2021, 11(5), 2349–2363 CrossRef CAS PubMed.
- L. A. Armas and R. R. Recker, Pathophysiology of osteoporosis: new mechanistic insights, Endocrinol. Metab. Clin. North Am., 2012, 41(3), 475–486 CrossRef CAS PubMed.
- A. Chopra, Osteoporosis: a new understanding of its impact and pathogenesis, J. Am. Osteopath. Assoc., 2000, 100(1 Suppl), S1–S4 CAS.
- P. R. Ebeling, H. H. Nguyen, J. Aleksova, A. J. Vincent, P. Wong and F. Milat, Secondary Osteoporosis, Endocr. Rev., 2022, 43(2), 240–313 CrossRef PubMed.
- J. M. Lane, L. Russell and S. N. Khan, Osteoporosis, Clin. Orthop. Relat. Res., 2000,(372), 139–150 CrossRef CAS PubMed.
- V. Fischer and M. Haffner-Luntzer, Interaction between bone and immune cells: Implications for postmenopausal osteoporosis, Semin. Cell Dev. Biol., 2022, 123, 14–21 CrossRef PubMed.
- L. Batoon, S. M. Millard, L. J. Raggatt, A. C. Wu, S. Kaur and L. W. H. Sun,
et al., Osteal macrophages support osteoclast-mediated resorption and contribute to bone pathology in a postmenopausal osteoporosis mouse model, J. Bone Miner. Res., 2021, 36(11), 2214–2228 CrossRef CAS PubMed.
- P. J. Marie, D. Felsenberg and M. L. Brandi, How strontium ranelate, via opposite effects on bone resorption and formation, prevents osteoporosis, Osteoporosis Int., 2011, 22(6), 1659–1667 CrossRef CAS PubMed.
- Z. Mei, X. Dong, Y. Qian, D. Hong, Z. Xie and G. Yao,
et al., Association between the metabolome and bone mineral density in a Chinese population, EBioMedicine, 2020, 62, 103111 CrossRef CAS PubMed.
- J. Luo, Z. Yang, Y. Ma, Z. Yue, H. Lin and G. Qu,
et al., LGR4 is a receptor for RANKL and negatively regulates osteoclast differentiation and bone resorption, Nat. Med., 2016, 22(5), 539–546 CrossRef CAS PubMed.
- W. Sun, S. Guo, Y. Li, J. Li, C. Liu and Y. Chen,
et al., Anoctamin 1 controls bone resorption by coupling Cl(-) channel activation with RANKL-RANK signaling transduction, Nat. Commun., 2022, 13(1), 2899 CrossRef CAS PubMed.
- X. Chen, X. Zhi, P. Pan, J. Cui, L. Cao and W. Weng,
et al., Matrine prevents bone loss in ovariectomized mice by inhibiting RANKL-induced osteoclastogenesis, FASEB J., 2017, 31(11), 4855–4865 CrossRef CAS PubMed.
- Y. H. Cheon, J. Y. Kim, J. M. Baek, S. J. Ahn, H. Y. Jun and M. Erkhembaatar,
et al., WHI-131 Promotes Osteoblast Differentiation and Prevents Osteoclast Formation and Resorption in Mice, J. Bone Miner. Res., 2016, 31(2), 403–415 CrossRef CAS PubMed.
- K. E. Poole, G. M. Treece, R. A. Pearson, A. H. Gee, M. A. Bolognese and J. P. Brown,
et al., Romosozumab Enhances Vertebral Bone Structure in Women With Low Bone Density, J. Bone Miner. Res., 2022, 37(2), 256–264 CrossRef CAS PubMed.
- G. Shen, H. Ren, Q. Shang, W. Zhao, Z. Zhang and X. Yu,
et al., Foxf1 knockdown promotes BMSC osteogenesis in part by activating the Wnt/beta-catenin signalling pathway and prevents ovariectomy-induced bone loss, EBioMedicine, 2020, 52, 102626 CrossRef PubMed.
- J. Korvala, H. Juppner, O. Makitie, E. Sochett, D. Schnabel and S. Mora,
et al., Mutations in LRP5 cause primary osteoporosis without features of OI by reducing Wnt signaling activity, BMC Med. Genet., 2012, 13, 26 CrossRef CAS PubMed.
- R. Baron and M. Kneissel, WNT signaling in bone homeostasis and disease: from human mutations to treatments, Nat. Med., 2013, 19(2), 179–192 CrossRef CAS PubMed.
- Y. Jiang, N. Krishnan, J. Zhou, S. Chekuri, X. Wei and A. V. Kroll,
et al., Engineered Cell-Membrane-Coated Nanoparticles Directly Present Tumor Antigens to Promote Anticancer Immunity, Adv. Mater., 2020, 32(30), e2001808 CrossRef PubMed.
- Y. Gao, Y. Zhu, X. Xu, F. Wang, W. Shen and X. Leng,
et al., Surface PEGylated Cancer Cell Membrane-Coated Nanoparticles for Codelivery of Curcumin and Doxorubicin for the Treatment of Multidrug Resistant Esophageal Carcinoma, Front. Cell Dev. Biol., 2021, 9, 688070 CrossRef PubMed.
- X. Ai, D. Wang, A. Honko, Y. Duan, I. Gavrish and R. H. Fang,
et al., Surface Glycan Modification of Cellular Nanosponges to Promote SARS-CoV-2 Inhibition, J. Am. Chem. Soc., 2021, 143(42), 17615–17621 CrossRef CAS PubMed.
- F. Oroojalian, M. Beygi, B. Baradaran, A. Mokhtarzadeh and M. A. Shahbazi, Immune Cell Membrane-Coated Biomimetic Nanoparticles for Targeted Cancer Therapy, Small, 2021, 17(12), e2006484 CrossRef PubMed.
- J. Zhuang, H. Gong, J. Zhou, Q. Zhang, W. Gao and R. H. Fang,
et al., Targeted gene silencing in vivo by platelet membrane-coated metal-organic framework nanoparticles, Sci. Adv., 2020, 6(13), eaaz6108 CrossRef CAS PubMed.
- Y. Zhang, K. Cai, C. Li, Q. Guo, Q. Chen and X. He,
et al., Macrophage-Membrane-Coated
Nanoparticles for Tumor-Targeted Chemotherapy, Nano Lett., 2018, 18(3), 1908–1915 CrossRef CAS PubMed.
- J. H. Park, Y. Jiang, J. Zhou, H. Gong, A. Mohapatra and J. Heo,
et al., Genetically engineered cell membrane–coated nanoparticles for targeted delivery of dexamethasone to inflamed lungs, Sci. Adv., 2021, 7(25), eabf7820 CrossRef CAS PubMed.
- Z. Chai, X. Hu, X. Wei, C. Zhan, L. Lu and K. Jiang,
et al., A facile approach to functionalizing cell membrane-coated nanoparticles with neurotoxin-derived peptide for brain-targeted drug delivery, J. Controlled Release, 2017, 264, 102–111 CrossRef CAS PubMed.
- Y. Zhou, Y. Deng, Z. Liu, M. Yin, M. Hou and Z. Zhao,
et al., Cytokine-scavenging nanodecoys reconstruct osteoclast/osteoblast balance toward the treatment of postmenopausal osteoporosis, Sci. Adv., 2021, 7(48), eabl6432 CrossRef CAS PubMed.
- D. Shao, F. Zhang, F. Chen, X. Zheng, H. Hu and C. Yang,
et al., Biomimetic Diselenide-Bridged Mesoporous Organosilica Nanoparticles as an X-ray-Responsive Biodegradable Carrier for Chemo-Immunotherapy, Adv. Mater., 2020, 32(50), e2004385 CrossRef PubMed.
- L. Rao, Q. F. Meng, Q. Huang, P. Liu, L. L. Bu and K. K. Kondamareddy,
et al., Photocatalytic Degradation of Cell Membrane Coatings for Controlled Drug Release, Adv. Healthcare Mater., 2016, 5(12), 1420–1427 CrossRef CAS PubMed.
- N. Jan, A. Madni, S. Khan, H. Shah, F. Akram and A. Khan,
et al., Biomimetic cell membrane-coated poly(lactic-co-glycolic acid) nanoparticles for biomedical applications, Bioeng. Transl. Med., 2023, 8(2), e10441 CrossRef CAS PubMed.
- P. Diaz-Saldivar and J. P. Huidobro-Toro, ATP-loaded biomimetic nanoparticles as controlled release system for extracellular drugs in cancer applications, Int. J. Nanomed., 2019, 14, 2433–2447 CrossRef CAS PubMed.
- W. Gao, L. Xiao, Y. Mu and Y. Xiao, Targeting macrophage endocytosis via platelet membrane coating for advanced osteoimmunomodulation, iScience, 2022, 25(10), 105196 CrossRef CAS PubMed.
- A. P. Bidkar, P. Sanpui and S. S. Ghosh, Red Blood Cell-Membrane-Coated Poly(Lactic-co-glycolic Acid) Nanoparticles for Enhanced Chemo- and Hypoxia-Activated Therapy, ACS Appl. Bio Mater., 2019, 2(9), 4077–4086 CrossRef CAS PubMed.
- J. Xiong, M. Wu, J. Chen, Y. Liu, Y. Chen and G. Fan,
et al., Cancer-Erythrocyte Hybrid Membrane-Camouflaged Magnetic Nanoparticles with Enhanced Photothermal-Immunotherapy for Ovarian Cancer, ACS Nano, 2021, 15(12), 19756–19770 CrossRef CAS PubMed.
- L. J. Chen, X. Zhao, Y. Y. Liu and X. P. Yan, Macrophage membrane coated persistent luminescence nanoparticle@MOF-derived mesoporous carbon core-shell nanocomposites for autofluorescence-free imaging-guided chemotherapy, J. Mater. Chem. B, 2020, 8(35), 8071–8083 RSC.
- Q. Xia, Y. Zhang, Z. Li, X. Hou and N. Feng, Red blood cell membrane-camouflaged nanoparticles: a novel drug delivery system for antitumor application, Acta Pharm. Sin. B, 2019, 9(4), 675–689 CrossRef PubMed.
- Y. Li, J. Che, L. Chang, M. Guo, X. Bao and D. Mu,
et al., CD47- and Integrin alpha4/beta1-Comodified-Macrophage-Membrane-Coated Nanoparticles Enable Delivery of Colchicine to Atherosclerotic Plaque, Adv. Healthcare Mater., 2022, 11(4), e2101788 CrossRef PubMed.
- H. Y. Chen, J. Deng, Y. Wang, C. Q. Wu, X. Li and H. W. Dai, Hybrid cell membrane-coated nanoparticles: A multifunctional biomimetic platform for cancer diagnosis and therapy, Acta Biomater., 2020, 112, 1–13 CrossRef CAS PubMed.
- D. Zou, Z. Wu, X. Yi, Y. Hui, G. Yang and Y. Liu,
et al., Nanoparticle elasticity regulates the formation of cell membrane-coated nanoparticles and their nano-bio interactions, Proc. Natl. Acad. Sci. U. S. A., 2023, 120(1), e2214757120 CrossRef CAS PubMed.
- Y. Zou, Y. Sun, Y. Wang, D. Zhang, H. Yang and X. Wang,
et al., Cancer cell-mitochondria hybrid membrane coated Gboxin loaded nanomedicines for glioblastoma treatment, Nat. Commun., 2023, 14(1), 4557 CrossRef CAS PubMed.
- J. H. Park, A. Mohapatra, J. Zhou, M. Holay, N. Krishnan and W. Gao,
et al., Virus-Mimicking Cell Membrane-Coated Nanoparticles for Cytosolic Delivery of mRNA, Angew. Chem., Int. Ed., 2022, 61(2), e202113671 CrossRef CAS PubMed.
- L. Rao, B. Cai, L. L. Bu, Q. Q. Liao, S. S. Guo and X. Z. Zhao,
et al., Microfluidic Electroporation-Facilitated Synthesis of Erythrocyte Membrane-Coated Magnetic Nanoparticles for Enhanced Imaging-Guided Cancer Therapy, ACS Nano, 2017, 11(4), 3496–3505 CrossRef CAS PubMed.
- L. Rao, Q. F. Meng, L. L. Bu, B. Cai, Q. Huang and Z. J. Sun,
et al., Erythrocyte Membrane-Coated Upconversion Nanoparticles with Minimal Protein Adsorption for Enhanced Tumor Imaging, ACS Appl. Mater. Interfaces, 2017, 9(3), 2159–2168 CrossRef CAS PubMed.
- Y. Du, T. Deng, Y. Cheng, Q. Zhao, H. Xia and Y. Ji,
et al., Enhancing Bone Regeneration through CDC20-Loaded ZIF-8 Nanoparticles Wrapped in Erythrocyte Membranes with Targeting Aptamer, Adv. Healthcare Mater., 2024, 13(7), e2302725 CrossRef PubMed.
- L. Fan, A. Wei, Z. Gao and X. Mu, Current progress of mesenchymal stem cell membrane-camouflaged nanoparticles for targeted therapy, Biomed. Pharmacother., 2023, 161, 114451 CrossRef CAS PubMed.
- M. Wang, Y. Xin, H. Cao, W. Li, Y. Hua and T. J. Webster,
et al., Recent advances in mesenchymal stem cell membrane-coated nanoparticles for enhanced drug delivery, Biomater. Sci., 2021, 9(4), 1088–1103 RSC.
- H. H. Wu, Y. Zhou, Y. Tabata and J. Q. Gao, Mesenchymal stem cell-based drug delivery strategy: from cells to biomimetic, J. Controlled Release, 2019, 294, 102–113 CrossRef CAS PubMed.
- Y. Cui, B. Lv, Z. Li, C. Ma, Z. Gui and Y. Geng,
et al., Bone-Targeted Biomimetic Nanogels Re-Establish Osteoblast/Osteoclast Balance to Treat Postmenopausal Osteoporosis, Small, 2024, 20(6), e2303494 CrossRef PubMed.
- N. Khatoon, Z. Zhang, C. Zhou and M. Chu, Macrophage membrane coated nanoparticles: a biomimetic approach for enhanced and targeted delivery, Biomater. Sci., 2022, 10(5), 1193–1208 RSC.
- L. Rao, S. K. Zhao, C. Wen, R. Tian, L. Lin and B. Cai,
et al., Activating Macrophage-Mediated Cancer Immunotherapy by Genetically Edited Nanoparticles, Adv. Mater., 2020, 32(47), e2004853 CrossRef PubMed.
- Y. Han, C. Gao, H. Wang, J. Sun, M. Liang and Y. Feng,
et al., Macrophage membrane-coated nanocarriers Co-Modified by RVG29 and TPP improve brain neuronal mitochondria-targeting and therapeutic efficacy in Alzheimer's disease mice, Bioact. Mater., 2021, 6(2), 529–542 CAS.
- J. Lopes, D. Lopes, M. Pereira-Silva, D. Peixoto, F. Veiga and M. R. Hamblin,
et al., Macrophage Cell Membrane-Cloaked Nanoplatforms for Biomedical Applications, Small Methods, 2022, 6(8), e2200289 CrossRef PubMed.
- C. Gao, Q. Huang, C. Liu, C. H. T. Kwong, L. Yue and J. B. Wan,
et al., Treatment of atherosclerosis by macrophage-biomimetic nanoparticles via targeted pharmacotherapy and sequestration of proinflammatory cytokines, Nat. Commun., 2020, 11(1), 2622 CrossRef CAS PubMed.
- Q. Yu, Y. Huang, X. Chen, Y. Chen, X. Zhu and Y. Liu,
et al., A neutrophil cell membrane-biomimetic nanoplatform based on L-arginine nanoparticles for early osteoarthritis diagnosis and nitric oxide therapy, Nanoscale, 2022, 14(32), 11619–11634 RSC.
- X. Wu, Z. Lin, C. Zhao, L. Liu, K. Zhang and J. Lai,
et al., Neutrophil membrane-coated immunomagnetic nanoparticles for efficient isolation and analysis of circulating tumor cells, Biosens. Bioelectron., 2022, 213, 114425 CrossRef CAS PubMed.
- F. Zhang, J. Zhuang, Z. Li, H. Gong, B. E. de Avila and Y. Duan,
et al., Nanoparticle-modified microrobots for in vivo antibiotic delivery to treat acute bacterial pneumonia, Nat. Mater., 2022, 21(11), 1324–1332 CrossRef CAS PubMed.
- Y. Liu, M. He, Y. Yuan, C. Nie, K. Wei and T. Zhang,
et al., Neutrophil-Membrane-Coated Biomineralized Metal-Organic Framework Nanoparticles for Atherosclerosis Treatment by Targeting Gene Silencing, ACS Nano, 2023, 17(8), 7721–7732 CrossRef CAS PubMed.
- M. Kang, J. Hong, M. Jung, S. P. Kwon, S. Y. Song and H. Y. Kim,
et al., T-Cell-Mimicking Nanoparticles for Cancer Immunotherapy, Adv. Mater., 2020, 32(39), e2003368 CrossRef PubMed.
- W. Ma, D. Zhu, J. Li, X. Chen, W. Xie and X. Jiang,
et al., Coating biomimetic nanoparticles with chimeric antigen receptor T cell-membrane provides high specificity for hepatocellular carcinoma photothermal therapy treatment, Theranostics, 2020, 10(3), 1281–1295 CrossRef CAS PubMed.
- W. Wang, F. Wu, M. Mohammadniaei, M. Zhang, Y. Li and Y. Sun,
et al., Genetically edited T-cell membrane coated AIEgen nanoparticles effectively prevents glioblastoma recurrence, Biomaterials, 2023, 293, 121981 CrossRef CAS PubMed.
- X. Wei, G. Zhang, D. Ran, N. Krishnan, R. H. Fang and W. Gao,
et al., T-Cell-Mimicking Nanoparticles Can Neutralize HIV Infectivity, Adv. Mater., 2018, 30(45), e1802233 CrossRef PubMed.
- Y. Yu, Y. Gao and Y. Yu, “Waltz” of Cell Membrane-Coated Nanoparticles on Lipid Bilayers: Tracking Single Particle Rotation in Ligand-Receptor Binding, ACS Nano, 2018, 12(12), 11871–11880 CrossRef CAS PubMed.
- S. Li, L. Wang, Y. T. Gu, L. Lin, M. M. Zhang and M. Jin,
et al., Biomimetic immunomodulation by crosstalk with nanoparticulate regulatory T cells, Matter, 2021, 4(11), 3621–3645 CrossRef CAS.
- C. Jimenez-Jimenez, A. Moreno-Borrallo, B. Dumontel, M. Manzano and M. Vallet-Regi, Biomimetic camouflaged nanoparticles with selective cellular internalization and migration competences, Acta Biomater., 2023, 157, 395–407 CrossRef CAS PubMed.
- Y. Chen, M. Zhu, B. Huang, Y. Jiang and J. Su, Advances in cell membrane-coated nanoparticles and their applications for bone therapy, Biomater. Adv., 2023, 144, 213232 CrossRef CAS PubMed.
- H. Maeda, T. Kasuga and L. L. Hench, Preparation of poly(L-lactic acid)-polysiloxane-calcium carbonate hybrid membranes for guided bone regeneration, Biomaterials, 2006, 27(8), 1216–1222 CrossRef CAS PubMed.
- L. Tan, Y. Hu, M. Li, Y. Zhang, C. Xue and M. Chen,
et al., Remotely-activatable extracellular matrix-mimetic hydrogel promotes physiological bone mineralization for enhanced cranial defect healing, Chem. Eng. J., 2022, 431(4), 133382 CrossRef CAS.
- H. Xu, Y. Jia, J. Li, X. Huang, L. Jiang and T. Xiang,
et al., Niloticin inhibits osteoclastogenesis by blocking RANKL-RANK interaction and suppressing the AKT, MAPK, and NF-kappaB signaling pathways, Biomed. Pharmacother., 2022, 149, 112902 CrossRef CAS PubMed.
- H. Fu, L. Wang, Q. Bao, D. Ni, P. Hu and J. Shi, Acid Neutralization and Immune Regulation by Calcium-Aluminum-Layered Double Hydroxide for Osteoporosis Reversion, J. Am. Chem. Soc., 2022, 144(20), 8987–8999 CrossRef CAS PubMed.
- X. Liu, F. Li, Z. Dong, C. Gu, D. Mao and J. Chen,
et al., Metal-polyDNA nanoparticles reconstruct osteoporotic microenvironment for enhanced osteoporosis treatment, Sci. Adv., 2023, 9(31), eadf3329 CrossRef CAS PubMed.
- W. Zhou, Z. Lin, Y. Xiong, H. Xue, W. Song and T. Yu,
et al., Dual-Targeted Nanoplatform Regulating the Bone Immune Microenvironment Enhances Fracture Healing, ACS Appl. Mater. Interfaces, 2021, 13(48), 56944–56960 CrossRef CAS PubMed.
- C. Ding, C. Yang, T. Cheng, X. Wang, Q. Wang and R. He,
et al., Macrophage-biomimetic porous Se@SiO(2) nanocomposites for dual modal immunotherapy against inflammatory osteolysis, J. Nanobiotechnol., 2021, 19(1), 382 CrossRef CAS PubMed.
- Y. Deng, M. Ren, P. He, F. Liu, X. Wang and C. Zhou,
et al., Genetically engineered cell membrane-coated nanoparticles for antibacterial and immunoregulatory dual-function treatment of ligature-induced periodontitis, Front. Bioeng. Biotechnol., 2023, 11, 1113367 CrossRef PubMed.
Footnote |
† These authors contributed equally to this work. |
|
This journal is © The Royal Society of Chemistry 2024 |