DOI:
10.1039/D4NJ02217C
(Paper)
New J. Chem., 2024,
48, 13251-13260
Ixora coccinea flower-derived green luminescent carbon quantum dots for Fe3+ recognition and preparation of Pd nanoparticles for the Suzuki–Miyaura coupling and cyanation process†
Received
11th May 2024
, Accepted 25th June 2024
First published on 26th June 2024
Abstract
The solvothermal method of producing spherically shaped and highly fluorescent tiny (∼3 nm) nitrogen-doped carbon quantum dots (NCQDs) with high quantum yield (43%) from Ixora coccinea flowers and o-phenylenediamine has been approached sustainably. Since these NCQDs exhibit excitation-dependent fluorescence activity, they were used to detect the Fe3+ ion selectively in real-time in human blood serum, because it is a component of human blood. The limit of detection (LOD) of the NCQDs was found to be 60 nM. We also synthesized palladium nanoparticles utilizing the NCQDs without additional reducing or stabilizing agents because these quantum dots serve as both. Numerous characterization techniques were used to characterize the nanoparticles, and all the characterizations showed the presence of NCQDs, which stabilize the nanoparticles. Palladium nanoparticles’ small size (4 nm) and large surface area (35.77 m2 g−1) enable them to exhibit strong catalytic activity in Suzuki–Miyaura coupling and cyanation processes. It is obvious that synthetic palladium nanoparticles and NCQDs have a variety of uses in the fields of catalysis and sensing respectively.
1. Introduction
Because of its high quantum yield, photoluminescence, low toxicity, and biocompatibility, carbon quantum dots have garnered significant attention among all the nanomaterials as an alternative to fluorescent organic molecules for a variety of applications, including bioimaging, biocatalysis, and sensing.1 When purifying single-walled carbon nanotubes in 2004, Xu et al. unintentionally found carbon dots. However, in 2006, Sun et al. effectively proved the synthetic process of fluorescence emissive carbon dots with surface passivation and gave the term “carbon quantum dots” (CQDs).2,3 These CQDs are quasispherical, zero-dimensional nanocrystals smaller than 10 nm.4 There are several ways to synthesize CQD, including bottom-up methods like hydrothermal,5 solvothermal,6 pyrolysis,7 and microwave irradiation,8 and top-down methods like arc discharge,9 laser ablation,10 electrochemical exfoliation,11 plasma treatment,12 and chemical oxidation.13,14 Because bottom-up synthesis is inexpensive, easy to carry out, and environmentally friendly, it is classified as a green synthetic approach.15 The common use of this CQD in the field of sensors due to its sensing ability of various analytes such as Ni2+, Pd2+, Fe3+, Fe2+, Mg2+, K+, Cu2+, and many more via quenching methods has been reported. This sensing of various analytes by CQDs is also due to different functional groups present on the surface of the quantum dots. Functional groups like –OH, –NH2, and –COOH, etc. transfer their electrons to the metals and cause quenching in the fluorescence intensities.9 In the year 2022, Nagaraj et al. synthesized CQDs from the endosperm of Borassus flabellifer for the detection of Fe3+ ions.11 In 2016, A Tyagi et al. synthesized CQDs from lemon peel extract as a photocatalyst and for the sensing of Cr6+ ions.12 Various heteroatom-doped quantum dots have also been used in the field of sensing and are giving high quantum yields due to the functional groups present on its surface after doping with heteroatoms.10 In 2022, G. Ge et al. synthesized nitrogen-doped CQDs using a hydrothermal method for the selective detection of Fe3+ ions.13
These quantum dots are famous for their electron-donating as well as electron-withdrawing capabilities. They can donate electrons to the electron-lacking species and accept electrons from electron-rich species.16 As we know, the surface of the CQDs is covered by various functional groups that can be used as a source for the preparation of metal nanoparticles. These CQDs act as reducing as well as stabilizing agents and the metal nanoparticles have numerous applications in the field of catalysis.17,18
The carbon–carbon (C–C) coupling reaction is one of the most fascinating areas of study in organic chemistry. The Suzuki–Miyaura coupling reaction is currently the most widely used technique for creating C–C bonds.19 Numerous industries including pharmaceuticals, plastics, natural goods, etc., use the biaryl compounds that result from C–C coupling processes.20 Palladium complexes with ligands (such as phosphene, imidazole, and N-heterocyclic carbenes) acting as catalysts can be used to create these molecules.21–23 There are additional uses for aryl nitriles in polymers, natural goods, and pharmaceuticals.24,25 However, several hazardous cyanide sources, including potassium cyanide,26 sodium cyanide,27 and zinc cyanide,28 are employed in the production of these aryl cyanide compounds using a palladium catalyst.29 However, K4[Fe(CN)6]·3H2O is a relatively inexpensive and non-toxic cyanide source from which T. Schareina et al. produced aryl cyanide in 2004.30 However, it is tedious to synthesize such compounds because these palladium catalysts are more expensive and the reactions are time-consuming. To overcome these situations, various nanoparticles have been used to synthesize these compounds. There are various palladium nanoparticle syntheses such as green methods31,32 and from quantum dots.33–35 They are used for aryl cyanations31,36 and C–C bond formation.33,34,37
In this work, we present the production of nitrogen-doped carbon quantum dots (NCQDs) using o-phenylenediamine and Ixora coccinea flowers. These NCQDs serve as a reducing and stabilizing agent during the synthesis of palladium nanoparticles (PdNPs) and as a fluorescent probe for the selective detection of the Fe3+ ion. These PdNPs produced from NCQDs have been employed as catalysts in aryl cyanation processes and Suzuki–Miyaura coupling reactions.
2. Experimental
A summary of the dual applications of nitrogen-doped carbon quantum dots (NCQDs), namely fluorescence detection of Fe3+, and Pd nanoparticle (PdNP) synthesis from the prepared NCQDs, is given in the Experimental section. Additionally, how the nanoparticles will be useful in Suzuki–Miyaura coupling and cyanation reactions is also described briefly.
2.1 Material
Ixora coccinea flowers were collected from VIT Vellore. All necessary chemicals and solvents were purchased from chemical suppliers such as Avra, Sigma Aldrich, and TCI chemicals. Using a Bruker Ascent 400 Hz spectrometer, the chemical structure of the produced molecule was examined using 1H (100 MHz) spectra. Tetramethylsilane or TMS was used as the internal standard and CDCl3 and DMSO are used as the solvents when measuring the chemical shift. To record FTIR data, a JASCO 4100 spectrometer was utilized. The absorption studies from UV-Visible spectra were measured on a Hitachi (model 2910) and the FL emission studies were recorded on a Hitachi model (F-7000). Bruker, D8-Advance P-XRD was used for the powder XRD analysis of the PdNPs.
2.2 Preparation of NCQDs
Ixora coccinea flowers, also known as West Indian jasmine flowers, were gathered, cleaned of debris with double distilled water, and then allowed to air dry for two days, after which the flowers were ground into a fine powder using a mortar and pestle. One gram of this powder was extracted using ethanol for 2 hours. Then 40 mL of the extract was mixed with 200 mg of o-phenylenediamine and placed in an autoclave set at 180 °C for six hours. Then, bright green emissive NCQDs were formed as shown in Fig. 1. The obtained quantum dots were allowed to cool to ambient temperature and were filtered through Whatman filter paper and then centrifuged at 6000 rpm for 15 min. After that, pure NCQDs were obtained by dialysis using a cellulose membrane in a glass container and refrigerated for further use to detect Fe3+ and for preparation of Pd nanoparticles. The quantum yield of this NCQD was found to be 43.5% (S1).
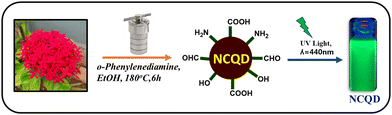 |
| Fig. 1 Preparation of NCQDs from Ixora coccinea flowers. | |
2.3 Fluorescence detection of Fe3+
Using double distilled water, stock solutions of different metals were prepared with a concentration of 1 mM from their corresponding salts. Each of the solutions was diluted to 100 μM concentration. Already prepared NCQDs were diluted 50 times. A cuvette was filled with 2 mL of this NCQD solution, and its fluorescence spectrum was measured at the excitation wavelength of 400 nm. Then, 100 μL of each metal salt solution (100 μM) was introduced one at a time to the cuvette, and the fluorescence was measured. This experiment shows that out of all metal salts, only Fe3+ ions change the fluorescence intensity, which makes it clear that this NCQD is sensitive towards Fe3+ ions only.
2.4 Synthesis of PdNPs
Pd nanoparticles (PdNPs) were synthesized using the previously prepared NCQDs. This procedure involved filling a round-bottom flask with 100 mL of 10 Mmol of Pd(OAc)2 in water, adding 20 mL of NCQD gradually, and then keeping the mixture in a stirred condition in an oil bath at 80 °C. It was noted that after two hours, the compound solution colour changed from light brown to black, indicating that Pd(II) reduced to Pd(0), and the formation of Pd nanoparticles31 and schematic representations are shown in Fig. 2. Subsequently, the mixture was repeatedly centrifuged using water, ethanol, and acetone to eliminate any water-soluble components. The resultant nanoparticles were oven-dried and then confirmed using several characterization techniques. After confirmation, these nanoparticles were used for Suzuki–Miyaura coupling and cyanation reaction and excellent reaction yields were obtained.
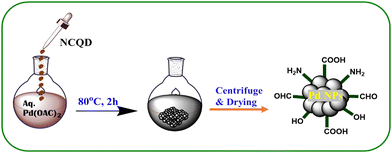 |
| Fig. 2 Preparation of PdNPs from NCQDs. | |
2.5 General protocols for Suzuki–Miyaura-coupling reaction catalyzed by PdNPs
The catalytic activity of PdNPs was utilized for the Suzuki–Miyaura coupling process. In this specific process, a combination of solvents, namely EtOH
:
H2O (1
:
1 v/v), was used to dissolve aryl halide (1 equivalent), boronic acid (1.15 equivalent), potassium carbonate (2 equivalents), and PdNPs (0.3 mol%). After that, an inert atmosphere of nitrogen was used to screen the reaction mixture, and it was stirred under the optimized conditions (Table S2, ESI†). Thin layer chromatography (TLC) was used to monitor the reaction, and after the completion of the reaction, filtering was used to recover the catalyst. After that, the reaction mixture was extracted using a brine solution and ethyl acetate, and the organic layer was collected before being dried against anhydrous Na2SO4. Next, the product was purified in column chromatography using n-hexane and ethyl acetate as the eluent. Using H1 NMR spectroscopy, the final products were characterized (the recyclability of the catalyst after 3 cycles has been shown in the ESI† (Fig. S6)).
2.6 General protocols for cyanation reaction catalyzed by PdNPs
We used these specific PdNPs for the cyanation reaction after obtaining excellent yields from the earlier reactions. In a 50 mL round-bottom flask, aryl halide (1 equivalent), K4[Fe(CN)6]·3H2O (0.18 equivalent), Na2CO3 (1.5 equivalent), PdNPs (0.5 mol%), and DMF as a solvent have been mixed and the optimized reaction conditions (Table S3, ESI†) were found. For the cyanation reactions, K4[Fe(CN)6]·3H2O was employed as a cyanide source. TLC was used to track the reaction, and the catalyst was removed after the completion of the reaction using a simple filtration method. Additionally, ethyl acetate and brine solution were used to extract the product from the reaction mixture. The ethyl acetate layer was then collected and dried using Na2SO4. After that, n-hexane and ethyl acetate were used as an eluent to purify the products in column chromatography. Then the purified product was confirmed using H1 NMR (the recyclability of the catalyst after 3 cycles has been shown in the ESI† (Fig. S6)).
3. Results and discussion
3.1 Characterization of the NCQDs and PdNPs
3.1.1 Characterization and optical behavior of the NCQDs.
FTIR characterization was used to obtain the evidence of NCQD's functional groups, as shown in Fig. 3. The broad peak at 3345 cm−1 displays the asymmetric stretching vibration of the –OH and –NH groups.38 The aliphatic stretching vibrations C–H, C
N, C
O, C
C, –CH2, and –OH of carboxylic acid, and –C–O of ether are represented by the peaks at 2922, 2129, 1721, 1617, 1505, 1450, 1273, and 1043 cm−1 in the synthesized NCQDs, respectively. Using HRTEM analysis, the size and shape of the NCQDs were examined. The average particle size of the quantum dots was determined to be approximately ∼3 nm, and they were present in a spherical shape, well distributed in the solution, as depicted in Fig. S1 (ESI†).
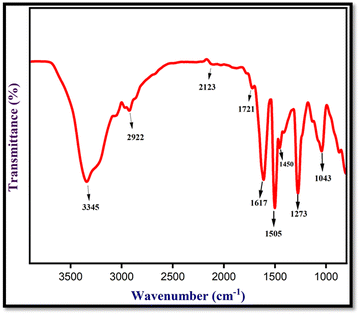 |
| Fig. 3 The FTIR spectrum of NCQDs. | |
The NCQDs showed a light-yellow colour when viewed with the naked eye and they exhibited a bright green emission under a UV lamp. To investigate the optical properties of the synthesized NCQDs, UV-visible and fluorescence spectra were recorded. In Fig. 4, the absorption spectrum shows 3 different bands at 280, 370, and 440 nm where the absorption peak at 280 nm is attributed to the π–π* transition of C
C and C
N, which arises during the synthesis process. Peaks at 370 and 440 nm can be attributed to the n–π* transition of C
O and N
C (which comes due to the nitrogen doping). When excited at 440 nm, the NCQDs exhibit green emission at 500 nm, which suggests that it has a typical luminescent property. Like other carbon dots, this NCQD exhibits excitation-dependent photoluminescence (PL) behaviour (Fig. S2(a), ESI†); this could be because of surface and NCQD size imperfections. Using quinine sulphate as a reference, the quantum yield of the NCQDs was found to be 43.6%. The photostability of the NCQDs was demonstrated by the fact that, following an hour of irradiation at 440 nm, there was no discernible change in fluorescence intensity.
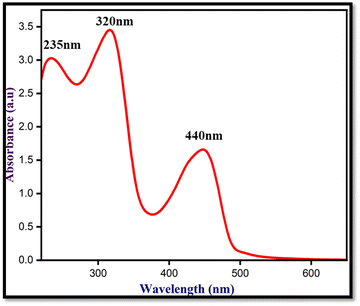 |
| Fig. 4 UV-visible analysis of NCQDs. | |
The pH effect of the NCQDs with different pH values (from 4 to 12) was investigated, which is shown in (Fig. S2(b), ESI†). 0.1 M HCl and NaOH were used and adjusted for the preparation of pH solutions using a pH meter as per the required pH. At 500 nm, the emission showed lower intensity at pH 4 than at neutral and basic pH, which can be attributed to surface coverage of the NCQDs with –OH, –NH2, and –COOH functional groups. However, in neutral pH, deprotonation of the respective functional groups39 occurred and did not show any significant change, confirming the stability of the NCQDs at neutral pH.
3.1.2 Selective and sensitive detection of Fe3+.
The prepared NCQDs were applied as a fluorescent probe to detect Fe3+ ions selectively. Several cations (K+, Ca2+, Mg2+, Cr3+, Fe2+, Fe3+, Co2+, Ni2+, Zr4+, Sr4+, Cu2+, Ag+, Zn2+, Cd2+, Pb2+, Li+, and Hg2+) of the corresponding nitrate salts were taken for the selectivity test. In this specific experiment, 2 mL of 50 times diluted NCQDs were mixed with 100 μm of each metal cation, and the resulting fluorescence emission occurred at 500 nm with the excitation wavelength at 440 nm. There is a noticeable shift in the fluorescence intensity following the addition of the Fe3+ ion. Apart from Fe3+, all the metal ions showed no or negligible change in their fluorescence intensity, as shown in Fig. 5(a). Hence, it is obvious that the NCQDs are selective towards Fe3+ ions only. When Fe3+ ions were added to the NCQD solutions, the green emissive solution changed to a non-emissive solution under UV light or generally quenched upon the addition of Fe3+, as shown in Fig. 5(b). This selectivity was brought about by the complex that is formed between the hydroxyl, the carboxylic group found in the NCQD, and the Fe3+ ion.40
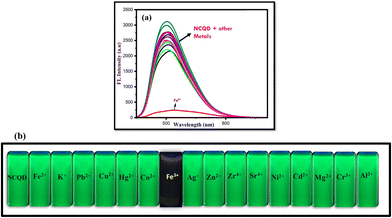 |
| Fig. 5 (a) Selectivity study of NCQDs with different cations, and (b) NCQDs after the addition of Fe3+ along with other cations. | |
Additionally, the NCQDs were tested for selectivity to Fe3+ in the presence of other ions. 100 μm of the most common interfering cations: K+, Mg2+, Cr3+, Fe2+, Co2+, Ni2+, Zr4+, Sr4+, Cu2+, Ag+, Zn2+, Cd2+, Pb2+, Al3+ and Hg2+ along with 100 μm of Fe3+ were added to NCQDs, as illustrated in Fig. 6(a). The results indicate that the addition of various metal ions in addition to Fe3+ did not significantly alter the detection of Fe3+ ions. As shown in Fig. 6(b), upon adding Fe3+ to the NCQDs, the fluorescence intensity of the NCQDs gradually decreased. Increasing the Fe3+ concentration caused a decline in the fluorescence intensity of the NCQDs. The titration was carried out until the addition of 100 μm Fe3+ to the NCQD solution. The emission intensity of the NCQDs decreased after the addition of Fe3+ within 1 second, as given in Fig. S3(a) (ESI†).
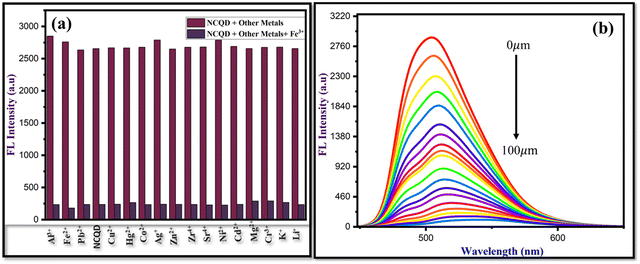 |
| Fig. 6 (a) Interference studies of Fe3+ with different cations and (b) titration of different concentrations of Fe3+ ion with NCQDs. | |
For the calculation of the limit of detection (LoD) of Fe3+, the relative fluorescence response of the NCQDs (F0/F) versus the concentration of Fe3+ (in μm) is given in Fig. S3(b) (ESI†). Where F0 represents the fluorescence intensity before Fe3+ addition and F represents after addition. The correlation coefficient was found to be 0.98271 with a linear range of 1 to 8 μm, which indicates a good linearity relationship. The LOD was found to be 0.06 μm, calculated from the formula,
where
σ represents standard deviation. The LOD obtained for this one is comparably low with the others. The comparison table for different carbon quantum dots is given in the table given in Table S1 (ESI
†).
3.1.3 Real-time applications.
To determine the practical relevance and real-time application of NCQDs with Fe3+, different water samples and human blood serum were tested for Fe3+ content. The tap water and lake water samples were collected from the Vellore Institute of Technology, Vellore, for the analysis. Both the water samples were spiked with a known concentration of Fe3+, but in the case of blood serum, Fe3+ was measured directly. After that, the fluorescence intensities were analyzed for each sample of different concentrations. The recovery of Fe3+ varied from 97.2% to 99.3% (Tables 1 and 2).
Table 1 Determination of Fe3+ ions spiked in environmental water samples
Sample |
Spiked Fe3+ (μM) |
Fe3+ found (μM) |
Recovery (%) |
RSD (n = 3) (%) |
Tap water |
25 |
24.3 |
97.2 |
1.28 |
50 |
48.7 |
97.4 |
1.27 |
75 |
74.2 |
98.9 |
1.29 |
|
Lake water |
25 |
24.5 |
98 |
0.98 |
50 |
48.9 |
97.8 |
0.97 |
75 |
74.5 |
99.3 |
0.98 |
Table 2 Determination of Fe3+ ions in human blood serum
Samples |
Added (μL) |
Found (μm) |
Blood serum |
25 |
24.8 |
50 |
48.6 |
75 |
74.5 |
3.1.4 Sensing mechanism of NCQDs with Fe3+.
The sensing mechanism of NCQDs selectively towards Fe3+ is given in Fig. S4 (ESI†), which is explained as follows. The NCQDs show bright green emissive fluorescence without the addition of Fe3+. This may be due to the fact that when the light falls into the NCQDs, it captures the energy, and the electron moves from the ground state (HOMO) to the excited state (LUMO). When the relaxation occurs, the molecule releases the photons and causes the fluorescence emission.41
The surface of the NCQDs was covered with –OH, –COOH, and –NH2 groups, as proven by FTIR analysis. Due to the half-filled orbital and distinct coordination of Fe3+, the NCQDs exhibited a relatively high degree of selectivity towards Fe3+. Fe3+ may interact with the functional group-covered NCQD surface. When the five half-filled orbitals of Fe3+ combined with those groups on the surface of the NCQDs, they absorbed the excited electrons to form a non-radiative combination that quenched the fluorescence.42 The schematic representation is illustrated in Fig. S4 (ESI†).
3.2 Characterization of palladium nanoparticles (PdNPs)
The synthesized palladium nanoparticles (PdNPs) from the NCQDs were characterized using various characterization techniques, such as Fourier transform infrared spectroscopy (FT-IR), powder X-ray diffraction (p-XRD), field emission scanning electron microscope (FESEM), energy dispersivee X-ray (EDS), high-resolution transmission electron microscopy (HRTEM), Brunauer–Emmett–Teller (BET), thermogravimetric analysis (TGA), and induced coupled plasma-optical emission spectroscopy (ICP-OES). The FT-IR characterization was performed for the PdNPs, which is given in Fig. 7(a). The peaks at 3369, 2929, 2122, 1520, 1415, and 1033 cm−1 are attributed to –OH & –NH, aliphatic and aromatic –CH, C
N, aliphatic –CH2, –OH of carboxylic acid, and –C–O of ether stretching vibrations, respectively. This is due to the absorption of phytochemicals present in the NCQDs during the reduction and formation process of the PdNPs. The peaks are slightly shifted due to the interaction of the PdNPs and the NCQDs.
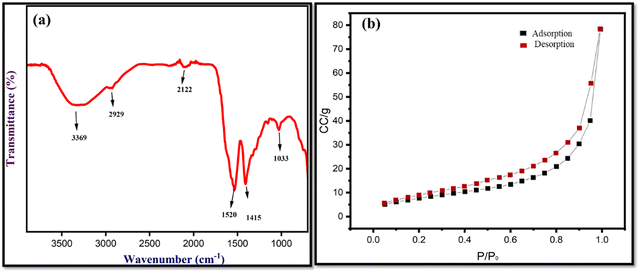 |
| Fig. 7 (a) FTIR analysis of NCQDs and PdNPs. (b) BET analysis of PdNPs. | |
To determine the surface area as well as the porosity of the PdNPs, BET analysis was employed. From the study, it was found that it had a surface area of 35.77 m2 g−1. The N2 adsorption–desorption isotherm of the PdNPs is shown in Fig. 7(b). It exhibits a type II isotherm with a hysteresis loop, Fig. 7(b), which indicates the characteristics of a mesoporous material. Due to its larger surface area, it acts as an excellent nanocatalyst for the reactions.
To get the morphology of the PdNPs, FESEM analysis was done. From the analysis, we observed that the nanoparticles exhibited a spherical morphology Fig. 8(a)–(c). In their morphology, it is clearly visible that the quantum dots are nicely coated with the nanoparticles. For the confirmation of elements present in the PdNPs, EDAX analysis was carried out. This confirmed the palladium, carbon, nitrogen, and oxygen present in the nanoparticles and they are uniformly distributed, which is due to the interaction of the NCQDs and PdNPs as shown in Fig. 8(d). ICP-OES analysis confirmed that the PdNPs have a palladium content of 50.28% w/w.
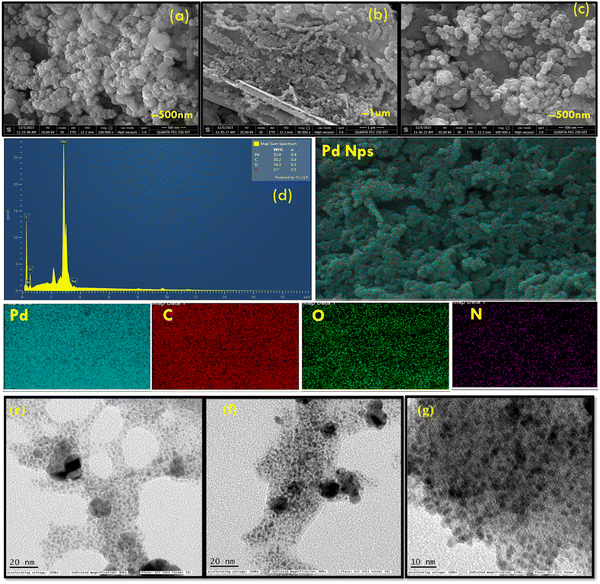 |
| Fig. 8 (a)–(c) FESEM Image of PdNPs, (d) EDAX image of PdNPs, and (e)–(g) HRTEM image of PdNPs. | |
For the confirmation of the magnified morphology and size of the PdNPs, HRTEM analysis was carried out. From the HRTEM analysis, it was confirmed that the shape of the PdNPs exhibited a spherical morphology, and the size ranged from 4 to 5 nm, which is shown in Fig. 8(e)–(g).
Thermogravimetric analysis was performed to determine the thermal stability of the PdNPs and the absorbed chemicals of NCQDs on the PdNPs, Fig. 9(a). The TG analysis was carried out at a heating rate of 10 °C per minute in a nitrogen atmosphere from 40 to 800 °C. The initial weight loss of 5–6%, which occurred up to 150 °C temperature, was due to the moisture absorbed by the PdNPs. Almost 18% sharp weight loss occurred at 400 °C due to the removal of volatile organic molecules that are present in the NCQDs. A total of around 30% weight loss at around 800 °C is due to the phytochemicals that are present in the NCQD absorbed in the PdNPs.31
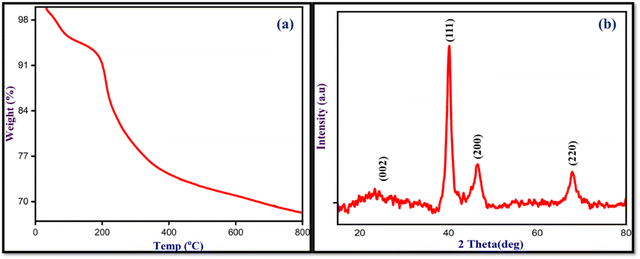 |
| Fig. 9 (a) TGA image and (b) XRD image of PdNPs. | |
To determine the precise composition and crystallinity of the prepared PdNPs, a powder X-ray diffraction analysis was carried out (Fig. 9(b)). The large peak at about 2θ = 23° shows the existence of carbon dots; furthermore, there are three notable peaks at 2θ = 40.03°, 46.51° and 68.03° corresponding to the diffractive indices as (111), (200) and (220) which validates the PdNP nanoparticle's formation from NCQDs. The size of the Pd nanoparticles is 4.4 nm. This was calculated using the formula D = (0.94λ)/β
cos
θ, which has a good acceptance with the HRTEM result. It is confirmed by the observed evidence that Pd nanoparticles have been produced from the quantum dots, and their small size makes it a clear choice for good catalytic conversions in coupling processes. XRD data after 3 times recycled PdNPs after Suzuki–Miyaura coupling and cyanation reactions have been given in Fig. S6 (ESI†).
3.2.1 Application of PdNPs in Suzuki–Miyaura coupling reaction.
The PdNPs were first tested for the Suzuki–Miyaura coupling reactions. To optimize the reaction conditions, phenylboronic acid of substituted benzene and bromobenzene were selected as model reactions. EtOH:H2O was a convenient solvent system for the optimized reactions. As shown in Table S2 (ESI†), a different set of reactions was carried out to get the optimized reaction conditions. The reaction did not proceed without a base and Pd catalyst (Table S1, Sl. no. 11 and 12, ESI†). During the optimization reactions, a variety of bases, including K2CO3, Na2CO3, NaOH, and KOH, as well as a variety of solvents, including EtOH, H2O, DMF, THF, acetonitrile, acetone, and 1,4-dioxane, were utilized at varying temperatures. However, the reaction proceeded smoothly under the ideal reaction conditions, which were the EtOH
:
H2O (1
:
1) solvent system, K2CO3 (2 equivalents) as the base, and PdNPs (0.3 mol%) at 40 °C (Table S2, Sl. no. 17, ESI†). The yield did not rise further even with increases in temperature, catalyst loading, and time (Table S2, entries 16 and 18, ESI†). Thus, aryl halide (1 equivalent), phenylboronic acid (1.15 equivalent), PdNPs (0.3 mol%), and K2CO3 (2 equivalent) at 40 °C for 1.5 hours are the ideal reaction conditions for the Suzuki–Miyaura reaction. The plausible mechanism for the Suzuki–Miyaura coupling reaction with PdNPs has been given in Fig. S5(a) (ESI†).
The substrate scope in the Suzuki–Miyaura coupling reaction with PdNPs with the optimized conditions was analyzed with various substituted groups of aryl halide as well as phenylboronic acid and has been given in Table 3. The substituted aryl halide and phenylboronic acid gave excellent yields in the optimized conditions. The products were purified by column chromatography and confirmed by H1 NMR. As compared to aryl chloride, aryl bromide gave excellent yields in these particular reaction conditions.
Table 3 Substrates of Suzuki–Miyaura coupling reactions with different aryl halides and phenylboronic acids
3.2.2 Application of PdNPs in cyanation reaction.
This catalyst was used for the aryl halide cyanation process after demonstrating good catalytic activity of PdNPs in the Suzuki–Miyaura coupling reactions. K4[Fe(CN)6]·3H2O was selected as the cyanide source for this specific reaction due to its non-toxic nature and environmental friendliness. For this particular reaction, bromobenzene was selected as a model substrate, similar to the Suzuki–Miyaura reactions.
For the optimization of the reactions, various bases like K2CO3, Na2CO3, KOH, NaOH and various solvents like EtOH, H2O, THF, acetonitrile, and DMF, were used with different temperatures with or without the presence of Pd catalyst. But with Na2CO3 (1.5 equivalents), DMF (6 mL) solvent at 110 °C with PdNPs (0.5 mol%) and K4[Fe(CN)6]·3H2O (0.18 equivalent as source of cyanide) for 3.5 h, it turned out to be the best condition (Table S4 Sl. no. 16, ESI†). The plausible mechanism is given in the ESI† in Fig. S5(b).
The substrate scope in the aryl halide cyanation reaction with PdNPs with the optimized conditions was analyzed with various substituents on the aryl halide and the data are given in Table 3. The substituted aryl halide and K4[Fe(CN)6] 3H2O give excellent yields in the optimized conditions. The products were purified by column chromatography and confirmed by H1 NMR. As compared to aryl chloride, aryl bromide gave excellent yields in these particular reaction conditions (Table 4).
Table 4 Aryl cyanation reactions with different aryl halides and K4[Fe(CN)6]·3H2O
4. Conclusion
The current work demonstrated the sustainable synthesis of nitrogen-doped carbon quantum dots (NCQD) from Ixora coccinea flowers and o-phenylenediamine using a solvothermal technique. The synthetic NCQDs have a surface coated with many functional groups and measure ∼3 nanometres in size. They produced fluorescence with a bright green colour. These NCQDs were discovered to have a quantum yield of 43.5%. The research reports that these NCQDs can detect Fe3+ ions and may find practical usage in real-time applications and in human serum. In addition, these NCQDs serve as a stabilizing and reducing agent when palladium nanoparticles (PdNPs) are being prepared. The functional groups in the NCQDs stabilize the nanoparticles and allow them to reduce from Pd(II) to Pd(0). Furthermore, these PdNPs catalyze the aryl cyanation and Suzuki–Miyaura coupling reactions. Due to their tiny size and large surface area, these nanoparticles, which are derived from NCQDs, provide excellent yields for both processes. In comparison to other reported catalysts, the yields are comparably good.
Data availability
The data supporting this article have been included as part of the ESI.†
Conflicts of interest
There is no conflict of declaration.
Acknowledgements
Namrata P. Hota expresses gratitude to Vellore Institute of Technology for its Research Associateship, which enables her to get financial support. The DST-FIST NMR facility at VIT University is acknowledged.
References
- Y. Liu, L. Jiang, B. Li, X. Fan, W. Wang, P. Liu, S. Xu and X. Luo, J. Mater. Chem. B, 2019, 7, 3053–3058 RSC.
- X. Xu, R. Ray, Y. Gu, H. J. Ploehn, L. Gearheart, K. Raker and W. A. Scrivens, J. Am. Chem. Soc., 2004, 126, 12736–12737 CrossRef CAS PubMed.
- Y.-P. Sun, B. Zhou, Y. Lin, W. Wang, K. S. Fernando, P. Pathak, M. J. Meziani, B. A. Harruff, X. Wang and H. Wang, J. Am. Chem. Soc., 2006, 128, 7756–7757 CrossRef CAS PubMed.
- X. Zhang, J. Lu, X. Li, L. Chai and Y. Jiao, New J. Chem., 2017, 41, 702 RSC.
- Y. Liu, Q. Zhou, J. Li, M. Lei and X. Yan, Sens. Actuators, B, 2016, 237, 597–604 CrossRef CAS.
- P. Zhao, X. Li, G. Baryshnikov, B. Wu, H. Ågren, J. Zhang and L. Zhu, Chem. Sci., 2018, 9, 1323–1329 RSC.
- C. Wang, M. Yang, H. Shi, Z. Yao, E. Liu, X. Hu, P. Guo, W. Xue and J. Fan, Dyes Pigm., 2022, 204, 110431 CrossRef CAS.
- M. Xu, S. Xu, Z. Yang, M. Shu, G. He, D. Huang, L. Zhang, L. Li, D. Cui and Y. Zhang, Nanoscale, 2015, 7, 15915–15923 RSC.
- W. Li, Z. Zhang, B. Kong, S. Feng, J. Wang, L. Wang, J. Yang, F. Zhang, P. Wu and D. Zhao, Angew. Chem., Int. Ed., 2013, 52, 8151–8155 CrossRef CAS.
- Q. Xu, T. Kuang, Y. Liu, L. Cai, X. Peng, T. S. Sreeprasad, P. Zhao, Z. Yu and N. Li, J. Mater. Chem. B, 2016, 4, 7204–7219 RSC.
- M. Nagaraj, S. Ramalingam, C. Murugan, S. Aldawood, J.-O. Jin, I. Choi and M. Kim, Environ. Res., 2022, 212, 113273 CrossRef CAS PubMed.
- A. Tyagi, K. M. Tripathi, N. Singh, S. Choudhary and R. K. Gupta, RSC Adv., 2016, 6, 72423–72432 RSC.
- G. Ge, L. Li, M. Chen, X. Wu, Y. Yang, D. Wang, S. Zuo, Z. Zeng, W. Xiong and C. Guo, Nanomaterials, 2022, 12, 986 CrossRef CAS PubMed.
- T. Arumugham, M. Alagumuthu, R. G. Amimodu, S. Munusamy and S. K. Iyer, Sustainable Mater. Technol., 2020, 23, e00138 CrossRef CAS.
- H. H. Jing, F. Bardakci, S. Akgöl, K. Kusat, M. Adnan, M. J. Alam, R. Gupta, S. Sahreen, Y. Chen and S. C. Gopinath, J. Funct. Biomater., 2023, 14, 27 CrossRef CAS.
- I. Srivastava, J. S. Khamo, S. Pandit, P. Fathi, X. Huang, A. Cao, R. T. Haasch, S. Nie, K. Zhang and D. Pan, Adv. Funct. Mater., 2019, 29, 1902466 CrossRef.
- I. Srivastava, J. S. Khamo, S. Pandit, P. Fathi, X. Huang, A. Cao, R. T. Haasch, S. Nie, K. Zhang and D. Pan, Adv. Funct. Mater., 2019, 29, 1902466 CrossRef.
- M. Jayanthi, S. Megarajan, S. B. Subramaniyan, R. K. Kamlekar and A. Veerappan, J. Mol. Liq., 2019, 278, 175–182 CrossRef CAS.
- M. C. D'Alterio, È. Casals-Cruañas, N. V. Tzouras, G. Talarico, S. P. Nolan and A. Poater, Chem. – Eur. J., 2021, 27, 13481–13493 CrossRef PubMed.
- J. Magano and J. R. Dunetz, Chem. Rev., 2011, 111, 2177–2250 CrossRef CAS.
- R. Martin and S. L. Buchwald, Acc. Chem. Res., 2008, 41, 1461–1473 CrossRef CAS.
- S. S. Ng, Z. Chen, O. Y. Yuen and C. M. So, Org. Biomol. Chem., 2022, 20, 1373–1378 RSC.
- S. Xu, K. Song, T. Li and B. Tan, J. Mater. Chem. A, 2015, 3, 1272–1278 RSC.
- H. Yu, R. N. Richey, W. D. Miller, J. Xu and S. A. May, J. Org. Chem., 2011, 76, 665–668 CrossRef CAS PubMed.
- M. Nasrollahzadeh, B. Jaleh, P. Fakhri, A. Zahraei and E. Ghadery, RSC Adv., 2015, 5, 2785–2793 RSC.
- M. Sundermeier, A. Zapf, M. Beller and J. Sans, Tetrahedron Lett., 2001, 42, 6707–6710 CrossRef CAS.
- A. V. Ushkov and V. V. Grushin, J. Am. Chem. Soc., 2011, 133, 10999–11005 CrossRef CAS.
- H. Kubota and K. C. Rice, Tetrahedron Lett., 1998, 39, 2907–2910 CrossRef CAS.
- D. T. Cohen and S. L. Buchwald, Org. Lett., 2015, 17, 202–205 CrossRef CAS PubMed.
- T. Schareina, A. Zapf and M. Beller, J. Organomet. Chem., 2004, 689, 4576–4583 CrossRef CAS.
- V. Kandathil, R. B. Dateer, B. Sasidhar, S. A. Patil and S. A. Patil, Catal. Lett., 2018, 148, 1562–1578 CrossRef CAS.
- S. Vinodhini, B. S. M. Vithiya and T. A. A. Prasad, J. King Saud Univ., Sci., 2022, 34, 102017 CrossRef.
- R. Bayan and N. Karak, ACS Omega, 2017, 2, 8868–8876 CrossRef CAS PubMed.
- D. Dey, T. Bhattacharya, B. Majumdar, S. Mandani, B. Sharma and T. K. Sarma, Dalton Trans., 2013, 42, 13821–13825 RSC.
- P. Chandrasekaran, T. N. J. I. Edison and M. G. Sethuraman, Int. J. Hydrogen Energy, 2020, 45, 28800–28811 CrossRef CAS.
- T. Baran and M. Nasrollahzadeh, Inorg. Chem. Commun., 2020, 119, 108117 CrossRef CAS.
- S. Lebaschi, M. Hekmati and H. Veisi, J. Colloid Interface Sci., 2017, 485, 223–231 CrossRef CAS.
- T. Boobalan, M. Sethupathi, N. Sengottuvelan, P. Kumar, P. Balaji, B. Gulyás, P. Padmanabhan, S. T. Selvan and A. Arun, ACS Appl. Nano Mater., 2020, 3, 5910–5919 CrossRef CAS.
- S. Li, Y. Li, J. Cao, J. Zhu, L. Fan and X. Li, Anal. Chem., 2014, 86, 10201–10207 CrossRef CAS.
- V. Raveendran, A. R. S. Babu and N. K. Renuka, RSC Adv., 2019, 9, 12070–12077 RSC.
- J. Yu, C. Liu, K. Yuan, Z. Lu, Y. Cheng, L. Li, X. Zhang, P. Jin, F. Meng and H. Liu, Nanomaterials, 2018, 8, 233 CrossRef PubMed.
- S. Munusamy, S. Sawminathan, T. Arumugham, M. Casales Díaz, S. Godavarthi and M. K. Kesarla, J. Nanomater., 2021, 2021, 1–11 CrossRef.
|
This journal is © The Royal Society of Chemistry and the Centre National de la Recherche Scientifique 2024 |