DOI:
10.1039/D3NJ03799A
(Perspective)
New J. Chem., 2024,
48, 437-505
Advanced graphene-based (photo & electro) catalysts for sustainable & clean energy technologies
Received
13th August 2023
, Accepted 8th November 2023
First published on 9th November 2023
Abstract
Due to the wide range of uses of graphene and its composites in electrocatalysis and photocatalysis, there has been a lot of interest in these materials. The most recent advancements in the use of graphene-based materials for these two important catalytic processes are highlighted in this review. The special characteristics of graphene, such as its high surface area and superior charge transport, have allowed for improved light absorption and effective electron–hole separation in the context of photocatalysis. Additionally, the photocatalytic performance for many environmental and energy-related applications has been enhanced by the addition of additional materials to graphene composites. However, graphene's better electrical conductivity and sizable active surface area have made it possible to use it as a superior catalyst support in electrocatalysis, encouraging electrochemical events including oxygen evolution and reduction reactions. This review offers insights into the potential of graphene and graphene-based composites for sustainable and clean energy technologies by providing a thorough overview of current developments, difficulties, and future possibilities in the fields of photocatalysis and electrocatalysis.
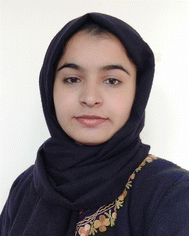
Raheela Akhter
| Raheela Akhter completed her BSc from Govt. Degree College for Women Sopore. She received her MSc in chemistry from the University of Kashmir, Hazratbal, Srinagar, and BEd from the University of Kashmir, Hazratbal, Srinagar. Currently, she is pursuing her PhD in materials chemistry/catalysis under the supervision of Dr Shrikant S. Maktedar at Materials Chemistry & Engineering Research Laboratory, Department of Chemistry, National Institute of Technology Srinagar, J&K. |
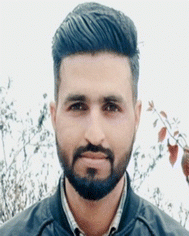
Shokat Hussain
| Shokat Hussain completed his BSc from Govt. Post Graduate College Rajouri. He received his MSc in chemistry from the University of Jammu, BEd from the Central University of Kashmir and BLIS from IGNOU. Currently, he is pursuing his PhD in materials chemistry/catalysis under the supervision of Dr Shrikant S. Maktedar at Materials Chemistry & Engineering Research Laboratory, Department of Chemistry, National Institute of Technology Srinagar, J&K. |
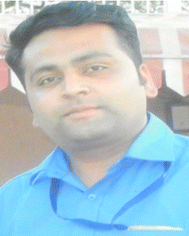
Shrikant S. Maktedar
| Dr Shrikant S. Maktedar is Assistant Professor in the Department of Chemistry, National Institute of Technology (Ministry of Education, Government of India), Srinagar, J&K, India. His research group focuses on the study of advanced materials for energy, environment & healthcare sustainability. Furthermore, in line with the current research objectives, the group is passionately addressing the emerging challenges in society through real-time prototypes. |
1. Introduction
Two-dimensional carbon compound graphene, which has a honeycomb lattice structure, has become recognized as a remarkable nanomaterial with exceptional qualities and a wide range of uses. It offers a promising foundation for numerous technological breakthroughs owing to its superior electrical conductivity, large surface area, and mechanical strength. Among the many uses it can be put to, its use in photocatalysis and electrocatalysis holds significant promise, providing promising answers for long-term energy conversion, environmental cleanup, and enhanced material synthesis. Due to the special qualities and adaptable surface chemistry of graphene, the study of graphene-based materials for photocatalytic and electrocatalytic applications has attracted significant attention recently. While electrocatalysis uses electrical energy to speed up chemical transformations, photocatalysis uses light energy to fuel chemical reactions. In order to address issues like the production of clean energy, water purification, the reduction of air pollution, and the synthesis of chemicals with added value, both sectors have demonstrated great promise.1–6 In order to provide a thorough and organised overview of recent developments and successes in the photocatalytic and electrocatalytic applications of graphene-based materials, this review paper seeks to present a comprehensive and systematic analysis of those fields. The review will go over the many applications and features that have been investigated in this quickly developing subject. We will go into detail about the photocatalytic uses of graphene and its composites, concentrating on their functions in water splitting, hydrogen production from biomass, pollutant degradation, organic synthesis, energy storage, microplastics degradation, carbon dioxide (CO2) reduction, antibacterial disinfection, air purification, nitrogen fixation, and more. The review will also look at the electrocatalytic uses of graphene-based materials, including their contributions to fuel cells, the oxygen reduction reaction (ORR), the hydrogen evolution reaction (HER), the carbon dioxide (CO2) reduction reaction, the nitrogen (N2) reduction reaction, supercapacitors, the oxidation of organic pollutants, the oxygen evolution reaction (OER) in water splitting, the alcohol oxidation reaction, and more.7–16
Harnessing graphene's incredible properties, we explore how it is reshaping the world of green energy technology. From photocatalysis for pollution cleanup to electrocatalysis for clean energy production, graphene is leading the charge. But as we dive into these breakthroughs, we must also consider their potential impact on the environment. Graphene's power lies in its conductivity and surface chemistry, making it perfect for driving clean energy solutions like fuel cells and water splitting. However, its introduction into ecosystems raises concerns about toxicity and long-term effects. We need to tread carefully to ensure responsible innovation. This review delves into the environmental implications, benefits, and challenges of graphene-based technologies. By addressing potential issues, we pave the way for a greener, more sustainable future while protecting our planet.
2. Graphene and its forms
Fig. 1 presents graphene and its derivatives.17 Graphene is a planar sheet with single atom thickness consisting of sp2-hybridized atoms of carbon, and it has attained a valuable position in both science and engineering research since 2004.18 The 2D lattice structure has a honey comb appearance making graphene, though thinnest, the strongest material in the universe,19 with many surprising chemical as well as physical properties. The properties shown by pristine graphene include excellent thermal conductivity (5000 W m−1 K−1),20,21 ambipolar electric field effect, unconventional quantum Hall effect,22–25 room temperature carrier mobility (200
000 cm2 V−1 s−1),26,27 good electrical conductivity (2000 S m−1),28 good surface area (2630 m2 g−1),29 high environmental compatibility, good mechanical strength30 low production cost,31 and a very good adsorption tendency for organic and inorganic molecules.32–34Fig. 2 presents the honeycomb lattice of monolayer graphene.35
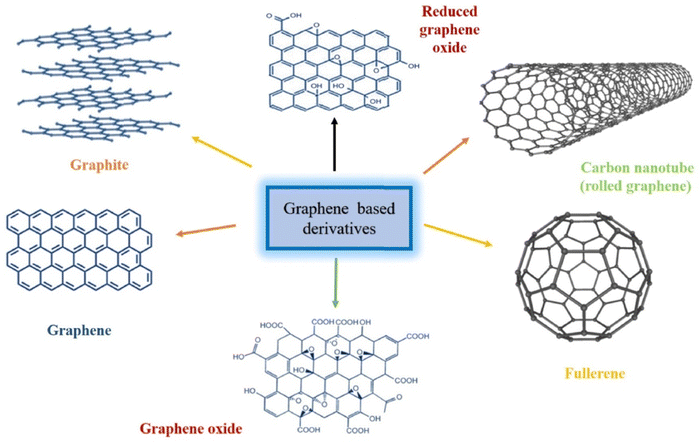 |
| Fig. 1 Graphene and its derivatives.17 | |
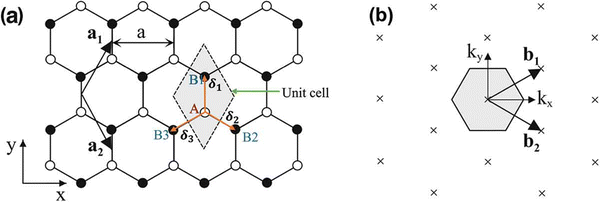 |
| Fig. 2 (a) Honeycomb lattice of monolayer graphene, where white (black) circles indicate carbon atoms on A (B) sites, and (b) the reciprocal lattice of monolayer graphene, where the shaded hexagon is the corresponding Brillouin zone.35 | |
Graphene offers a promising way for building up of hybrid graphene/semiconductor composites to be used as photocatalysts.36–39 Since Fujishima and Honda's discovery of photoelectrochemical (PEC) water splitting on TiO2 electrodes in 1972, heterogeneous photocatalysis has received international attention as a green and promising process.40
Most of the photocatalysts show little quantum yield, low visible light utility rate, and low stability, which are posing several challenges in the design and development of practically applicable heterogeneous photocatalysts.41
2.1. Pristine graphene: monolayer graphene film
Chemical vapour deposition (CVD) is regarded as a common method for monolayer graphene formation when needed in small quantities because of its high cost and low production yield. Moreover methods like chemical reduction, epitaxial growth, thermal exfoliation, and mechanical exfoliation are also employed for the preparation of graphene.42–46 The CVD process is carried out in a reaction assembly wherein the substrate material is placed; vaporous carbon atoms are accumulated at the substrate surface and the other gases are evolved out. Temperature plays an important role in the deposition process.43,44 The CVD method for formation of graphene is a process that is carried out in two phases.45,47 Paralysis is the initial step which allows the accumulation of carbon atoms on the substrate material surface. In the second phase, heat treatment is given to cause the separated carbon atoms to aggregate on the substrate surface, forming a single layer structure.48 Graphene films developed by the CVD method have many characteristics specified by layer thickness and other criteria.49 Copper in the form of CuO has been used as a common substrate material for development of graphene layers.50 In 2015, Yang et al. reported fabrication of a graphene film through a soft–hard template approach on a Si/SiO2 substrate as depicted in Fig. 3. To start, TMOS and H2O were combined and agitated to induce partial hydrolyzation of TMOS. Next, a solution of CTAB and pyrene was introduced, and the resulting mixture was stirred briefly before being evenly spread on a quartz or silicon substrate and left to dry naturally. Throughout this procedure, the hydrophobic pyrene demonstrated instability within the aqueous solution, preferring to integrate into the CTAB micelles as the malleable template. Simultaneously, non-covalent interactions, like ionic forces and hydrogen bonding, between the charged segment of CTAB and the freshly formed SiO2 facilitated the generation of SiO2 layers on both sides of the CTAB micelles, functioning as a solid template. Consequently, the combination of pre-hydrolyzed TMOS, CTAB, and pyrene in a three-part self-assembly culminated in the development of a SiO2/CTAB/pyrene composite resembling a layered sandwich structure.51
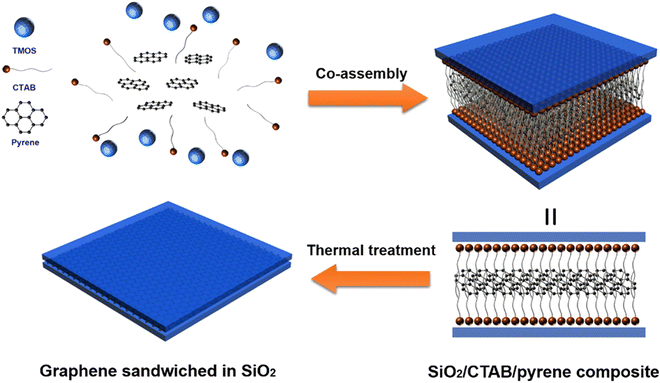 |
| Fig. 3 Synthesis of a graphene film through the soft–hard template pathway.51 | |
Pristine graphene's exceptional properties have paved the way for groundbreaking advancements in photocatalytic and electrocatalytic applications. Due to its superior electron mobility and large surface area, graphene excels in degrading pollutants and purifying water through photocatalysis. Simultaneously, its impressive conductivity and surface versatility make it a top contender for driving clean energy solutions, such as fuel cells and metal–air batteries, via electrocatalysis. However, ensuring its safe integration and addressing potential environmental impacts are key for fostering responsible and sustainable innovation.
2.2. Graphene oxide (GO)
The precursor of graphene oxide is graphite. Graphite is a 3D material which contains numerous graphene layers.52 Oxygen-carrying functional groups are adhered to the surface of graphite through the oxidation process, thus forming graphite oxide from graphite42,52 (Fig. 4).
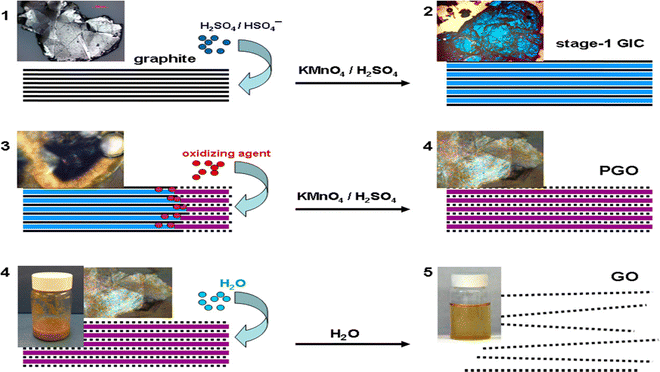 |
| Fig. 4 Bulk graphite conversion into GO with associated micrographic pictures or sample appearances at each stage. The three phases represent the development of the final GO product as well as the two intermediate products (stage-1 GIC or graphite intercalation compound, and PGO or pristine graphite oxide). The wide blue lines indicate the H2SO4/HSO4− intercalant; the wide purple lines represent a layer of a mixture of H2SO4/HSO4− intercalant with the reduced form of the oxidising agent. Graphene layers are represented by solid black lines. The black dotted lines indicate single layers of GO.53 | |
When graphite oxide is subjected to sonication, graphene oxide (GO) is obtained. The surface of graphene can be functionalized with different oxygenated functional groups.42,52,54 These functional groups increase the hydrophilicity of graphene layers, thus leading to the separation of graphene layers.42,54 Graphite oxide is multi-layered while graphene oxide is several-layered or single-layered.42,54,55 The selective functionalization of graphene oxide is carried out for the purpose of specific applications. GO shows greater solubility in natural fluids and polar solvents.42,56
Graphene oxide's exceptional properties are driving transformative advancements in both photocatalytic and electrocatalytic applications. Its unique structure and functional groups enable efficient pollutant degradation and water purification through photocatalysis. Additionally, its tunable surface chemistry makes it a promising catalyst for various clean energy technologies, including fuel cells and water splitting, under electrocatalysis. To ensure its sustainable integration, careful assessment of environmental implications and toxicity concerns is crucial for responsible and ethical utilization.
2.3. Reduced graphene oxide (rGO)
GO after undergoing a special reduction process forms reduced graphene oxide (rGO).56,57 The methods employed here are thermal reduction, chemical reduction, and electrochemical reduction.58 The chemical reduction method produces rGO which usually has higher surface area and thus higher electrical conductivity.58 The chemical reduction of GO on adding hydrazine is also a suitable method, but it causes small N-doping on graphene sheets throughout the reduction process.59,60 rGO is produced in large amounts in a short time when GO undergoes the thermal reduction process.57,58 The disadvantage with thermal reduction lies in the heat treatment which can destroy graphene structure because of the creation of a greater pressure of CO2. The yield reported so far is 25–35 percent.59 The imperfections and vacancies created in rGO also affect its mechanical strength. Several commercial methods for production of reduced graphene oxide nanoplatelets have also been reported.57–59 Reduced graphene oxide of good quality is produced when the electrochemical reduction method is used.61 In the electrochemical procedure, the substrates (mainly indium tin oxide or glass) are coated with a film of GO, and a current is passed through it. The rGO produced has a high carbon to oxygen ratio and has shown the same conductivity as that of silver.62 After rGO is formed, it is functionalized selectively for the desired applications. Fig. 5 shows some important methods for converting GO into rGO.63
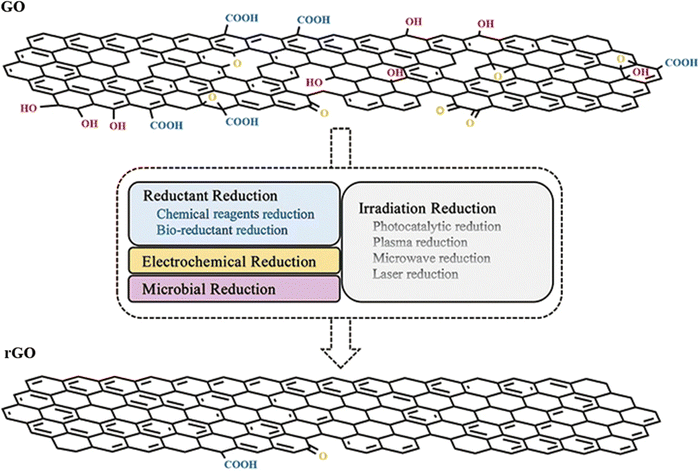 |
| Fig. 5 Synthetic routes to rGO from GO.63 Reproduced (adapted) with permission from ref. 63. Copyright [2020] [Springer Nature]. | |
In the field of electronic devices, while there has been extensive research on horizontal electron transport in graphene oxide (GO) sheets, studies on vertical electron transport are scarce. However, understanding vertical transport is crucial for precisely controlling the performance of GO-based devices. Previous research has focused on analyzing the GO structure using techniques such as high-resolution transmission electron microscopy (HR-TEM), scanning tunneling microscopy (STM), Raman spectroscopy, and carrier dynamics. Yet, these methods have limitations in capturing the complete chemical and electronic structure of single-layer GO. To address this, the authors employed conductive atomic force microscopy (C-AFM) to simultaneously visualize the mechanical and electrical properties of single-layer GO, revealing distinct domain structures determined by the prevalence of sp2- or sp3-carbon regions. The study emphasizes the heterogeneous conductivity of single-layer GO at the nanoscale, highlighting the significance of the chemical composition, particularly oxygen density, in determining its properties. Additionally, the local current–voltage (I–V) characteristics in the vertical direction of the GO sheet showcase differing conductance values for regions rich in sp2 and sp3 carbon. The utilization of friction, conductance mapping, and scanning transmission X-ray microscopy (STXM) further confirmed the presence of GO domains with varying sp2 to sp3 ratios at a submicron scale. Fig. 6 presents the direct observation of domain structures on single-layer GO by simultaneously mapping the mechanical and electrical properties using C-AFM.64
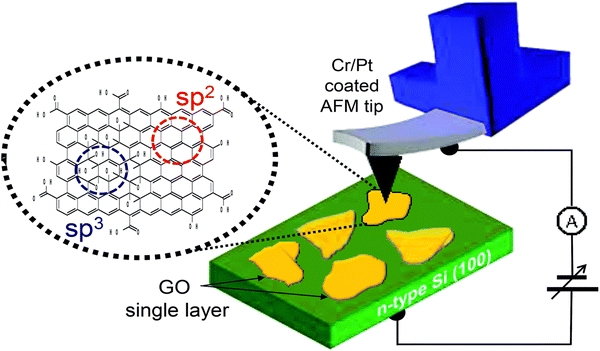 |
| Fig. 6 Scheme for probing the electrical characterization of single-layer GO on SiO2/n-Si that is based on the Lerf–Klinowski model using conductive AFM. The red dotted circle indicates an aromatic region (sp2 carbon) and the blue dotted circle shows an oxygenated aliphatic region (sp3 carbon).64 Reproduced (adapted) with permission from ref. 64. Copyright [2016] [RSC]. | |
3. Photocatalytic applications of graphene-based materials
3.1. Water splitting and hydrogen production
Photocatalytic water splitting using solar energy and semiconductors has attracted considerable attention as a promising approach to address solar energy storage and achieve cost-effective, environmentally friendly H2 fuel production.65 However, when the Gibbs free energy shift is considered, it is clear that photocatalytic water splitting, which is thermodynamically uphill, presents larger hurdles than downhill photocatalytic degradation events, such as organic compound oxidation using oxygen molecules. Since the pioneering work of Honda and Fujishima in 1972, various heterogeneous photocatalysts have been widely utilized in the photocatalytic hydrogen production from water reduction66–70 for metal oxides,71–75 for sulfides,76–78 for (oxy) nitrides,79–82 for organic dyes,83–88 for metal-free C3N4, and89,90 for SiC. In recent years, graphene has been extensively integrated into composite photocatalysts for both half-reaction water splitting (H2 and O2) and overall water splitting systems to enhance their photocatalytic activity. The primary focus of this article will be to explore the utilization of graphene in these two systems.
3.1.1. Half-reaction water splitting for hydrogen evolution.
Metal sulfides in the form of semiconductors have emerged as the catalysts with the most potential for photocatalysis aided water splitting, mainly due to their higher H2-evolution efficiencies in the presence of a reagent under solar light illumination.91–96 It is widely acknowledged that incorporating graphene as a H2-evolution assisting co-catalyst can significantly enhance the photocatalytic efficiency of metal sulfides.97 For example, researchers demonstrated a 4.5-fold increase in the photocatalytic H2-evolution rate by modifying Zn0.8Cd0.2S with 0.25 wt% reduced graphene oxide (rGO) in the form of a co-catalyst (Fig. 7A), surpassing even the performance of 1 wt% Pt-loaded Zn0.8Cd0.2S (Fig. 7B).98 The introduction of rGO as a co-catalyst is believed to facilitate the efficient transfer and separation of charge carriers, resulting in an increased number of surface activity centres for reducing water, thereby substantially enhancing the H2-evolution rate (Fig. 7C). Furthermore, the introduction of NiS into the binary ZnxCd1−xS/rGO nanocomposites led to the formation of ternary hybrids which exhibited a significantly improved photocatalytic H2-production in the presence of sunlight which is attributed to the mutual synergetic effect between rGO and NiS in comparison to ZnxCd1−xS. The establishment of a p–n junction in between NiS and CdS specifically facilitated the migration of holes as charge carriers from CdS to NiS, effectively curbing the acute CdS photocorrosion. These findings suggest that formation of multi-junction photocatalysts from 0D–2D hybrids holds promise for enhancing the photocatalytic efficiency of metal sulfides.99–101 Moreover, the insufficiency and high cost of noble metal co-catalysts of H2-evolution have limited their working applications in water reduction under solar light irradiation. As a result, researchers have turned their attention to the development and utilization of earth rich metal-based co-catalysts for H2 evolution, such as Mo, Co, Ni, and Cu-based co-catalysts. The incorporation of specialized earth-rich co-catalysts on to graphene to form co-catalysts in the form of a composite shows great promise as a direction to create highly efficient H2-evolution co-catalysts for nanoscale metal sulfides, warranting further investigation in future studies.102–107
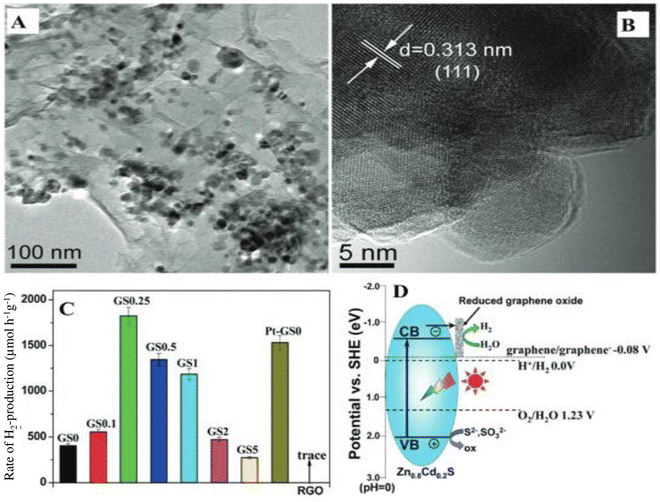 |
| Fig. 7 (A) and (B) present the combination of NiS/Zn0.5Cd0.5S/rGO TEM images. (C) Comparison of photocatalytic H2-production activity for the Zn0.5Cd0.5S, NiS/Zn0.5Cd0.5S, Zn0.5Cd0.5S/rGO, and NiS/Zn0.5Cd0.5S/rGO samples under simulated solar irradiation. (D) A schematic illustration of the charge transfer and separation in the NiS/Zn0.5Cd0.5S/rGO ternary composite.105 Reproduced (adapted) with permission from ref. 105. Copyright [2016] [Wiley]. | |
3.1.2. Overall water splitting.
Due to the intricacy of the four-electron O2-evolution half reaction, it is generally recognized that a more challenging process than photocatalytic H2-evolution is water splitting. In order to create a rather weak oxygen–oxygen bond, this reaction includes the two water molecules that are oxidized with four electrons and the elimination of four protons.108 Remarkably, graphene has demonstrated the ability to facilitate photocatalytic overall water splitting in certain cases. N-doped GO quantum dots (NGO-QDs), made from graphene oxide sheets, were shown to be capable of photocatalytic overall water splitting when exposed to visible light by Yeh et al.109 NGO-QDs had a band gap of 2.2 eV, which allowed them to absorb visible light and produce exaction. With the aid of oxygen functionalities grafted onto the graphene surface, the NGO-QD construction led to the creation of p–n type photochemical diodes, where n-conductivity was induced by nitrogen atoms embedded in the graphene frame and p-conductivity by the oxygen functionalities. Thus, the production of H2 and O2 gases was controlled by the p- and n-domains, respectively. Only H2 evolution was catalysed by nitrogen-free QDs with p-type conductivity when exposed to radiation, proving that electron injection was more advantageous due to band bending in the p-type domains. To increase the rate of H2 and O2 evolution, NGO-QDs should be further optimized.
A photocatalyst using the Z-scheme that uses a solid-state electron mediator to split water has been discovered to function like graphene more recently owing to the material's excellent electrical conductivity. In a Z-scheme water-splitting system with Ru/SrTiO3:Rh and BiVO4 as the H2 and O2 evolution photocatalysts, respectively, Iwase et al.110 showed, for instance, that rGO served as a solid-state electron mediator, boosting the photocatalytic activity. rGO was able to transmit photo-generated electrons from BiVO4's conduction band to Ru/SrTiO3:Rh when illuminated by visible light. As a result, a full cycle of Z-scheme photocatalytic water splitting was accomplished by the co-catalyst Rh, which used the excited electrons in Ru/SrTiO3:Rh to catalyse the reduction of water to H2, and the holes left in the valence band of BiVO4 could oxidize water to O2. It was discovered that the Z-scheme system's electron transfer was greatly influenced by the degree of GO's reduction and hydrophobicity as an electron mediator. When compared to the system without rGO ((Ru/SrTiO3:Rh)–BiVO4), the (Ru/SrTiO3:Rh)–(RGO/BiVO4) system demonstrated a significantly greater rate of photocatalytic H2-production. This was accomplished by establishing the ideal balance of GO reduction and a high degree of GO hydrophilicity. Recently, Kudo and colleagues111 showed that p-type metal sulphide photocatalysts with a p-type semiconductor character as an H2-evolving photocatalyst and the rGO–TiO2 composite as an O2-evolving photocatalyst might be combined to produce Z-schematic total water splitting in a stoichiometric amount. But in this system, the high band gap of TiO2 prevented the photocatalytic efficiency from being improved. The more promising visible-light semiconductors like BiVO4 should take the place of TiO2 to fully use the solar spectrum.
3.1.3. Photoelectrochemical water splitting.
Efforts towards transforming sustainable solar energy into storable chemical fuels have attracted considerable attention. Among various technologies, there is a significant research focus on photoelectrochemical (PEC) water splitting, a process capable of producing environmentally friendly hydrogen fuel. This method is currently under intense investigation. Given that PEC water splitting shares a similar operational principle with photovoltaic devices, an effective photovoltaic material is considered an ideal candidate for serving as a photoelectrode in PEC solar water splitting. The study revolves around the crucial issue of maintaining the stability of catalysts within photoelectrochemical (PEC) cells. Specifically, the focus is on investigating the impact of chemically reduced graphene oxide (rGO) layers on the performance and longevity of Cu(In,Ga)Se2 (CIGS) photocathodes. The method employed for the study involved the application of rGO onto a CIGS/CdS heterojunction using a simple spray coating technique, followed by the deposition of Pt catalysts. The integration of rGO demonstrated a notable enhancement in the current–potential characteristics of the system. This improvement was attributed to the effective transfer of charges, which subsequently led to the suppression of charge recombination. Of significant importance was the substantial increase in the stability of the CIGS/CdS/rGO/Pt setup compared to the traditional CIGS/CdS/Pt configuration. This increase was evidenced by the preservation of the Pt catalysts over an extended period of time, indicating the effectiveness of the rGO layer in safeguarding the catalysts. Furthermore, cyclic voltammetry (CV) measurements revealed that the introduction of rGO resulted in heightened charge transfer rates, with the concentration of rGO playing a key role in this enhancement. This finding highlights the multifaceted role of rGO within PEC cells, acting as an adhesive interlayer for catalysts, a protective barrier for the underlying absorber stacks, and a facilitator for efficient electron transport to the catalysts. Overall, the research emphasizes the potential of incorporating rGO in PEC systems as a pivotal means of improving their stability and performance, thereby contributing to the advancement of sustainable solar energy conversion technologies (Fig. 8).112
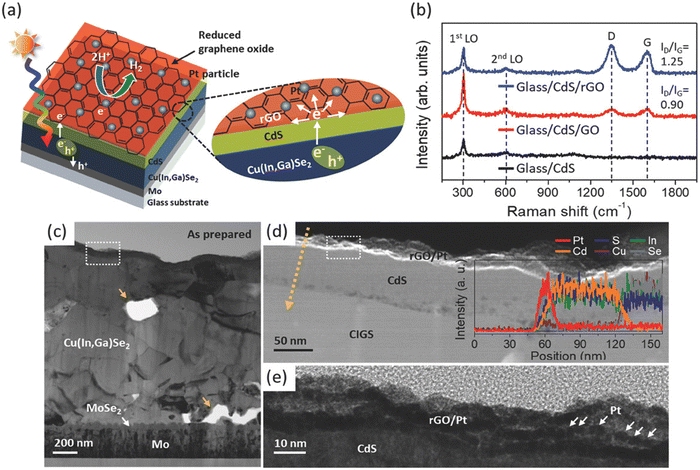 |
| Fig. 8 (a) Schematic illustration of the CIGS/CdS/rGO/Pt photocathode for hydrogen evolution reaction (illustration is not to scale). (b) Raman spectra of CdS, GO, and rGO using a 514 nm excitation laser. 1st and 2nd longitudinal optical modes present phonon vibrational modes of CdS. (c) Cross-sectional TEM image of an as-prepared glass/Mo/MoSe2/CIGS/CdS/rGO−0.25/Pt photocathode. The orange arrows mark voids formed in the CIGS absorber. (d) HAADF image (from the dotted box in (c)) presenting clear interfaces between CIGS, CdS, and rGO−0.25/Pt. The inset is a line scan of elemental distributions along the yellow dotted arrow. (e) High resolution TEM image (from the dotted box in (d)) showing the distribution of Pt nanoparticles on CdS. The white arrows indicate the formation of discrete Pt nanoparticles.112 Reproduced (adapted) with permission from ref. 112. Copyright [2018] [Wiley]. | |
3.2. CO2 reduction
In recent times, the rapid increase in anthropogenic carbon dioxide leading to global warming and fossil fuel shortages has emerged as a significant global challenge. To address this issue sustainably, converting CO2 into valuable energy-bearing compounds (e.g., CO, methane, and methanol) using sunlight as an energy source has become a promising solution. The use of effective and practical semiconductors for CO2 reduction with water has advanced significantly over the past 20 years since the initial affirmation of photoactivated catalytic CO2 reduction by Inoue and coworkers in 1979.113–115
Among these semiconductor materials, the use of reduced graphene oxide (rGO)/graphene oxide (GO) and semiconductor combinations has gained popularity as a strategy to enhance the activity of semiconductors for photocatalytic CO2 transformation to important hydrocarbons due to their excellent stability, low cost, and non-toxic nature.116–120 The optimal content of graphene in composite photocatalysts is crucial for boosting the efficiency of CO2 photoreduction since an excessive amount of graphene can lead to light shielding, ultimately reducing the activity of CO2 photoreduction. For example, the optimized rGO-CdS nanorod composite photocatalyst (Fig. 9A) produced 2.51 mol g−1 h−1 of CH4 at an rGO content of 0.5 wt% (as shown in Fig. 9B). This rate was ten times higher than that of pure CdS nanorods due to the promoted charge separation and it even outperformed an optimized Pt-CdS nanorod photocatalyst under identical reaction conditions (Fig. 9C).121 Similar results were obtained for the photocatalytic conversion of CO2 into CO, where the optimized Cu2O/0.5% rGO composites had the best activity because of the reduced electron–hole recombination, efficient charge transfer, and protective properties of rGO.122–124 Carbon dioxide (CO2) photoreduction activity is greatly increased when graphene is used because it can increase the electron conductivity and quicken the flow of electrons to the reaction's active sites. It has been demonstrated that the intimate contact interface created by in situ growth effectively increases the photocatalytic activity for the selective transformation of CO2 to C2H6.125
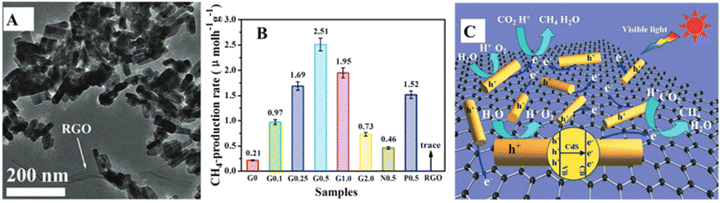 |
| Fig. 9 (A) TEM images of the G0.5 sample. (B) A comparison of the photocatalytic CH4-production rate of different samples under visible-light irradiation (Gx, where x represents the weight percentage of rGO, ranging from 0% to 2.0%). (C) A schematic illustration of the charge transfer and separation in the rGO-CdS nanorod system under visible-light illumination.115 Reproduced (adapted) with permission from ref. 115. Copyright [2013] [RSC]. | |
Moreover, the fabrication of 2D layered composite photocatalysts can further improve the selective photoreduction of CO2. With a face-to-face configuration, graphene has a larger semiconductor surface area, which favours charge separation and increases CO2 photoreduction activity. For instance, stable hollow spheres made of Ti0.91O2 and graphene nanosheets showed CO and CH4 generation rates that were around 5 times higher than those of TiO2 alone.126 Similarly, due to the more advantageous charge transfer from TiO2 nanosheets to rGO at the close 2D–2D interfaces, rGO/TiO2 nanosheet films had significantly better activity for selective CH4 synthesis from CO2 photoreduction than SWCNT/TiO2 nanosheet films under UV illumination.127
Additionally, by connecting with GO as a support, 2D layered double hydroxides (LDHs) might show greatly enhanced efficiency due to the improved CO2 uptake capacity and multicycle stability of the assembly.128–130 To increase the activity and selectivity of CO2 photoreduction, it is therefore necessary to investigate the possibility of mixing Earth-abundant substances with graphene as co-catalysts (such as grafted amine groups, LDHs, MgO, and NaOH).131–140
3.3. Hydrogen production from biomass
In the pursuit of cleaner fuels, molecular hydrogen gas (dihydrogen, H2) is regarded as a highly promising energy carrier,141–143 particularly when obtained from renewable sources. Integrating the significance of hydrogen in fuel cells for clean energy generation with the utilization of renewable feedstocks holds immense potential. However, non-renewable fossil fuels are currently used to manufacture the majority of H2.144 Through a variety of chemical procedures,145–148 which may be broadly divided into two classes: (i) direct gasification and (ii) indirect transformation of biomass into platform chemicals followed by reforming, hydrogen can be produced from biomass as a practical substitute. Fig. 10 schematically depicts these different routes. H2 and CO are the main products of direct gasification of raw lignocellulosic materials.145–151 The indirect transformation method, on the other hand, uses a variety of pathways, with the first stage depending on either pyrolysis or hydrolysis, producing monomeric (or oligomeric) oxygenated molecules that are then reformed into a gaseous stream rich in H2 and CO2.144,145,147,148,151–153 As previously indicated, gasification entails the one-step conversion of biomass at high temperatures (usually 750–1000 °C) with oxygen and/or steam. The biomass can either be raw or minimally processed through mechanical means. A mixture containing H2, CO, and various concentrations of CO2, CH4, other gaseous chemicals, and solid by-products such as char are produced by this process.144–150 Gasification is a flexible technology that can be used with a variety of biomass feedstocks, but it is constrained by the difficult operating conditions.
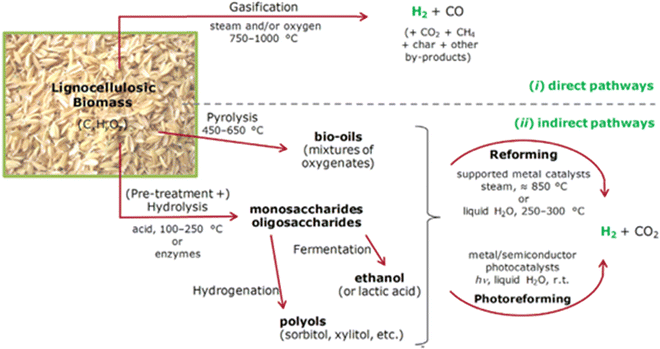 |
| Fig. 10 As shown, there are two main pathways that can be used to produce hydrogen from lignocellulosic biomass: (i) direct gasification pathways and (ii) indirect methods based on the reforming of oxygenated derivatives.151 Reproduced (adapted) with permission from ref. 151. Copyright [2022] [Elsevier]. | |
The initial biomass undergoes processing in the first stage to generate liquid streams in the second series of transformations, known as the indirect routes. The high molecular weight, cross-linked biopolymers (cellulose, hemicelluloses, and lignin) are broken down into mixtures of oxygenated, lower molecular weight chemicals throughout this process. The two basic techniques employed in this situation are pyrolysis and hydrolysis.145,146,149,150 Pyrolysis is one method for producing bio-oils, which are an intricate mixture of oxygenated materials, while hydrolysis is a different method145,146,149,150 Under a variety of process settings, bio-oils or specific bio-oil fractions can be reformed in the presence of water (either in liquid form or as steam), producing H2-rich gaseous streams. With supported nickel or noble metal catalysts, this process is normally carried out at high temperatures (about 850 °C) and brief residence durations.154–156 An alternative approach uses liquid aqueous phase reforming at cooler temperatures (250–350 °C).152 High temperatures, though, may cause non-selective reactions in both situations. Saccharides are prone to disintegration via a number of mechanisms, including dehydration or polymerization, and many compounds containing functional groups, such as carbonyls or carboxylic acids, may experience a range of degradation processes (e.g., condensation or cyclization). The creation of carbonaceous deposits that cause catalyst deactivation, the production of large amounts of CO, and the pyrolysis of saccharides even before they hit the catalyst bed are therefore some frequent difficulties in the thermocatalytic reforming of bio-oils.154–156 However, one of the most dependable methods for valorizing such unstable and complicated biomass products continues to be reforming of pyrolysis oils for H2 generation. The aqueous phase reforming of various oxygenated substrates produced from lignocellulose hydrolysis and subsequent transformations is another key indirect mechanism for biomass-to-hydrogen conversion (Fig. 10).151 This approach is supported by earlier research on the catalytic aqueous phase reforming of several model oxygenates.157,158 The process selectivity can be changed depending on the catalyst utilised. For instance, Pt, Pd, or Ni–Sn catalysts, preferably supported on Al2O3, favour H2 generation while minimising the generation of alkanes.153 These catalysts have significant C–C scission, dehydrogenation, and water–gas shift activity while having low C–O scission or methanation activity. In conclusion, promising pathways in the biomass-to-hydrogen scenario involve two-stage processes that involve the conversion of lignocellulosic biomass into light oxygenates, followed by the aqueous phase reforming of these oxygenates to produce H2. These processes are carried out under significantly milder conditions than one-stage treatments like gasification. However, thermocatalytic processes can result in degradation or side reactions that have a negative impact on H2 selectivity (50–60% of the theoretical maximum) and generate a significant amount of alkanes.151,153 They are also energy-intensive, frequently requiring high temperatures and pressurized systems. As a result, continuous work is still required to optimise the reforming process eqn (1) without restricting other transformations, and any advancement in this area would undoubtedly be advantageous to biomass-to-hydrogen technology.
| CxHyOz + (2x − z)H2O → (2x + y/2 − z)H2 + xCO2 | (1) |
3.4. Organic pollutant removal
While graphene has been extensively employed in conjunction with different semiconducting materials to explore their photocatalytic capabilities, the present discussion will concentrate specifically on TiO2 and ZnO owing to their well-suited band gap energies, matched conduction band (CB) and valence band (VB) levels that enable redox reactions, and their potential for commercial applications. Moreover, the possibility of integrating graphene with LDH (layered double hydroxide) is emphasized as a promising area for future investigation in the domain of photocatalysis due to its growing research prospects.
3.4.1. Graphene/TiO2 photocatalysts.
TiO2 is a highly promising photocatalyst that has been extensively studied for water purification due to its strong oxidizing abilities, chemical stability, cost-effectiveness, and non-toxic nature. However, its large band gap (3.2 eV) restricts its activation to only UV radiation, making it less cost-effective for utilizing solar light, which consists of both UV and visible light. Another limitation of TiO2 is the rapid recombination of charge carriers, which hampers its photocatalytic efficiency. To overcome these challenges and enhance its photocatalytic ability, researchers have explored various strategies, including coupling TiO2 with other semiconductors, doping it with metal or non-metal ions, and creating composites with graphene.
The combination of graphene and TiO2 has been found to significantly improve the photo-degradation ability. Graphene enhances the specific surface area and captures photo-induced electrons and holes in TiO2, thereby increasing the conduction current. Numerous studies have demonstrated the enhanced photocatalytic activity of graphene–TiO2 composites, highlighting their potential for diverse applications.
Introducing metals (Pt, Au, Ag) through physical or chemical methods has also been investigated to enhance TiO2's photocatalytic activity. For instance, modification with silver nanoparticles has been found to improve excitation and electron transfer, leading to better photo-degradation of dyes. Additionally, the size and reduction degree of graphene in the composites play a crucial role in their photocatalytic performance. Medium-sized graphene platelets favor charge transfer and light absorption, resulting in higher pollutant degradation rates.
Naturally occurring polymers like chitosan have been studied to improve adsorption during the photocatalytic process. Composite materials of TiO2/chitosan/graphene have shown enhanced photocatalytic capabilities due to the uniform distribution of their lamellar pore structure.
Various synthesis techniques and materials have been employed to produce graphene–TiO2 composites with low defect levels and high photocatalytic performance. For instance, graphite intercalation compounds have been used as an alternative source for graphene production, leading to composites with enhanced stability in photocatalytic reactions.
Overall, recent advances in graphene-based TiO2 composites have demonstrated significant improvements in photocatalytic degradation of organic pollutants, especially dyes. The incorporation of different metals, magnetic oxides, and novel materials, as well as the use of various synthesis techniques, has contributed to enhancing the photocatalytic efficiency of these composites.159–184Fig. 11 presents SEM images and other data for TiO2 based catalysts and their individual materials.166Fig. 12 and 13 present the mechanism of degradation of RhB by Ag–TiO2/rGO166 and the mechanism of MB degradation under (a) UV and (b) solar irradiation.180
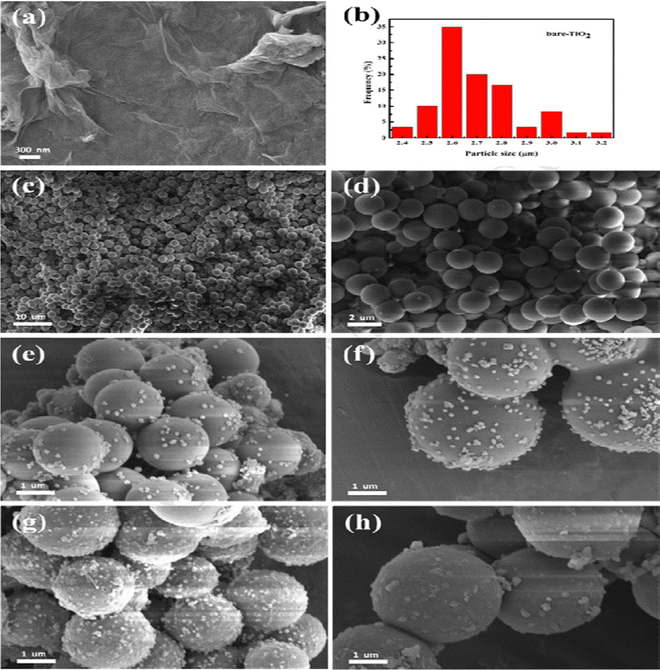 |
| Fig. 11 SEM images of (a) GO, (b) and (c) bare-TiO2, (d) bare TiO2 magnified, (e) 1% Ag–TiO2, (f) 2% Ag–TiO2, (g) 3% Ag–TiO2, and (h) 2% Ag–TiO2/rGO.166 Reproduced (adapted) with permission from ref. 166. Copyright [2019] [Elsevier]. | |
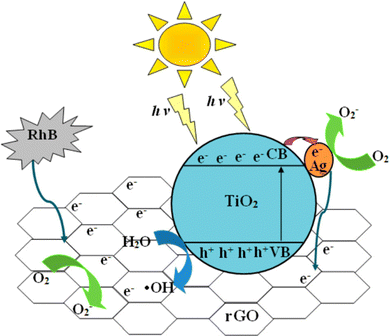 |
| Fig. 12 Mechanism of degradation of RhB by Ag–TiO2/rGO.166 Reproduced (adapted) with permission from ref. 166. Copyright [2019] [Elsevier]. | |
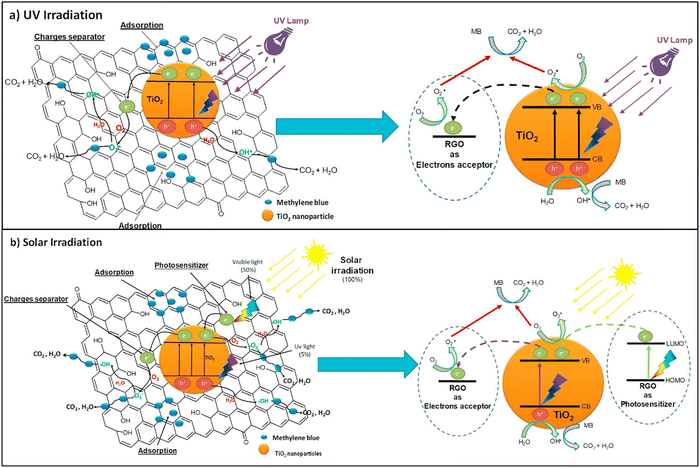 |
| Fig. 13 Mechanism of MB degradation under (a) UV and (b) solar irradiation.180 Reproduced (adapted) with permission from ref. 180. Copyright [2019] [Elsevier]. | |
3.4.2. Graphene/ZnO photocatalysts.
Because of its favourable optical, mechanical, electrical, anti-fouling, and anti-bacterial characteristics, ZnO is an n-type semiconductor with remarkable potential as a photocatalyst for wastewater treatment.159,185,186 However, due to its high band gap (3.22 eV) and quick charge carrier recombination rate, its applicability under visible light for wastewater treatment is restricted. Researchers have investigated several techniques to solve these issues, and among them, ZnO/graphene composites have demonstrated outstanding potential with increased photocatalytic activity.159,185,186
In research studies, several types of ZnO have been examined, including zero-dimensional, one-dimensional (1D), two-dimensional (2D), and three-dimensional (3D) structures. The effectiveness of dye degradation is determined by various parameters, the most important of which is the adsorption of dye molecules on the photocatalyst surface. Graphene derivatives act as catalyst supports, and their huge surface area considerably improves pollutant adsorption capabilities. Furthermore, graphene's high conductivity aids electron transport to the surface, preventing recombination with holes in the valence band (VB), where oxidative interactions with pollutant molecules occur.159,185–189
Various synthesis methods, including hydrothermal, photochemical, sonochemical, sol–gel, and microwave-assisted, have been employed for creating ZnO/graphene composites.190–196 The choice of synthesis method impacts the composite's photocatalytic ability, as certain methods result in enhanced performance due to better dispersion of ZnO nanorods on 3D graphene sheets or larger surface area.197,198
Recent research has focused on developing sunlight-driven photocatalysts, with the widespread adoption of doping metal atoms (Cu, Ag, Au, Pt, Pd) on ZnO and graphene composites.193,194,199–201 Ternary composites, such as Mn-doped ZnO/rGO, have exhibited high efficiency in degrading dyes under solar irradiation, attributed to the trapping ability of Mn2+ and the presence of crystal defects and a narrow band gap.202 Combining ZnO nanorods with a graphene hydrogel and Ag nanoparticles has led to enhanced photocatalytic degradation under both UV and visible light.203 The surface plasmon resonance effect of Ag nanoparticles, as well as the support of the 3D graphene hydrogel, contributed to better pollutant adsorption and charge carrier separation.203 Other studies have investigated the effect of various morphologies of ZnO (nanodisks, nanorods, and nanospheres) anchored on GO, with the ZnO nanospheres/GO composite demonstrating the best organic pollutant degradation efficiency. Multiple cycle stability experiments have yielded encouraging results.204,205
In conclusion, ZnO/graphene composites hold great promise as efficient photocatalysts for the degradation of organic pollutants. Various synthesis methods and morphological features of ZnO play significant roles in their photocatalytic performance. The continuous development of sunlight-driven photocatalysts and innovative composite structures is advancing the field of wastewater treatment. Fig. 14 presents the degradation mechanism of dyes with Mn doped ZnO/rGO.201Fig. 15 shows SEM and TEM images of the ZnO/rGO composite203 and Fig. 16 shows a schematic illustration of preparation of rZG (ZnO nanorods and GO), dZG (ZnO nanodisks and GO) and sZG (ZnO nanospheres and GO).205
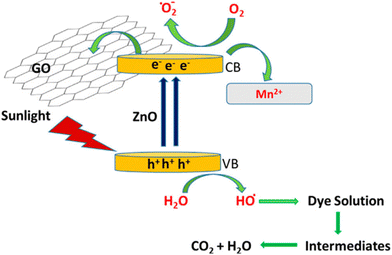 |
| Fig. 14 Degradation mechanism of dyes with Mn doped ZnO/rGO.201 Reproduced (adapted) with permission from ref. 201. Copyright [2018] [Elsevier]. | |
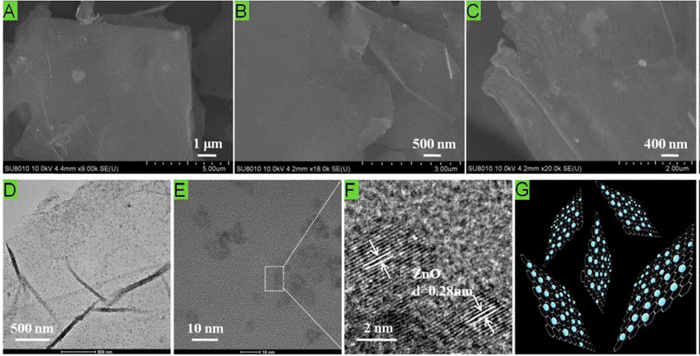 |
| Fig. 15 (A)–(G) SEM and TEM images of some photochemically active important ZnO/rGO composites.203 Reproduced (adapted) with permission from ref. 203. Copyright [2018] [Elsevier]. | |
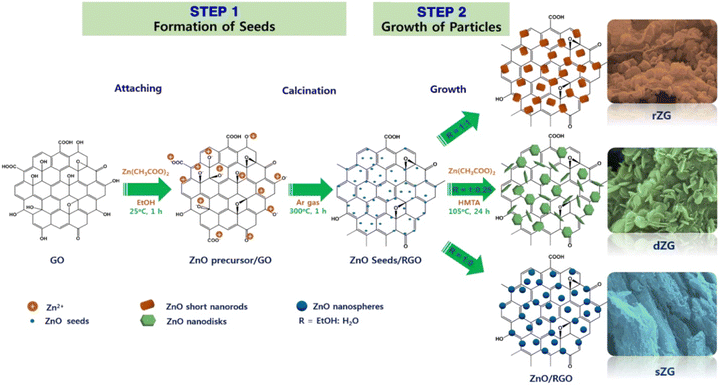 |
| Fig. 16 Schematic illustration of preparation of rZG (ZnO nanorods and GO), dZG (ZnO nanodisks and GO) and sZG (ZnO nanospheres and GO).205 Reproduced (adapted) with permission from ref. 205. Copyright [2019] [Elsevier]. | |
3.4.3. Graphene with LDH.
In the past decade, researchers have actively explored high-efficiency photocatalysts, resulting in the development of nano photocatalysts, benefiting from advances in nanotechnology and nano-science. Among these materials, LDH (layered double hydroxide) has emerged as a promising candidate due to its flexible composition and unique structure.206–208 Numerous studies have demonstrated the excellent efficiency of LDH in pollutant removal from water.209,210 However, two functional challenges have limited its large-scale application: (1) recombination of electron–hole pairs and (2) aggregation of nanoplates, leading to reduced photocatalytic activity.211–213
To address these issues, researchers have investigated metal doping of LDH with elements like Tb, Fe, Ce, and Ni, among others.214–217 Fe, in particular, has shown promise due to its electronic configuration, low cost, and non-toxic nature.218,219 While metal doping helps tackle the issue of electron–hole recombination, the problem of LDH nanoplate aggregation remains unresolved. To overcome these challenges, coupling LDH with graphene has emerged as a viable solution. Graphene offers ideal nanoplates with a large specific surface area and superior electron charge mobility. Various graphene-based hybrid nanocomposites that display enhanced photocatalytic activity have been reported.220–223 Some of these nanocomposites were produced through time-consuming and complex processes, such as electrostatic self-assembly or co-precipitation.
Another study by Z. Wang et al.223 introduces a groundbreaking approach for the synthesis of a NiFe layered double hydroxide/reduced graphene oxide (NiFe-LDH/RGO) composite. The process, carried out through a facile one-pot solvothermal method, effectively utilizes LDH as a separator. Notably, the subsequent deposition of Pt nanoparticles was achieved via electrodeposition. A comprehensive depiction of the synthetic pathway and the preparation process is provided in Fig. 17. The integration of NiFe-LDH with RGO not only created a robust composite but also facilitated the creation of additional active sites for Pt nanoparticles, leading to improved binding capabilities. This binding enhancement resulted in a more uniform distribution of Pt nanoparticles on the graphene nanosheets, effectively preventing their aggregation during methanol oxidation.
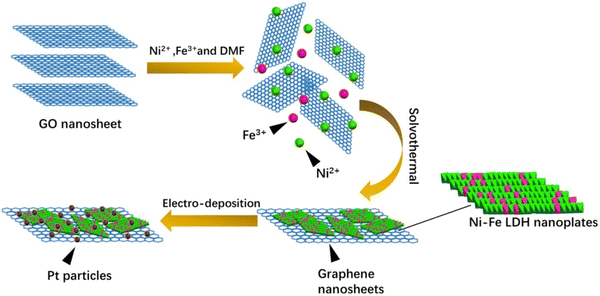 |
| Fig. 17 Illustration of the synthetic procedure for the Pt/NiFe-LDH/RGO nanocatalysts.223 Reproduced (adapted) with permission from ref. 223. Copyright [2018] [Elsevier]. | |
Kuen et al.224 used a simple one-step hydrothermal process to create CoAl-LDH/g-C3N4/rGO ternary heterojunctions with a new 2D/2D/2D layout. Under visible light irradiation, this photocatalyst dramatically improved its effectiveness in degrading Congo Red dye (CR) and tetracycline antibiotic (TC) contaminants in water. Due to their unique 2D/2D/2D structure, the ternary heterojunctions (LDH/CN/rGO) exhibited prolonged visible light absorption while preventing direct recombination of photoinduced electron–hole pairs. The photocatalytic performance of LDH/CN/rGO-15, with 15% LDH and 1% rGO on CN, was the best, destroying about 99% of CR within 30 minutes of light illumination. Furthermore, even after five consecutive deterioration cycles, these ternary heterojunctions maintained good stability with no substantial decrease in performance. Fig. 18 shows photocatalytic performance and TOC removal (%) over the LCR-15 photocatalyst in the degradation of CR and TC and photocatalytic mechanism for the decomposition of CR and TC pollutants over the LDH/CN/RGO ternary heterojunction system.224
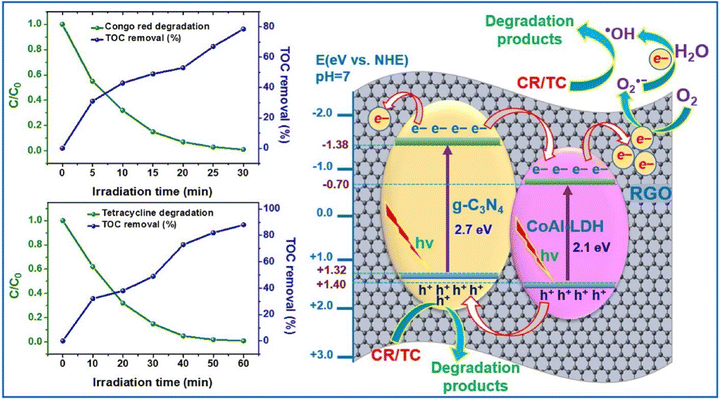 |
| Fig. 18 Photocatalytic performance and TOC removal (%) over the LCR-15 photocatalyst in the degradation of CR and TC and photocatalytic mechanism for the decomposition of CR and TC pollutants over the LDH/CN/RGO ternary heterojunction system.224 Reproduced (adapted) with permission from ref. 224. Copyright [2019] [Elsevier]. | |
In conclusion, LDH coupled with graphene and other metal elements has shown great potential in enhancing photocatalytic performance, addressing recombination and aggregation issues. These novel nano photocatalysts offer promising solutions for pollutant removal and water purification.207–226
3.5. Photocatalytic organic transformations
Selective organic transformation reactions are being conducted using photocatalytic processes. In these processes, photo-induced charge carriers play a crucial role in facilitating redox reactions that lead to the production of various oxidized and reduced products. Specifically, organic reactants can undergo oxidation by accepting holes or reduction by accepting electrons. This allows for the performance of both oxidation and reduction reactions.227 To enhance electron transport and promote rapid charge separation, graphene is employed as a support matrix. As a result, the photoactivity of semiconductors involved in selective oxidation and reduction processes is significantly increased. Detailed discussions and examples regarding this matter will follow in the subsequent sections. A schematic representation of photocatalytic organic transformation involving both oxidation and reduction processes is given in Fig. 19.
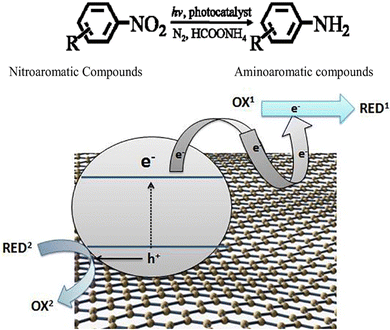 |
| Fig. 19 Selective organic transformations utilizing graphene-based semiconductor photocatalysts [OX1: oxidised reactants, RED1: reduced organic products, RED2: reduced organic reactants, OX2: oxidised products].228 Reproduced (adapted) with permission from ref. 228. Copyright [2021] [Elsevier]. | |
3.5.1. Selective reduction process.
Nitroaromatic molecules can be converted into the corresponding amino compounds by a selective photocatalytic process. For this purpose, Liu et al. conducted research in which they synthesized CdS nanowires on rGO sheets. As a hole scavenger, ammonium formate was used to create composites with increased photoactivity for the selective reduction of nitroaromatic compounds.229 Chang et al. conducted more research on the selective reduction process by creating a number of graphene/ZnO/Au nanocomposites by a straightforward hydrothermal technique.230 ZnO in the composite surface produced electron–hole pairs during photoexcitation when exposed to UV light. Due to their smaller work functions, graphene and Au nanorods both experienced rapid electron transport from ZnO to graphene. The reduction of charge carrier recombination caused by this effective electron transfer boosted the photocatalytic activity.231
3.5.2. Selective oxidation process.
To demonstrate the selective photocatalytic oxidation of benzyl alcohol into aldehydes and acids, Zhang et al. created CdS/graphene composites with various quantities of graphene in their study.232 Additionally, the effectiveness of these semiconductor composites was examined by the researchers. An appropriate charge transfer mechanism was used, and this significantly increased the photocatalytic activity. It was found that the photocatalytic efficacy of GR/CdS composites was effectively increased by the addition of a suitable amount of graphene.
In light of the rapid advancement in materials science, there has been a substantial increase in the use and requirement of catalytic organic synthesis. Photocatalysis has found application in the oxidation of diverse organic compounds, encompassing alcohols, hydrocarbons, aromatics, and amines. This approach exhibits distinct characteristics when compared to conventional methods, notably operating under mild conditions and displaying environmental friendliness. A comprehensive comprehension of the mechanisms governing these reactions is pivotal for enhancing catalyst efficiency and selectivity, while also preventing the complete mineralization of organic substances into CO2 and water.
The subsequent sections will individually highlight these intriguing reactions and examine the influence of the reaction environment, aiding in the understanding of the forthcoming strategies for controlling selectivity, which holds paramount importance in organic synthesis. The selective oxidation of alcohols represents a key pathway for synthesizing carbonyl compounds. Traditional alcohol oxidation often entails thermocatalytic procedures or the use of potent oxidizing agents like chromate, permanganate, and hypervalent iodine. These conventional processes invariably generate harmful waste and typically exhibit low selectivity towards the desired high-value chemicals due to the harsh conditions employed. In contrast, the photocatalytic oxidation of alcohols presents a more environmentally sustainable alternative for the synthesis of carbonyl compounds. In the following discussion, we delve into the fundamental mechanism of alcohol oxidation, using benzyl alcohol oxidation as a model reaction.233
3.6. Antibacterial and biocidal disinfection
Numerous antimicrobial materials have undergone extensive research to safeguard humans, animals, and the environment. These include metals like silver and copper,234–236 titania,237–241 and organic compounds such as antibiotics and chlorine compounds. While many of these materials have found widespread use in everyday products, concerns persist regarding environmental contamination, potential toxicity,242 antibiotic-resistant bacteria (ARB), and high costs. In this context, carbon-based compounds, particularly graphene, have emerged as promising antimicrobial materials. It is worth noting that the study of the antimicrobial activity of graphene species is a relatively new topic, with the first report on GO/TiO2 published by Akhavan and Ghaderi in 2009.243 This report indicated that GO/TiO2 composites exhibited significantly higher activity than titania, with GO itself being inactive both under UV-vis irradiation and in dark conditions, merely accelerating the activity of titania. Subsequently, in 2010, the same authors revealed that graphene nanosheets, in the form of nano-walls, displayed bactericidal activity against both Gram-negative (Escherichia coli) and Gram-positive (Staphylococcus aureus) bacteria, with reduced graphene oxide (rGO) demonstrating higher activity than GO.244 For instance, studies have shown that GO exhibits higher bactericidal activity than graphene (prepared by reducing GO with Allium cepa extracts), which may be attributed to the typical aggregation of graphene.245 Additionally, the concentration of GO significantly affects bacterial viability, with low concentrations of GO promoting bacterial growth, and high concentrations inhibiting it.246 The morphology of GO membranes, including roughness, sharpness, and curvature, has also been found to influence bactericidal effects, with rougher or sharper membranes exhibiting higher activity.247–251 Interestingly, culture media have been shown to influence bactericidal effects, with GO and rGO exhibiting antibacterial activity in one medium (Luria–Bertani, LB) due to oxidative stress (deficiency of Mg2+ and Ca2+ ions), while being less effective in another medium (Mueller–Hinton, MH) with higher concentrations of Mg2+ and Ca2+ ions.247,248 Notably, Gusev et al. proposed that rGO does not exhibit direct bacterial toxicity, but efficient removal of live/dead bacteria occurs through filtration upon aggregation of bacteria and rGO. Furthermore, the inhibited growth of bacteria in nutrient broth (NB) medium is attributed to nutrient adsorption on rGO, not antibacterial activity.248 Carbon, not necessarily in graphene form, could also be responsible for bactericidal activity, as demonstrated by the comparable antibacterial activity of graphene (rGO) and carbon-modified titania samples in the dark and under visible light irradiation. Three main mechanisms of bactericidal activity of graphene and its compounds have been proposed, involving cell membrane damage,244,252 oxidative stress caused by reactive oxygen species (ROS),253 and charge transfer.254 Moreover, graphene-based materials have shown potential in combating microorganisms that cause chronic wounds and diseases due to prolonged inflammation, persistent microbial infections, and drug-resistant microbial biofilms. For example, graphene oxide (GO) has been studied for its antimicrobial and antibiofilm properties against bacterial wound pathogens such as S. aureus, Pseudomonas aeruginosa, and yeast Candida albicans. The interactions between GO and microorganisms, including hydrogen bond interactions between abundant carbonyl and hydroxyl groups in polysaccharides and GO, and π–π interactions between DNA/RNA bases and graphenic domains in GO, lead to the inhibition of microorganisms' aggregation and adhesion on surfaces.255,256 Moreover, a study has suggested that poly(ethylene glycol-amine)-derivatized GO nanosheets (PEG-GO) can stimulate and activate macrophages, enhancing phagocytosis of C. albicans, thereby potentially preventing some chronic infectious diseases.257 Additionally, GO has been modified with various organic compounds, such as chitosan, curcumin, antimicrobial agents, and dyes, to enhance its antimicrobial activity and biocompatibility.249,258–276 Furthermore, graphene composites, with metals and semiconductor photocatalysts like TiO2, ZnO, and NiO, have shown improved antimicrobial activity through enhanced charge transport, reduced charge carrier recombination, and visible light absorption.277–287 For example, the combination of Cu/ZnO with rGO resulted in oxidative stress and damage to bacterial cell membranes, leading to bactericidal effects.279Fig. 20 presents the antibacterial mechanism of CuZnO@rGO nanocomposites.279
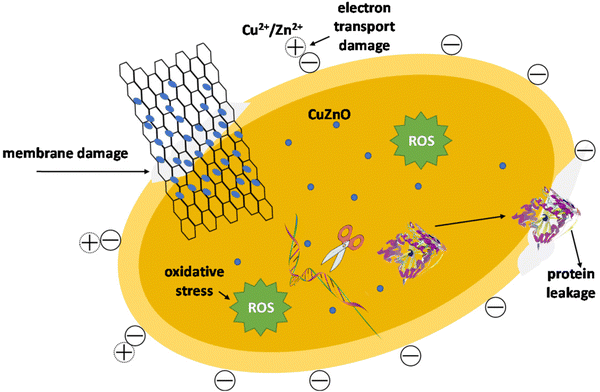 |
| Fig. 20 Antibacterial mechanism of CuZnO@rGO nanocomposites.279 | |
Additionally, the development of laser-induced graphene (LIG) filters has demonstrated efficient bacterial capture and destruction through Joule heating.288–290 Graphene derivatives have also been explored as antiviral materials to combat various viruses,291 with the blocking of virus binding sites being the main mechanism. Fig. 21 presents SEM images of B. subtilis cells without treatment and treated with 10 mg mL−1 GO@CS@TiO2 for 2 h. NPs1, NPs2 and NPs3 indicate the ratio of GO/CS/TiO2 equal to 1
:
20
:
2, 1
:
20
:
4 (the best activity), and 1
:
20
:
8, respectively.284
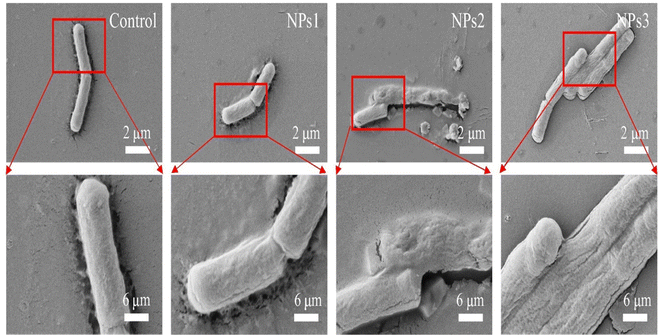 |
| Fig. 21 SEM images of B. subtilis cells without treatment and treated with 10 mg mL−1 GO@CS@TiO2 for 2 h. NPs1, NPs2 and NPs3 indicate the ratio of GO/CS/TiO2 equal to 1 : 20 : 2, 1 : 20 : 4 (the best activity), and 1 : 20 : 8, respectively.284 Reproduced (adapted) with permission from ref. 284. Copyright [2017] [Elsevier]. | |
Overall, graphene-based materials, particularly G/GO/rGO, hold promise as efficient antimicrobial agents, especially when combined with other antimicrobial agents, metals, or photocatalysts. These materials have found applications in diverse fields, including bandages, gauze, paper for food packaging, face masks, water purification filters, and next-generation biocomposites for dental and bone implants. Their potential for use in biosensors for the sensitive and rapid diagnosis of viral infections is also being explored.
3.7. Photocatalytic fuel cells
The photocatalytic fuel cell (PFC) is a system that simultaneously facilitates the breakdown of organic pollutants and generates electricity under optical excitation. This process involves both oxidation and reduction reactions, where contaminants convert chemical energy into electrical energy. Lina et al. introduced an innovative multifunctional PFC system that combines organic pollutant removal, hydrogen evolution, and electrical energy generation. They achieved this by utilizing Fe–MoS2/rGO as the photocathode and ZnFe2O4/Ag/Ag3VO4 as the photoanode composites, which were hydrothermally synthesized on carbon fiber cloth (CFC) and carbon felt (CF), respectively.292 In this system, formic acid aids in the degradation of methyl orange (MO) and berberine (BBR), but MO and BBR impede hydrogen generation. The photo electrodes absorb photons, generating electron–hole pairs. The bias applied over the photo electrodes facilitates the transfer of photo-induced electrons from the photoanode to the photocathode, and protons combine with the electrons to produce hydrogen. Reduced recombination of photo-induced carriers enhances contaminant removal and hydrogen evolution through efficient electron transfer. The organic pollutants are oxidized by the holes and reactive oxidative species (ROS). An illustration of the operating mechanism for the removal process in photocatalytic fuel cells under double LED is shown in Fig. 22. The electrons accepted by the protons dissociate formic acid (HCOOH) to produce hydrogen on FeMoS2/rGO@CFC, while at ZnFe2O4/Ag/Ag3VO4@CF, water (H2O) is oxidized to produce oxygen (O2) by H+ (holes). The reaction of O2 with H+ generates hydrogen peroxide (H2O2) via ˙O2− and ˙HO2−·H2O2 can be further decomposed into ˙OH. The photo-induced holes and reactive oxidative species eliminate MO and HCOOH over the photo electrodes, resulting in the removal of organic pollutants and the evolution of hydrogen. The PFC system was evaluated for organic pollutant removal and hydrogen evolution. The study demonstrated that with 15.2 mg cm−2 Fe–MoS2/rGO in 2 hours, approximately 1.65 μmol g−1 of hydrogen was collected, and approximately 9.34 mg L−1 of MO was removed.293
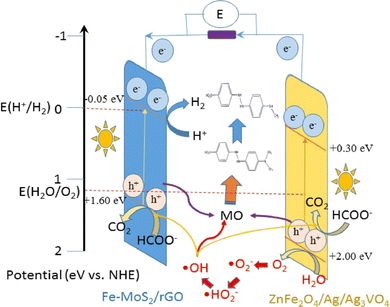 |
| Fig. 22 Schematic depiction of the suggested removal mechanics in a PFC under double LED.290 Reproduced (adapted) with permission from ref. 290. Copyright [2020] [Elsevier]. | |
3.8. NOx oxidation
Nitric oxides (NOx), which include NO, N2O, and NO2, are widely present in the environment and are associated with a range of detrimental effects, such as haze, acid rain, global warming, and the eutrophication of natural water bodies.294,295 In order to remove NOx, many methods are used, including wet approaches, catalytic removal, storage-reduction, and continuously regenerating traps (CRT). This work emphasizes, among other methods, photocatalytic NOx oxidation and breakdown as well as selective photocatalytic and catalytic reduction. The reduction of NOx occurs through photocatalytic mechanisms with the assistance of photogenerated electrons. The processes that occur during breakdown are listed in eqn (2)–(8):296,297 | NO(ads) + e−→ N(ads) + O(ads) | (2) |
| NO(ads) + N(ads) → N2O(ads) | (3) |
| NO(ads) + O(ads) → NO2(ads) | (4) |
| 2NO(ads) → O2(ads) + N2(ads) | (7) |
| 2N2O(ads) →O2(ads) + 2N2(ads) | (8) |
As shown in eqn (9)–(14),298 the radical species produced from the photogenerated electrons and holes are also used for the photocatalytic oxidation of NOx. | HNO2 + %OH→ NO2 + H2O | (10) |
| 2NO + %O2− + 3e−→ 2NO2 | (13) |
| 3NO2 + 2OH−→ 2NO3− + NO + H2O | (14) |
Selective catalytic reduction, which uses NH3, H2, CO, or hydrocarbons in the presence of a catalyst at temperatures between 300 and 400 °C, has been the subject of substantial research to address NOx pollution. On the other hand, similar reducing agents, photocatalysts, and light energy are frequently used in the selective photocatalytic process. eqn (15) and (17) provide a summary of the selective catalytic process specifically in the presence of ammonia:298
| O2 + 4N2 + 6H2O → 4NO + 4NH3 + O2 | (15) |
| 7N2 + 12H2O → 6NO2 + 8NH3 | (16) |
| 3N2 + 6H2O → 2NO2 + 4NH3 + O2 | (17) |
Researchers have been studying the photocatalytic conversion of NO
x with graphene-based photocatalysts more and more over time. Recently, they used a hydrothermal method to create (BiO)
2CO
3–BiO
2−x/graphene ternary nanostructures that removed more NO than the individual compounds. After 30 minutes of exposure to simulated solar light, the photocatalyst achieved a 60.7% NO removal rate and showed good stability over 5 cycles when it had an optimum composition of 35% BiO
2−x.
299 See
Fig. 23.
298
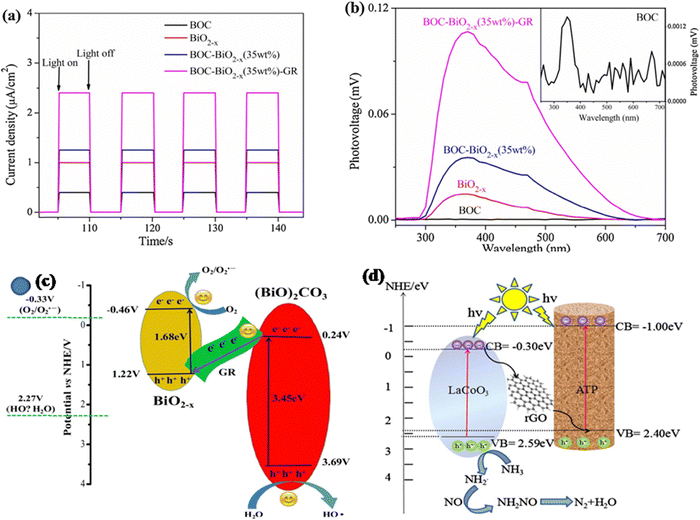 |
| Fig. 23 (a) and (b) Measurements of photocurrent and surface photovoltage in (BiO)2CO3–BiO2−x/GR, (c) the charge transfer mechanism in (BiO2)CO3–BiO2−x/GR,298 reproduced (adapted) with permission from ref. 298. Copyright [2019] [Elsevier], and (d) the NOx elimination process in LaCoO3/ATP/rGO.297 Reproduced (adapted) with permission from ref. 297. Copyright [2018] [Elsevier]. | |
In a different study, Hu and colleagues investigated the elimination of NO in a continuous flow reactor utilizing graphitic carbon nitride quantum dots/graphene oxide-indium vanadate (CNQDs/GO-InVO4) aerogel hybrids. They were able to create a photocatalyst with higher activity by carefully regulating the GO (25%)/CNQDs (75%) to InVO4 (30%) ratio. Electrostatic, hydrogen bonding, and stacking interactions allowed semiconductor nanoparticles to be anchored to the GO sheets.300 Improved photocatalytic activity was the result of the addition of GR material to photocatalysts, which was related to effective charge separation and transportation that decreased charge recombination. Photoluminescence (PL), electrochemical impedance spectroscopy (EIS), surface photovoltage (SPV), photocurrent measurements (PC), and photovoltage (SPV) measurements were used to demonstrate this.299,300 SnO2 and Sn vacancies in Zn2SnO4 were created by graphene during the hydrothermal synthesis of Zn2SnO4/GR nanocomposites. Better NO removal was achieved because to the addition of GR, which also increased specific surface area, charge separation, and migration. Further increases in GR loading resulted in decreased activity because of the shielding effect of GR on Zn2SnO4–SnO2, which had an impact on light harvesting. The optimum GR loading was found to be 3%.301
Under simulated solar light the proposed Z-scheme mechanism for NO removal over LaCoO3/ATP/rGO involved electron transfer from the conduction band of LaCoO3 to the valence band of ATP via the rGO sheet, whereas under visible light only LaCoO3 was excited, leading to lower photocatalytic activity.302 Promising results were obtained from a new binary nanocomposite of GO and amine-functionalized titanium metal organic framework (NH2-MIL-125(Ti)). As the photocatalyst was being prepared, GO served as an antenna for microwave absorption, creating hot patches on the surface that allowed for even dispersion of MOF particles and powerful interactions between the MOF and GO. Under visible light illumination, this nanocomposite had the maximum activity, removing 50% and 65% of NO and acetaldehyde, respectively.303 With the connection of Bi-NPs and GO increasing the NO removal effectiveness, bismuth nanoparticles (Bi-NPs) anchored on GO displayed better plasmonic NO photocatalytic removal under UV irradiation.304,305 Similar to this, following 300 °C annealing, a binary composite ZnCo2O4 generated from a MOF supported on rGO had the maximum NO activity under visible and solar-like light (GZC300). Fig. 24 and 25 present microwave and low-temperature treatment for ZnCo2O4/rGO synthesis and production of the reactive species during NO oxidation over ZnCo2O4/rGO.303
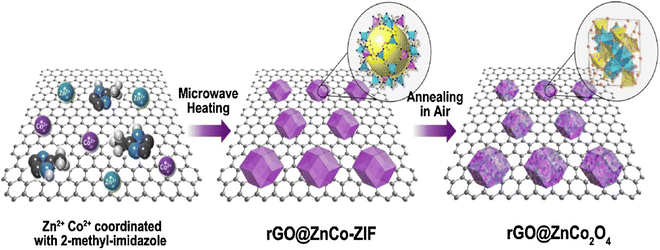 |
| Fig. 24 Microwave and low-temperature treatment for ZnCo2O4/rGO synthesis.303 Reproduced (adapted) with permission from ref. 303. Copyright [2018] [Elsevier]. | |
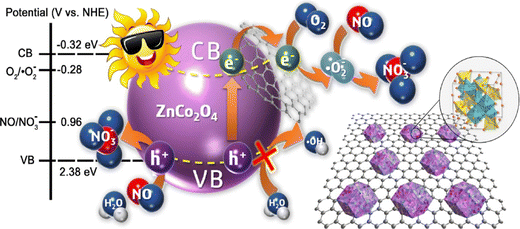 |
| Fig. 25 The production of the reactive species during NO oxidation over ZnCo2O4/rGO according to the mechanism proposed in ref. 303. Reproduced (adapted) with permission from ref. 303. Copyright [2018] [Elsevier]. | |
The photocatalyst's activity was greatly impacted by the annealing temperature.306 Under UV-vis light exposure, a multipurpose melamine sponge (MS) coated with gC3N4/GO demonstrated improved photocatalytic capacity for NO oxidation, adsorption of dyes from organic solvents, and CO2 reduction. The nanocomposite showed a significant capacity for adsorption and the majority of NO was transformed to NO3.307 Under visible light irradiation, monolithic tungsten oxide/graphene oxide aerogels (WO3/GO) showed excellent selectivity with 51% NO elimination, which was 3.3 times better than that of pure WO3. The photocatalyst was kept from being sucked into the reactor gas lines and allowed for simple recycling owing to the nanocomposite's flexibility and monolithic design, which also made it possible to manipulate its form and size.308
Overall, adding graphene-based materials to photocatalysts makes them more effective in removing NO. To achieve the optimum photocatalytic performance, optimal loading and careful design are essential. Cleaner air is ensured via solar-driven photocatalytic water splitting and CO2 reduction, which are promising solutions for long-term, cost-effective NOx removal.309
3.9. Removal of heavy metals
Hazardous heavy metals may become less harmful species when used in photocatalytic treatment of water contaminated with heavy metals using GR-based heterojunctions. Additionally, the presence of GR (graphene), which has significant adsorption properties, enables simultaneous photocatalytic reduction/oxidation and metal adsorption.310 A recent study examined the photocatalytic activity of a new visible light binary nanocomposite made of reduced graphene oxide (rGO) and Al4SiC4 for the degradation of RhB and MO as well as for the reduction of Cr(VI). The Al4SiC4/rGO heterostructure successfully eliminated both contaminants from single and mixed solutions at an optimized rGO loading of 2.5%. After 60 minutes of visible light irradiation, it removed 96% and 45% of Cr(VI) and RhB, respectively, from mixed solutions. The solution pH was critical in the photocatalytic destruction of dye molecules, while the presence of dye molecules had no effect on the photoreduction efficiency of Cr(VI), confirming the nanocomposite's potential for wastewater treatment.311
By using a 400 °C calcined Ni2O5/GR hybrid with 4% GR loading, the synergistic oxidation of 4-chlorophenol (4-CP) and Cr(VI) was studied. The activity towards 4-CP increased as the GR loading increased from 1% to 4%, and then reduced as a result of the excess GR's shielding effect, which made it more difficult for Nb2O5 to access visible photons. 4-CP helped with the photocatalytic reduction of Cr(VI), which was not seen without the substance. After three hours of exposure to visible light in the presence of 50 mg L−1 of 4-CP, Cr(VI) reduction peaked at 92%, demonstrating the role of 4-CP as an electron donor and GR as a structure-directing and morphology-controlling substance. Additionally, 4-CP oxidation and mineralization increased and reached their peak activity in the presence of Cr(VI).
Numerous factors, such as the kind and quantity of pollutants, the pH of the solution, the nature of the photocatalyst, and the characteristics of the photocatalyst surface, affect how well a photocatalytic system removes pollutants from a mixed matrix solution. By enabling charge separation and transfer, as well as helping to concentrate pollutant molecules on the photocatalyst surface, GR materials play a part in improving the photocatalytic process. For instance, during the simultaneous reduction of Cr(VI) and oxidation of dyes over Al4SiC4/rGO binary nanocomposites, visible light was used to excite both the semiconductor and RhB. RhB's photoexcited electrons were moved into the Al4SiC4 conduction band and engaged in Cr(VI) reduction there. Additionally, some of these electrons were carried to the rGO sheet, where they were converted to Cr(III) pore sizes.311
A somewhat different scenario was observed for the simultaneous Cr(VI) reduction and 4-CP degradation through a ligand-to-metal charge transfer (LMCT) process. The huge band gap of Nb2O5/GR prevented it from being activated by visible light. However, the Nb2O5/GR composite became active when 4-CP was introduced, allowing for the withdrawal of both 4-CP and Cr(VI). A complex between 4-CP and Nb2O5 was formed as part of the charge transfer pathway, and this combination was excitable by visible light. From the complex, the photogenerated electrons proceeded to the Nb2O5 conduction band. These electrons then moved to the surface of GR, where the reduction of Cr(VI) occurred. The electrons were also grabbed by O2 to produce the superoxide radical, which aided in the breakdown of the 4-CP phenoxyl radicals. In another work, adding citric acid to the reaction medium increased Cr(VI) elimination because citric acid served as a hole scavenger, reducing electron–hole recombination.312 Several GR-based materials have been tailored for multifunctional applications, ranging from heavy metal reduction to dye degradation, disinfection, and fuel generation. Such materials show promise in treating real water samples that often contain multiple pollutant species, making them a valuable tool for achieving environmental sustainability.310–326Fig. 26 shows examples of the hypothesised LMCT mechanism, which show simultaneous 4-CP oxidation and Cr(VI) reduction over a Nb2O5/GR composite and a glucose/rGO complex, respectively.312 And Fig. 27 presents (a) UV-vis-NIR spectra, (b) EIS plots of ZnS/rGO (ZR) with various rGO loadings, and (c) reactive species production in TiO2/GO nanostructures and subsequent redox reactions leading to Pb2+ and Cd2+ detoxification.314,319
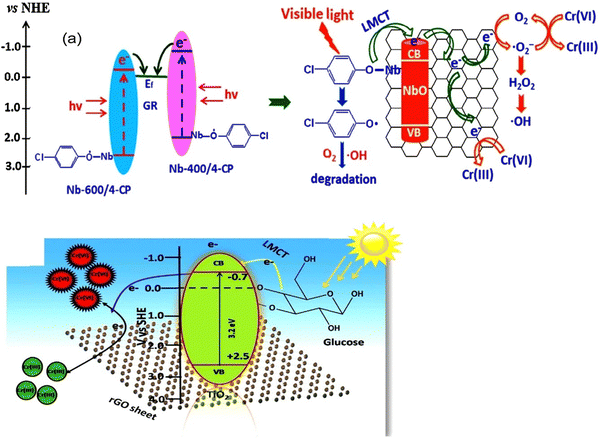 |
| Fig. 26 Examples of the hypothesised LMCT mechanism, which show simultaneous 4-CP oxidation and Cr(VI) reduction over a Nb2O5/GR composite and a glucose/rGO complex, respectively.312 Reproduced (adapted) with permission from ref. 312. Copyright [2020] [Elsevier]. | |
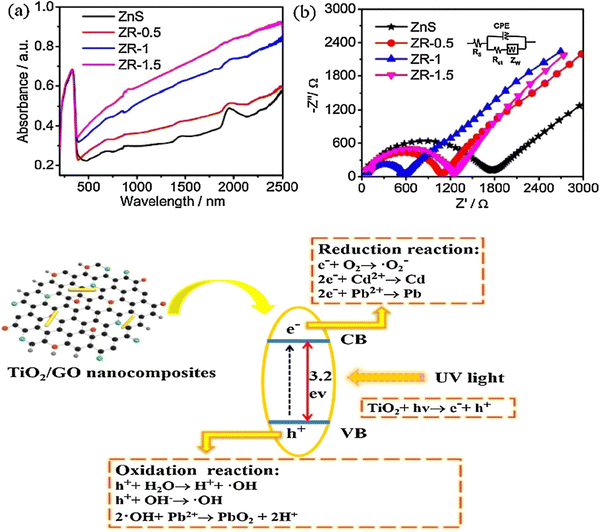 |
| Fig. 27 (a) UV-vis-NIR spectra, (b) EIS plots of ZnS/rGO (ZR) with various rGO loadings, and (c) reactive species production in TiO2/GO nanostructures and subsequent redox reactions leading to Pb2+ and Cd2+ detoxification.314,319 Reproduced (adapted) with permission from ref. 314. Copyright [2018] [Elsevier], and ref. 319. Copyright [2018]. [RSC]. | |
3.10. Degradation of microplastics
Pollutants that have the potential to affect the environment because of their persistence and unpredictability are referred to as emerging contaminants (ECs). Hormones, microplastics, medications, surfactants, and personal care items are a few examples of ECs. Microplastics are regularly found in a variety of water sources, including freshwater, wastewater, groundwater, and oceans. Researchers have therefore been working hard to find effective ways to get rid of them.327 Due to their sluggish kinetics of degradation, high stability, increased hydrophobicity, prolonged residence duration, and ability to absorb other pollutants, microplastics are a major source of worry for environmentalists and biologists.328 Microplastics' negative effects result from a number of factors, including their innate physical and chemical characteristics, capacity to sorb other contaminants, and variation in transport paths. Due to their small size, conventional removal techniques like sedimentation are ineffective against microplastics. In order to rid the biota of microplastics, researchers have concentrated on creating cutting-edge removal and filtration methods.329–331 Adsorption on green algae, dynamic membranes, and sophisticated filtration technologies are a few of the physical removal approaches that have been researched.332,333 For instance, Sundbaek et al.334 studied the sorption of fluorescent polystyrene microplastic particles on Fucus vesiculosus seaweed and found that the efficacy of adhesion was great, at about 94.5%. According to the researchers, the sorption mechanism involves “trapping” microplastics inside the microalgae's microchannels. Furthermore, Yuan et al.335 investigated and modelled the polystyrene microplastics adsorption by three-dimensional reduced graphene oxide (3-D rGO) sheets, indicating a maximum adsorption capability of 617.28 mg g−1 under particular circumstances. Strong covalent interactions between the polystyrene benzene ring and the rGO carbon ring were identified as the adsorption mechanism, and these findings were confirmed by Langmuir's adsorption isotherm and a pseudo-second-order kinetic model.336Fig. 28 presents SEM images of polyethylene before and after interacting with alkoxy-silyl functionalized particles to produce agglomerates.336
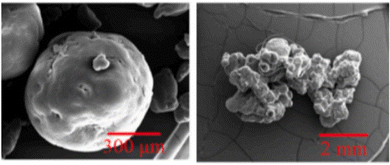 |
| Fig. 28 SEM images of polyethylene before and after interacting with alkoxy-silyl functionalized particles to produce agglomerates.336 | |
Chemical processes have also been investigated as a first stage in a more intricate filtration system for eliminating microplastics, such as agglomeration utilizing alkoxy-silyl functionalized particles.337 Wastewater treatment facilities frequently use tertiary treatments to get rid of up to 99% of these difficult-to-remove pollutants. These facilities are known to be significant sources of microplastics entering seas. Based on the morphology of the microplastics discovered in the influents, McCormick et al.338 studied the primary, secondary, and tertiary wastewater treatment stages that were the most effective at removing plastics. Nine typical microplastic morphologies were found, with films, fibres, fragments, and pellets being the most frequent types in wastewater treatment systems. The removal efficiency varied for various shapes during various phases of treatment, highlighting the necessity of removing microplastics before releasing the effluent into aquatic settings to protect aquatic environments and marine ecosystems. Conventional removal techniques do have some drawbacks, though, such as high operation and maintenance costs and technical challenges in scalability and industrialization. As a result, researchers must overcome the difficult problem of creating efficient removal techniques that can be used in industrial applications and to enhance current filtration systems.339
Adsorption technologies have drawn the most attention among the investigated removal techniques due to their practical and cost-effective removal of a variety of organic and inorganic contaminants.339 Through intermolecular interactions, a substance (adsorbate) builds up on the surface of a porous solid or liquid (adsorbent) during the process of adsorption. While adsorption isotherms like the Langmuir model and Freundlich model offer insights into the nature of adsorption in monolayer or multilayer form with respect to the adsorbent's surface structure, adsorption kinetics can be analysed using pseudo-first-order and pseudo-second-order models.340 Adsorbents can be made of synthetic or natural materials. Natural adsorbents including charcoal, biochar, clays, and zeolites are found in nature, but synthetic adsorbents, which can be used to remove microplastics and bioplastics, are produced using agricultural and industrial waste.341,342 Due to their good adsorption properties and affordability, activated carbon and graphene-based materials (GBMs) are often utilised as adsorbents, particularly in the removal of microplastics and bioplastics.343,344 While biochar is frequently made by heating up biomass waste at high temperatures in an oxygen-limited environment, activated carbon is made by heating up coal at high temperatures without oxygen. Biochar, charcoal, and activated carbon are desirable adsorbents for a variety of applications due to their adaptability, flexibility, functionalization potential, and resilience.345
Environmental implications of graphene based photocatalysis.
Graphene-based photocatalysis presents a revolutionary approach with significant potential for addressing pressing environmental challenges. Through its unique electronic, optical, and chemical properties, graphene serves as an efficient photocatalyst in various applications, including water purification, air pollution control, hydrogen production, carbon dioxide reduction, self-cleaning surfaces, wastewater treatment, and antibacterial uses. The utilization of graphene-based photocatalysts in water purification can lead to cleaner water sources by effectively breaking down harmful organic contaminants. Moreover, their ability to decompose toxic gases contributes to cleaner air and improved urban air quality, benefiting public health.
The prospects of sustainable hydrogen production and CO2 reduction using graphene-based photocatalysts hold promise for a cleaner and greener energy future, mitigating climate change and promoting renewable energy sources. The incorporation of graphene photocatalytic coatings on surfaces opens doors to self-cleaning properties, reducing maintenance efforts and enhancing the longevity of infrastructure and equipment. Furthermore, in addressing industrial wastewater treatment, these photocatalysts demonstrate their ability to remove harmful compounds and heavy metals, safeguarding ecosystems and water resources. Lastly, the application of graphene-based photocatalysts in water disinfection and antimicrobial coatings can contribute to controlling the spread of harmful pathogens, safeguarding public health. While the potential benefits of graphene-based photocatalysis are vast, there remain challenges that require further research and development, such as scalability, cost-effectiveness, and long-term stability.
Despite these challenges, researchers are actively working towards refining this technology, unlocking its full potential, and making it a viable and sustainable solution for environmental remediation and energy production. In the years to come, advancements in graphene-based photocatalysis are expected to drive positive change, offering innovative and eco-friendly solutions to some of the most pressing environmental issues we face today. As we move towards a greener and more sustainable future, the promise of graphene-based photocatalysis shines brightly on the horizon.
4. Electrocatalytic applications of graphene-based materials
4.1. Fuel cells
Fuel cells represent a series of energy conversion devices that continuously generate electricity when supplied with fuels. Due to the direct conversion of chemical energy to electricity, fuel cells boast significantly higher system efficiency compared to combustion engines, while also emitting fewer pollutants. As a result, these cells have emerged as an appealing solution for addressing global energy and environmental challenges, contributing to cleaner and more sustainable living. An electrolyte layer is placed between two electrodes in a conventional fuel cell (Fig. 29).346 The fuel oxidises on the anode surface, producing electrons that pass through an external circuit to decrease O2 at the cathode. Concurrently, mobile charge carriers (H+, OH−, CO2, or O2) cross the electrolytes, guaranteeing the completion of the circuit. The fuel cells are categorized based on the type of electrolyte they employ, with the phosphoric acid fuel cell (PAFC), polymer electrolyte membrane fuel cell (PEMFC), alkaline fuel cell (AFC), molten carbonate fuel cell (MCFC), and solid-oxide fuel cell (SOFC) being the main types.346 PAFCs, PEMFCs, and AFCs are appropriate for cars and portable applications because they run at relatively moderate temperatures (300 °C), but MCFCs and SOFCs flourish at high working temperatures (500 °C) and show promise for stationary applications.
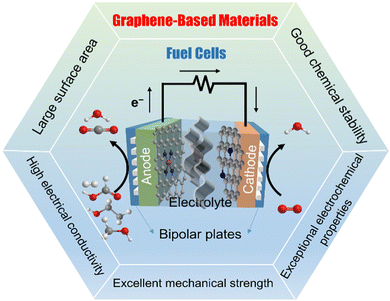 |
| Fig. 29 Schematic illustration of integration of graphene-based materials in every component of a fuel cell.347 | |
Choosing the correct materials for fuel cell components presents challenges in terms of electrochemical performance, efficiency, and durability.346 Fortunately, graphene and its derivatives showcase exceptional chemical, electronic, and mechanical properties, positioning them as viable alternatives for fuel cell applications (Fig. 29). Recent years have witnessed significant efforts in harnessing the potential of graphene-based materials within fuel cells. These materials prove to be excellent electrocatalyst supports, augmenting the active site count and streamlining electron transport for reactions involving fuel oxidation and oxygen reduction (ORR).348,349
Metal-free graphene materials have emerged as compelling contenders for oxygen reduction reaction (ORR) in fuel cells, attributed to their high electrocatalytic activity, excellent tolerance to poisoning, and cost-effectiveness. Considerable insights have been gained in comprehending the impact of electronic structure modification, doping configurations, defects, or functional groups of graphene on fuel cell performance.350,351 Additionally, the incorporation of graphene-based materials in polymer membranes enhances ionic conductivity and reduces fuel crossover.352 These materials show significant potential as proton-exchange membranes because they have strong proton conductivity and are impervious to water, H2, and methanol.353 Moreover, beyond electrolytes and electrodes, graphene-based materials contribute to improved current collection, fuel/air distribution, and bipolar plate stability.354 Extensive reviews on the catalytic and electrochemical characteristics of graphene-based materials have previously been published.355–361 Recent evaluations have mostly focused on particular fuel cell application domains, such as ORR,349–351 fuel oxidation,347,348,362 membranes,352 and bipolar plates.354
Oxygen reduction reaction in fuel cells.
Oxygen reduction reaction in fuel cells involves the conversion of chemical energy into electrical energy through fuel oxidation catalyzed by immobilized catalysts on electrodes.363,364 Fuel cells present tremendous potential as clean and efficient power sources for electric vehicles (EVs) due to their high energy conversion efficiency, low operating temperatures, minimal or zero emissions, and impressive energy and power density.365 However, the sluggish kinetics of the ORR (O2 + 4H+ + 4e−/2H2O) remains a significant impediment to achieving higher energy conversion efficiency and large-scale commercialization of fuel cells.366 As a result, efficient electrocatalysts for cathode ORR in fuel cells and Li–air batteries are becoming increasingly important.367–369 Despite these challenges, Pt-based materials have historically served as active electrocatalysts for both anodes and cathodes in fuel cells. Notably, Pt-based cathode electrocatalysts are susceptible to the crossover effect, in which fuel molecules move across the membrane from the anode to the cathode, causing CO poisoning, carbonate precipitation, and a fall in the system pH.370–372 Moreover, the high cost and limited natural reserves of Pt pose significant barriers to fuel cell technology's widespread availability.373 To address these concerns, there is a growing interest in replacing Pt with metal or metal-free catalysts.374 Carbon (e.g., active carbon, porous carbon, carbon nanotubes, graphene, etc.), mesoporous silica, carbides, conducting polymers, and metals have all been investigated as nanostructured catalyst supports to improve catalytic activity and durability by utilising the electroactive surface area of the catalysts. Graphene, a 2D single layer sheet of hexagonal carbon atoms, has emerged as a potential catalyst support due to its high surface area, excellent electrical conductivity, chemical and environmental durability, and strong interaction with catalyst NPs.375 Graphene also has a particular benefit in that it can be manipulated to construct 3D networks with active NP loadings as ORR catalysts.373 These systems are highly desirable as they allow the utilization of graphene sheets' distinctive topographies, alongside macroporosity and multidimensional electron transport pathways.374,376
The effectiveness of incorporating heteroatoms (N, P, B) into sp2 hybridized carbon frameworks in graphene to enhance electrical properties and chemical activities has been validated by both theoretical calculations and experiments.377 Initially, the higher electronegativity of N (3.04) compared to C atoms (2.55) was proposed as the reason behind the increased activity, resulting in the formation of positive charge density on adjacent C atoms and favorable O2 adsorption.378,379 Recent studies have also demonstrated significant catalytic activity in other carbon materials doped with low electronegativity atoms, such as P-doped graphite layers (2.19)380 and B-doped CNTs (2.04).381 Interestingly, experiments using elements with similar electronegativity to carbon, such as sulphur (2.58) and selenium (2.55) doped in graphene as a cathode for the ORR, have yielded compelling results.382 The Se/S-doped graphene demonstrated excellent electrocatalytic performance, including catalytic activity, long-term stability, and high methanol tolerance in alkaline media for the ORR. Theoretical studies based on simulation calculations have confirmed that introducing charged sites by disrupting the electroneutrality of graphitic materials is crucial for enhancing ORR activity, regardless of the dopant type.383 As a result, customizing the electronic arrangement of graphene through doping has emerged as a practical approach to produce significantly improved materials for the ORR in fuel cells. Researchers have put considerable efforts into overcoming the mentioned challenges and enhancing electrocatalyst performance by using graphene as a conductive and elastically robust substrate to prevent structural changes in cathodic materials. In fact, some research groups have explored graphene itself as a promising cathodic catalyst for ORR by incorporating heteroatoms.
For instance, Zhang et al.370 developed a straightforward method to prepare N-doped graphene with amino functional groups for the cathodic ORR. The amino-functionalized graphene exhibited good performance with better tolerance to the fuel crossover effect. Additionally, they investigated the effect of different nitrogen centers on the catalytic activity of the cathodic ORR, leading to experimental results that revealed the involvement of graphitic and amino types of nitrogen in determining the onset potential and electron transfer number, while the total content of graphitic and pyridinic nitrogen atoms contributed to enhancing the current density in the electrocatalytic activity for the ORR (Fig. 30)370
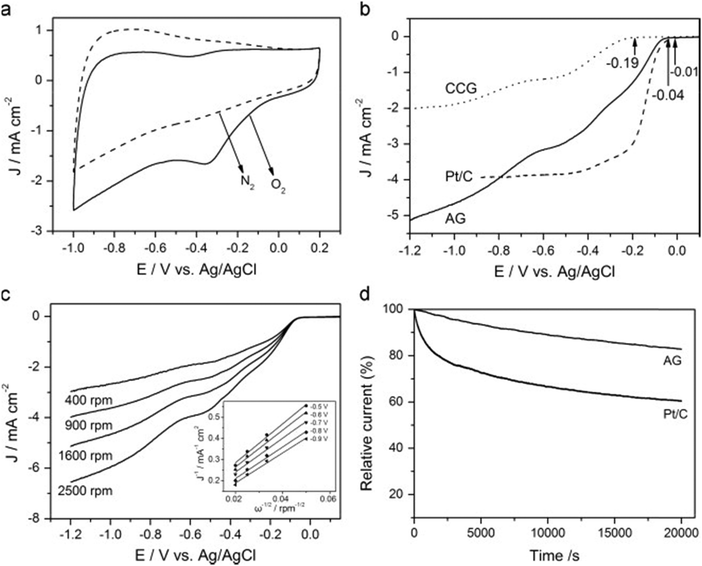 |
| Fig. 30 Illustration of various experimental results related to the electrochemical performance of different electrodes in an O2-saturated 0.1 M KOH solution. (a) Cyclic voltammetry (CV) curves of AG (as-grown graphene) under both N2- and O2-saturated conditions at a scanning rate of 100 mV s−1. (b) Linear sweep voltammetry (LSV) curves of CCG (chemically converted graphene), Pt/C (platinum/carbon), and AG electrodes in an O2-saturated 0.1 M KOH solution at a scanning rate of 10 mV s−1 and a rotation speed of 1600 rpm. (c) Rotating disk electrode (RDE) curves of the AG electrode in an O2-saturated 0.1 M KOH solution, showcasing different rotation speeds while recording at a scanning rate of 10 mV s−1. The inset demonstrates the Koutecký–Levich (K–L) plots of J1 (the limiting current density) versus u(1/2) (the rotation rate) at various electrode potentials, derived from the RDE measurements. (d) Current–time response of AG and Pt/C electrodes at 0.28 V in an O2-saturated 0.1 M KOH solution, with the experiment conducted at a rotation speed of 1600 rpm.370 Reproduced (adapted) with permission from ref. 370. Copyright [2013] [Elsevier]. | |
Yang et al. synthesized S-doped graphene and discovered that the electronegativity of the doping element was not crucial, whereas disrupting the electroneutrality of graphitic planes played a significant role in enhancing O2 adsorption and serving as the center for oxygen catalysis, resulting in improved performance of the doped graphene.
Mahmood et al.365 created a graphene-based cobalt sulphide composite and found that the inclusion of graphene boosted conductivity, provided a wide surface area, and supplied functionalized centres for greater O2 adsorption, all of which resulted in higher ORR performance. They also looked at ORR catalysts in acidic medium and determined that treating the electrocatalyst with acid to remove inactive species improved performance.
Zhang et al.367 proposed an N-doped graphene composite with iron phthalocyanine (FePc), creating a new non-precious metal electrocatalyst class. Their findings demonstrated that N-doping graphene with FePc supplied multiple catalytically active centres, resulting in an electrocatalyst with high activity, greater conductivity, and overall better performance and fuel tolerance compared to commercial Pt/C (Fig. 31).
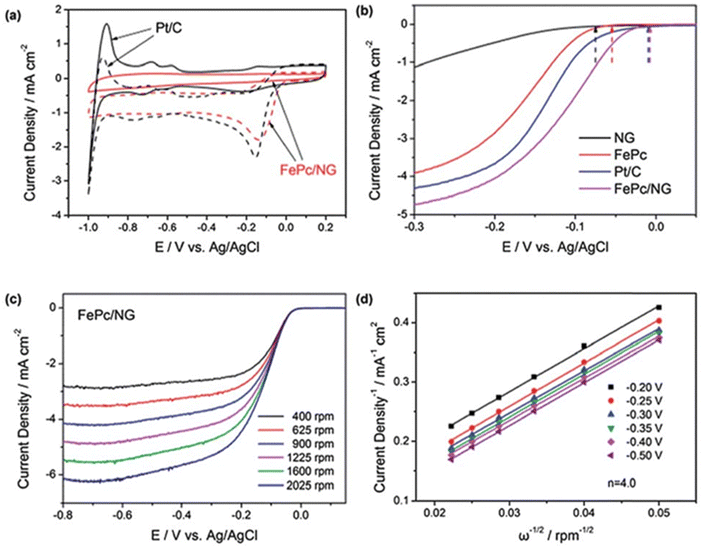 |
| Fig. 31 Illustration of various experimental results pertaining to the electrochemical performance of different catalysts in an O2-saturated 0.1 M KOH solution. (a) Cyclic voltammetry (CV) curves of Pt/C and FePc/N-doped graphene catalysts, with the former under both O2-saturated (dotted) and N2-saturated (solid) conditions. (b) Linear sweep voltammetry (LSV) curves of N-doped graphene, FePc, Pt/C, and FePc/N-doped graphene electrodes in an O2-saturated 0.1 M KOH solution at a scan rate of 10 mV s−1 and a rotation speed of 1600 rpm. (c)Rotating disk electrode (RDE) curves of FePc/N-doped graphene in O2-saturated 0.1 M KOH, showcasing different rotation speeds while recording at a scan rate of 10 mV s−1. (d) The Koutecký–Levich (K–L) plots of the FePc/N-doped graphene electrode derived from RDE measurements.367 Reproduced (adapted) with permission from ref. 367. Copyright [2012] [RSC]. | |
Furthermore, Zhang et al.384 recently discovered that doping phosphorus into graphene's graphitic plane increased oxygen reduction on the cathode of fuel cells more efficiently compared to regular graphene, since phosphorus activated graphene's neutral p-electronic cloud.
Wu et al.373 pioneered the path for improved electrocatalytic performance by developing 3D graphene networks with a bigger surface area and multidimensional channel for O2 flow compared to traditional 2D graphene sheets (Fig. 32). Adopting solutions like 3D support of electrochemically active and conductive substrates with high-performance non-precious electrocatalysts shows promise for future fuel cell realization.385
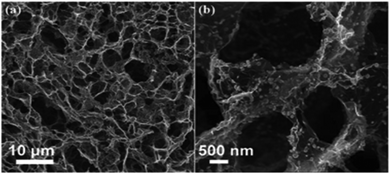 |
| Fig. 32 SEM images revealing the microstructure of two samples: (a) 3D graphene and (b) 3D graphene composited with Fe3O4.372 Reproduced (adapted) with permission from ref. 372. Copyright [2012] [ACS]. | |
4.2. ORR
The ORR plays a critical role as the basic cathodic reaction that straightly impacts the whole activity of a fuel cell. In an aqueous solution, the ORR can proceed via a four-electron reduction of O2 to form H2O as the end product (i.e., O2 + 4H+ + 4e− → 2H2O) or a reduction with two-electrons, generating H2O2 as an intermediate product in a two-step process (i.e., O2 + 4H+ + 2e− → H2O2 + 2H+ + 2e− → 2H2O).386,387 Due to the involvement of various e−/H+ transfers, the ORR inherently suffers from slow reaction kinetics in the absence of electrocatalysts.387 Currently, Pt as well as Pt-based metals stand out as efficient catalysts towards ORR. Additionally, incorporating Pt-based catalysts onto graphene as well as its derivatives can cause enhancement in the stability and efficiency of ORR, owing to the favourable surface area and overwhelming electrical conductivity shown by graphene. Numerous graphene or Pt-based catalysts of graphene derivatives are employed for the ORR.
For example, Kou et al. impregnated Pt nanoparticles onto functionalized graphene nanosheets, resulting in increased ECSA, improved efficiency of ORR, and prominent stability compared to the commercial E-TEK catalyst.388 Zhu et al. developed a hybrid 3D nanostructured film by willingly constructing ionic liquid-modified nanosheets of graphene and Pt nanoparticles to improve the mass transfer of reactants to the electrocatalysts.389 The ionic-liquid-modified graphene stabilized the Pt nanoparticles and formed a close interfacial contact, resulting in increased ORR activity and stability. Although MPt (M = Fe, Co, and Ni) alloys on standard carbon supports show promise as effective ORR catalysts,390–394 they lack stability under the acidic conditions of the ORR, since etching of the M component during the reaction could destroy the MPt alloy nanostructures.
However, employing a solution-phase-based self-assembly approach to anchor FePt nanoparticles onto graphene nanosheets (Fig. 33a) resulted in the FePt/graphene (FePt/G) catalyst exhibiting higher ORR activity in comparison to carbon (KetjenEC-300J)-supported FePt (FePt/C) and commercial Pt/C in 0.1 M HClO4 (Fig. 33b and c).393 Notably, the FePt/G catalyst demonstrated remarkable stability, with almost no activity decrease observed even after 10
000 potential cycles (Fig. 33d). The enhanced ORR activity of FePt/G could be attributed to the close contact between graphene and FePt, facilitating p-electron polarization from graphene to FePt, thus making the FePt surface more readily accessible for O2 absorption and activation.
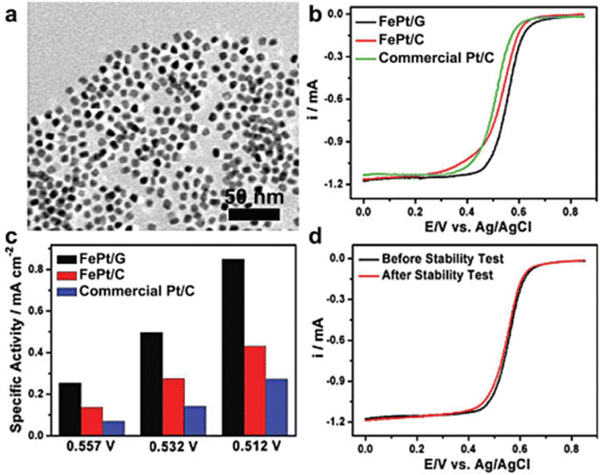 |
| Fig. 33 (a) The transmission electron microscope (TEM) image of the FePt/graphene catalyst. (b) Polarization curves of three different catalysts – FePt/G, FePt/C, and commercial Pt/C – for oxygen reduction reaction (ORR). (c) A comparison of ORR specific activity within the 0.512–0.557 V potential range (versus Ag/AgCl) for FePt/G, FePt/b, and commercial Pt/C catalysts. (d) The ORR polarization curves of the FePt/G catalyst before and after undergoing 10 000 potential sweeps between 0.4 and 0.8 V.393 Reproduced (adapted) with permission from ref. 393. Copyright [2012] [ACS]. | |
Despite graphene's promising advantages, there is a likelihood of graphene layers restacking due to strong π–π interactions, resulting in a significant blockage of active sites for the loaded catalysts and hindering reactant diffusion, thereby retarding catalytic activity.395 To address this issue, Li et al. combined the rGO-based Pt catalyst with the greater-surface-area carbon black to effectively prevent rGO nanosheet restacking.396 Furthermore, the rGO served as a barrier to prevent Pt leaching, while the carbon black served as an efficient site to renucleate diffused Pt species. The ECSA of Pt remained at >95% after 20
000 sweeping cycles, demonstrating a much increased ORR activity and durability for this hybrid catalyst. Recently, graphene-supported noble-metal single-atom catalysts for the ORR have been developed in an effort to decrease the use of noble metals and the price of electrocatalysts. Contrary to conventional nanoparticle-based catalysts, single-atom catalysts typically show not only considerably stronger interactions with their supports but also a reduced metal–metal coordination effect, allowing for flexibility in changing catalytic activity and chemoselectivity.397,398
Choi et al. recently produced an effective atomically dispersed Pt catalyst on a carbon-templated zeolite via wet impregnation.399,400 Using a 3D network of graphene nanoribbons with S-functionalized edges as a carbon support, they produced Pt–S4 complexes, which provided a large number of binding sites for individual Pt atoms (Fig. 34a and b).
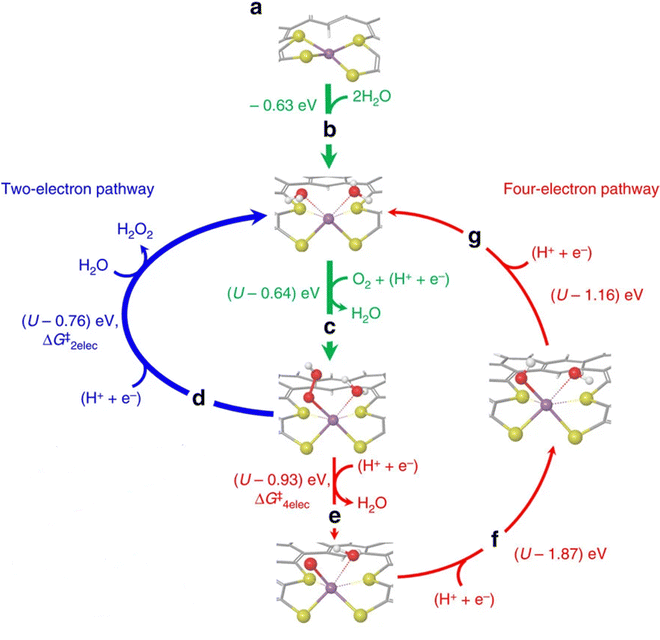 |
| Fig. 34 Illustration of the proposed oxygen reduction reaction (ORR) mechanism over the single-atom Pt/S-doped zeolite-templated carbon nanocatalyst. (a) Activation of the Pt center occurs by substituting two S atoms of the Pt–S4 complex with two O atoms from H2O molecules. (b) The first reduction of O2 takes place through proton-coupled electron transfer (PCET), leading to the formation of OOH intermediates. (c) In the two-electron pathway, another PCET event generates H2O2, which is then replaced by an H2O molecule, restoring the initial state where Pt is coordinated with two thiolates and two H2O molecules. (d) The four-electron pathway involves PCET leading to O–O bond dissociation, resulting in the production of H2O. (e) Another four-electron pathway leads to the creation of OH intermediates on the O atom through a third PCET event. (f) The fourth PCET event involving the OH intermediates produces H2O, restoring the Pt–S4 complex to its initial active state. In the figure, the blue, red, yellow, and purple spheres represent H, O, S, and Pt atoms, respectively.399 | |
The catalyst they created in the ORR did not produce H2O directly via the four-electron pathway. Instead, it followed the two-electron pathway, creating H2O2 with over 95% selectivity through the catalysis of Pt–S4 centres. The predicted reaction process is shown in Fig. 34c: activating the Pt–S4 centre by replacing its ligand with two H2O molecules; reducing O2 by two electrons via PCET to produce OOH intermediates; and forming H2O2via a second PCET before replacing the H2O2 molecule with one H2O molecule to return the Pt–S4 centre to its initial activated state.
This mechanism is distinct from the four-electron pathway of ORR, which comprises the second PCET's O–O bond dissociation and the first H2O formation (Fig. 34d), as well as the third and fourth PCET's second H2O formation (Fig. 34e and f). With the development of single-atom catalysts, this research paves the way for improving the selectivity of other electrochemical reactions while demonstrating a promising strategy for manufacturing H2O2. Later, a single-atom Ru catalyst for the ORR on nitrogen-doped graphene was also created.399 Contrary to the single-atom Pt catalyst, which adopted the two-step, two-electron strategy, the single-atom Ru catalyst followed the four-electron pathway and displayed high onset and half-wave potentials of 0.89 and 0.75 V (vs. RHE). This catalyst displayed exceptional toughness and resistance to CO and methanol poisoning. Pd, a noble metal being researched as a potential replacement for Pt for effective ORR processes, is similar to Pt in terms of its valence shell electrical structure and lattice constant.400 In comparison to the Pt/graphene catalyst in an alkaline solution, Seo et al. developed a uniformly dispersed Pd/graphene catalyst with higher mass and specific ORR activity.401 Additionally, a number of graphene-supported Pd-based alloy catalysts, including PdAg, PdAu, and PdY, showed a respectably high ORR activity.397,402,403
Although bulk Au and Ag are not capable of catalysing the ORR, their nanosized equivalents are. For instance, Yin et al. discovered that the catalytic activity for ORR of commercial Pt/C was comparable to that of surfactant-free Au nanoclusters on rGO (Au/rGO) at a rather high potential of −0.08 V (vs. Ag/AgCl). The Au/rGO catalyst performed similarly well in terms of methanol tolerance, and its excellent stability may be attributed to the addition of rGO, which prevented Au clusters from aggregating and leaching during the ORR. Similar to this, graphene-supported Ag clusters were developed and showed competitive ORR performance in comparison to an industrial Pt/C catalyst.404–406
An innovative process involving ball milling and subsequent pyrolysis was employed to successfully create an edge-activated S-doped Fe–N–graphene (EA-SFeNG). By introducing S and generating edge sites within the Fe–N–graphene structure, the performance of EA-SFeNG in the oxygen reduction reaction (ORR) was significantly enhanced. Specifically, the onset potential shifted from 0.91 VRHE to 1.0 VRHE, and the half-wave potential increased from 0.77 VRHE to 0.848 VRHE. The catalytic capabilities of EA-SFeNG were found to be comparable to those of commercial 20 wt% Pt/C (Vonset: 1.05 V, V1/2: 0.865 V), while exhibiting greater durability in alkaline media compared to the Pt catalyst. This heightened ORR activity was attributed to an augmented defect density and the formation of SOx bonds within the EA-SFeNG structure. Additionally, experimental evidence indicated a significant reduction in the work function of Fe–N–graphene from 4.06 eV to 4.01 eV due to the increased edge density and S doping, thereby improving the ORR kinetics of EA-SFeNG.407
4.3. HER
Due to its high energy density (140 kJ g−1) and clean combustion byproduct (H2O), hydrogen (H2) is seen as a possible future clean energy source.408 Electrochemical water reduction offers a low-cost, efficient, and environmentally friendly way to produce H2, attracting significant research interest. As mentioned earlier, graphene serves as an excellent support in electrocatalytic applications, providing improved conductivity, increased exposed active sites, and prevention of noble-metal nanostructure aggregation and leaching. Among noble-metal nanostructures, Pt-based catalysts are extensively investigated due to Pt's high intrinsic HER activity.409,410 For example, Mazzaro and colleagues conducted a study on the electrocatalytic activity of monodispersed Pt nanoparticles supported on high-quality graphene nanosheets in 0.1 M phosphate buffer with a pH of 6.8. The research revealed that these nanoparticles demonstrated remarkable hydrogen evolution reaction (HER) performance, achieving a turnover frequency of 4600 h−1 at zero overpotential. Notably, this rate was nearly three times higher than that of the commercially available Pt/C electrocatalyst.411 Ojani and collaborators worked on enhancing the number of active sites for the hydrogen evolution reaction (HER) by developing graphene-supported hollow Pt nanospheres through the use of cobalt nanoparticles as a sacrificial template.412 In comparison to solid Pt nanoparticles supported on graphene, the hollow Pt nanospheres displayed superior electrocatalytic performance for the HER. Despite Pt's excellent HER capabilities, its widespread application is hindered by its high cost and limited availability.413 Consequently, there is a persistent focus on reducing Pt usage and maximizing atom efficiency in the design of HER electrocatalysts.414–416
Many graphene-supported Pt-based nanocatalysts for the hydrogen evolution process (HER) have consequently been developed by scientists. An example is the distinctive Pd@Pt/graphene hybrid structure that Bai and colleagues414 developed, which has a changeable Pt shell thickness ranging from 0.8 to 3.2 nm. According to their research, this hybrid material's HER activity increased as the Pt shell thickness was decreased. The most active catalyst, for example, had a Pt thickness of 0.8 nm and produced a Tafel slope of 10 mV dec−1 and a phenomenal current density of up to 791 mA cm2 at −300 mV (vs. RHE).417
Pt alloys formed with nonprecious metals including Cu, Fe, Co, and Ni are an efficient way to minimise the amount of expensive Pt used and maximise hydrogen adsorption energy.418 For instance, Zhong et al. studied a PtFe alloy supported on nanoporous graphene sheets that showed improved electrochemical surface area (ECSA), more hydrogen evolution reaction (HER) activity, and superior stability when compared to Pt/C in an acidic environment. Theoretical simulations showed that the strong connection between the PtFe alloy nanoparticles and the graphene nanosheets caused a downshift in the PtFe alloy's d-band centre. This promoted charge transfer, which in turn increased the HER activity. Researchers have made advancements in creating noble metal nanocomposites using graphene for hydrogen evolution reaction (HER) applications in addition to platinum (Pt)-based catalysts. A good example of this is the PdTe/rGO nanocomposites that Jiao and colleagues created after successfully synthesizing PdTe nanowires on reduced graphene oxide (rGO) nanosheets. These materials effectively catalysed HER under alkaline conditions. The PdTe/rGO nanocomposites have a number of benefits, such as a low cost, a large surface area, and great electrical conductivity. These catalysts additionally displayed exceptional activity and long-term stability in acidic solutions. PdAu bimetallic nanoplates supported on rGO, created by Jiang et al., are yet another noteworthy example. Two unique PdAu structures, the Au@Pd core–shell structure and the Pd@PdAu alloy core–shell structure, were produced by carefully regulating the galvanic replacement and coreduction rates. The latter of the two structures on rGO demonstrated better HER performance than the former and was even on par with the commercial Pt/C catalyst. This improved performance was attributed to the PdAu alloy's cooperative effect and the PdAu nanoplates' effective electron coupling with rGO.419–426Fig. 35(a) and (b) represent three distinct Pd@Pt/graphene hybrid structures with varied Pt shell thicknesses. These distinctive hybrid configurations are shown in the diagrammatic representations and related TEM pictures, from left to right.415
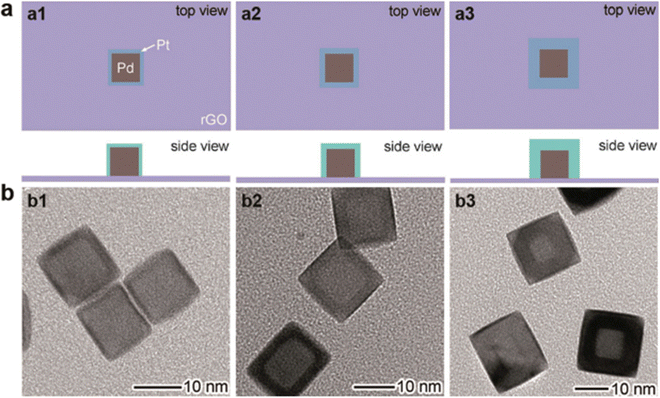 |
| Fig. 35 (a) and (b) Three distinct Pd@Pt/graphene hybrid structures with varied Pt shell thicknesses. These distinctive hybrid configurations are shown in the diagrammatic representations and related TEM pictures, from left to right.415 Reproduced (adapted) with permission from ref. 415. Copyright [2018] [Wiley]. | |
4.4. Electrochemical sensors
Graphene has demonstrated remarkable electrochemical properties, establishing itself as a highly promising electrode material for electroanalysis.427–431 The vast potential of graphene and graphene composites in the field of bioanalysis and in the field of environmental analysis has led to the development of numerous advanced electrochemical sensors.428,432 In the context of enzyme biosensors, graphene's high electrocatalytic activity towards H2O2 and its ability to facilitate direct electrochemistry of glucose oxidase (GOD) make it a magnificent electrode material. The various glucose sensors based on graphene are reported in the literature.428,429,432–434 For example, Shan et al.428 used a graphene/polyethylenimine-functionalized ionic liquid nanocomposite-modified electrode to create the first graphene-based glucose biosensor. This biosensor demonstrated a broad linear glucose response range (2 to 14 mM, R = 0.994), good reproducibility (relative standard deviation of the current response to 6 mM glucose at 0.5 V was 3.2% for ten consecutive measurements), and high stability (response current maintained at +4.9% after one week).
Another study revealed a glucose biosensor based on chemically reduced graphene oxide (CR-GO) with outstanding electrochemical performance for glucose detection. Graphene-based biosensors, such as the CR-GO-based biosensor, provide considerably increased amperometric signals for glucose sensing, with a broad linear range (0.01–10 mM), high sensitivity (20.21 mA mM cm2), and a low detection limit of 2.00 mM (S/N = 3). The GOD/CR-GO/GC electrode's linear range for glucose detection outperforms that of other carbon material-based electrodes such as carbon nanotubes435 and carbon nanofibers.436 Furthermore, the GOD/CR-GO/GC electrode's detection limit for glucose is lower than that of other previously reported carbon material-based biosensors.434,436–439 This biosensor exhibits a rapid response to glucose (91 s to steady-state response) and high stability (91% signal retention for 5 hours), making it a potential candidate for continuously measuring plasma glucose levels in diabetes diagnosis. Kang et al.432 utilized chitosan as a biocompatible dispersant to construct glucose biosensors with graphene, achieving excellent sensitivity (37.93 mA mM−1 cm2) and long-term stability for glucose measurement.
Biosensors based on graphene/metal nanoparticles (NPs) have also been created. Shan et al.440 for example, presented a graphene/AuNPs/chitosan composite film-based biosensor with high electrocatalytic activity towards H2O2 and O2. Wu et al.433 presented a glucose biosensor based on GOD/graphene/PtNPs/chitosan with a detection limit of 0.6 mM glucose. These better results were ascribed to graphene's enormous surface area and high electrical conductivity, as well as the synergistic interaction of graphene and metal nanoparticles.433,440 The catalytic activity of functionalized graphene in NADH oxidation implies that it might be used in dehydrogenase biosensors. Zhou et al. created an ethanol biosensor based on graphene-ADH, and the ADH-graphene-GC electrode outperformed the ADH-graphite/GC and ADH/GC electrodes in terms of response time, linear range, and detection limit for ethanol. These improvements are due to the efficient transfer of the substrate and products via graphene matrices containing enzymes, as well as graphene's intrinsic biocompatibility.427
Graphene-based electrochemical DNA biosensors offer high sensitivity, high selectivity, and low cost for detecting specific DNA sequences or mutated genes associated with human disease, making them a promising platform for patient diagnosis.441,442 Additionally, these biosensors allow for device miniaturization, making them suitable for small volume samples. Among various electrochemical DNA sensors, the one based on the direct oxidation of DNA is the simplest.442 Zhou et al. developed an electrochemical DNA sensor using chemically reduced graphene oxide (CR-GO).
The CR-GO/GC electrode demonstrates efficient separation of current signals from the four free bases of DNA (guanine, adenine, thymine, and cytosine), enabling simultaneous detection of all four bases. Neither graphite nor glassy carbon electrodes show this capability. The exceptional performance of CR-GO/GC is attributed to its antifouling properties and high electron transfer kinetics for base oxidation, which result from the abundance of edge-plane-like defective sites and oxygen-containing functional groups on the CR-GO surface, providing numerous active sites and facilitating electron transfer between the electrode and species in solution.415,416
Furthermore, the CR-GO/GC electrode effectively separates all four DNA bases in both single-stranded DNA (ssDNA) and double-stranded DNA (ds-DNA) at physiological pH without requiring a prehydrolysis step. This unique feature allows the detection of single-nucleotide polymorphism (SNP) sites for short oligomers with specific sequences on the CR-GO/GC electrode without the need for hybridization or labeling processes. These excellent capabilities are attributed to the physicochemical properties of CR-GO, including its single-sheet nature, high conductivity, large surface area, antifouling properties, and high electron transfer kinetics. Fig. 36 shows differential pulse voltammograms (DPV) for graphene/GC (green), graphite/GC (red), and bare GC electrodes in 0.1 M pH 7.0 PBS for a combination of DNA free bases (G, A, T, and C), ssDNA, and dsDNA. G, A, T, C, ssDNA, or dsDNA concentrations: 10 mg ml−1.401
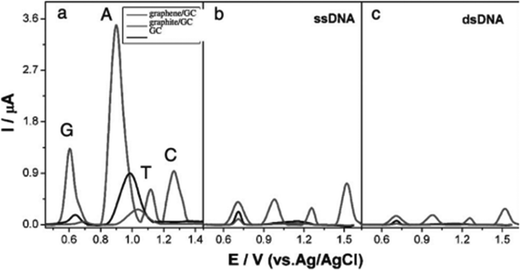 |
| Fig. 36 Differential pulse voltammograms (DPV) for graphene/GC (green), graphite/GC (red), and bare GC electrodes in 0.1 M pH 7.0 PBS for a combination of DNA free bases (G, A, T, and C), ssDNA, and dsDNA. G, A, T, C, ssDNA, or dsDNA concentrations: 10 mg ml−1.401 Reproduced (adapted) with permission from ref. 401. Copyright [2013] [Wiley]. | |
4.4.1. Graphene-based electrochemical sensors for heavy metal ions.
For environmental analysis, graphene-based electrochemical sensors have been developed, especially for the detection of heavy metal ions such as Pb2+ and Cd2+. Li et al.443,444 demonstrated that Nafion–graphene composite film-based electrochemical sensors not only have enhanced sensitivity for detecting metal ions (Pb2+ and Cd2+), but they also have reduced interferences owing to the synergistic impact of graphene nanosheets and Nafion.443 On graphene electrodes, the stripping current signal is greatly improved. The detection range for Pb2+ and Cd2+ is broad (0.5 mg L−1 −50 mg L−1 for Pb2+ and 1.5 mg L−1 −30 mg L−1 for Cd2+). The detection limits (S/N = 3) for both Cd2+ and Pb2+ are 0.02 mg L−1, showing that Nafion–graphene composite film-based electrodes are more sensitive than Nafion film-modified bismuth electrodes445 and the ordered mesoporous carbon-coated GCE,446 but equivalent to Nafion/CNT-coated bismuth film electrodes.447 The improved performance is due to graphene's unique features, such as nanosized graphene sheets, nanoscale thickness of these sheets, and strong conductivity. These features enable graphene to strongly adsorb target ions, increase surface concentration, improve sensitivity, and reduce surfactant fouling.443,444,448,449
4.4.2. Electrochemical behavior of GCE modified with ErGO/PEDOT:PSS.
The hydrazine sensors were made using a poly(3,4 ethylenedioxythiophene):poly(styrenesulfonate) (PEDOT:PSS) composite and electrochemically reduced graphene oxide (ErGO) placed onto a glassy carbon electrode (GCE). Fig. 37a depicts the electrochemical behaviour of 1.5 × 103 M hydrazine in phosphate buffers at pH 7 and pH 10 and Fig. 37b depicts cyclic voltammograms of 0.1 M pH 7 phosphate buffer and 1.5 × 103 M hydrazine in 0.1 M phosphate buffer at pH 7, obtained with the bare GCE and ErGO/PEDOT:PSS/GCE. The maximal oxidation potential of hydrazine at pH 7, with ErGO/PEDOT:PSS/GCE electrodes, was found to be 0.34 V versus Ag/AgCl, as opposed to 0.39 V vs. AgCl at pH 10. Furthermore, the peak current for hydrazine oxidation was 76 A at pH 7, and 74 μA at pH 10. Based on the potential values obtained, the hydrazine oxidation employing ErGO/PEDOT:PSS/GCE is more favourable at pH 7 than at pH 10. As a result, pH 7 phosphate buffer was used for the following studies. To learn more about the electrochemical behaviour of the modified electrode, cyclic voltammograms were recorded at a scan rate of 100 mV s−1 using 0.1 M pH 7 phosphate buffer as an electrolyte and 1.5 × 103 M hydrazine in the electrolyte. Under comparable experimental circumstances, the voltammograms produced with the naked GCE and ErGO/PEDOT:PSS-modified GCE were compared. A minor signal was found while measuring 0.1 M pH 7 phosphate buffer with the bare GCE, as shown in Fig. 3b. When the ErGO/PEDOT:PSS-modified GCE was used to measure 1.5 × 103 M hydrazine in a pH 7 electrolyte, a greater background current was detected than with the naked GCE.
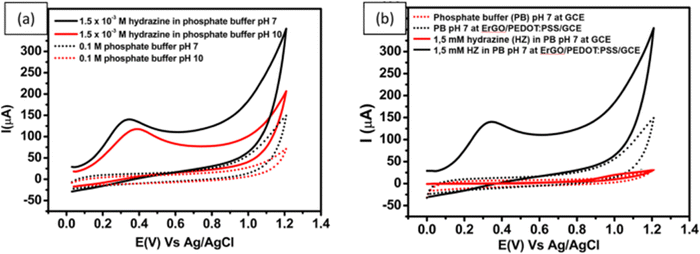 |
| Fig. 37 (a) Cyclic voltammograms of 1.5 × 103 M hydrazine in phosphate buffer at pH 7 and pH 10, obtained with ErGO/PEDOT:PSS/GCE; (b) cyclic voltammograms of 0.1 M pH 7 phosphate buffer and 1.5 × 103 M hydrazine in 0.1 M phosphate buffer at pH 7, obtained with the bare GCE and ErGO/PEDOT:PSS/GCE.449 | |
This outcome was predicted since the alteration of the GCE surface with ErGO/PEDOT:PSS was expected to cause changes in surface morphology, resulting in increased surface roughness and the formation of more electroactive sites. This increase in electroactive sites and better surface roughness add to the ErGO/PEDOT:PSS composite's increased conductivity and electrocatalytic activity, resulting in a larger background current. Furthermore, using the ErGO/PEDOT:PSS-modified GCE to detect 1.5 × 103 M hydrazine led the oxidation peak to move to a potential of 0.33 V versus Ag/AgCl, as opposed to 1.01 V vs. Ag/AgCl seen with the naked GCE. Furthermore, the peak current intensity (Ipa) for hydrazine oxidation observed with the ErGO/PEDOT:PSS-modified GCE was 30 times greater than that with the naked GCE. This considerable increase in Ipa and hydrazine oxidation at a lower applied voltage can be attributed to the synergistic effects of ErGO/PEDOT:PSS composite's greater surface area and better electron transport to the surface of modified electrodes. The enhanced electrocatalytic activity of the ErGO/PEDOT:PSS composite is also due to the nature of hydrazine as an electron donor, which facilitates charge transfer processes on the modified electrode's surface. Based on the findings, the ErGO/PEDOT:PSS composite is well-suited for electrocatalytic and sensing applications, notably hydrazine detection.450
4.5. CO2RR
It is well acknowledged that one of the main causes of global warming is the buildup of CO2 in the atmosphere, which is mostly brought on by the usage of fossil fuels. The process of CO2RR (carbon dioxide reduction reaction), which converts CO2 to renewable fuels, is one potential remedy for this problem. Due to its controllability and simplicity, electrochemical CO2 reduction has become one of the most used methods. In comparison to other electrochemical reactions, the CO2RR is a more complicated process since it can proceed along two-, four-, six-, or eight-electron reduction pathways, resulting in the generation of a variety of gaseous and aqueous products such as carbon monoxide, methane, ethane, methanol, ethanol, and formate. The CO2RR poses difficulties, particularly given that the equilibrium potentials of a few key products are similar and nearly identical to those of the hydrogen evolution process (HER). Therefore, it is very difficult to selectively reduce CO2 to desired compounds without concomitant HER.
Researchers have looked into the use of noble-metal nanoparticles, which have demonstrated promising applications in the CO2RR, to address these issues. When noble metal nanoparticles are combined with graphene, the catalytic activity is improved by lowering the overpotential and raising the electrochemically active surface area (ECSA). For instance, to further enhance the CO2RR process, Rogers et al. used narrow graphene nanoribbons (GNRs) as a support for Au nanoparticles (AuNPs).451Fig. 38a, b, and c show the three separate Au/GNR composites that were created, labelled as 1-AuNP, 2a-AuNP, and 2b-AuNP, respectively. Chevron-GNRs are designated as “1”, whereas ordinary cove-GNRs and ester-functionalized cove-GNRs are designated as “2a” and “2b”, respectively. All three types of GNRs were uniformly loaded with Au nanoparticles that had a size of about 8 nm. Although the Brunauer–Emmett–Teller surface areas of these Au/GNR nanocomposites were relatively similar to those of the carbon-black-Au nanoparticles (Cblack-AuNPs), they showed significantly higher ECSA. All three Au/GNR composites outperformed the Cblack-AuNPs in terms of partial currents (Fig. 38d) and Faraday efficiencies for CO generation. This enhanced performance was attributed to the growth of Mott–Schottky heterojunctions at the interface between the GNRs and the AuNPs.
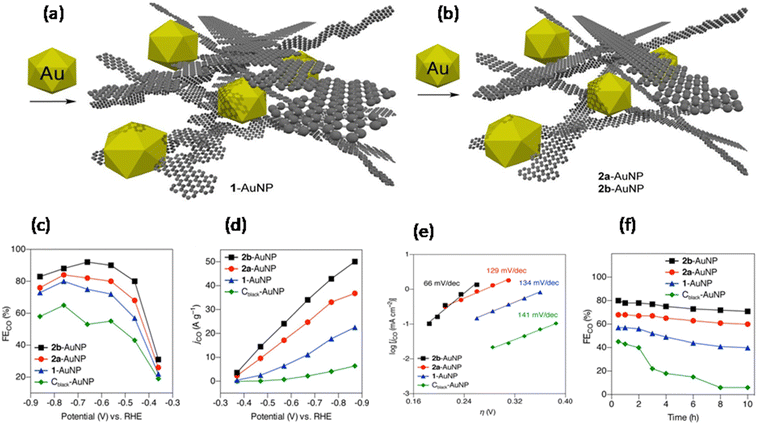 |
| Fig. 38 Illustration of the schematic representations of nanocomposites 1-AuNP (a) and 2-AuNP (b). The faradaic efficiency (c), current (d), Tafel slope (e), and stability (f) were studied for CO production using composite electrodes of 1-AuNP (blue triangles), 2a-AuNP (red circles), 2b-AuNP (black squares), and carbon-black-AuNP (green diamonds).450 Reproduced (adapted) with permission from ref. 450. Copyright [2017] [ACS]. | |
When compared to the Cblack-AuNPs, the Tafel slopes of 2a-AuNP and 1-AuNP were similar, while 2b-AuNP had a much smaller Tafel slope (66 mV dec−1) (Fig. 38e). A new reaction mechanism was implied by such a discrepancy, which pointed to a discrete rate-limiting phase. The inclusion of methyl carboxylate groups on the GNRs, which stabilised the transition state of the electron-transfer step and changed the reaction mechanism, might be attributed, based on the experimental data, to the improved activity and selectivity of 2b-AuNP. In addition, as compared to the Cblack-AuNPs, all three Au/GNR nanocomposites showed improved stability (Fig. 38f).
In addition to single-element catalysts like Au, noble-metal alloys have also been investigated as CO2RR catalysts. For instance, PdIn nanoparticles wrapped in 3D rGO (PdIn/3D-rGO) were created by He et al., which showed lower overpotential and higher current density452 than PdIn nanoparticles on 2D rGO. Furthermore, PdIn/3D-rGO catalysts performed better than both Pd/3D-rGO and In/3D-rGO catalysts, demonstrating a synergistic interaction between Pd and In. There is currently no experimental report on graphene-based single-atom catalysts for the CO2RR, despite the remarkable efficiency of graphene-based single-atom catalysts in many electrochemical processes. However, there have been some published theoretical studies. A porphyrin-like metal-functionalized graphene structure, for instance, was theoretically studied by Tripkovic et al.453 for a selective CO2RR to methane and methanol. They found that single noble-metal atoms behaved differently from their nanoparticle counterparts, and that the HER predominated the catalytic reaction when late transition metals like Ag, Au, Pd, Pt, Rh, Ir, Ru, and Os were used as electrocatalysts as opposed to the CO2RR. He and Jagvaral454 discovered that when graphene with imperfections served as the support for a single-atom catalyst, CO2RR was favoured over HER. On graphene with imperfections, they found that five single-metal atom catalysts, including Ag, Cu, Pd, Pt, and Co, might increase the rate-limiting potential to produce C1 hydrocarbons. It should be noted that single Ag atoms exhibited the lowest overpotential, while single Cu atoms might favourably produce methanol.455
Ammonia (NH3) plays a vital role in the chemical industry as a base product, finding extensive applications in both industry and agriculture. It serves as a crucial precursor for the production of HNO3 and urea, making it indispensable for manufacturing fertilizers to enhance agricultural yields and meet the growing demand for food due to the increasing world population. Another significant advantage of ammonia lies in its potential as a hydrogen carrier, boasting a high hydrogen content of approximately 17.65 wt% and requiring only mild storage conditions (25 °C, ≈9–10 bar).456 However, due to the stability of the N
N bond, achieving satisfactory yields necessitates severe reaction conditions. Traditionally, ammonia synthesis relies on the Haber–Bosch (HB) process, which presently accounts for 1–3% of the total global energy consumption and contributes to environmental pollution through carbon dioxide emissions.457 Therefore, there is a pressing need to develop a clean and environmentally friendly ammonia production process to replace the HB method in the long run.
4.6. NRR
Because of their unique properties, such as a large surface area, tunable porosity, various types of defects, high conductivity, and exceptional stability, graphene and graphene-derived materials have piqued the interest of researchers as potential catalysts for the nitrogen reduction reaction (NRR).458 These characteristics allow for more effective mass and electron transmission, resulting in more active sites for NRR enhancement.459,460 The electrical and structural characteristics of graphene may be altered by managing the flaws in its backbone, hence increasing its catalytic activity. Doping elements such as nitrogen, boron, oxygen, sulphur, phosphorus, and fluorine, as well as changing vacancies, can boost graphene's NRR activity.459–466Fig. 39 is a schematic representation of graphene functionalization methods used in the construction of catalysts for the electrochemical reduction of nitrogen to ammonia.467
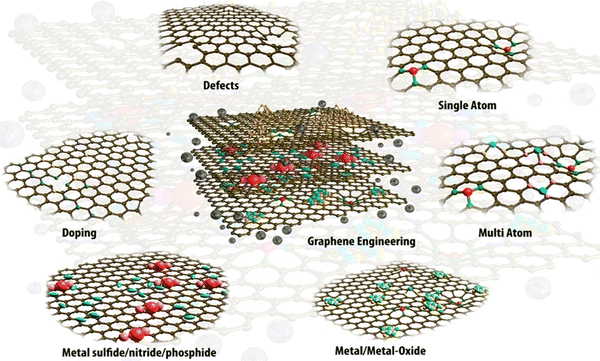 |
| Fig. 39 Schematic representation of graphene functionalization methods used in the construction of catalysts for the electrochemical reduction of nitrogen to ammonia.467 Reproduced (adapted) with permission from ref. 467. Copyright [2021] [ACS]. | |
As previously noted, various studies in the literature have investigated the anchoring of zero-dimensional (0D) defects, such as atom doping, in graphene to improve its electrocatalytic activity.468 One-dimensional (1D) defects, represented by edges, were also shown to alter graphene's catalytic activity, with the coordination nature of both 0D and 1D defects having a major impact on overall performance. Introducing flaws into graphene doped with mono- or multi-heteroatoms has emerged as a viable technique for increasing NRR activity.469
A recent work used defect engineering to change the surface of reduced graphene oxide (rGO) using tannic acid (TA-rGO/CP) to increase NRR activity. Fig. 40a depicts the NH3 yield rate (RNH3) and the related faradaic efficiencies (FEs) of TA-rGO/CP at a potential of −0.75 V. Fig. 40b depicts the total quantity of ammonia produced (mNH3) with various catalysts after 2 hours of electrolysis at a potential of −0.75 V. The defect-rich carbon was stable enough as an electrocatalyst, providing an RNH3 of 17.02 g h−1 mg−1 cat. in 0.5 M LiClO4, with an optimized FE of around 4.83% (Fig. 40c and d).
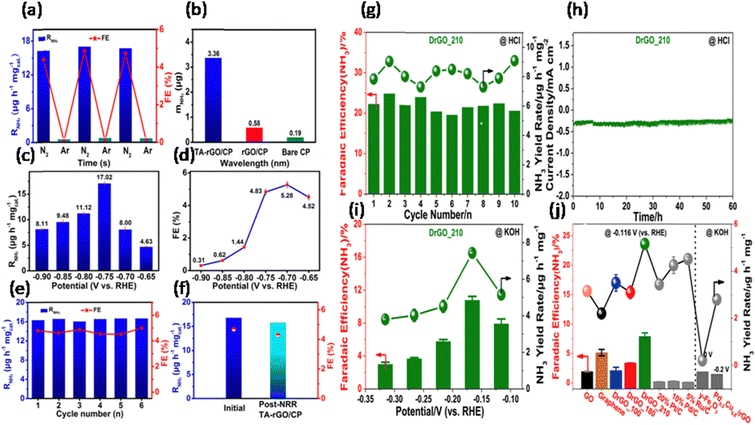 |
| Fig. 40 (a) The yield rate of ammonia (RNH3) and related faradaic efficiencies (FEs) of the TA-rGO/CP catalyst at a potential of approximately 0.75 V. (b) The amount of ammonia produced during a two-hour electrolysis using various catalysts at a potential of 0.75 V. The faradaic efficiencies of TA-rGO/CP for the NRR at different potentials are depicted in (c and d), along with a graphical representation of the variance in ammonia yield rate. (e) Demonstrates the stability performance of TA-rGO/CP as the catalyst for repeated NRR catalysis at a potential of 0.75 volts. An evaluation of RNH3 and FEs at a potential of 0.75 V before and after a two-hour NRR with TA-rGO/CP as the catalyst is shown in (f).469 Reproduced (adapted) with permission from ref. 469. Copyright [2019] [ACS]. Furthermore, in the stability investigation, panel (g) shows NH3 yield rates and computed faradaic efficiencies, and panel (h) presents a chronoamperometry study at a potential of 0.116 V on a DrGO_210 electrode. Panel (i) displays DrGO_210's potential profile in a KOH electrolyte at various potentials, while panel (j) provides the yield rates and computed faradaic efficiencies of NH3 from different electrodes at a potential of 0.116 V in a 0.1 M KOH electrolyte that has been purged with N2 gas.470 Reproduced (adapted) with permission from ref. 470. Copyright [2021] [ACS]. | |
Fig. 40e also depicts the stability test for TA-rGO/CP under repeated NRR at −0.75 V. Fig. 40f depicts the change in RNH3 and FEs after 2 hours of catalysis at a potential of −0.75 V, which corresponds to the NRR for TA-rGO/CP. This study indicated that employing organic compounds to change a carbon network is a viable way to improve its N2-fixing capabilities.471
Another example of defect engineering is the molten salt approach of creating faulty graphene without any doping element to improve N2 adsorption for ammonia synthesis.472 Experiments and DFT simulations demonstrated that defect locations were the active sites for N adsorption and activation. The entry of the N dopant from NH3 into the faulty graphene framework as a result of the NRR was shown, highlighting the critical importance of defect sites for activating N2.472
By modifying flaws in rGO, metal-free electrocatalysis of aqueous N2 to synthesize NH3 has also been demonstrated.466 Due to significant N2 binding but not H, the defect sites, which comprised −COOH, double vacancy (DV), and single vacancy (SV) unsaturated carbon sites, displayed increased selectivity towards NH3. Furthermore, computed free energies for the N2 reduction process to defect-rich rGO, −COOH, and defect-rich rGO-DV indicated that the thermodynamic overpotentials of graphene-based metal-free catalysts were as good as those previously reported for the most effective transition-metal (TM)-based catalysts. These metal-free, rGO-based, dopant-free catalysts achieved a FE of roughly 22.0% against RHE in 0.1 M HCl and 10.8% against RHE in 0.1 M KOH (Fig. 40g–j). These numbers are good compared to past reports. Furthermore, at low overpotentials, NH3 emission rates larger than 7.3 g h−1 mg−1 were achieved in both alkaline and acidic environments.466,473–476
4.7. Supercapacitors
Supercapacitors have garnered significant attention due to their potential to meet current and future energy demands. They possess attributes such as rapid charge and discharge rates, extended lifespan, simple geometry, and eco-friendly nature, making them a viable alternative or complement to batteries. This emerging technology is also compatible with flexible and wearable electronics.477–480 Supercapacitors find application in hybrid electric vehicles due to their incredibly short charging time. Moreover, their utility extends beyond road transportation and encompasses aerospace, industrial, and electronic gadget sectors. Supercapacitors can be classified as electric double layer capacitors, which store energy electrostatically, and pseudocapacitors, which store energy through redox reactions. Hybrid electrodes combine both electrochemical and electrostatic processes to store energy.481,482
Although extensive research has been conducted on supercapacitors, their widespread adoption remains limited. The composition and shape of the electrode have the greatest effect on supercapacitor performance.483,484 Supercapacitors may charge and discharge quickly due to energy storage at the electrode and electrolyte interface. They have a high power density of about 10 kW kg−1 and a long life of over 100
000 cycles. Their energy density, on the other hand, is a significant issue, with significantly lower levels.485 The essential components, such as electrolyte solutions, binders, and electrode materials, have a substantial influence on supercapacitor performance.486
Two electrodes, a separator, an electrolyte, and a covering body or container make up a supercapacitor (Fig. 41).
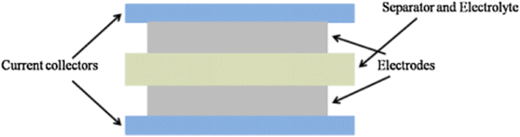 |
| Fig. 41 Internal structure of a supercapacitor.487 Reproduced (adapted) with permission from ref. 487. Copyright [2021] [Elsevier]. | |
Because of their outstanding electrical conductivity and huge surface area, graphene and its composites have been extensively researched as possible electrode materials. There are numerous forms of graphene-based electrodes, including pure graphene, graphene composites with metal oxides, conductive polymers, ferrites, and other sophisticated materials.488–491 Graphene-based electrodes have received a lot of interest because of their amazing conductivity, optimum power density, and exceptional mechanical qualities. However, graphene has significant drawbacks, such as low capacitance and low energy density, since it stores energy predominantly through electrostatic adsorption and desorption processes. To overcome these limitations, researchers have turned to the development of graphene composites, resulting in various hybrid materials that have demonstrated extraordinary results for supercapacitor applications.492–499 Intensive research efforts have been devoted to enhancing the energy density, capacitance value, and cyclic efficiency of supercapacitors, leading to numerous reports on this subject. Graphene-based electrodes have played a crucial role in realizing efficient supercapacitors.
Efficient and stable electrode materials are required for use in supercapacitors. Although graphene has a low specific capacitance because it stores charge mostly on the electrode's surface in the electric double layer (EDL), it cannot offer the requisite energy and power density on its own. Therefore, fabricating composite electrodes by combining two different materials to leverage their strengths and mitigate their shortcomings has been a logical approach.500–505 Composite electrodes have shown remarkable performance in supercapacitors. Among the various composite options, graphene with conducting polymers is considered a novel and promising electrode material for supercapacitors.504 Additionally, conducting polymers can find utility in flexible electronic devices due to their high specific capacitance, rapid charge–discharge behavior, and excellent mechanical flexibility. However, they do have some limitations, such as poor electron transport ability and stability issues. By embedding graphene within the polymer network, the electrochemical performance of conducting polymers can be significantly improved.
The optimum graphene-to-conducting polymer ratio must be determined in order to obtain maximal capacitance and longer cycle life in composite electrodes. A polyaniline–graphene composite with a 1
:
1 ratio, for example, has a specific capacitance ranging from 300 to 500 F g−1 (Fig. 42).504 The use of an ambipolar conducting polymer in conjunction with vertically standing graphene demonstrated potential supercapacitor properties.505,506 This composite solved the issue of conducting both holes and electrons, resulting in an 88% increase in cyclic life after 10
000 cycles. The polymer-coated vertically standing graphene sheets had several electrolyte access sites, resulting in a significant capacitance increase that was roughly 5 times larger (62.8 mF cm−2) than that of vertically standing graphene alone. Another good example is the polyaniline/reduced graphene composite electrode, which functions as a high-performance flexible electrode for supercapacitors in complex systems.507 After 2000 cycles, it had a capacitance value of around 299 F g−1 and a capacitance retention of 88.5%.507 Furthermore, in a 1 M H2SO4 electrolyte, a hydrothermal-assisted porous polyaniline and reduced graphene oxide composite electrode demonstrated a high specific capacitance of 420 F g−1.508 A polyaniline/reduced graphene oxide nanocomposite electrode generated by a diffusion-driven layer-by-layer approach had a specific capacitance of 438 F g−1. The high electrochemical performance of these composites is due to the effective connection between the included graphene and the polymer network. Finally, in a 1 M H2SO4 electrolyte, a hydrothermally reduced porous graphene–polyaniline nanofiber composite demonstrated a specific capacitance value of 357 F g−1.509,510 These examples demonstrate the great potential of graphene–conducting polymer composites as high-performance supercapacitor electrodes.
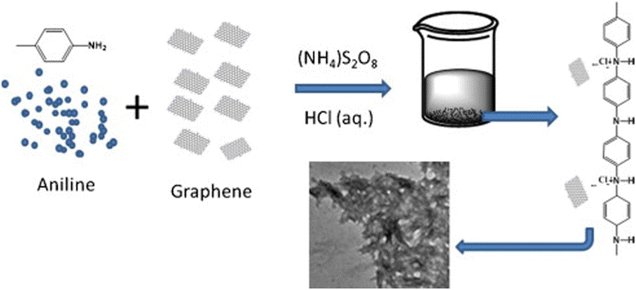 |
| Fig. 42 Schematic of the synthesis process of the graphene and conducting polymer composite electrode.504 Reproduced (adapted) with permission from ref. 504. Copyright [2011] [Elsevier]. | |
High-surface-area graphene–polyaniline nanocomposites, with a specific capacitance value of 257 F g−1 in a 1 M H2SO4 electrolyte solution, were used as high-performance supercapacitor electrodes. Polyaniline grafted reduced graphene oxide yielded a specific capacitance of 250 F g−1 in the same electrolyte. The combination of graphene with conducting polymers increases the electrical conductivity and mechanical toughness of the composite. As a result, the final composite not only has a higher capacitance but also retains it well during charging and discharging cycles. The polyaniline and graphene nanoribbon nanocomposite proved to be an effective electrode for supercapacitors with a specific capacitance of 431 F g−1 in a 1 M H2SO4 solution. Graphene composites with conducting polymers considerably improve electrochemical properties. Electrodes formed by deposition of polyaniline on graphene paper demonstrated extremely high specific capacitance values ranging from 22 to 64 mF cm−2, depending on the amount and thickness of deposition.511–514
After 2000 cycles, nylon-6/reduced graphene oxide/polyaniline nanocomposites had a specific capacitance of 38 F g−1 at 100 mV s−1 and a cyclic retention of 98%.515 The capacitance of graphene and polyethylenedioxythiophene (PEDOT) nanocomposites at 0.1 A g−1 was 374 F g−1.516 Layered reduced graphene oxide and polyindole composite materials had improved electrochemical properties, such as a high specific capacitance of 322.8 F g−1 at 1.0 A g−1 and a retention rate of 94.5% after 1000 cycles.517 ZnS/rGO/PANI-based composites demonstrated high capacitive performance after 1000 cycles, with a capacitance of 1045.3 F g−1 and a cyclic stability of 160% at 1 A g−1.518 The specific capacitance of reduced graphene oxide modified by a conducting polymer by hydrothermal polymerization was 202.7 F g−1.519 The good synergetic effect of PEDOT and rGO allowed for great stability and reversibility during the charge and discharge process, with a specific capacitance value of 1286 F g−1 and 95% retention after 6000 cycles.520
The PVA-GQD (graphene quantum dots)/PEDOT nanocomposite displayed good stability, maintaining 98% after 1000 cycles and a specific capacitance of 291.86 F g−1 at 2.0 A g−1.521 The increased capacitance is caused by quick ion transport between the electrolyte and the electrode. The GO/PPy/PANI ternary composite demonstrated outstanding cyclic retention of 91% after 2000 cycles as a binder-free electrode for a flexible solid-state symmetric supercapacitor.522 It had a specific capacitance of 131 F g−1 measured at an 8 A g−1 current. The combination of graphene paper electrodes with an ionic liquid and PVA polymer resulted in the construction of a solid-state supercapacitor. After 6000 cycles, it had a specific capacitance of 222.96 F g−1 and a capacitance retention of 60%.523,524
4.8. Adsorption and oxidation of organic pollutants
Fig. 43 presents the structure of (a) graphene and (b) graphene oxide.525 Because of their adsorption, oxidation, and catalytic capabilities, graphene, graphene oxide (GO), and their composites have been widely used in wastewater treatment. Their huge pore capacity, excellent conductivity, complex surface chemistry, and very wide aspect ratio make them ideal for adsorption of organic pollutants in wastewater and catalysis. The sheet-like, resonating, polyaromatic system of graphene subsidiaries plays an important role in facilitating interactions, hydrogen bonding, and/or electrostatic interactions with organic pollutants found in wastewater, such as dyes, pharmaceutical waste, and agricultural and industrial effluents, all of which contain highly reactive unsaturated aromatic rings and oxygen-rich functional groups526–528
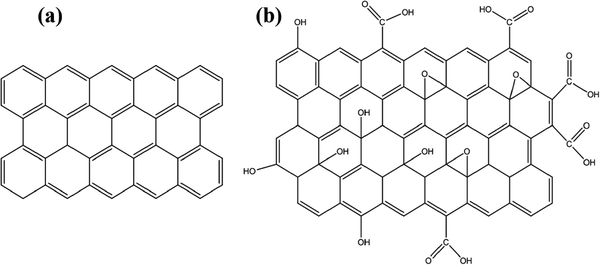 |
| Fig. 43 Structure of (a) graphene and (b) graphene oxide.525 Reproduced (adapted) with permission from ref. 525. Copyright [2019] [ACS]. | |
Graphene-based wastewater treatment of organic contaminants has been widely researched and well-documented.529 The combination of graphene with various metallic or nonmetallic entities creates new composites or improves the effectiveness of existing materials for pollutant adsorption, oxidation, or catalysis, resulting in their disintegration into harmless constituents or easy separation via techniques such as filtration, magnetic separation, or a simple solvent wash. The adaptability of graphene-based composites in tackling a wide variety of contaminants separately makes them a viable alternative for overcoming the shortcomings of present wastewater treatment systems. Graphene-based materials have demonstrated enhanced usability in adsorption,530,531 advanced oxidation532,533 and catalysis for the removal of diverse organic pollutants, including polyaromatic hydrocarbons (PAH) and their derivatives, dyes, pesticides and pharmaceutical waste such as antibiotics as well as other organic entities generated during the manufacturing processes of various industries.534–539
PAHs are known to have harmful effects on human health, being linked to skin, blood, bladder, and liver cancer540,541 as well as cardiovascular diseases.542 Monocyclic aromatic hydrocarbons like toluene, xylene, and benzene also pose a threat to human well-being as they affect the central nervous system and its activity.543 These compounds are often released into the environment due to improper waste disposal, leaks, accidents, etc., mainly originating from industrial activities. Other organic pollutants, such as bisphenol A (BPA), phenols, biphenyls, anthracene, pyrene, phenanthrene, and fluoranthene, are also disturbing the aquatic environment and presenting health problems.544,545 Pharmaceutical waste, which contains medications such as aspirin, acetaminophen, dorzolamide, ketoprofen, and ciprofloxacin, is also harmful to the environment and human health since it is developed for biocompatibility and is difficult to remove and biodegrade even when present at low quantities.546 Dye and pigments, such as methylene blue (MB), methyl orange (MO), and reactive black, are another class of aromatic hydrocarbons that pollute the environment by changing the colour of water and blocking sunlight.547Fig. 44 displays the structures of some of these pollutants.
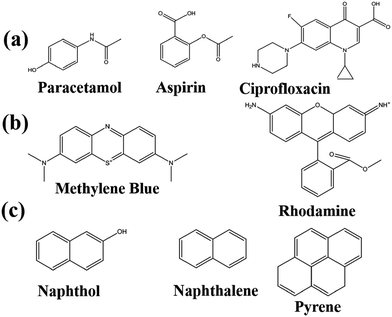 |
| Fig. 44 The structures of some of the pollutants from (a) pharmaceuticals, (b) dyes, and (c) other chemical substances.525 Reproduced (adapted) with permission from ref. 525. Copyright [2019] [ACS]. | |
Adsorption using graphene-based materials and their metal oxide composites has played a crucial role in the removal and management of pharmaceuticals, dyes, and other organic pollutants. Research on antibiotics found that the quantity of aromatic rings in the antibiotics impacted the adsorption rate on carbon-based materials. Adsorption of ofloxacin (OFL), sulfadiazine (SD), sulfamethoxazole (SMX), sulfamethazine (SMZ), cephalexin (CFX), and tetracycline (TC) with various degrees of aromatic rings was achieved using graphene-based materials. The following examples illustrate the adsorption of aromatic-based substances and dyes by graphene-based materials.
Acid Orange 8 (AO 8) and Direct Red 23 (DR23), both ionic azo dyes, were studied for adsorption from aqueous solutions. The Langmuir and Redlich Peterson models accurately reflected the adsorption isotherms, with maximal adsorption capacities of 29 mg g−1 and 15.3 mg g−1, respectively. For both colours, the adsorption followed a pseudo-second-order model, and the reaction was spontaneous. The adsorption mainly occurred due to electrostatic interactions at pH less than 7, with possibilities of hydrogen bonding and π–π stacking as well.273 GO adsorbed methylene blue (MB) at pH 6 and 298 K, showing an adsorption intensity of 714 mg g−1 with an efficiency of 99%. The adsorption was influenced by pH, ionic strength, and dissolved organic matter, with preference for low temperatures and high pH, as supported by the Freundlich isotherm. Another study observed MB adsorption by GO at pH 3 and 298 K, with an adsorption capacity of 1.939 mg g−1 according to the Langmuir adsorption model, and the process was found to be exothermic. An increase in pH enhanced the dye adsorption onto GO. Fig. 45 illustrates this adsorption process. Exfoliated GO demonstrated excellent adsorption intensity for cationic dyes (MB, RhB, MV) due to the presence of oxygen functional groups. The observed adsorption capacities were 17.3 mg g−1 for MB and 2.47 mg g−1 for MV (both at pH 6), and 1.24 mg g−1 for RhB (at pH 10).
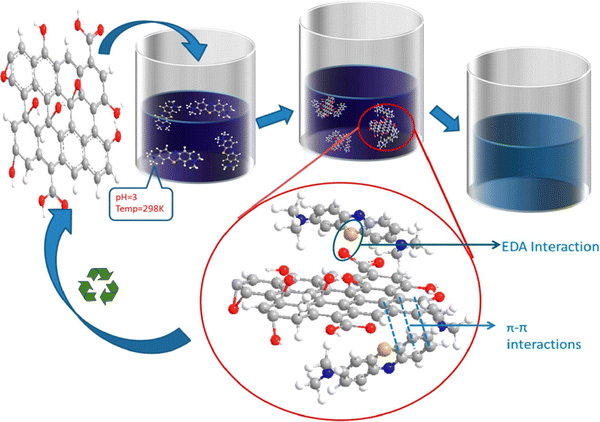 |
| Fig. 45 The EDA interaction between the sulphur (yellow) of MB and the oxygen (red) of GO, as well as the interaction between the aromatic rings, is highlighted in the adsorption of methylene blue on GO.548 Reproduced (adapted) with permission from ref. 548. Copyright [2019] [ACS]. | |
During the adsorption process in the basic media, rhodamine B (RhB) displayed both negative and positive charges in its structure. Graphene oxide (GO) displayed outstanding adsorption characteristics at 298 K and pH 6, with an extent of adsorption of 243.90 mg g−1 after 5 hours of contact time. Adsorption occurred in a monolayer, following the Langmuir adsorption isotherm, and was driven by electron exchange.549In situ GO reduction using sodium hydrosulfide led to improved acridine orange adsorption capabilities, with reported adsorption capacities of 3333 mg g−1 and 1428 mg g−1. GO effectively removed Malachite green (MG) from solutions with varying pH (4 to 9) at 298 K, showing adsorption intensities ranging from 4.821 to 7.613 mmol g−1.550 GO also adsorbed Basic Blue 41 (BB41), Basic Red 18, and Basic Red 46 (BR18, BR46) from solutions at 298 K and at an initial concentration of 5 mg L−1, with reported adsorption intensities of 1429 mg g−1, 1250 mg g−1, and 476 mg g−1, respectively. The Langmuir adsorption isotherm indicated that the reaction was classified as chemisorption. The extent of adsorption varied with the degree of oxidation of GO, resulting in adsorption capacities ranging from 40.6 mg g−1 to 570.4 mg g−1 for six differently oxidized GO samples at pH 7, 298 K, and a contact time of 15 minutes551 Another instance involved the synthesis of GO with various levels of oxidation, which was discovered to be pH independent and demonstrated significant affinity for methylene blue (MB) in water. Increased GO oxidation led to an increase in adsorption, and electrostatic interactions caused the binding to transition from parallel stacking to vertical stacking.552 For GO synthesised using the modified Hummers–Offeman technique, the amount of adsorption was found to be 351 mg g−1 for MB and 248 mg g−1 for Malachite green (MaG).
Nano-functionalized Fe3O4(NH2–Fe3O4) particles were incorporated into superparamagnetic GO–Fe3O4 nanocomposites to enhance the removal of methylene blue (MB) and neutral red (NR) from wastewater. The adsorption intensities for MB and NR were reported as 167.2 mg g−1 and 171.3 mg g−1, respectively. The addition of NH2–Fe3O4 particles prevented agglomeration and improved the composite's efficiency in wastewater treatment. Another composite, graphene–sand, immobilized with asphalt, effectively removed rhodamine 6G (R6G) with an adsorption capacity of 75.4 mg g−1 at 303 K and 6 hours of contact time. This composite also exhibited removal efficiency for the pesticide chlorpyrifos. In another study, the same graphene–sand composite demonstrated an adsorption intensity of 55.5 mg g−1 for R6G with an 8 hour contact time and could be easily regenerated using acetone.553,554
GO-wrapped magnetite nanoclusters (Fe3O4@GO) were prepared, forming a hybrid core–shell nanostructure through weak electrostatic attraction between negatively charged GO and Fe3O4. The composite effectively adsorbed cationic dyes methylene blue (MB) and rhodamine B (RhB) as well as the anionic dye methyl orange (MO), at the rates of 131.10 mg g−1, 34.50 mg g−1, and 39.95 mg g−1, respectively, at 303 K. The adsorbent could be easily separated by magnetic separation and demonstrated excellent regeneration and stability (Fig. 46). Additionally, rGO supported ferrite exhibited efficient removal of 92% RhB and 100% MB. The ferrites, which could be Mn, Zn, Co, or Ni, were homogeneously distributed over rGO.
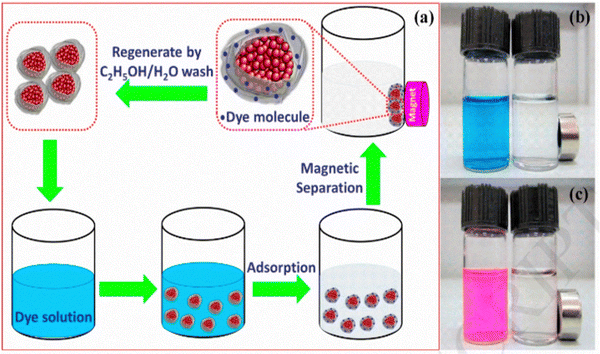 |
| Fig. 46 (a) Schematic illustration of RhB and MB adsorption using Fe3O4@GO and catalyst recycling through magnetic separation and ethanol/water washing afterward. Images of the dye solutions for (b) MB and (c) RhB before and after the adsorption procedure and the separation of the adsorbent.555 Reproduced (adapted) with permission from ref. 555. Copyright [2018] [Elsevier]. | |
In another study, the adsorption of rhodamine B (RhB) was compared between graphene oxide (GO) and Bi2O3@GO as shown in Fig. 47. At 408 K and pH 4, using 5 mg of adsorbent, the adsorption capacity increased from 64% to 80.7% for GO and Bi2O3@GO, respectively, within 65 minutes. The adsorption process followed both Langmuir and Temkin isotherms, as well as pseudo-first-order and intraparticle diffusion models, effectively.556
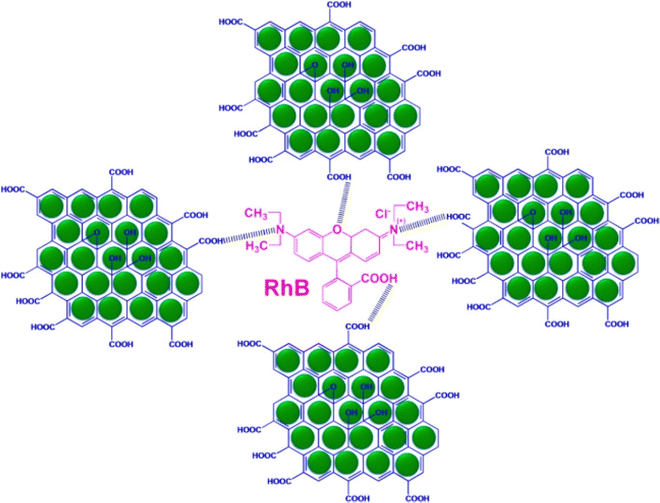 |
| Fig. 47 Illustration of the binding mechanism of the rhodamine B (RhB) dye during the adsorption process of the Bi2O3@GO composite.556 Reproduced (adapted) with permission from ref. 556. Copyright [2018] [Elsevier]. | |
Graphene-carbon nanotube hybrids (G-CNT) with a cylindrical shape generated by self-assembly were used to efficiently remove methylene blue (MB). Based on the Freundlich adsorption isotherm, the adsorption capacity of MB was calculated to be 81.97 mg g−1 after 3 hours of contact time, yielding an efficiency of 97%. Adsorption of MB and RhB onto Cu2O–graphene and Mg(OH)2–graphene composites was also observed. Cu2O–graphene was created by heating graphene and copper ions in the presence of glucose, whereas Mg(OH)2–graphene was created via chemical deposition and possessed a mesoporous structure. A sonochemically synthesized GO-[Zn2(oba)2(bpfb)]·[(DMF)5] metal–organic framework nanocomposite (GOTMU-23) for MB eradication showed an excellent 90% elimination efficiency in 2 minutes.557
4.9. OER
Water electrolysis stands as one of the most efficient and reliable methods to produce hydrogen from renewable energy sources like solar, wind, and hydropower. Conversely, fuel cells offer a highly efficient and clean means of converting hydrogen energy into electricity with zero emissions. Electrochemical energy technologies, including fuel cells and metal–air batteries, serve as a temporary medium to store and release electricity as required.558–562 For all these systems, the oxygen evolution reaction (OER) or water oxidation, along with the oxygen reduction reaction (ORR) or hydrogen evolution reaction (HER), constitutes the core electrochemical reaction.563,564 The OER mechanism is quite complex due to the involvement of four electron–proton coupled reactions.565 Notably, the OER can occur in acidic, neutral, or alkaline media, making it highly pH-dependent.566 Under acidic and neutral conditions, two water molecules (H2O) undergo oxidation to form four protons (H+) and oxygen molecules (O2). In contrast, under basic conditions, the reaction proceeds by oxidizing hydroxyl groups (OH−) to produce H2O and O2:
4OH− ↔ 2H2O(l) + O2(g) + 4e− alkaline solution |
2H2O(l) ↔ 4H+ + O2(g) + 4e− acidic solution |
4.9.1. Doping-induced modified graphene composites for OER.
It has been noted that doping graphene materials with other elements can enhance their electrocatalytic performance. It is now widely acknowledged that the formerly perfect graphene for electrocatalysis cannot be further improved and that additional components (such as dopants) are needed in order to bring it back to its previous glory. As a result, numerous studies have concentrated on externally doping graphene with different elements like B, S, N, and P in order to increase the efficiency of water splitting.567–569 Only a few highly exceptional cases indicated that doping S or B reduced electrocatalytic activity; the effect was always electrocatalytic, regardless of whether the doping atoms were electron donating or electron withdrawing. Joshi et al. used pyrolysis and hydrothermal processing to create 80 iridium oxide nanoparticles (IrO2–B–rGO) supported on reduced graphene oxide. As observed in the TEM picture, iridium oxide nanoparticles were dispersed across B–rGO sheets. The lattice spacing of iridium oxide nanoparticles, which corresponds to the material's (200) plane in the HR-TEM picture, was 2.38 ± 0.20 Å. According to the XPS analysis, the composite comprises O, C, B, and Ir. The composite had an onset potential of 1.44 V and a Tafel slope of 124.8 mV dec−1 when utilised as an electrocatalyst for the oxygen evolution process (OER). Kumar et al. used a simple hydrothermal approach to create a composite of cobalt oxide and nitrogen-doped reduced graphene oxide (Co3O4/NrGO).570,571 Raman spectroscopy revealed peaks for the sp3 carbon at 1180 cm−1, the disordered carbon D band at 1350 cm−1, the amorphous sp2 carbon at 1540 cm−1, the E2g mode at 1590 cm−1, and the intercalation in graphite at 1620 cm−1. The XPS analysis confirmed that C, N, O, and Co were present in the composite. The composite was used as an electrocatalyst in the oxygen evolution process (OER) and oxygen reduction reaction (ORR). Co3O4/NRGO with a 30 wt% Co content had an OER overpotential of 1.64 V. The composite displayed an onset potential of 0.94 V and a half-wave potential of 0.88 V for the ORR. A CoTiO3/nitrogen-doped reduced graphene oxide composite (CoTiO3/NrGO) was produced by Luque-Centenoet et al. using a gel process.572Fig. 48 depicts the reaction mechanism for the OER on T-GO in 0.5 M KOH and the potential electron transport routes573 and Fig. 49(A)–(D) shows screenshots from an in situ experiment using monolayer graphene and chronoamperometry with a voltage held at 1.61 V (vs. RHE). The captions indicate the following time frames: 10, 20, 30, and 40 seconds, respectively. The black arrows show where the bubbles are migrating and causing damage to the electrode's surface (solution: 0.1 M KOH).574
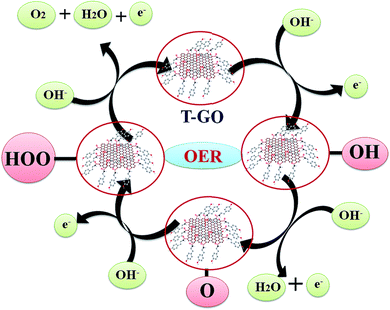 |
| Fig. 48 The reaction mechanism for the OER on T-GO in 0.5 M KOH and the potential electron transport routes.573 Reproduced (adapted) with permission from ref. 573. Copyright [2019] [RSC]. | |
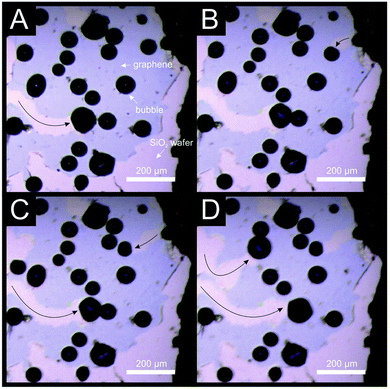 |
| Fig. 49 Screenshots from an in situ experiment using monolayer graphene and chronoamperometry with a voltage held at 1.61 V (vs. RHE). The captions indicate the following time frames: 10, 20, 30, and 40 seconds, respectively. The black arrows show where the bubbles are migrating and causing damage to the electrode's surface (solution: 0.1 M KOH).574 Reproduced (adapted) with permission from ref. 574. Copyright [2021] [RSC]. | |
Elemental analysis revealed that the composite's elemental composition after three hours of annealing was 19%, 20%, 36%, and 4.6% for Co, Ti, C, and N, respectively. The SEM picture revealed CoTiO3 nanoparticles dispersed over N-rGO sheets. Raman spectroscopy showed peaks at 159, 203, 228, 260, 330, 377, 448, and 688 cm−1, which are associated with CoTiO3, also displayed peaks at 155, 199, 395, 509, and 609 cm−1, showing prominent ones at 398 and 608 cm−1 corresponding to D and G bands of graphene, respectively. The peaks observed at 1350 and 1590 cm−1 also confirmed the presence of graphene via Raman spectroscopy. The composite was employed as an electrocatalyst in the oxygen evolution and reduction reactions (OER and ORR). The composite had an onset potential of 1.53 V for the OER after 3 hours of annealing. The ORR onset potential of the composite was 0.93 V. Tong et al. created cobalt oxide nanoparticles with B,N decorations attached to graphene (Fig. 48).573 The typical CoO peak patterns were discovered by the composite's XRD investigation. In the Raman spectroscopy, the graphene D and G bands as well as a peak for CoO at 680.1 cm−1 all showed strong peaks. In the TEM pictures, CoOx NPs were visible scattered over graphene sheets. The composite was used as an electrocatalyst in the oxygen evolution process (OER) and oxygen reduction reaction (ORR). The OER overpotential for the combination was 295 mV. It displayed an ORR half-wave potential of 805 mV, which is positive. Sapneret et al.575 chemically produced reduced graphene oxide (Ly-rGO) with L-lysine functionalization. The composite was used as an electrocatalyst for the oxygen evolution process (OER), displaying an overpotential of 0.33 V and a Tafel slope of 80 mV dec−1. Sun et al. developed Ni–Co–B nanosheets with reduced graphene oxide (Ni–Co–B/rGO) (Fig. 49).574 The unique orthorhombic Ni3B and Co4B peaks, as well as an expansive peak at 26 that matched rGO, were all visible in the XRD pattern. A D band, which stands for disordered carbon, and a G band, which stands for sp2 carbon, were visible in the Raman spectra. When used as an electrocatalyst for the oxygen evolution process (OER), the composite had an overpotential of 280 mV (Fig. 50). Xing et al. produced a manganese vanadium oxide@nitrogen-doped reduced graphene oxide composite (MnVOx@N-rGO) via a simple bottom-up approach. In brief, a mixture of GO, anhydrous acetonitrile (ACN), NH4OH, and water was agitated for 12 hours at 80 degrees Celsius before being cooked in an autoclave for 6 hours at 180 degrees Celsius, centrifuged, washed with ethanol, and dried to remove N-rGO. The same procedure was used to create MnVOx@N-rGO, which was then annealed for 4 hours at 440 °C or for 1 hour at 900 °C. The process was also used to create a combination of Mn4V4, ACN, GO, water, and NH4OH.576,577
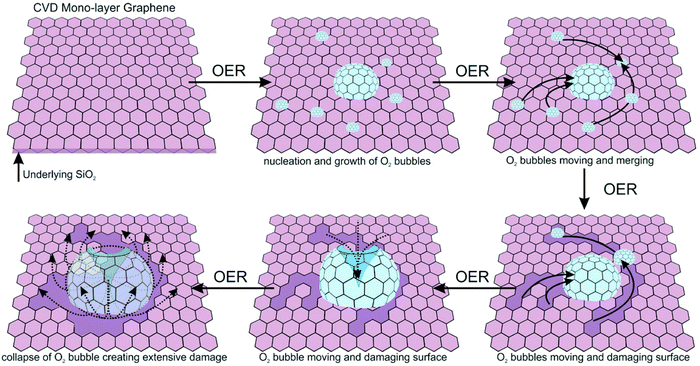 |
| Fig. 50 Schematic illustration of the formation, fusion, and collapse of oxygen bubbles that result in surface structural damage to the monolayer graphene electrode (induced by the OER).574 Reproduced (adapted) with permission from ref. 574. Copyright [2021] [RSC]. | |
4.9.2. Metal organic framework/graphene composites.
A novel family of materials known as metal organic frameworks (MOFs) are made up of both organic and inorganic components that have substantial porous morphological characteristics. The most intriguing aspect of MOF-based materials is that their properties may be easily tuned by altering their organic linker while maintaining a constant metal site, potentially improving their electrocatalytic activity.578–581 The following factors contribute to the MOF composite's high reactivity: (1) because the generated Ni-MOF nanoparticles were uniformly scattered and the strong electrical connection between the MOF and the graphene sheet prevented the MOF from clumping, the Ni active species were totally exposed; (2) furthermore, the 3D graphene sheets and Ni-MOF in the electrocatalyst had a synergistic effect on the surface morphologies, exposing more Ni active sites and improving charge transfer, which resulted in a significant increase in OER electroactivity. Fang et al. presented a hybrid iron–cobalt decorated N-doped rGO material (Fe–Co-CN/rGO-700) for an electrocatalytic water splitting process in an alkaline environment. Fig. 51 shows the synthetic process that was used. It was found that the given electrocatalyst requires overpotential values of 215 mV and 308 mV for the HER and OER, respectively. The extensively scattered Fe/Co species on the sheet-like graphene network with porosity had a significant role in the strong electrocatalytic activity of the N-doped graphene material. The DFT studies also found that the composite's redox behaviour was further enhanced by the increase in the number of electronic states close to the Fermi level. Wang et al. reported the formation of an MOF-derived CuO nanoflower and graphene composite (CuO NF@G/CF) for the OER reaction under alkaline conditions via a normal pulse voltage (NPV)-assisted method using HKUST-1 as the Cu-based MOF precursor.582–584 The CuO NFs were conventionally synthesized via the NPV treatment of the intermediately formed CuO NPs, as shown in Fig. 52. When used for the OER in 1 M KOH solution, the as-prepared CuO NF@G/CF displayed an overpotential of 320 mV for achieving a current density of 10 mA cm−2 with a low Tafel slope value of 63 mV dec−1.585
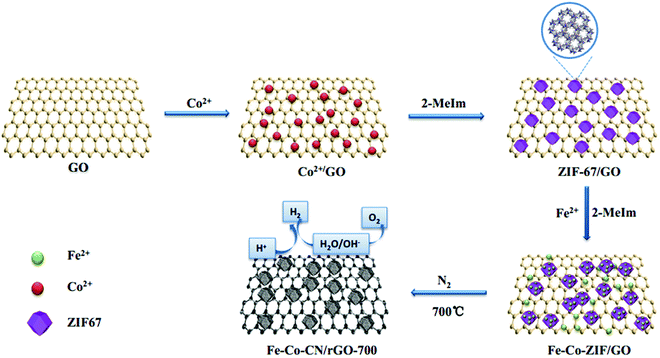 |
| Fig. 51 Diagrammatic representation of the synthesis of Fe–Co-CN/rGO-700.574 Reproduced (adapted) with permission from ref. 574. Copyright [2021] [RSC] | |
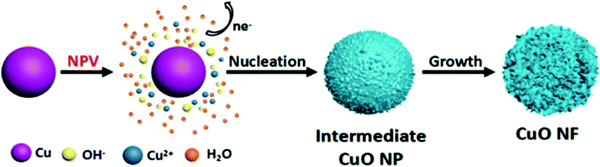 |
| Fig. 52 Diagrammatic representation of the synthesis of CuO NFs.574 Reproduced (adapted) with permission from ref. 574. Copyright [2021] [RSC]. | |
4.10. Alcohol oxidation reaction
The oxidation of alcohols is an important reaction in direct alcohol fuel cells (DAFCs). DAFCs use liquid alcohols like methanol and ethanol as chemical fuels because they are easier to store and refill. However, the slow kinetics of the alcohol oxidation reaction severely limits the performance of DAFCs. Noble-metal nanocatalysts, known for their exceptional performance in this reaction, have abundantly exposed catalytic active sites and the right amount of binding energy to the important reaction intermediates. Additionally, using graphene as a basic support could aid to further increase the stability and total catalytic activity. Additionally, the powerful interaction between electrons from the metal catalyst and the graphene substrate allows for the tuning of the density of states of noble-metal nanoparticles, further enhancing their catalytic abilities.586,587 The methanol as well as ethanol oxidation processes (MOR and EOR) have both recently utilised a large number of noble-metal nanostructures based on graphene. For instance, Li et al. developed Pt nanoclusters, in which graphene acted as a support, as a MOR catalyst, and their diameter ranged from 5 to 6 nm.588 The developed Pt/graphene catalyst demonstrated nearly two times higher peak current density and greater stability when compared to the commercial Pt/Vulcan catalyst. Later, using an electrochemical seed-mediated approach, Liu et al. created tetrahexahedral Pt nanoparticles in which graphene acted as a support.589 This catalyst's peak current density in the forward scan was 0.52 A mg−1, which was 1.4 and 1.7 times more than that of commercial Pt/C catalysts and spherical Pt nanoparticles/graphene, respectively. In addition, Wu et al. used MnOx colloids as the reducing agent and the sacrifice template to create a Pt/rGO nanocomposite.590 In terms of catalytic activity and durability towards the MOR, the Pt/rGO catalyst greatly outperformed commercial Pt/C (20 wt% Pt on Vulcan XC72R carbon) owing to its tiny particle size (1.8 ± 0.6 nm), homogeneous dispersal of Pt nanoparticles on rGO, excellent conductivity, and stability. As MOR catalysts, graphene-based Rh and Pd nanostructures are also being used in addition to Pt.591–593 For instance, Kang et al. proposed a one-pot hydrothermal method for producing Rh nanosheets supported by rGO (Rh/rGO NSs).591 The Rh/rGO NSs demonstrated exceptional MOR activity in alkaline media when compared to the commercial Pt/C catalyst, with 3.6 times higher mass activity at 0.61 V (vs. RHE) and a negative shift equal to 120 mV in the onset potential. This was noticeably better than that of the Rh nanoparticles and Rh/rGO hybrids. Importantly, the greater peak current ratio of the forward to reverse scans revealed that the Rh/rGO NSs had stronger resistance to CO poisoning. Additionally, Guo et al. created uniformly decorated Pd nanoparticles as tiny as 2.7 nm on graphene nanosheets. With regard to both MOR and EOR, this catalyst demonstrated strong catalytic activity and endurance.593 The significant CO poisoning effect still occurs despite the high activity of nanocomposites of single-element noble metals based on graphene because CO may cover more of the active surface sites and cause the catalysts to deactivate quickly. Preparing bimetallic nanoparticles in which one noble metal serves as the active centre of alcohol oxidation and the other metal functions as the agent for CO removal is one approach to solving this issue. The catalyst may concurrently achieve strong activity and superior tensile strength towards the alcohol oxidation process by utilising this synergistic result. Rao et al. recently documented the synthesis of cubic PtRh alloys on graphene with various atomic ratios due to Rh being the most often utilised CO removal agent.594 Their findings demonstrated that the alloy catalyst with a 1
:
1 atomic ratio displayed the best activity in the full oxidation of ethanol to carbon dioxide. The EOR reaction process was further studied using an electrochemical in situ Fourier transform infrared spectroscopy approach, which highlighted the crucial function of Rh in encouraging C–C bond breaking. Later, Hsieh et al. created bimetallic PdRh nanoparticles for the MOR on rGO nanosheets with various atomic ratios.595 They discovered that while the nearby Rh sites lessened the poisoning of active Pd centres, the Pd sites were in charge of hydrogen adsorption and methanol oxidation. As a result, the production of the PdRh alloy was crucial for the high CO tolerance, excellent stability towards the MOR, and good activity. According to their findings, the PdRh/rGO catalyst had the highest performance, with a low Tafel slope of 63 mV dec−1. Rh is expensive, thus it would be ideal to substitute alternative less expensive metals for it while still preserving the high mass activity of catalysts based on noble metals. Ni has recently been mentioned as a promising possibility. Li et al. developed a reduction method, for instance, to manufacture a truncated-octahedral PtNi alloy on rGO with various atomic ratios.596 The Pt3Ni alloy on rGO showed improved performance in the MOR due to the alteration of the Pt electronic structure with Ni. Another illustration is the use of graphene-supported NixPd100−x by Ahmed and Jeon for EOR, where they discovered that the Ni50Pd50/graphene catalyst had improved long-term stability and mass activity in alkaline solutions.597 However, it was thought that acetic acid, rather than CO2, was the major byproduct. X-Ray photoelectron spectroscopy (XPS) examination, however, proved that Ni was in an oxidised state. Huang et al. provided more evidence to support the critical role of oxidised Ni.598 The preparation of the Pt/Ni(OH)2/rGO ternary hybrid involved a two-step solution procedure. The presence of Pt nanoparticles was confirmed by TEM and HAADF STEM images (Fig. 53a and b). The elemental mapping revealed that Pt signals fit well with the form of Pt nanoparticles that were surrounded by Ni(OH)2 in large quantities (Fig. 53c and d). With 42.5 weight percent Pt and 10.5 weight percent Ni, the Pt/Ni(OH)2/rGO-4 CV curve showed an ECSA of 64.1 m2 g−1, which ranks among the top Pt-based catalyst values.
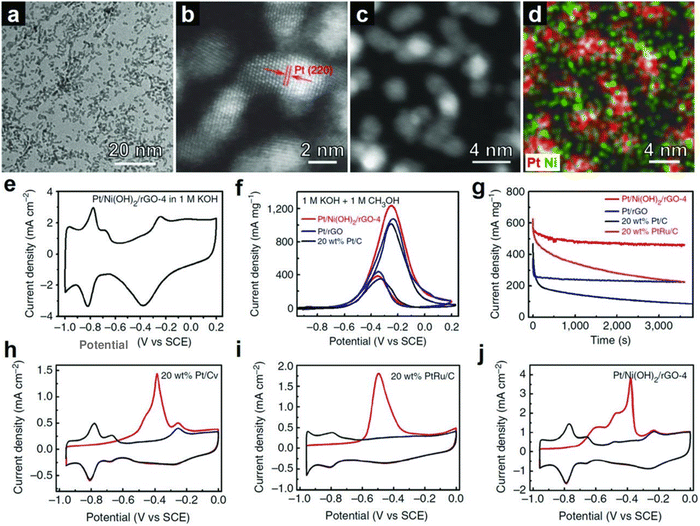 |
| Fig. 53 (a)–(c) TEM, HRTEM, and HAADF-STEM images of the Pt/Ni(OH)2/rGO ternary hybrid. (d) The mapping image that corresponds to (c), which combines Pt and Ni. (e) CV curve of Pt/Ni(OH)2/rGO−4 in 1 M KOH. (f) CV curves of commercial, Pt/Ni(OH)2/rGO-4, and 20 wt% Pt/C in 1 M methanol/1 M KOH. (g) Comparison of the short-term endurance of Pt/rGO, commercial 20 wt% Pt/C and Pt/Ni(OH)2/rGO-4 at 0.30 V (versus SCE) in 1 M methanol/1 M KOH.589 (h)–(j) CO stripping studies of commercial 20 wt% Pt/C (h), commercial 20 wt% PtRu/C (i), and Pt/Ni(OH)2/rGO (j) in 1 M KOH. Reproduced (adapted) with permission from ref. 589. Copyright [2018] [Wiley]. | |
Additionally, the ternary hybrid had a forward scan initial peak current density of 1236 mA mg−1, which was higher than that of both 20 wt% Pt/C and Pt/rGO (1076 mA mg−1) (Fig. 53f). However, Pt/C maintained only 20% of the original current density, while PtRu/C dropped to 220 mA mg−1 after 1 hour of operation. This ternary catalyst maintained a current density of 460 mA mg−1 after 1 hour of operation (Fig. 53g). The CO-stripping voltammetry clearly supported Ni(OH)2's crucial function in CO removal. Compared to Pt/C and PtRu/C, at a substantially more negative potential of −0.7 to −0.5 V, the Pt/Ni(OH)2/rGO catalyst displayed a broad CO electro-oxidation peak. This peak (Fig. 53h–j) was formed as a result of the CO oxidation at the Pt sites on the periphery of Ni(OH)2 being substantially easier due to the bifunctional interaction between Pt and Ni(OH)2. Graphene, which provided high electrical conductivity and prevented the aggregation of supported Pt and Ni(OH)2, Pt, which served as the MOR's active centre and assisted with the oxidative removal of carbonaceous toxins from the nearby Pt sites, and Ni(OH), which assisted with the oxidative removal of toxins from the nearby Pt sites, all worked together to contribute to the MOR's excellent activity and durability.597,599
Environmental implications of graphene based electrocatalysis.
Graphene-based electrocatalysis offers immense potential for revolutionizing environmental applications and addressing pressing challenges in sustainability and pollution control. The exceptional properties of graphene as an efficient catalyst in electrochemical reactions have opened up new avenues for cleaner and more efficient energy conversion, water treatment, and environmental monitoring. The use of graphene-based electrocatalysts in energy storage and conversion technologies, such as fuel cells and batteries, can lead to enhanced efficiency and storage capacities, promoting the adoption of clean and renewable energy sources. Additionally, in water electrolysis, graphene-based electrocatalysts lower the energy requirements for hydrogen production, making the process more energy-efficient and economically viable for large-scale hydrogen generation, a crucial component of the clean energy transition. In the fight against climate change, graphene-based electrocatalysts show promise in reducing carbon dioxide emissions by efficiently converting CO2 into valuable chemicals and fuels through electrochemical reduction, providing a potential solution for carbon capture and utilization. The environmental monitoring capabilities of graphene-based electrocatalytic sensors offer valuable tools for detecting pollutants in air, water, and soil, supporting efforts to assess environmental quality and safeguard ecosystems and human health. Furthermore, in various electrochemical processes for chemical synthesis and wastewater treatment, graphene-based electrocatalysts present greener and more sustainable alternatives, minimizing the environmental impact of industrial processes. As with any emerging technology, challenges in scalability, stability, and cost-effectiveness remain, necessitating ongoing research and development. However, the continued advancement in materials science and electrocatalytic engineering is expected to overcome these obstacles and unlock the full potential of graphene-based electrocatalysis in the quest for a cleaner, healthier, and more sustainable planet. In the years to come, graphene-based electrocatalysis is poised to play a crucial role in shaping a greener future, contributing significantly to environmental preservation and fostering a transition towards a more sustainable global society. With concerted efforts and innovative applications, graphene-based electrocatalysis has the potential to become a transformative force in environmental science and technology.
5. Conclusion and future perspectives
Graphene and its composites have proven to be highly adaptable and promising materials for both photocatalytic and electrocatalytic applications. The distinct characteristics of graphene, such as its expansive surface area, exceptional electrical conductivity, and customizable properties, have significantly enhanced catalytic processes. By integrating graphene with other photocatalytic materials, light absorption and charge separation have improved, resulting in more effective pollutant degradation and solar energy conversion. In electrocatalysis, graphene-based materials have exhibited remarkable catalytic activity for oxygen evolution and reduction processes, offering potential solutions for sustainable energy conversion and storage. The meticulous engineering and design of graphene composites have further amplified their catalytic performance and stability, making them viable contenders for various environmental remediation, energy conversion, and storage technologies. There are a number of promising opportunities for further research and development of graphene and composites based on it in the fields of photocatalysis and electrocatalysis in the future. Among the crucial aspects of the future are:
Rational design
Ongoing research into the rational design and synthesis of composites based on graphene will make it possible to create materials that are specifically tailored to have better catalytic performance and selectivity.
Enhanced photocatalysis
Development of highly effective and robust photocatalysts for use in solar-driven water splitting and pollutant degradation may be facilitated by improvements in graphene's integration with other semiconductors and functional materials.
Electrocatalytic applications
Additional research into the function of graphene as a support material for different electrocatalysts may result in innovative techniques to improve the efficiency of energy conversion and storage devices, such as fuel cells and metal–air batteries.
Mechanistic understanding
Better control and optimisation of the catalytic characteristics of graphene-based materials will be made possible by deeper understanding of the underlying mechanics of the catalytic processes involving these materials.
Scalability and cost-effectiveness
For the practical use of graphene-based catalytic materials in real-world applications, it will be essential to address the issues of scalability and cost-effectiveness associated with large-scale synthesis and commercialization.
Environmental sustainability
Graphene-based materials have the potential to be used in clean and sustainable energy technologies such as photocatalysis and electrocatalysis. The effects of these materials on the environment and their potential for recycling should be the main topics of research.
To sum up, graphene and composites made of graphene show great potential as adaptable and effective catalytic materials for a variety of applications. These materials are likely to play a significant part in tackling the world's energy and environmental concerns, paving the way for a sustainable and cleaner future, with continuous research and development.
Conflicts of interest
There are no conflicts to declare.
Acknowledgements
RA is highly thankful to the Ministry of Education (MoE) for financial assistance through the Institute fellowship. SH is highly thankful for the fellowship under the CSIR-JRF Scheme [File No. 09/0984(15809)2022-EMR-I]. SSM is grateful to the Director, National Institute of Technology, Srinagar, J&K for basic infrastructural and laboratory facilities.
References
- X. Li, J. Yu, S. Wageh, A. A. Al-Ghamdi and J. Xie, Graphene in Photocatalysis: A Review, Small, 2016, 12, 6640–6696 CrossRef CAS PubMed.
- X. An and J. C. Yu, Graphene-based photocatalytic composites, RSC Adv., 2011, 1, 1426 RSC.
- X.-Q. Wang and Q. He, Progress in Photocatalytic Degradation of Organic Wastewater by Graphene Binary Composites, E3S Web Conf., 2021, 261, 02029 CrossRef CAS.
- L. K. Putri, W.-J. Ong, W. S. Chang and S.-P. Chai, Heteroatom doped graphene in photocatalysis: A review, Appl. Surf. Sci., 2015, 358, 2–14 CrossRef CAS.
- P. V. Kamat, Graphene-Based Nanoassemblies for Energy Conversion, J. Phys. Chem. Lett., 2011, 2, 242–251 CrossRef CAS.
- S. Hussain and S. S. Maktedar, Structural, functional and mechanical performance of advanced Graphene-based composite hydrogels, Results Chem., 2023, 6, 101029 CrossRef CAS.
- H. Wang, X. Liu, P. Niu, S. Wang, J. Shi and L. Li, Porous Two-Dimensional Materials for Photocatalytic and Electrocatalytic Applications, Matter, 2020, 2, 1377–1413 CrossRef.
- M. Faraldos and A. Bahamonde, Environmental applications of titania-graphene photocatalysts, Catal. Today, 2017, 285, 13–28 CrossRef CAS.
- A. Ganguly, O. Anjaneyulu, K. Ojha and A. K. Ganguli, Oxide-based nanostructures for photocatalytic and electrocatalytic applications, CrystEngComm, 2015, 17, 8978–9001 RSC.
- B. Zhang, H.-H. Wang, H. Su, L.-B. Lv, T.-J. Zhao, J.-M. Ge, X. Wei, K.-X. Wang, X.-H. Li and J.-S. Chen, Nitrogen-doped graphene microtubes with opened inner voids: Highly efficient metal-free electrocatalysts for alkaline hydrogen evolution reaction, Nano Res., 2016, 9, 2606–2615 CrossRef CAS.
- W. Qian, S. Xu, X. Zhang, C. Li, W. Yang, C. R. Bowen and Y. Yang, Differences and Similarities of Photocatalysis and Electrocatalysis in Two-Dimensional Nanomaterials: Strategies, Traps, Applications and Challenges, Nano-Micro Lett., 2021, 13, 156 CrossRef CAS.
- R. Akhter and S. S. Maktedar, MXenes: A comprehensive review of synthesis, properties, and progress in supercapacitor applications, J. Materiomics, 2023, 9(6), 1196–1241 CrossRef.
- S. Yousuf and S. S. Maktedar, Influence of quince seed mucilage-alginate composite hydrogel coatings on quality of fresh walnut kernels during refrigerated storage, J. Food Sci. Technol., 2022, 59, 4801–4811 CrossRef CAS.
- S. Yousuf and S. S. Maktedar, Utilization of quince (Cydonia oblonga) seeds for production of mucilage: functional, thermal and rheological characterization, Sustainable Food Technol., 2023, 1, 107–115 RSC.
- G. Avashthi, S. S. Maktedar and M. Singh, Surface-Induced in Situ Sonothermodynamically Controlled Functionalized Graphene Oxide for in Vitro Cytotoxicity and Antioxidant Evaluations, ACS Omega, 2019, 4, 16385–16401 CrossRef CAS.
- G. Avashthi, S. S. Maktedar and M. Singh, Sonochemically N-functionalized graphene oxide towards optically active photoluminescent bioscaffold, Ultrason. Sonochem., 2019, 58, 104651 CrossRef CAS.
- A. Hashmi, V. Nayak, K. R. Singh, B. Jain, M. Baid, F. Alexis and A. K. Singh, Potentialities of graphene and its allied derivatives to combat against SARS-CoV-2 infection, Mater. Today Adv., 2022, 13, 100208 CrossRef CAS.
- K. S. Novoselov, A. K. Geim, S. V. Morozov, D. Jiang, Y. Zhang, S. V. Dubonos, I. V. Grigorieva and A. A. Firsov, Electric Field Effect in Atomically Thin Carbon Films, Science, 2004, 306, 666–669 CrossRef CAS.
- A. K. Geim, Graphene: Status and Prospects, Science, 2009, 324, 1530–1534 CrossRef CAS PubMed.
- A. A. Balandin, S. Ghosh, W. Bao, I. Calizo, D. Teweldebrhan, F. Miao and C. N. Lau, Superior Thermal Conductivity of Single-Layer Graphene, Nano Lett., 2008, 8, 902–907 CrossRef CAS PubMed.
- A. A. Balandin, Thermal properties of graphene and nanostructured carbon materials, Nat. Mater., 2011, 10, 569–581 CrossRef CAS PubMed.
- Y. Zhang, Y.-W. Tan, H. L. Stormer and P. Kim, Experimental observation of the quantum Hall effect and Berry's phase in graphene, Nature, 2005, 438, 201–204 CrossRef CAS PubMed.
- K. S. Novoselov, E. McCann, S. V. Morozov, V. I. Fal’ko, M. I. Katsnelson, U. Zeitler, D. Jiang, F. Schedin and A. K. Geim, Unconventional quantum Hall effect and Berry's phase of 2π in bilayer graphene, Nat. Phys., 2006, 2, 177–180 Search PubMed.
- K. S. Novoselov, Z. Jiang, Y. Zhang, S. V. Morozov, H. L. Stormer, U. Zeitler, J. C. Maan, G. S. Boebinger, P. Kim and A. K. Geim, Room-Temperature Quantum Hall Effect in Graphene, Science, 2007, 315, 1379 CrossRef CAS PubMed.
- K. S. Novoselov, A. K. Geim, S. V. Morozov, D. Jiang, M. I. Katsnelson, I. V. Grigorieva, S. V. Dubonos and A. A. Firsov, Two-dimensional gas of massless Dirac fermions in graphene, Nature, 2005, 438, 197–200 CrossRef CAS.
- K. I. Bolotin, K. J. Sikes, Z. Jiang, M. Klima, G. Fudenberg, J. Hone, P. Kim and H. L. Stormer, Ultrahigh electron mobility in suspended graphene, Solid State Commun., 2008, 146, 351–355 CrossRef CAS.
- X. Du, I. Skachko, A. Barker and E. Y. Andrei, Approaching ballistic transport in suspended graphene, Nat. Nanotechnol., 2008, 3, 491–495 CrossRef CAS.
- Z.-S. Wu, W. Ren, L. Gao, J. Zhao, Z. Chen, B. Liu, D. Tang, B. Yu, C. Jiang and H.-M. Cheng, Synthesis of Graphene Sheets with High Electrical Conductivity and Good Thermal Stability by Hydrogen Arc Discharge Exfoliation, ACS Nano, 2009, 3, 411–417 CrossRef CAS PubMed.
- M. D. Stoller, S. Park, Y. Zhu, J. An and R. S. Ruoff, Graphene-Based Ultracapacitors, Nano Lett., 2008, 8, 3498–3502 CrossRef CAS PubMed.
- C. Lee, X. Wei, J. W. Kysar and J. Hone, Measurement of the Elastic Properties and Intrinsic Strength of Monolayer Graphene, Science, 2008, 321, 385–388 CrossRef CAS PubMed.
- A. K. Geim and K. S. Novoselov, The rise of graphene, Nat. Mater., 2007, 6, 183–191 CrossRef CAS.
- P. Lazar, F. Karlický, P. Jurečka, M. Kocman, E. Otyepková, K. Šafářová and M. Otyepka, Adsorption of Small Organic Molecules on Graphene, J. Am. Chem. Soc., 2013, 135, 6372–6377 CrossRef CAS.
- V. Chandra, J. Park, Y. Chun, J. W. Lee, I.-C. Hwang and K. S. Kim, Water-Dispersible Magnetite-Reduced Graphene Oxide Composites for Arsenic Removal, ACS Nano, 2010, 4, 3979–3986 CrossRef CAS.
- Q. Xiang, J. Yu and M. Jaroniec, Graphene-based semiconductor photocatalysts, Chem. Soc. Rev., 2012, 41, 782–796 RSC.
- G. Yang, L. Li, W. B. Lee and M. C. Ng, Structure of graphene and its disorders: a review, Sci. Technol. Adv. Mater., 2018, 19, 613–648 CrossRef CAS PubMed.
- G. Xie, K. Zhang, B. Guo, Q. Liu, L. Fang and J. R. Gong, Graphene-Based Materials for Hydrogen Generation from Light-Driven Water Splitting, Adv. Mater., 2013, 25, 3820–3839 CrossRef CAS PubMed.
- M.-Q. Yang, N. Zhang, M. Pagliaro and Y.-J. Xu, Artificial photosynthesis over graphene–semiconductor composites. Are we getting better?, Chem. Soc. Rev., 2014, 43, 8240–8254 RSC.
- Q. Xiang and J. Yu, Graphene-Based Photocatalysts for Hydrogen Generation, J. Phys. Chem. Lett., 2013, 4, 753–759 CrossRef CAS PubMed.
- A. Fujishima and K. Honda, Electrochemical Photolysis of Water at a Semiconductor Electrode, Nature, 1972, 238, 37–38 CrossRef CAS.
- X. Li, J. Yu, J. Low, Y. Fang, J. Xiao and X. Chen, Engineering heterogeneous semiconductors for solar water splitting, J. Mater. Chem. A, 2015, 3, 2485–2534 RSC.
- S. C. Roy, O. K. Varghese, M. Paulose and C. A. Grimes, Toward Solar Fuels: Photocatalytic Conversion of Carbon Dioxide to Hydrocarbons, ACS Nano, 2010, 4, 1259–1278 CrossRef CAS PubMed.
- S. K. Tiwari, V. Kumar, A. Huczko, R. Oraon, A. D. Adhikari and G. C. Nayak, Magical Allotropes of Carbon: Prospects and Applications, Crit. Rev. Solid State Mater. Sci., 2016, 41, 257–317 CrossRef CAS.
- Y. Yang, R. Liu, J. Wu, X. Jiang, P. Cao, X. Hu, T. Pan, C. Qiu, J. Yang, Y. Song, D. Wu and Y. Su, Bottom-up Fabrication of Graphene on Silicon/Silica Substrate via a Facile Soft-hard Template Approach, Sci. Rep., 2015, 5, 13480 CrossRef CAS.
- S. K. Tiwari, R. K. Mishra, S. K. Ha and A. Huczko, Evolution of Graphene Oxide and Graphene: From Imagination to Industrialization, ChemNanoMat, 2018, 4, 598–620 CrossRef CAS.
- J. Zhang, S. Li, B. Tang, Z. Wang, G. Ji, W. Huang and J. Wang, High Photocatalytic Performance of Two Types of Graphene Modified TiO2 Composite Photocatalysts, Nanoscale Res. Lett., 2017, 12, 457 CrossRef PubMed.
- L. Liao, H. Peng and Z. Liu, Chemistry Makes Graphene beyond Graphene, J. Am. Chem. Soc., 2014, 136, 12194–12200 CrossRef CAS PubMed.
- J.-H. Lee, E. K. Lee, W.-J. Joo, Y. Jang, B.-S. Kim, J. Y. Lim, S.-H. Choi, S. J. Ahn, J. R. Ahn, M.-H. Park, C.-W. Yang, B. L. Choi, S.-W. Hwang and D. Whang, Wafer-Scale Growth of Single-Crystal Monolayer Graphene on Reusable Hydrogen-Terminated Germanium, Science, 2014, 344, 286–289 CrossRef CAS.
- S. P. Surwade, S. N. Smirnov, I. V. Vlassiouk, R. R. Unocic, G. M. Veith, S. Dai and S. M. Mahurin, Water desalination using nanoporous single-layer graphene, Nat. Nanotechnol., 2015, 10, 459–464 CrossRef CAS.
- W. K. Park, Y. Yoon, Y. H. Song, S. Y. Choi, S. Kim, Y. Do, J. Lee, H. Park, D. H. Yoon and W. S. Yang, High-efficiency exfoliation of large-area mono-layer graphene oxide with controlled dimension, Sci. Rep., 2017, 7, 16414 CrossRef PubMed.
- S. K. Tiwari, A. Huczko, R. Oraon, A. De Adhikari and G. C. Nayak, A time efficient reduction strategy for bulk production of reduced graphene oxide using selenium powder as a reducing agent, J. Mater. Sci., 2016, 51, 6156–6165 CrossRef CAS.
- Y. Yang, R. Liu, J. Wu, X. Jiang, P. Cao, X. Hu, T. Pan, C. Qiu, J. Yang, Y. Song, D. Wu and Y. Su, Bottom-up Fabrication of Graphene on Silicon/Silica Substrate via a Facile Soft-hard Template Approach, Sci. Rep., 2015, 5, 13480 CrossRef CAS PubMed.
- D. Lin, Y. Liu, Z. Liang, H.-W. Lee, J. Sun, H. Wang, K. Yan, J. Xie and Y. Cui, Layered reduced graphene oxide with nanoscale interlayer gaps as a stable host for lithium metal anodes, Nat. Nanotechnol., 2016, 11, 626–632 CrossRef CAS PubMed.
- A. M. Dimiev and J. M. Tour, Mechanism of Graphene Oxide Formation, ACS Nano, 2014, 8, 3060–3068 CrossRef CAS.
- J. Abraham, K. S. Vasu, C. D. Williams, K. Gopinadhan, Y. Su, C. T. Cherian, J. Dix, E. Prestat, S. J. Haigh, I. V. Grigorieva, P. Carbone, A. K. Geim and R. R. Nair, Tunable sieving of ions using graphene oxide membranes, Nat. Nanotechnol., 2017, 12, 546–550 CrossRef CAS PubMed.
- C. K. Chua and M. Pumera, Chemical reduction of graphene oxide: a synthetic chemistry viewpoint, Chem. Soc. Rev., 2014, 43, 291–312 RSC.
- W.-J. Ong, L.-L. Tan, S.-P. Chai and S.-T. Yong, Graphene oxide as a structure-directing agent for the two-dimensional interface engineering of sandwich-like graphene–g-C3N4 hybrid nanostructures with enhanced visible-light photoreduction of CO2 to methane, Chem. Commun., 2015, 51, 858–861 RSC.
- S. Baradaran, E. Moghaddam, W. J. Basirun, M. Mehrali, M. Sookhakian, M. Hamdi, M. R. N. Moghaddam and Y. Alias, Mechanical properties and biomedical applications of a nanotube hydroxyapatite-reduced graphene oxide composite, Carbon, 2014, 69, 32–45 CrossRef CAS.
- X. Cao, D. Qi, S. Yin, J. Bu, F. Li, C. F. Goh, S. Zhang and X. Chen, Ambient Fabrication of Large-Area Graphene Films via a Synchronous Reduction and Assembly Strategy, Adv. Mater., 2013, 25, 2957–2962 CrossRef CAS PubMed.
- Y. Su, V. G. Kravets, S. L. Wong, J. Waters, A. K. Geim and R. R. Nair, Impermeable barrier films and protective coatings based on reduced graphene oxide, Nat. Commun., 2014, 5, 4843 CrossRef CAS PubMed.
- C.-N. Yeh, K. Raidongia, J. Shao, Q.-H. Yang and J. Huang, On the origin of the stability of graphene oxide membranes in water, Nat. Chem., 2015, 7, 166–170 CrossRef CAS PubMed.
- S. Y. Toh, K. S. Loh, S. K. Kamarudin and W. R. W. Daud, Graphene production via electrochemical reduction of graphene oxide: Synthesis and characterisation, Chem. Eng. J., 2014, 251, 422–434 CrossRef CAS.
- L. Yang, D. Liu, J. Huang and T. You, Simultaneous determination of dopamine, ascorbic acid and uric acid at electrochemically reduced graphene oxide modified electrode, Sens. Actuators, B, 2014, 193, 166–172 CrossRef CAS.
- J. Feng, Y. Ye, M. Xiao, G. Wu and Y. Ke, Synthetic routes of the reduced graphene oxide, Chem. Pap., 2020, 74, 3767–3783 CrossRef CAS.
- H. Lee, N. Son, H. Y. Jeong, T. G. Kim, G. S. Bang, J. Y. Kim, G. W. Shim, K. C. Goddeti, J. H. Kim, N. Kim, H.-J. Shin, W. Kim, S. Kim, S.-Y. Choi and J. Y. Park, Friction and conductance imaging of sp2- and sp3-hybridized subdomains on single-layer graphene oxide, Nanoscale, 2016, 8, 4063–4069 RSC.
- X. Li, J. Yu, J. Low, Y. Fang, J. Xiao and X. Chen, Engineering heterogeneous semiconductors for solar water splitting, J. Mater. Chem. A, 2015, 3, 2485–2534 RSC.
- J. Yu, Y. Hai and B. Cheng, Enhanced Photocatalytic H2-Production Activity of TiO2 by Ni(OH)2 Cluster Modification, J. Phys. Chem. C, 2011, 115, 4953–4958 CrossRef CAS.
- J. Wen, X. Li, W. Liu, Y. Fang, J. Xie and Y. Xu, Photocatalysis fundamentals and surface modification of TiO2 nanomaterials, Chin. J. Catal., 2015, 36, 2049–2070 CrossRef CAS.
- T. Zhang, K. Zhao, J. Yu, J. Jin, Y. Qi, H. Li, X. Hou and G. Liu, Photocatalytic water splitting for hydrogen generation on cubic, orthorhombic, and tetragonal KNbO3 microcubes, Nanoscale, 2013, 5, 8375 RSC.
- J. Yu, Y. Hai and M. Jaroniec, Photocatalytic hydrogen production over CuO-modified titania, J. Colloid Interface Sci., 2011, 357, 223–228 CrossRef CAS PubMed.
- Y. Ma, X. Wang, Y. Jia, X. Chen, H. Han and C. Li, Titanium Dioxide-Based Nanomaterials for Photocatalytic Fuel Generations, Chem. Rev., 2014, 114, 9987–10043 CrossRef CAS PubMed.
- Z. Sun, X. Liu, Q. Yue, H. Jia and P. Du, Cadmium Sulfide Nanorods Decorated with Copper Sulfide via One-Step Cation Exchange Approach for Enhanced Photocatalytic Hydrogen Evolution under Visible Light, ChemCatChem, 2015, 8, 157–162 CrossRef.
- Z. Sun, H. Zheng, J. Li and P. Du, Extraordinarily efficient photocatalytic hydrogen evolution in water using semiconductor nanorods integrated with crystalline Ni2P cocatalysts, Energy Environ. Sci., 2015, 8, 2668–2676 RSC.
- X. Zong, H. Yan, G. Wu, G. Ma, F. Wen, L. Wang and C. Li, Enhancement of Photocatalytic H2 Evolution on CdS by Loading MoS2 as Cocatalyst under Visible Light Irradiation, J. Am. Chem. Soc., 2008, 130, 7176–7177 CrossRef CAS.
- H. Yan, J. Yang, G. Ma, G. Wu, X. Zong, Z. Lei, J. Shi and C. Li, Visible-light-driven hydrogen production with extremely high quantum efficiency on Pt–PdS/CdS photocatalyst, J. Catal., 2009, 266, 165–168 CrossRef CAS.
- X. Zong, J. Han, G. Ma, H. Yan, G. Wu and C. Li, Photocatalytic H2 Evolution on CdS Loaded with WS2 as Cocatalyst under Visible Light Irradiation, J. Phys. Chem. C, 2011, 115, 12202–12208 CrossRef CAS.
- K. Maeda and K. Domen, New Non-Oxide Photocatalysts Designed for Overall Water Splitting under Visible Light, J. Phys. Chem. C, 2007, 111, 7851–7861 CrossRef CAS.
- K. Maeda and K. Domen, Photocatalytic Water Splitting: Recent Progress and Future Challenges, J. Phys. Chem. Lett., 2010, 1, 2655–2661 CrossRef CAS.
- Y. Moriya, T. Takata and K. Domen, Recent progress in the development of (oxy)nitride photocatalysts for water splitting under visible-light irradiation, Coord. Chem. Rev., 2013, 257, 1957–1969 CrossRef CAS.
- S. Min and G. Lu, Enhanced Electron Transfer from the Excited Eosin Y to mpg-C3N4 for Highly Efficient Hydrogen Evolution under 550 nm Irradiation, J. Phys. Chem. C, 2012, 116, 19644–19652 CrossRef CAS.
- Q. Li, L. Chen and G. Lu, Visible-Light-Induced Photocatalytic Hydrogen Generation on Dye-Sensitized Multiwalled Carbon Nanotube/Pt Catalyst, J. Phys. Chem. C, 2007, 111, 11494–11499 CrossRef CAS.
- C. Kong, S. Min and G. Lu, Dye-sensitized cobalt catalysts for high efficient visible light hydrogen evolution, Int. J. Hydrogen Energy, 2014, 39, 4836–4844 CrossRef CAS.
- W. Zhang, C. Kong and G. Lu, Super-paramagnetic nano-Fe3O4/graphene for visible-light-driven hydrogen evolution, Chem. Commun., 2015, 51, 10158–10161 RSC.
- J. Jiang, J. Yu and S. Cao, Au/PtO nanoparticle-modified g-C3N4 for plasmon-enhanced photocatalytic hydrogen evolution under visible light, J. Colloid Interface Sci., 2016, 461, 56–63 CrossRef CAS.
- M. S. Akple, J. Low, S. Wageh, A. A. Al-Ghamdi, J. Yu and J. Zhang, Enhanced visible light photocatalytic H2-production of g-C3N4/WS2 composite heterostructures, Appl. Surf. Sci., 2015, 358, 196–203 CrossRef CAS.
- J. Wen, X. Li, H. Li, S. Ma, K. He, Y. Xu, Y. Fang, W. Liu and Q. Gao, Enhanced visible-light H2 evolution of g-C3N4 photocatalysts via the synergetic effect of amorphous NiS and cheap metal-free carbon black nanoparticles as co-catalysts, Appl. Surf. Sci., 2015, 358, 204–212 CrossRef CAS.
- Y. Zhong, J. Yuan, J. Wen, X. Li, Y. Xu, W. Liu, S. Zhang and Y. Fang, Earth-abundant NiS co-catalyst modified metal-free mpg-C3N4/CNT nanocomposites for highly efficient visible-light photocatalytic H2 evolution, Dalton Trans., 2015, 44, 18260–18269 RSC.
- S. Cao and J. Yu, g-C3N4-Based Photocatalysts for Hydrogen Generation, J. Phys. Chem. Lett., 2014, 5, 2101–2107 CrossRef CAS.
- X. Zhou, Q. Gao, X. Li, Y. Liu, S. Zhang, Y. Fang and J. Li, Ultra-thin SiC layer covered graphene nanosheets as advanced photocatalysts for hydrogen evolution, J. Mater. Chem. A, 2015, 3, 10999–11005 RSC.
- X. Zhou, X. Li, Q. Gao, J. Yuan, J. Wen, Y. Fang, W. Liu, S. Zhang and Y. Liu, Metal-free carbon nanotube–SiC nanowire heterostructures with enhanced photocatalytic H2 evolution under visible light irradiation, Catal. Sci. Technol., 2015, 5, 2798–2806 RSC.
- J. Zhang, Y. Wang, J. Zhang, Z. Lin, F. Huang and J. Yu, Enhanced Photocatalytic Hydrogen Production Activities of Au-Loaded ZnS Flowers, ACS Appl. Mater. Interfaces, 2013, 5, 1031–1037 CrossRef CAS.
- J. Yuan, J. Wen, Q. Gao, S. Chen, J. Li, X. Li and Y. Fang, Amorphous Co3O4 modified CdS nanorods with enhanced visible-light photocatalytic H2-production activity, Dalton Trans., 2015, 44, 1680–1689 RSC.
- J. Zhang, S. Z. Qiao, L. Qi and J. Yu, Fabrication of NiS modified CdS nanorod p–n junction photocatalysts with enhanced visible-light photocatalytic H2-production activity, Phys. Chem. Chem. Phys., 2013, 15, 12088 RSC.
- J. Yu, Y. Yu, P. Zhou, W. Xiao and B. Cheng, Morphology-dependent photocatalytic H2-production activity of CdS, Appl. Catal., B, 2014, 156–157, 184–191 CrossRef CAS.
- Y. Hong, J. Zhang, F. Huang, J. Zhang, X. Wang, Z. Wu, Z. Lin and J. Yu, Enhanced visible light photocatalytic hydrogen production activity of CuS/ZnS nanoflower spheres, J. Mater. Chem. A, 2015, 3, 13913–13919 RSC.
- Q. Li, H. Meng, P. Zhou, Y. Zheng, J. Wang, J. Yu and J. Gong, Zn1−xCdxS Solid Solutions with Controlled Bandgap and Enhanced Visible-Light Photocatalytic H2-Production Activity, ACS Catal., 2013, 3, 882–889 CrossRef CAS.
- Q. Li, X. Li, S. Wageh, A. A. Al-Ghamdi and J. Yu, CdS/Graphene Nanocomposite Photocatalysts, Adv. Energy Mater., 2015, 5(14), 1500010 CrossRef.
- J. Zhang, J. Yu, M. Jaroniec and J. R. Gong, Noble Metal-Free Reduced Graphene Oxide-ZnxCd1−xS Nanocomposite with Enhanced Solar Photocatalytic H2-Production Performance, Nano Lett., 2012, 12, 4584–4589 CrossRef CAS.
- Q. Li, B. Guo, J. Yu, J. Ran, B. Zhang, H. Yan and J. R. Gong, Highly Efficient Visible-Light-Driven Photocatalytic Hydrogen Production of CdS-Cluster-Decorated Graphene Nanosheets, J. Am. Chem. Soc., 2011, 133, 10878–10884 CrossRef CAS PubMed.
- Z. Fang, Y. Wang, J. Song, Y. Sun, J. Zhou, R. Xu and H. Duan, Immobilizing CdS quantum dots and dendritic Pt nanocrystals on thiolated graphene nanosheets toward highly efficient photocatalytic H2 evolution, Nanoscale, 2013, 5, 9830 RSC.
- B. Zhu, B. Lin, Y. Zhou, P. Sun, Q. Yao, Y. Chen and B. Gao, Enhanced photocatalytic H2 evolution on ZnS loaded with graphene and MoS2 nanosheets as cocatalysts, J. Mater. Chem. A, 2014, 2, 3819–3827 RSC.
- D. Lang, T. Shen and Q. Xiang, Roles of MoS2 and Graphene as Cocatalysts in the Enhanced Visible-Light Photocatalytic H2 Production Activity of Multiarmed CdS Nanorods, ChemCatChem, 2015, 7, 943–951 CrossRef CAS.
- T. Jia, A. Kolpin, C. Ma, R. C.-T. Chan, W.-M. Kwok and S. C. E. Tsang, A graphene dispersed CdS–MoS2 nanocrystal ensemble for cooperative photocatalytic hydrogen production from water, Chem. Commun., 2014, 50, 1185–1188 RSC.
- K. Chang, Z. Mei, T. Wang, Q. Kang, S. Ouyang and J. Ye, MoS2/Graphene Cocatalyst for Efficient Photocatalytic H2 Evolution under Visible Light Irradiation, ACS Nano, 2014, 8, 7078–7087 CrossRef CAS PubMed.
- B. Zhu, B. Lin, Y. Zhou, P. Sun, Q. Yao, Y. Chen and B. Gao, Enhanced photocatalytic H2 evolution on ZnS loaded with graphene and MoS2 nanosheets as cocatalysts, J. Mater. Chem. A, 2014, 2, 3819–3827 RSC.
- J. Zhang, L. Qi, J. Ran, J. Yu and S. Z. Qiao, Ternary NiS/ZnxCd1−xS/Reduced Graphene Oxide Nanocomposites for Enhanced Solar Photocatalytic H2-Production Activity, Adv. Energy Mater., 2014, 4(10), 1301925 CrossRef.
- G. Xie, K. Zhang, B. Guo, Q. Liu, L. Fang and J. R. Gong, Graphene-Based Materials for Hydrogen Generation from Light-Driven Water Splitting, Adv. Mater., 2013, 25, 3820–3839 CrossRef CAS PubMed.
- X. Li, J. Yu, J. Low, Y. Fang, J. Xiao and X. Chen, Engineering heterogeneous semiconductors for solar water splitting, J. Mater. Chem. A, 2015, 3, 2485–2534 RSC.
- T.-F. Yeh, C.-Y. Teng, S.-J. Chen and H. Teng, Nitrogen-Doped Graphene Oxide Quantum Dots as Photocatalysts for Overall Water-Splitting under Visible Light Illumination, Adv. Mater., 2014, 26, 3297–3303 CrossRef CAS.
- A. Iwase, Y. H. Ng, Y. Ishiguro, A. Kudo and R. Amal, Reduced Graphene Oxide as a Solid-State Electron Mediator in Z-Scheme Photocatalytic Water Splitting under Visible Light, J. Am. Chem. Soc., 2011, 133, 11054–11057 CrossRef CAS.
- K. Iwashina, A. Iwase, Y. H. Ng, R. Amal and A. Kudo, Z-Schematic Water Splitting into H2 and O2 Using Metal Sulfide as a Hydrogen-Evolving Photocatalyst and Reduced Graphene Oxide as a Solid-State Electron Mediator, J. Am. Chem. Soc., 2015, 137, 604–607 CrossRef CAS PubMed.
- T. Inoue, A. Fujishima, S. Konishi and K. Honda, Photoelectrocatalytic reduction of carbon dioxide in aqueous suspensions of semiconductor powders, Nature, 1979, 277, 637–638 CrossRef CAS.
- B. Koo, S. Byun, S. Nam, S. Moon, S. Kim, J. Y. Park, B. T. Ahn and B. Shin, Reduced Graphene Oxide as a Catalyst Binder: Greatly Enhanced Photoelectrochemical Stability of Cu(In,Ga)Se2 Photocathode for Solar Water Splitting, Adv. Funct. Mater., 2018, 28(16), 1705136 CrossRef.
- S. N. Habisreutinger, L. Schmidt-Mende and J. K. Stolarczyk, Photocatalytic Reduction of CO2 on TiO2 and Other Semiconductors, Angew. Chem., Int. Ed., 2013, 52, 7372–7408 CrossRef CAS PubMed.
- X. Li, J. Wen, J. Low, Y. Fang and J. Yu, Design and fabrication of semiconductor photocatalyst for photocatalytic reduction of CO2 to solar fuel, Sci. China Mater., 2014, 57, 70–100 CrossRef.
- J. Yu, J. Jin, B. Cheng and M. Jaroniec, A noble metal-free reduced graphene oxide–CdS nanorod composite for the enhanced visible-light photocatalytic reduction of CO2 to solar fuel, J. Mater. Chem. A, 2014, 2, 3407 RSC.
- W. Tu, Y. Zhou, Q. Liu, S. Yan, S. Bao, X. Wang, M. Xiao and Z. Zou, An In Situ Simultaneous Reduction-Hydrolysis Technique for Fabrication of TiO2-Graphene 2D Sandwich-Like Hybrid Nanosheets: Graphene-Promoted Selectivity of Photocatalytic-Driven Hydrogenation and Coupling of CO2 into Methane and Ethane, Adv. Funct. Mater., 2012, 23, 1743–1749 CrossRef.
- X. An, K. Li and J. Tang, Cu2O/Reduced Graphene Oxide Composites for the Photocatalytic Conversion of CO2, ChemSusChem, 2014, 7, 1086–1093 CrossRef CAS.
- W. Tu, Y. Zhou, Q. Liu, Z. Tian, J. Gao, X. Chen, H. Zhang, J. Liu and Z. Zou, Robust Hollow Spheres Consisting of Alternating Titania Nanosheets and Graphene Nanosheets with High Photocatalytic Activity for CO2 Conversion into Renewable Fuels, Adv. Funct. Mater., 2012, 22, 1215–1221 CrossRef CAS.
- J. Low, B. Cheng, J. Yu and M. Jaroniec, Carbon-based two-dimensional layered materials for photocatalytic CO2 reduction to solar fuels, Energy Storage Mater., 2016, 3, 24–35 CrossRef.
- J. Low, S. Cao, J. Yu and S. Wageh, Two-dimensional layered composite photocatalysts, Chem. Commun., 2014, 50, 10768 RSC.
- Q. Xiang, J. Yu and M. Jaroniec, Preparation and Enhanced Visible-Light Photocatalytic H2-Production Activity of Graphene/C3N4 Composites, J. Phys. Chem. C, 2011, 115, 7355–7363 CrossRef CAS.
- N. Zhang, M.-Q. Yang, Z.-R. Tang and Y.-J. Xu, Toward Improving the Graphene–Semiconductor Composite Photoactivity via the Addition of Metal Ions as Generic Interfacial Mediator, ACS Nano, 2013, 8, 623–633 CrossRef.
- Y. Zhang, N. Zhang, Z.-R. Tang and Y.-J. Xu, Improving the photocatalytic performance of graphene–TiO2 nanocomposites via a combined strategy of decreasing defects of graphene and increasing interfacial contact, Phys. Chem. Chem. Phys., 2012, 14, 9167 RSC.
- Y. T. Liang, B. K. Vijayan, K. A. Gray and M. C. Hersam, Minimizing Graphene Defects Enhances Titania Nanocomposite-Based Photocatalytic Reduction of CO2 for Improved Solar Fuel Production, Nano Lett., 2011, 11, 2865–2870 CrossRef CAS PubMed.
- W. Tu, Y. Zhou, Q. Liu, Z. Tian, J. Gao, X. Chen, H. Zhang, J. Liu and Z. Zou, Robust Hollow Spheres Consisting of Alternating Titania Nanosheets and Graphene Nanosheets with High Photocatalytic Activity for CO2 Conversion into Renewable Fuels, Adv. Funct. Mater., 2012, 22, 1215–1221 CrossRef CAS.
- Y. T. Liang, B. K. Vijayan, O. Lyandres, K. A. Gray and M. C. Hersam, Effect of Dimensionality on the Photocatalytic Behavior of Carbon–Titania Nanosheet Composites: Charge Transfer at Nanomaterial Interfaces, J. Phys. Chem. Lett., 2012, 3, 1760–1765 CrossRef CAS.
- A. Garcia-Gallastegui, D. Iruretagoyena, V. Gouvea, M. Mokhtar, A. M. Asiri, S. N. Basahel, S. A. Al-Thabaiti, A. O. Alyoubi, D. Chadwick and M. S. P. Shaffer, Graphene Oxide as Support for Layered Double Hydroxides: Enhancing the CO2 Adsorption Capacity, Chem. Mater., 2012, 24, 4531–4539 CrossRef CAS.
- C. Li, M. Wei, D. G. Evans and X. Duan, Layered Double Hydroxide-based Nanomaterials
as Highly Efficient Catalysts and Adsorbents, Small, 2014, 10, 4469–4486 CrossRef CAS.
- J. Wang, X. Mei, L. Huang, Q. Zheng, Y. Qiao, K. Zang, S. Mao, R. Yang, Z. Zhang, Y. Gao, Z. Guo, Z. Huang and Q. Wang, Synthesis of layered double hydroxides/graphene oxide nanocomposite as a novel high-temperature CO2 adsorbent, J. Energy Chem., 2015, 24, 127–137 CrossRef.
- S. Liu, J. Xia and J. Yu, Amine-Functionalized Titanate Nanosheet-Assembled Yolk@Shell Microspheres for Efficient Cocatalyst-Free Visible-Light Photocatalytic CO2 Reduction, ACS Appl. Mater. Interfaces, 2015, 7, 8166–8175 CrossRef CAS.
- X. Li, J. Yu, S. Wageh, A. A. Al-Ghamdi and J. Xie, Graphene in Photocatalysis: A Review, Small, 2016, 12, 6640–6696 CrossRef CAS.
- Y. Liao, S.-W. Cao, Y. Yuan, Q. Gu, Z. Zhang and C. Xue, Efficient CO2 Capture and Photoreduction by Amine-Functionalized TiO2, Chem. – Eur. J., 2014, 20, 10220–10222 CrossRef CAS PubMed.
- Y. Le, D. Guo, B. Cheng and J. Yu, Amine-functionalized monodispersed porous silica microspheres with enhanced CO2 adsorption performance and good cyclic stability, J. Colloid Interface Sci., 2013, 408, 173–180 CrossRef CAS PubMed.
- J. Yu, Y. Le and B. Cheng, Fabrication and CO2 adsorption performance of bimodal porous silica hollow spheres with amine-modified surfaces, RSC Adv., 2012, 2, 6784 RSC.
- S. Xie, Y. Wang, Q. Zhang, W. Fan, W. Deng and Y. Wang, Photocatalytic reduction of CO2 with H2O: significant enhancement of the activity of Pt–TiO2 in CH4 formation by addition of MgO, Chem. Commun., 2013, 49, 2451 RSC.
- L. Liu, C. Zhao, D. Pitts, H. Zhao and Y. Li, CO2 photoreduction with H2O vapor by porous MgO–TiO2 microspheres: effects of surface MgO dispersion and CO2 adsorption–desorption dynamics, Catal. Sci. Technol., 2014, 4, 1539–1546 RSC.
- L. Liu, C. Zhao, H. Zhao, D. Pitts and Y. Li, Porous microspheres of MgO-patched TiO2 for CO2 photoreduction with H2O vapor: temperature-dependent activity and stability, Chem. Commun., 2013, 49, 3664 RSC.
- Q. Li, L. Zong, C. Li and J. Yang, Photocatalytic reduction of CO2 on MgO/TiO2 nanotube films, Appl. Surf. Sci., 2014, 314, 458–463 CrossRef CAS.
- X. Meng, S. Ouyang, T. Kako, P. Li, Q. Yu, T. Wang and J. Ye, Photocatalytic CO2 conversion over alkali modified TiO2 without loading noble metal cocatalyst, Chem. Commun., 2014, 50, 11517–11519 RSC.
-
A. Zuttel, A. Borgschulte and L. Schlapbach, Hydrogen as a Future Energy Carrier, Wiley-VCH Verlag GmbH & Co., KGaA, Weinheim, 2008 Search PubMed.
- N. Armaroli and V. Balzani, The Hydrogen Issue, ChemSusChem, 2010, 4, 21–36 CrossRef PubMed.
- G. W. Crabtree, M. S. Dresselhaus and M. V. Buchanan, The Hydrogen Economy, Phys. Today, 2004, 57, 39–44 CrossRef CAS.
- R. M. Navarro, M. A. Peña and J. L. G. Fierro, Hydrogen Production Reactions from Carbon Feedstocks: Fossil Fuels and Biomass, Chem. Rev., 2007, 107, 3952–3991 CrossRef CAS PubMed.
- G. W. Huber, S. Iborra and A. Corma, Synthesis of Transportation Fuels from Biomass: Chemistry, Catalysts, and Engineering, Chem. Rev., 2006, 106, 4044–4098 CrossRef CAS.
- S. Zinoviev, F. Müller-Langer, P. Das, N. Bertero, P. Fornasiero, M. Kaltschmitt, G. Centi and S. Miertus, Next-Generation Biofuels: Survey of Emerging Technologies and Sustainability Issues, ChemSusChem, 2010, 3, 1106–1133 CrossRef CAS PubMed.
- R. M. Navarro, M. C. Sánchez-Sánchez, M. C. Alvarez-Galvan, F. del Valle and J. L. G. Fierro, Hydrogen production from renewable sources: biomass and photocatalytic opportunities, Energy Environ. Sci., 2009, 2, 35–54 RSC.
-
T. A. Milne, C. C. Elam and R. J. Evans, Hydrogen from biomass: state of the art and research challenges, National Renewable Energy Lab. (NREL), Golden, CO (United States), 2022 DOI:10.2172/792221.
- D. M. Alonso, J. Q. Bond and J. A. Dumesic, Catalytic conversion of biomass to biofuels, Green Chem., 2010, 12, 1493 RSC.
- A. Demirbaş, Biomass resource facilities and biomass conversion processing for fuels and chemicals, Energy Convers. Manage., 2001, 42, 1357–1378 CrossRef.
- G. W. Huber and J. A. Dumesic, An overview of aqueous-phase catalytic processes for production of hydrogen and alkanes in a biorefinery, Catal. Today, 2006, 111, 119–132 CrossRef CAS.
-
H. H. Mohamed, Green processes and sustainable materials for renewable energy production via water splitting, Sustainable Materials and Green Processing for Energy Conversion, 2022, pp. 169–212 DOI:10.1016/B978-0-12-822838-8.00007-7.
- R. R. Davda, J. W. Shabaker, G. W. Huber, R. D. Cortright and J. A. Dumesic, A review of catalytic issues and process conditions for renewable hydrogen and alkanes by aqueous-phase reforming of oxygenated hydrocarbons over supported metal catalysts, Appl. Catal., B, 2005, 56, 171–186 CrossRef CAS.
- D. Wang, S. Czernik, D. Montané, M. Mann and E. Chornet, Biomass to Hydrogen via Fast Pyrolysis and Catalytic Steam Reforming of the Pyrolysis Oil or Its Fractions, Ind. Eng. Chem. Res., 1997, 36, 1507–1518 CrossRef CAS.
- L. Garcia, R. French, S. Czernik and E. Chornet, Catalytic steam reforming of bio-oils for the production of hydrogen: effects of catalyst composition, Appl. Catal., A, 2000, 201, 225–239 CrossRef CAS.
- C. Rioche, S. Kulkarni, F. C. Meunier, J. P. Breen and R. Burch, Steam reforming of model compounds and fast pyrolysis bio-oil on supported noble metal catalysts, Appl. Catal., B, 2005, 61, 130–139 CrossRef CAS.
- R. D. Cortright, R. R. Davda and J. A. Dumesic, Hydrogen from catalytic reforming of biomass-derived hydrocarbons in liquid water, Nature, 2002, 418, 964–967 CrossRef CAS PubMed.
- G. W. Huber, J. W. Shabaker and J. A. Dumesic, Raney Ni–Sn Catalyst for H2 Production from Biomass-Derived Hydrocarbons, Science, 2003, 300, 2075–2077 CrossRef CAS PubMed.
- V. Scuderi, G. Impellizzeri, L. Romano, M. Scuderi, G. Nicotra, K. Bergum, A. Irrera, B. G. Svensson and V. Privitera, TiO2-coated nanostructures for dye photo-degradation in water, Nanoscale Res. Lett., 2014, 9, 458 CrossRef PubMed.
- N. Zhang, M.-Q. Yang, S. Liu, Y. Sun and Y.-J. Xu, Waltzing with the Versatile Platform of Graphene to Synthesize Composite Photocatalysts, Chem. Rev., 2015, 115, 10307–10377 CrossRef CAS PubMed.
- J. Zhang, Z. Xiong and X. S. Zhao, Graphene–metal–oxide composites for the degradation of dyes under visible light irradiation, J. Mater. Chem., 2011, 21, 3634 RSC.
- P. Avouris and C. Dimitrakopoulos, Graphene: synthesis and applications, Mater. Today, 2012, 15, 86–97 CrossRef CAS.
- K. Zhou, Y. Zhu, X. Yang, X. Jiang and C. Li, Preparation of graphene–TiO2 composites with enhanced photocatalytic activity, New J. Chem., 2011, 35, 353–359 RSC.
- Y. Yang, L. Xu, H. Wang, W. Wang and L. Zhang, TiO2/graphene porous composite and its photocatalytic degradation of methylene blue, Mater. Des., 2016, 108, 632–639 CrossRef CAS.
- Y. Zhang and C. Pan, TiO2/graphene composite from thermal reaction of graphene
oxide and its photocatalytic activity in visible light, J. Mater. Sci., 2010, 46, 2622–2626 CrossRef.
- W.-S. Wang, D.-H. Wang, W.-G. Qu, L.-Q. Lu and A.-W. Xu, Large Ultrathin Anatase TiO2 Nanosheets with Exposed {001} Facets on Graphene for Enhanced Visible Light Photocatalytic Activity, J. Phys. Chem. C, 2012, 116, 19893–19901 CrossRef CAS.
- T. Wang, T. Tang, Y. Gao, Q. Chen, Z. Zhang and H. Bian, Hydrothermal preparation of Ag–TiO2-reduced graphene oxide ternary microspheres structure composite for enhancing photocatalytic activity, Phys. E, 2019, 112, 128–136 CrossRef CAS.
- Y. Song, F. Massuyeau, L. Jiang, Y. Dan, P. Le Rendu and T. P. Nguyen, Effect of graphene size on the photocatalytic activity of TiO2/poly(3-hexylthiophene)/graphene composite films, Catal. Today, 2019, 321–322, 74–80 CrossRef CAS.
- J. Wang, M. Wang, J. Xiong and C. Lu, Enhanced photocatalytic activity of a TiO2/graphene composite by improving the reduction degree of graphene, New Carbon Mater., 2015, 30, 357–363 CrossRef CAS.
- X. Wang, X. Wang, J. Zhao, J. Song, L. Zhou, J. Wang, X. Tong and Y. Chen, An alternative to in situ photocatalytic degradation of microcystin-LR by worm-like N,P co-doped TiO2/expanded graphite by carbon layer (NPT-EGC) floating composites, Appl. Catal., B, 2017, 206, 479–489 CrossRef CAS.
- P. Niu, L. Zhang, G. Liu and H. Cheng, Graphene-Like Carbon Nitride Nanosheets for Improved Photocatalytic Activities, Adv. Funct. Mater., 2012, 22, 4763–4770 CrossRef CAS.
- C. Xing, Z. Wu, D. Jiang and M. Chen, Hydrothermal synthesis of In2S3/g-C3N4 heterojunctions with enhanced photocatalytic activity, J. Colloid Interface Sci., 2014, 433, 9–15 CrossRef CAS.
- H. Meng, F. Jijiang, Z. Chengcheng, P. Zhiguo, L. Yong and T. Jingyuan, Photocatalytic Property under Visible Light of the Nitrogen Doped SrTiO3/TiO2 Nanotube Arrays, Rare Met. Mater. Eng., 2015, 44, 1750–1753 Search PubMed.
- L. Gomathi Devi and R. Kavitha, A review on plasmonic metal TiO2 composite for generation, trapping, storing and dynamic vectorial transfer of photogenerated electrons across the Schottky junction in a photocatalytic system, Appl. Surf. Sci., 2016, 360, 601–622 CrossRef CAS.
- N. Acosta, C. Jiménez, V. Borau and A. Heras, Extraction and characterization of chitin from crustaceans, Biomass Bioenergy, 1993, 5, 145–153 CrossRef CAS.
- C. Chen, Y. Zhang, J. Zeng, F. Zhang, K. Zhou, C. R. Bowen and D. Zhang, Aligned macroporous TiO2/chitosan/reduced graphene oxide (rGO) composites for photocatalytic applications, Appl. Surf. Sci., 2017, 424, 170–176 CrossRef CAS.
- C. Martínez-Sánchez, F. Montiel-González, E. Díaz-Cervantes and V. Rodríguez-González, Unraveling the strength interaction in a TiO2-Graphene photocatalytic nanocomposite synthesized by the microwave hydrothermal method, Mater. Sci. Semicond. Process., 2019, 101, 262–271 CrossRef.
- A. Tibodee, P. Pannak, K. Akkarachaneeyakorn, T. Thaweechai and W. Sirisaksoontorn, Use of the graphite intercalation compound to produce low-defect graphene sheets for the photocatalytic enhancement of graphene/TiO2 composites, Mater. Chem. Phys., 2019, 235, 121755 CrossRef CAS.
- A. Ziarati Saravani, M. Nadimi, M. A. Aroon and A. Ebrahimian, Pirbazari, Magnetic TiO2/NiFe2O4/reduced graphene oxide nanocomposite as a recyclable photocatalyst for photocatalytic removal of methylene blue under visible light, J. Alloys Compd., 2019, 803, 291–306 CrossRef CAS.
- J. Yang, Z. Wen, X. Shen, J. Dai, Y. Li and Y. Li, A comparative study on the photocatalytic behavior of graphene-TiO2 nanostructures: Effect of TiO2 dimensionality on interfacial charge transfer, Chem. Eng. J., 2018, 334, 907–921 CrossRef CAS.
- H. E. Garrafa-Gálvez, C. G. Alvarado-Beltrán, J. L. Almaral-Sánchez, A. Hurtado-Macías, A. M. Garzon-Fontecha, P. A. Luque and A. Castro-Beltrán, Graphene role in improved solar photocatalytic performance of TiO2-RGO nanocomposite, Chem. Phys., 2019, 521, 35–43 CrossRef.
- J.-W. Chen, B. Yuan, J.-W. Shi, J.-C. E. Yang and M.-L. Fu, Reduced graphene oxide and titania nanosheet cowrapped coal fly ash microspheres alternately as a novel photocatalyst for water treatment, Catal. Today, 2018, 315, 247–254 CrossRef CAS.
- S. Ramanathan, S. Moorthy, S. Ramasundaram, H. K. Rajan, S. Vishwanath, S. Selvinsimpson, A. Durairaj, B. Kim and S. Vasanthkumar, Grape Seed Extract Assisted Synthesis of Dual-Functional Anatase TiO2 Decorated Reduced Graphene Oxide Composite for Supercapacitor Electrode Material and Visible Light Photocatalytic Degradation of Bromophenol Blue Dye, ACS Omega, 2021, 6, 14734–14747 CrossRef CAS PubMed.
- Q. Xiang, J. Yu and M. Jaroniec, Preparation and Enhanced Visible-Light Photocatalytic H2-Production Activity of Graphene/C3N4 Composites, J. Phys. Chem. C, 2011, 115, 7355–7363 CrossRef CAS.
- M.-C. Rosu, M. Coros, F. Pogacean, L. Magerusan, C. Socaci, A. Turza and S. Pruneanu, Azo dyes degradation using TiO2-Pt/graphene oxide and TiO2-Pt/reduced graphene oxide photocatalysts under UV and natural sunlight irradiation, Solid State Sci., 2017, 70, 13–20 CrossRef CAS.
- M. F. R. Samsudin, A. Mahmood and S. Sufian, Enhanced photocatalytic degradation of wastewater over RGO-TiO2/BiVO4 photocatalyst under solar light irradiation, J. Mol. Liq., 2018, 268, 26–36 CrossRef CAS.
- R. Shahbazi, A. Payan and M. Fattahi, Preparation, evaluations and operating conditions optimization of nano TiO2 over graphene based materials as the photocatalyst for degradation of phenol, J. Photochem. Photobiol., A, 2018, 364, 564–576 CrossRef CAS.
- L. Xu, L. Yang, E. M. J. Johansson, Y. Wang and P. Jin, Photocatalytic activity and mechanism of bisphenol a removal over TiO2−x/rGO nanocomposite driven by visible light, Chem. Eng. J., 2018, 350, 1043–1055 CrossRef CAS.
- H. Khan, Z. Jiang and D. Berk, Molybdenum doped graphene/TiO2 hybrid photocatalyst for UV/visible photocatalytic applications, Sol. Energy, 2018, 162, 420–430 CrossRef CAS.
- J. Huo, C. Yuan and Y. Wang, Nanocomposites of Three-Dimensionally Ordered Porous TiO2 Decorated with Pt and Reduced Graphene Oxide for the Visible-Light Photocatalytic Degradation of Waterborne Pollutants, ACS Appl. Nano Mater., 2019, 2, 2713–2724 CrossRef CAS.
- A. Ali, M. Shoeb, Y. Li, B. Li and M. A. Khan, Enhanced photocatalytic degradation of antibiotic drug and dye pollutants by graphene-ordered mesoporous silica (SBA 15)/TiO2 nanocomposite under visible-light irradiation, J. Mol. Liq., 2021, 324, 114696 CrossRef CAS.
- D. Fu, G. Han, Y. Chang and J. Dong, The synthesis and properties of ZnO–graphene nano hybrid for photodegradation of organic pollutant in water, Mater. Chem. Phys., 2012, 132, 673–681 CrossRef CAS.
- E. R. Ezeigwe, M. T. T. Tan, P. S. Khiew and C. W. Siong, One-step green synthesis of graphene/ZnO nanocomposites for electrochemical capacitors, Ceram. Int., 2015, 41, 715–724 CrossRef CAS.
- M. Ghorbani, H. Abdizadeh, M. Taheri and M. R. Golobostanfard, Enhanced photoelectrochemical water splitting in hierarchical porous ZnO/Reduced graphene oxide nanocomposite synthesized by sol-gel method, Int. J. Hydrogen Energy, 2018, 43, 7754–7763 CrossRef CAS.
- A. Umar, M. S. Chauhan, S. Chauhan, R. Kumar, G. Kumar, S. A. Al-Sayari, S. W. Hwang and A. Al-Hajry, Large-scale synthesis of ZnO balls made of fluffy thin nanosheets by simple solution process: Structural, optical and photocatalytic properties, J. Colloid Interface Sci., 2011, 363, 521–528 CrossRef CAS PubMed.
- A. Kołodziejczak-Radzimska and T. Jesionowski, Zinc Oxide—From Synthesis to Application: A Review, Materials, 2014, 7, 2833–2881 CrossRef.
- S. P. Lonkar, V. Pillai and A. Abdala, Solvent-free synthesis of ZnO-graphene nanocomposite with superior photocatalytic activity, Appl. Surf. Sci., 2019, 465, 1107–1113 CrossRef CAS.
- R. Cai, J. Wu, L. Sun, Y. Liu, T. Fang, S. Zhu, S. Li, Y. Wang, L. Guo, C. Zhao and A. Wei, 3D graphene/ZnO composite with enhanced photocatalytic activity, Mater. Des., 2016, 90, 839–844 CrossRef CAS.
- N. P. Herring, S. H. Almahoudi, C. R. Olson and M. S. El-Shall, Enhanced photocatalytic activity of ZnO–graphene nanocomposites prepared by microwave synthesis, J. Nanopart. Res., 2012, 14, 1277 CrossRef.
- Y. Liu, Y. Hu, M. Zhou, H. Qian and X. Hu, Microwave-assisted non-aqueous route to deposit well-dispersed ZnO nanocrystals on reduced graphene oxide sheets with improved photoactivity for the decolorization of dyes under visible light, Appl. Catal., B, 2012, 125, 425–431 CrossRef CAS.
- X. Liu, L. Pan, T. Lv, T. Lu, G. Zhu, Z. Sun and C. Sun, Microwave-assisted synthesis of ZnO–graphene composite for photocatalytic reduction of Cr(VI), Catal. Sci. Technol., 2011, 1, 1189 RSC.
- P. K. Labhane, L. B. Patle, G. H. Sonawane and S. H. Sonawane, Fabrication of ternary Mn doped ZnO nanoparticles grafted on reduced graphene oxide (RGO) sheet as an efficient solar light driven photocatalyst, Chem. Phys. Lett., 2018, 710, 70–77 CrossRef CAS.
- M. Kheirabadi, M. Samadi, E. Asadian, Y. Zhou, C. Dong, J. Zhang and A. Z. Moshfegh, Well-designed Ag/ZnO/3D graphene structure for dye removal: Adsorption, photocatalysis and physical separation capabilities, J. Colloid Interface Sci., 2019, 537, 66–78 CrossRef CAS PubMed.
- B. Xue and Y. Zou, High photocatalytic activity of ZnO–graphene composite, J. Colloid Interface Sci., 2018, 529, 306–313 CrossRef CAS PubMed.
- M. Zarrabi, M. Haghighi and R. Alizadeh, Sonoprecipitation dispersion of ZnO nanoparticles over graphene oxide used in photocatalytic degradation of methylene blue in aqueous solution: Influence of irradiation time and power, Ultrason. Sonochem., 2018, 48, 370–382 CrossRef CAS PubMed.
- V. Q. Nguyen, M. L. Baynosa, V. H. Nguyen, D. Tuma, Y. R. Lee and J.-J. Shim, Solvent-driven morphology-controlled synthesis of highly efficient long-life ZnO/graphene nanocomposite photocatalysts for the practical degradation of organic wastewater under solar light, Appl. Surf. Sci., 2019, 486, 37–51 CrossRef CAS.
- S. Prabhu, M. Pudukudy, S. Sohila, S. Harish, M. Navaneethan, D. Navaneethan, R. Ramesh and Y. Hayakawa, Synthesis, structural and optical properties of ZnO spindle/reduced graphene oxide composites with enhanced photocatalytic activity under visible light irradiation, Opt. Mater., 2018, 79, 186–195 CrossRef CAS.
- L. Wang, Z. Li, J. Chen, Y. Huang, H. Zhang and H. Qiu, Enhanced photocatalytic degradation of methyl orange by porous graphene/ZnO nanocomposite, Environ. Pollut., 2019, 249, 801–811 CrossRef CAS.
- S. K. Mandal, K. Dutta, S. Pal, S. Mandal, A. Naskar, P. K. Pal, T. S. Bhattacharya, A. Singha, R. Saikh, S. De and D. Jana, Engineering of ZnO/rGO nanocomposite photocatalyst towards rapid degradation of toxic dyes, Mater. Chem. Phys., 2019, 223, 456–465 CrossRef CAS.
- Z. Wu and L. Wang, Graphene oxide (GO) doping hexagonal flower-like ZnO as potential enhancer of photocatalytic ability, Mater. Lett., 2019, 234, 287–290 CrossRef CAS.
- S. Víctor-Román, E. García-Bordejé, J. Hernández-Ferrer, J. M. González-Domínguez, A. Ansón-Casaos, A. M. T. Silva, W. K. Maser and A. M. Benito, Controlling the surface chemistry of graphene oxide: Key towards efficient ZnO-GO photocatalysts, Catal. Today, 2020, 357, 350–360 CrossRef.
- Y. Areerob, J. Y. Cho, W. K. Jang and W.-C. Oh, Enhanced sonocatalytic degradation of organic dyes from aqueous solutions by novel synthesis of mesoporous Fe3O4-graphene/ZnO@SiO2 nanocomposites, Ultrason. Sonochem., 2018, 41, 267–278 CrossRef CAS PubMed.
- S. Dehghan, A. J. Jafari, M. FarzadKia, A. Esrafili and R. R. Kalantary, Visible-light-driven photocatalytic degradation of Metalaxyl by reduced graphene oxide/Fe3O4/ZnO ternary nanohybrid: Influential factors, mechanism and toxicity bioassay, J. Photochem. Photobiol., A, 2019, 375, 280–292 CrossRef CAS.
- M. Zarrabi, M. Haghighi, R. Alizadeh and S. Mahboob, Solar-light-driven photodegradation of organic dyes on sono-dispersed ZnO nanoparticles over graphene oxide: Sono vs. conventional catalyst design, Sep. Purif. Technol., 2019, 211, 738–752 CrossRef CAS.
- M. Ebrahimi, M. Samadi, S. Yousefzadeh, M. Soltani, A. Rahimi, T. Chou, L.-C. Chen, K.-H. Chen and A. Z. Moshfegh, Improved Solar-Driven Photocatalytic Activity of Hybrid Graphene Quantum Dots/ZnO Nanowires: A Direct Z-Scheme Mechanism, ACS Sustainable Chem. Eng., 2016, 5, 367–375 CrossRef.
- M. Qiang, H. Xiaomin, L. Ke, D. Rui, H. Zhang, X. Bo and Z. Kewen, Ultrasound-enhanced preparation and photocatalytic properties of graphene-ZnO nanorod composite, Sep. Purif. Technol., 2021, 259, 118131 CrossRef CAS.
- R. Beura, R. Pachaiappan and T. Paramasivam, Photocatalytic degradation studies of organic dyes over novel Ag-loaded ZnO-graphene hybrid nanocomposites, J. Phys. Chem. Solids, 2021, 148, 109689 CrossRef CAS.
- G. Fan, F. Li, D. G. Evans and X. Duan, Catalytic applications of layered double hydroxides: recent advances and perspectives, Chem. Soc. Rev., 2014, 43, 7040–7066 RSC.
- L. Mohapatra and K. Parida, A review on the recent progress, challenges and perspective of layered double hydroxides as promising photocatalysts, J. Mater. Chem. A, 2016, 4, 10744–10766 RSC.
- M. Y. Miao, J. T. Feng, Q. Jin, Y. F. He, Y. N. Liu, Y. Y. Du, N. Zhang and D. Q. Li, Hybrid Ni–Al layered double hydroxide/graphene composite supported gold nanoparticles for aerobic selective oxidation of benzyl alcohol, RSC Adv., 2015, 5, 36066–36074 RSC.
- B. Li, Y. Zhao, S. Zhang, W. Gao and M. Wei, Visible-Light-Responsive Photocatalysts toward Water Oxidation Based on NiTi-Layered Double Hydroxide/Reduced Graphene Oxide Composite Materials, ACS Appl. Mater. Interfaces, 2013, 5, 10233–10239 CrossRef CAS.
- Z. Huang, P. Wu, B. Gong, Y. Fang and N. Zhu, Fabrication and photocatalytic properties of a visible-light responsive nanohybrid based on self-assembly of carboxyl graphene and ZnAl layered double hydroxides, J. Mater. Chem. A, 2014, 2, 5534 RSC.
- J. L. Gunjakar, I. Y. Kim, J. M. Lee, N.-S. Lee and S.-J. Hwang, Self-assembly of layered double hydroxide 2D nanoplates with graphene nanosheets: an effective way to improve the photocatalytic activity of 2D nanostructured materials for visible light-induced O2 generation, Energy Environ. Sci., 2013, 6, 1008 RSC.
- Z. Wang, F. Zhang, H. Zou, Y. Yuan, H. Wang, J. Xia and Z. Wang, Preparation of a Pt/NiFe layered double hydroxide/reduced graphene oxide composite as an electrocatalyst for methanol oxidation, J. Electroanal. Chem., 2018, 818, 198–203 CrossRef CAS.
- W.-K. Jo and S. Tonda, Novel CoAl-LDH/g-C3N4/RGO ternary heterojunction with notable 2D/2D/2D configuration for highly efficient visible-light-induced photocatalytic elimination of dye and antibiotic pollutants, J. Hazard. Mater., 2019, 368, 778–787 CrossRef CAS PubMed.
- W.-K. Jo and S. Tonda, Novel CoAl-LDH/g-C3N4/RGO ternary heterojunction with notable 2D/2D/2D configuration for highly efficient visible-light-induced photocatalytic elimination of dye and antibiotic pollutants, J. Hazard. Mater., 2019, 368, 778–787 CrossRef CAS.
- X. Lang, X. Chen and J. Zhao, Heterogeneous visible light photocatalysis for selective organic transformations, Chem. Soc. Rev., 2014, 43, 473–486 RSC.
- S. Liu, Z. Chen, N. Zhang, Z.-R. Tang and Y.-J. Xu, An Efficient Self-Assembly of CdS Nanowires–Reduced Graphene Oxide Nanocomposites for Selective Reduction of Nitro Organics under Visible Light Irradiation, J. Phys. Chem. C, 2013, 117, 8251–8261 CrossRef CAS.
- S. Mishra and R. Acharya, Photocatalytic applications of graphene based semiconductor composites: A review, Mater. Today: Proc., 2021, 35, 164–169 CAS.
- P. Roy, A. P. Periasamy, C.-T. Liang and H.-T. Chang, Synthesis of Graphene-ZnO-Au Nanocomposites for Efficient Photocatalytic Reduction of Nitrobenzene, Environ. Sci. Technol., 2013, 47, 6688–6695 CrossRef CAS PubMed.
- D. P. Das, R. K. Barik, J. Das, P. Mohapatra and K. M. Parida, Visible light induced photo-hydroxylation of phenol to catechol over RGO–Ag3VO4 nanocomposites without the use of H2O2, RSC Adv., 2012, 2, 7377 RSC.
- N. Zhang, Y. Zhang, X. Pan, X. Fu, S. Liu and Y.-J. Xu, Assembly of CdS Nanoparticles on the Two-Dimensional Graphene Scaffold as Visible-Light-Driven Photocatalyst for Selective Organic Transformation under Ambient Conditions, J. Phys. Chem. C, 2011, 115, 23501–23511 CrossRef CAS.
- A. Chwalibog, E. Sawosz, A. Hotowy, J. Szeliga, S. Mitura, K. Mitura and M. Grodzik, Visualization of interaction between inorganic nanoparticles and bacteria or fungi, Int. J. Nanomed., 2010, 1085 CrossRef.
- L. Xiong and J. Tang, Strategies and Challenges on Selectivity of Photocatalytic Oxidation of Organic Substances, Adv. Energy Mater., 2021, 11(8), 2003216 CrossRef CAS.
- V. Sambhy, M. M. MacBride, B. R. Peterson and A. Sen, Silver Bromide Nanoparticle/Polymer Composites: Dual Action Tunable Antimicrobial Materials, J. Am. Chem. Soc., 2006, 128, 9798–9808 CrossRef CAS.
- K. Sunada, M. Minoshima and K. Hashimoto, Highly efficient antiviral and antibacterial activities of solid-state cuprous compounds, J. Hazard. Mater., 2012, 235–236, 265–270 CrossRef CAS PubMed.
- T. Matsunaga, R. Tomoda, T. Nakajima and H. Wake, Photoelectrochemical sterilization of microbial cells by semiconductor powders, FEMS Microbiol. Lett., 1985, 29, 211–214 CrossRef CAS.
- J. Gamage McEvoy and Z. Zhang, Antimicrobial and photocatalytic disinfection mechanisms in silver-modified photocatalysts under dark and light conditions, J. Photochem. Photobiol., C, 2014, 19, 62–75 CrossRef CAS.
- A. Markowska-Szczupak, K. Ulfig and A. W. Morawski, The application of titanium dioxide for deactivation of bioparticulates: An overview, Catal. Today, 2011, 169, 249–257 CrossRef CAS.
- D. Mitoraj, A. Jańczyk, M. Strus, H. Kisch, G. Stochel, P. B. Heczko and W. Macyk, Visible light inactivation of bacteria and fungi by modified titanium dioxide, Photochem. Photobiol. Sci., 2007, 6, 642–648 CrossRef CAS PubMed.
- A. Markowska-Szczupak, K. Wang, P. Rokicka, M. Endo, Z. Wei, B. Ohtani, A. W. Morawski and E. Kowalska, The effect of anatase and rutile crystallites isolated from titania P25 photocatalyst on growth of selected mould fungi, J. Photochem. Photobiol., B, 2015, 151, 54–62 CrossRef CAS PubMed.
- A. Markowska-Szczupak, M. Endo-Kimura, O. Paszkiewicz and E. Kowalska, Are Titania Photocatalysts and Titanium Implants Safe? Review on the Toxicity of Titanium Compounds, Nanomaterials, 2020, 10, 2065 CrossRef CAS PubMed.
- O. Akhavan and E. Ghaderi, Photocatalytic Reduction of Graphene Oxide Nanosheets on TiO2 Thin Film for Photoinactivation of Bacteria in Solar Light Irradiation, J. Phys. Chem. C, 2009, 113, 20214–20220 CrossRef CAS.
- O. Akhavan and E. Ghaderi, Toxicity of Graphene and Graphene Oxide Nanowalls Against Bacteria, ACS Nano, 2010, 4, 5731–5736 CrossRef CAS PubMed.
- P. Noorunnisa Khanam and A. Hasan, Biosynthesis and characterization of graphene by using non-toxic reducing agent from Allium Cepa extract: Anti-bacterial properties, Int. J. Biol. Macromol., 2019, 126, 151–158 CrossRef CAS PubMed.
- V. Palmieri, F. Bugli, M. C. Lauriola, M. Cacaci, R. Torelli, G. Ciasca, C. Conti, M. Sanguinetti, M. Papi and M. De, Spirito, Bacteria Meet Graphene: Modulation of Graphene Oxide Nanosheet Interaction with Human Pathogens for Effective Antimicrobial Therapy, ACS Biomater. Sci. Eng., 2017, 3, 619–627 CrossRef CAS PubMed.
- J. Jira, B. Rezek, V. Kriha, A. Artemenko, I. Matolínová, V. Skakalova, P. Stenclova and A. Kromka, Inhibition of E. coli Growth by Nanodiamond and Graphene Oxide Enhanced by Luria-Bertani Medium, Nanomaterials, 2018, 8, 140 CrossRef.
- A. Gusev, O. Zakharova, D. S. Muratov, N. S. Vorobeva, M. Sarker, I. Rybkin, D. Bratashov, E. Kolesnikov, A. Lapanje, D. V. Kuznetsov and A. Sinitskii, Medium-Dependent Antibacterial Properties and Bacterial Filtration Ability of Reduced Graphene Oxide, Nanomaterials, 2019, 9, 1454 CrossRef CAS.
- X. Li, J. Sun, Y. Che, Y. Lv and F. Liu, Antibacterial properties of chitosan chloride-graphene oxide composites modified quartz sand filter media in water treatment, Int. J. Biol. Macromol., 2019, 121, 760–773 CrossRef CAS.
- A. B. Alayande, S. Chae and I. S. Kim, Surface morphology-dependent spontaneous bacterial behaviors on graphene oxide membranes, Sep. Purif. Technol., 2019, 226, 68–74 CrossRef CAS.
- K. Biswas, D. De, J. Bandyopadhyay and P. Sen, Differential antibacterial response exhibited by graphene nanosheets toward Gram-positive bacterium Staphylococcus aureus, IET Nanobiotechnol., 2018, 12, 733–740 CrossRef PubMed.
- S. Liu, T. H. Zeng, M. Hofmann, E. Burcombe, J. Wei, R. Jiang, J. Kong and Y. Chen, Antibacterial Activity of Graphite, Graphite Oxide, Graphene Oxide, and Reduced Graphene Oxide: Membrane and Oxidative Stress, ACS Nano, 2011, 5, 6971–6980 CrossRef CAS PubMed.
- K. Krishnamoorthy, M. Veerapandian, L.-H. Zhang, K. Yun and S. J. Kim, Antibacterial Efficiency of Graphene Nanosheets against Pathogenic Bacteria via Lipid Peroxidation, J. Phys. Chem. C, 2012, 116, 17280–17287 CrossRef CAS.
- J. Li, G. Wang, H. Zhu, M. Zhang, X. Zheng, Z. Di, X. Liu and X. Wang, Antibacterial activity of large-area monolayer graphene film manipulated by charge transfer, Sci. Rep., 2014, 4, 4359 CrossRef PubMed.
- H. N. Nguyen, C. Chaves-Lopez, R. C. Oliveira, A. Paparella and D. F. Rodrigues, Cellular and metabolic approaches to investigate the effects of graphene and graphene oxide in the fungi Aspergillus flavus and Aspergillus niger, Carbon, 2019, 143, 419–429 CrossRef CAS.
- M. Di Giulio, R. Zappacosta, S. Di Lodovico, E. Di Campli, G. Siani, A. Fontana and L. Cellini, Antimicrobial and Antibiofilm Efficacy of Graphene Oxide against Chronic Wound Microorganisms, Antimicrob. Agents Chemother., 2018, 62 DOI:10.1128/aac.00547-18.
- R. Diez-Orejas, M. J. Feito, M. Cicuéndez, L. Casarrubios, J. M. Rojo and M. T. Portolés, Graphene oxide nanosheets increase Candida albicans killing by pro-inflammatory and reparative peritoneal macrophages, Colloids Surf., B, 2018, 171, 250–259 CrossRef CAS.
- P. Rajiv Gandhi and G. Chandramohan, Preparation Of Novel Chitosan-Graphene Oxide/Tin Oxide Nanocomposites For Anti-Bacterial Activities, Dig. J. Nanomater. Bios., 2020, 15, 561–568 CrossRef.
- M.-C. Yang, Y.-Q. Tseng, K.-H. Liu, Y.-W. Cheng, W.-T. Chen, W.-T. Chen, C.-W. Hsiao, M.-C. Yung, C.-C. Hsu and T.-Y. Liu, Preparation of Amphiphilic Chitosan–Graphene Oxide–Cellulose Nanocrystalline Composite Hydrogels and Their Biocompatibility and Antibacterial Properties, Appl. Sci., 2019, 9, 3051 CrossRef CAS.
- P. C. Bandara, E. T. Nadres, J. Peña-Bahamonde and D. F. Rodrigues, Impact of water chemistry, shelf-life, and regeneration in the removal of different chemical and biological contaminants in water by a model Polymeric Graphene Oxide Nanocomposite Membrane Coating, J. Water Process Eng., 2019, 32, 100967 CrossRef.
- F. Bugli, M. Cacaci, V. Palmieri, R. Di Santo, R. Torelli, G. Ciasca, M. Di Vito, A. Vitali, C. Conti, M. Sanguinetti, M. De Spirito and M. Papi, Curcumin-loaded graphene oxide flakes as an effective antibacterial system against methicillin-resistant Staphylococcus aureus, Interface Focus, 2018, 8, 20170059 CrossRef CAS.
- R. Ghorbanzadeh, H. Assadian, N. Chiniforush, S. Parker, B. Pourakbari, B. Ehsani, M. Y. Alikhani and A. Bahador, Modulation of virulence in Enterococcus faecalis cells surviving antimicrobial photodynamic inactivation with reduced graphene oxide-curcumin: An ex vivo biofilm model, Photodiagn. Photodyn. Ther., 2020, 29, 101643 CrossRef CAS PubMed.
- F. De Maio, V. Palmieri, G. Santarelli, G. Perini, A. Salustri, I. Palucci, M. Sali, J. Gervasoni, A. Primiano, G. Ciasca, M. Sanguinetti, M. De Spirito, G. Delogu and M. Papi, Graphene Oxide-Linezolid Combination as Potential New Anti-Tuberculosis Treatment, Nanomaterials, 2020, 10, 1431 CrossRef CAS PubMed.
- Y. Gao, J. Wu, X. Ren, X. Tan, T. Hayat, A. Alsaedi, C. Cheng and C. Chen, Impact of graphene oxide on the antibacterial activity of antibiotics against bacteria, Environ. Sci.: Nano, 2017, 4, 1016–1024 RSC.
- M. Ramasamy, S. S. Nanda, J.-H. Lee and J. Lee, Construction of Alizarin Conjugated Graphene Oxide Composites for Inhibition of Candida albicans Biofilms, Biomolecules, 2020, 10, 565 CrossRef CAS.
- R. C. Goy, D. de Britto and O. B. G. Assis, A review of the antimicrobial activity of chitosan, Polímeros, 2009, 19, 241–247 CrossRef CAS.
- I. M. Helander, E.-L. Nurmiaho-Lassila, R. Ahvenainen, J. Rhoades and S. Roller, Chitosan disrupts the barrier properties of the outer membrane of Gram-negative bacteria, Int. J. Food Microbiol., 2001, 71, 235–244 CrossRef CAS.
- J.-H. Lee, Y.-G. Kim, S. Yong Ryu and J. Lee, Calcium-chelating alizarin and other anthraquinones inhibit biofilm formation and the hemolytic activity of Staphylococcus aureus, Sci. Rep., 2016, 6, 19267 CrossRef CAS.
- R. K. Manoharan, J.-H. Lee, Y.-G. Kim and J. Lee, Alizarin and Chrysazin Inhibit Biofilm and Hyphal Formation by Candida albicans, Front. Cell. Infect. Microbiol., 2017, 7 DOI:10.3389/fcimb.2017.00447.
- H. Naeem, M. Ajmal, R. B. Qureshi, S. T. Muntha, M. Farooq and M. Siddiq, Facile synthesis of graphene oxide–silver nanocomposite for decontamination of water from multiple pollutants by adsorption, catalysis and antibacterial activity, J. Environ. Manage., 2019, 230, 199–211 CrossRef CAS.
- J. Peng, J. Lin, Z. Chen, M. Wei, Y. Fu, S. Lu, D. Yu and W. Zhao, Enhanced antimicrobial activities of silver-nanoparticle-decorated reduced graphene nanocomposites against oral pathogens, Mater. Sci. Eng., C, 2017, 71, 10–16 CrossRef CAS.
- K. Ko, M.-J. Kim, J.-Y. Lee, W. Kim and H. Chung, Effects of graphene oxides and silver-graphene oxides on aquatic microbial activity, Sci. Total Environ., 2019, 651, 1087–1095 CrossRef CAS PubMed.
- M. Cobos, I. De-La-Pinta, G. Quindós, M. J. Fernández and M. D. Fernández, Graphene Oxide–Silver Nanoparticle Nanohybrids: Synthesis, Characterization, and Antimicrobial Properties, Nanomaterials, 2020, 10, 376 CrossRef CAS PubMed.
- M. Cobos, I. De-La-Pinta, G. Quindós, M. Fernández and M. Fernández, Synthesis, Physical, Mechanical and Antibacterial Properties of Nanocomposites Based on Poly(vinyl alcohol)/Graphene Oxide–Silver Nanoparticles, Polymers, 2020, 12, 723 CrossRef CAS PubMed.
- S. V. Agarwalla, K. Ellepola, M. C. F. da Costa, G. J. M. Fechine, J. L. P. Morin, A. H. Castro Neto, C. J. Seneviratne and V. Rosa, Hydrophobicity of graphene as a driving force for inhibiting biofilm formation of pathogenic bacteria and fungi, Dent. Mater., 2019, 35, 403–413 CrossRef CAS PubMed.
- A. Arshad, J. Iqbal and Q. Mansoor, NiO-nanoflakes grafted graphene: an excellent photocatalyst and a novel nanomaterial for achieving complete pathogen control, Nanoscale, 2017, 9, 16321–16328 RSC.
- S. N. Kozuszko, M. A. Sánchez, M. I. G. de Ferro, A. M. Sfer, A. P. M. Madrid, K. Takabatake, K. Nakano, H. Nagatsuka and A. P. Rodríguez, Antibacterial Activity and Biocompability of Zinc Oxide and Graphite Particles as Endodontic Materials, J. Hard Tissue Biol., 2017, 26, 311–318 CrossRef CAS.
- A. F. Ghanem, A. A. Badawy, M. E. Mohram and M. H. Abdel, Rehim, Synergistic effect of zinc oxide nanorods on the photocatalytic performance and the biological activity of graphene nano sheets, Heliyon, 2020, 6, e03283 CrossRef.
- G. Ficociello, M. De Caris, G. Trillò, D. Cavallini, M. Sarto, D. Uccelletti and P. Mancini, Anti-Candidal Activity and In Vitro Cytotoxicity Assessment of Graphene Nanoplatelets Decorated with Zinc Oxide Nanorods, Nanomaterials, 2018, 8, 752 CrossRef.
- M. Janczarek, M. Endo-Kimura, Z. Wei, Z. Bielan, T. R. Mogan, T. M. Khedr, K. Wang, A. Markowska-Szczupak and E. Kowalska, Novel Structures and Applications of Graphene-Based Semiconductor Photocatalysts: Faceted Particles, Photonic Crystals, Antimicrobial and Magnetic Properties, Appl. Sci., 2021, 11, 1982 CrossRef CAS.
- Y. Liu, X. Zeng, X. Hu, J. Hu, Z. Wang, Y. Yin, C. Sun and X. Zhang, Two-dimensional g-C3N4/TiO2 nanocomposites as vertical Z-scheme heterojunction for improved photocatalytic water disinfection, Catal. Today, 2019, 335, 243–251 CrossRef CAS.
- B. R. Cruz-Ortiz, J. W. J. Hamilton, C. Pablos, L. Díaz-Jiménez, D. A. Cortés-Hernández, P. K. Sharma, M. Castro-Alférez, P. Fernández-Ibañez, P. S. M. Dunlop and J. A. Byrne, Mechanism of photocatalytic disinfection using titania-graphene composites under UV and visible irradiation, Chem. Eng. J., 2017, 316, 179–186 CrossRef CAS.
- R. Luo, Z. Xu and X. Lin, Preparation and characterisation of silver nanoparticles in an aqueous suspension of TETA modified graphene oxide and their antibacterial activity, Micro Nano Lett., 2018, 13, 369–373 CrossRef CAS.
- M. Cobos, I. De-La-Pinta, G. Quindós, M. J. Fernández and M. D. Fernández, One-step eco-friendly synthesized silver-graphene oxide/poly(vinyl alcohol) antibacterial nanocomposites, Carbon, 2019, 150, 101–116 CrossRef CAS.
- W. Xu, W. Xie, X. Huang, X. Chen, N. Huang, X. Wang and J. Liu, The graphene oxide and chitosan biopolymer loads TiO2 for antibacterial and preservative research, Food Chem., 2017, 221, 267–277 CrossRef CAS.
- H. Zhang, X. Lv, Y. Li, Y. Wang and J. Li, P25-Graphene Composite as a High Performance Photocatalyst, ACS Nano, 2009, 4, 380–386 CrossRef.
- M. Paszkiewicz-Gawron, E. Kowalska, M. Endo-Kimura, J. Zwara, A. Pancielejko, K. Wang, W. Lisowski, J. Łuczak, A. Zaleska-Medynska and E. Grabowska-Musiał, Stannates, titanates and tantalates modified with carbon and graphene quantum dots for enhancement of visible-light photocatalytic activity, Appl. Surf. Sci., 2021, 541, 148425 CrossRef CAS.
- K. A. Whitehead, M. Vaidya, C. M. Liauw, D. A. C. Brownson, P. Ramalingam, J. Kamieniak, S. J. Rowley-Neale, L. A. Tetlow, J. S. T. Wilson-Nieuwenhuis, D. Brown, A. J. McBain, J. Kulandaivel and C. E. Banks, Antimicrobial activity of graphene oxide-metal hybrids, Int. Biodeterior. Biodegrad., 2017, 123, 182–190 CrossRef CAS.
- T. Pulingam, K. L. Thong, Md. E. Ali, J. N. Appaturi, I. J. Dinshaw, Z. Y. Ong and B. F. Leo, Graphene oxide exhibits differential mechanistic action towards Gram-positive and Gram-negative bacteria, Colloids Surf., B, 2019, 181, 6–15 CrossRef CAS PubMed.
- M. G. Stanford, J. T. Li, Y. Chen, E. A. McHugh, A. Liopo, H. Xiao and J. M. Tour, Self-Sterilizing Laser-Induced Graphene Bacterial Air Filter, ACS Nano, 2019, 13, 11912–11920 CrossRef CAS.
- L. Tang, L. Liu, Q. Chen, F. Yang and X. Quan, The construction and performance of photocatalytic-fuel-cell with Fe–MoS2/reduced graphene oxide@carbon fiber cloth and ZnFe2O4/Ag/Ag3VO4@carbon felt as photo electrodes, Electrochim. Acta, 2020, 362, 137037 CrossRef CAS.
- L. Tang, L. Liu, Q. Chen, F. Yang and X. Quan, The construction and performance of photocatalytic-fuel-cell with Fe–MoS2/reduced graphene oxide@carbon fiber cloth and ZnFe2O4/Ag/Ag3VO4@carbon felt as photo electrodes, Electrochim. Acta, 2020, 362, 137037 CrossRef CAS.
- M. S. Sajna, S. M. Simon, N. V. Unnikrishnan and K. K. Sadasivuni, An Overview of Graphene-Based 2D/3D Nanostructures for Photocatalytic Applications, Top. Catal., 2022 DOI:10.1007/s11244-021-01539-5.
- World Health Organization, https://www.who.int/news-room/fact-sheets/detail/ambient-(outdoor)-air-quality-and-health, (accessed October 15, 2023).
- G. Liu and P.-X. Gao, A review of NOx storage/reduction catalysts: mechanism, materials and degradation studies, Catal. Sci. Technol., 2011, 1, 552 RSC.
- K. Teramura, T. Tanaka and T. Funabiki, Photoassisted Selective Catalytic Reduction of NO with Ammonia in the Presence of Oxygen over TiO2, Langmuir, 2003, 19, 1209–1214 CrossRef CAS.
- N. Bowering, G. S. Walker and P. G. Harrison, Photocatalytic decomposition and reduction reactions of nitric oxide over Degussa P25, Appl. Catal., B, 2006, 62, 208–216 CrossRef CAS.
- X. Li, X. Yan, X. Lu, S. Zuo, Z. Li, C. Yao and C. Ni, Photo-assisted selective catalytic reduction of NO by Z-scheme natural clay based photocatalyst: Insight into the effect of graphene coupling, J. Catal., 2018, 357, 59–68 CrossRef.
- Y. Jia, S. Li, J. Gao, G. Zhu, F. Zhang, X. Shi, Y. Huang and C. Liu, Highly efficient (BiO)2CO3–BiO2−x-graphene photocatalysts: Z-Scheme photocatalytic mechanism for their enhanced photocatalytic removal of NO, Appl. Catal., B, 2019, 240, 241–252 CrossRef CAS.
- J. Hu, D. Chen, N. Li, Q. Xu, H. Li, J. He and J. Lu, Fabrication of graphitic-C3N4 quantum dots/graphene-InVO4 aerogel hybrids with enhanced photocatalytic NO removal under visible-light irradiation, Appl. Catal., B, 2018, 236, 45–52 CrossRef CAS.
- Y. Li, X. Wu, W. Ho, K. Lv, Q. Li, M. Li and S. C. Lee, Graphene-induced formation of visible-light-responsive SnO2-Zn2SnO4 Z-scheme photocatalyst with surface vacancy for the enhanced photoreactivity towards NO and acetone oxidation, Chem. Eng. J., 2018, 336, 200–210 CrossRef CAS.
- X. Li, X. Yan, X. Lu, S. Zuo, Z. Li, C. Yao and C. Ni, Photo-assisted selective catalytic reduction of NO by Z-scheme natural clay based photocatalyst: Insight into the effect of graphene coupling, J. Catal., 2018, 357, 59–68 CrossRef.
- J. Hu, D. Chen, N. Li, Q. Xu, H. Li, J. He and J. Lu, 3D Aerogel of Graphitic Carbon Nitride Modified with Perylene Imide and Graphene Oxide for Highly Efficient Nitric Oxide Removal under Visible Light, Small, 2018, 14(19), e1800416 CrossRef PubMed.
- S. Xiao, D. Pan, R. Liang, W. Dai, Q. Zhang, G. Zhang, C. Su, H. Li and W. Chen, Bimetal MOF derived mesocrystal ZnCO2O4 on rGO with High performance in visible-light photocatalytic NO oxidization, Appl. Catal., B, 2018, 236, 304–313 CrossRef CAS.
- X. Li, W. Zhang, W. Cui, Y. Sun, G. Jiang, Y. Zhang, H. Huang and F. Dong, Bismuth spheres assembled on graphene oxide: Directional charge transfer enhances plasmonic photocatalysis and in situ DRIFTS studies, Appl. Catal., B, 2018, 221, 482–489 CrossRef CAS.
- R. Zhang, M. Ma, Q. Zhang, F. Dong and Y. Zhou, Multifunctional g-C3N4/graphene oxide wrapped sponge monoliths as highly efficient adsorbent and photocatalyst, Appl. Catal., B, 2018, 235, 17–25 CrossRef CAS.
- Z. Wang, S. Yan, Y. Sun, T. Xiong, F. Dong and W. Zhang, Bi metal sphere/graphene oxide nanohybrids with enhanced direct plasmonic photocatalysis, Appl. Catal., B, 2017, 214, 148–157 CrossRef CAS.
- L. Yang, Y. Liu, R. Zhang, W. Li, P. Li, X. Wang and Y. Zhou, Enhanced visible-light photocatalytic performance of a monolithic tungsten oxide/graphene oxide aerogel for nitric oxide oxidation, Chin. J. Catal., 2018, 39, 646–653 CrossRef CAS.
- M. Chen, Y. Huang, J. Yao, J. Cao and Y. Liu, Visible-light-driven N-(BiO)2CO3/Graphene oxide composites with improved photocatalytic activity and selectivity for NOx removal, Appl. Surf. Sci., 2018, 430, 137–144 CrossRef CAS.
- C. Xue, X. Yan, H. An, H. Li, J. Wei and G. Yang, Bonding CdS-Sn2S3 eutectic clusters on graphene nanosheets with unusually photoreaction-driven structural reconfiguration effect for excellent H2 evolution and Cr(VI) reduction, Appl. Catal., B, 2018, 222, 157–166 CrossRef CAS.
- Z. Fang, Q. Li, L. Su, J. Chen, K.-C. Chou and X. Hou, Efficient synergy of photocatalysis and adsorption of hexavalent chromium and rhodamine B over Al4SiC4/rGO hybrid photocatalyst under visible-light irradiation, Appl. Catal., B, 2019, 241, 548–560 CrossRef CAS.
- J. Yang, J. Hao, S. Xu, J. Dai, Y. Wang and X. Pang, Visible-light-driven photocatalytic degradation of 4-CP and the synergistic reduction of Cr(VI) on one-pot synthesized amorphous Nb2O5 nanorods/graphene heterostructured composites, Chem. Eng. J., 2018, 353, 100–114 CrossRef CAS.
- G. Mamba, G. Gangashe, L. Moss, S. Hariganesh, S. Thakur, S. Vadivel, A. K. Mishra, G. D. Vilakati, V. Muthuraj and T. T. I. Nkambule, State of the art on the photocatalytic applications of graphene based nanostructures: From elimination of hazardous pollutants to disinfection and fuel generation, J. Environ. Chem. Eng., 2020, 8, 103505 CrossRef CAS.
- J. Zhu, Z. Zhu, H. Zhang, H. Lu, W. Zhang, Y. Qiu, L. Zhu and S. Küppers, Calcined layered double hydroxides/reduced graphene oxide composites with improved photocatalytic degradation of paracetamol and efficient oxidation-adsorption of As(III), Appl. Catal., B, 2018, 225, 550–562 CrossRef CAS.
- Z. Ren, L. Li, B. Liu, X. Liu, Z. Li, X. Lei, C. Li, Y. Gong, L. Niu and L. Pan, Cr(VI) reduction in presence of ZnS/RGO photocatalyst under full solar spectrum radiation from UV/vis to near-infrared light, Catal. Today, 2018, 315, 46–51 CrossRef CAS.
- J. Wu, B. Liu, Z. Ren, M. Ni, C. Li, Y. Gong, W. Qin, Y. Huang, C. Q. Sun and X. Liu, CuS/RGO hybrid photocatalyst for full solar spectrum photoreduction from UV/Vis to near-infrared light, J. Colloid Interface Sci., 2018, 517, 80–85 CrossRef CAS.
- P. Xu, S. Huang, Y. Lv, Y. Chen, M. Liu and H. Fan, Surfactant-assisted hydrothermal synthesis of rGO/SnIn4S8 nanosheets and their application in complete removal of Cr(VI), RSC Adv., 2018, 8, 5749–5759 RSC.
- F. Zhang, Y. Zhang, G. Zhang, Z. Yang, D. D. Dionysiou and A. Zhu, Exceptional synergistic enhancement of the photocatalytic activity of SnS2 by coupling with polyaniline and N-doped reduced graphene oxide, Appl. Catal., B, 2018, 236, 53–63 CrossRef CAS.
- L. Zhang, A. Wang, N. Zhu, B. Sun, Y. Liang and W. Wu, Synthesis of butterfly-like BiVO 4/RGO nanocomposites and their photocatalytic activities, Chin. J. Chem. Eng., 2018, 26, 667–674 CrossRef CAS.
- H. Zhang, X. Wang, N. Li, J. Xia, Q. Meng, J. Ding and J. Lu, Synthesis and characterization of TiO2/graphene oxide nanocomposites for photoreduction of heavy metal ions in reverse osmosis concentrate, RSC Adv., 2018, 8, 34241–34251 RSC.
- S. M. Baizaee, M. Arabi and A. R. Bahador, A simple, one-pot, low temperature and pressure route for the synthesis of RGO/ZnO nanocomposite and investigating its photocatalytic activity, Mater. Sci. Semicond. Process., 2018, 82, 135–142 CrossRef CAS.
- M. Wu, L. Li, Y. Xue, G. Xu, L. Tang, N. Liu and W. Huang, Fabrication of ternary GO/g-C3N4/MoS2 flower-like heterojunctions with enhanced photocatalytic activity for water remediation, Appl. Catal., B, 2018, 228, 103–112 CrossRef CAS.
- A. Samal and D. P. Das, Transfiguring UV light active “metal oxides” to visible light active photocatayst by reduced graphene oxide hypostatization, Catal. Today, 2018, 300, 124–135 CrossRef CAS.
- J. Ding, J. Ming, D. Lu, W. Wu, M. Liu, X. Zhao, C. Li, M. Yang and P. Fang, Study of the enhanced visible-light-sensitive photocatalytic activity of Cr2O3-loaded titanate nanosheets for Cr(VI) degradation and H2 generation, Catal. Sci. Technol., 2017, 7, 2283–2297 RSC.
- L. Kashinath, K. Namratha and K. Byrappa, Microwave mediated synthesis and characterization of CeO2-GO hybrid composite for removal of chromium ions and its antibacterial efficiency, J. Environ. Sci., 2019, 76, 65–79 CrossRef CAS.
- D. K. Padhi, T. K. Panigrahi, K. Parida, S. K. Singh and P. M. Mishra, Green Synthesis of Fe3O4/RGO Nanocomposite with Enhanced Photocatalytic Performance for Cr(VI) Reduction, Phenol Degradation, and Antibacterial Activity, ACS Sustainable Chem. Eng., 2017, 5, 10551–10562 CrossRef CAS.
- M. de Los, Á. Bernal-Romero del Hombre Bueno, N. Boluda-Botella and D. Prats Rico, Removal of emerging pollutants in water treatment plants: adsorption of methyl and propylparaben onto powdered activated carbon, Adsorption, 2019, 25, 983–999 CrossRef.
- D. Barcelo and Y. Pico, Case studies of macro- and microplastics pollution in coastal waters and rivers: Is there a solution with new removal technologies and policy actions?, Case Stud. Chem. Environ. Eng., 2020, 2, 100019 CrossRef.
- P. U. Iyare, S. K. Ouki and T. Bond, Microplastics removal in wastewater treatment plants: a critical review, Environ. Sci.: Water Res. Technol., 2020, 6, 2664–2675 RSC.
- N. B. Hartmann, S. Rist, J. Bodin, L. H. Jensen, S. N. Schmidt, P. Mayer, A. Meibom and A. Baun, Microplastics as vectors for environmental contaminants: Exploring sorption, desorption, and transfer to biota, Integr. Environ. Assess. Manage., 2017, 13, 488–493 CrossRef.
- A. L. P. Silva, J. C. Prata, A. C. Duarte, A. M. V. M. Soares, D. Barceló and T. Rocha-Santos, Microplastics in landfill leachates: The need for reconnaissance studies and remediation technologies, Case Stud. Chem. Environ. Eng., 2021, 3, 100072 CrossRef CAS.
- K. B. Sundbæk, I. D. W. Koch, C. G. Villaro, N. S. Rasmussen, S. L. Holdt and N. B. Hartmann, Sorption of fluorescent polystyrene microplastic particles to edible seaweed Fucus vesiculosus, J. Appl. Phycol., 2018, 30, 2923–2927 CrossRef.
- S. Sharma and S. Chatterjee, Microplastic pollution, a threat to marine ecosystem and human health: a short review, Environ. Sci. Pollut. Res., 2017, 24, 21530–21547 CrossRef PubMed.
- A. McCormick, T. J. Hoellein, S. A. Mason, J. Schluep and J. J. Kelly, Microplastic is an Abundant and Distinct Microbial Habitat in an Urban River, Environ. Sci. Technol., 2014, 48, 11863–11871 CrossRef CAS.
- F. Yuan, L. Yue, H. Zhao and H. Wu, Study on the adsorption of polystyrene microplastics by three-dimensional reduced graphene oxide, Water Sci. Technol., 2020, 81, 2163–2175 CrossRef CAS PubMed.
- F. Almomani, R. Bhosale, M. Khraisheh, A. Kumar and T. Almomani, Heavy metal ions removal from industrial wastewater using magnetic nanoparticles (MNP), Appl. Surf. Sci., 2020, 506, 144924 CrossRef CAS.
- H. A. Waad, Al-M. Dana, Al-O. Amani, H. Neda and T. Muhammad, Insights into the removal of microplastics from water using biochar in the era of COVID-19: A mini review, Case Stud. Chem. Environ. Eng., 2021, 4, 100151 CrossRef.
- M. Al Sharabati, R. Abokwiek, A. Al-Othman, M. Tawalbeh, C. Karaman, Y. Orooji and F. Karimi, Biodegradable polymers and their nano-composites for the removal of endocrine-disrupting chemicals (EDCs) from wastewater: A review, Environ. Res., 2021, 202, 111694 CrossRef CAS.
- J. V. Gulmine, P. R. Janissek, H. M. Heise and L. Akcelrud, Degradation profile of polyethylene after artificial accelerated weathering, Polym. Degrad. Stab., 2003, 79, 385–397 CrossRef CAS.
- A. Al Bsoul, M. Hailat, A. Abdelhay, M. Tawalbeh, I. Jum’h and K. Bani-Melhem, Treatment of olive mill effluent by adsorption on titanium oxide nanoparticles, Sci. Total Environ., 2019, 688, 1327–1334 CrossRef CAS PubMed.
- W. F. Elmobarak and F. Almomani, Application of Fe3O4 magnetite nanoparticles grafted in silica (SiO2) for oil recovery from oil in water emulsions, Chemosphere, 2021, 265, 129054 CrossRef CAS PubMed.
- B. M. van Vliet and W. J. Weber. Jr., Comparative performance of synthetic adsorbents and activated carbon for specific compound removal from wastewaters, J. Water Pollut. Control Fed., 1981, 53, 1585–1598 CAS.
- A. Al Bsoul, M. Hailat, A. Abdelhay, M. Tawalbeh, A. Al-Othman, I. N. Al-kharabsheh and A. A. Al-Taani, Efficient removal of phenol compounds from water environment using Ziziphus leaves adsorbent, Sci. Total Environ., 2021, 761, 143229 CrossRef CAS.
- Y. Vasseghian, E.-N. Dragoi, F. Almomani and V. T. Le, Graphene derivatives in bioplastic: A comprehensive review of properties and future perspectives, Chemosphere, 2022, 286, 131892 CrossRef CAS PubMed.
- M. Padervand, E. Lichtfouse, D. Robert and C. Wang, Removal of microplastics from the environment. A review, Environ. Chem. Lett., 2020, 18, 807–828 CrossRef CAS.
- B. C. H. Steele and A. Heinzel, Materials for fuel-cell technologies, Nature, 2001, 414, 345–352 CrossRef CAS.
- S. Li, C. Cheng and A. Thomas, Carbon-Based Microbial-Fuel-Cell Electrodes: From Conductive Supports to Active Catalysts, Adv. Mater., 2017, 29(8), 1602547 CrossRef.
- H. Su and Y. H. Hu, Recent advances in graphene-based materials for fuel cell applications, Energy Sci. Eng., 2020, 9, 958–983 CrossRef.
- X. Zhou, J. Qiao, L. Yang and J. Zhang, A Review of Graphene-Based Nanostructural Materials for Both Catalyst Supports and Metal-Free Catalysts in PEM Fuel Cell Oxygen Reduction Reactions, Adv. Energy Mater., 2014, 4, 1301523 CrossRef.
- L. Yang, J. Shui, L. Du, Y. Shao, J. Liu, L. Dai and Z. Hu, Carbon-Based Metal-Free ORR Electrocatalysts for Fuel Cells: Past, Present, and Future, Adv. Mater., 2019, 31(13), 1804799 CrossRef.
- Y. Shao, Z. Jiang, Q. Zhang and J. Guan, Progress in Nonmetal-Doped Graphene Electrocatalysts for the Oxygen Reduction Reaction, ChemSusChem, 2019, 12, 2133–2146 CrossRef CAS.
- M. Perez-Page, M. Sahoo and S. M. Holmes, Single Layer 2D Crystals for Electrochemical Applications of Ion Exchange Membranes and Hydrogen Evolution Catalysts, Adv. Mater. Interfaces, 2019, 6(7), 1801838 CrossRef CAS.
- S. Hu, M. Lozada-Hidalgo, F. C. Wang, A. Mishchenko, F. Schedin, R. R. Nair, E. W. Hill, D. W. Boukhvalov, M. I. Katsnelson, R. A. W. Dryfe, I. V. Grigorieva, H. A. Wu and A. K. Geim, Proton transport through one-atom-thick crystals, Nature, 2014, 516, 227–230 CrossRef CAS.
- R. S. Singh, A. Gautam and V. Rai, Graphene-based bipolar plates for polymer electrolyte membrane fuel cells, Front. Mater. Sci., 2019, 13, 217–241 CrossRef.
- Y. Zhu, S. Murali, W. Cai, X. Li, J. W. Suk, J. R. Potts and R. S. Ruoff, Graphene and Graphene Oxide: Synthesis, Properties, and Applications, Adv. Mater., 2010, 22, 3906–3924 CrossRef CAS PubMed.
- A. Ambrosi, C. K. Chua, A. Bonanni and M. Pumera, Electrochemistry of Graphene and Related Materials, Chem. Rev., 2014, 114, 7150–7188 CrossRef CAS.
- S. Navalon, A. Dhakshinamoorthy, M. Alvaro and H. Garcia, Carbocatalysis by Graphene-Based Materials, Chem. Rev., 2014, 114, 6179–6212 CrossRef CAS PubMed.
- A. Ambrosi, C. K. Chua, N. M. Latiff, A. H. Loo, C. H. A. Wong, A. Y. S. Eng, A. Bonanni and M. Pumera, Graphene and its electrochemistry – an update, Chem. Soc. Rev., 2016, 45, 2458–2493 RSC.
- D. Chen, H. Feng and J. Li, Graphene Oxide: Preparation, Functionalization, and Electrochemical Applications, Chem. Rev., 2012, 112, 6027–6053 CrossRef CAS PubMed.
- X.-K. Kong, C.-L. Chen and Q.-W. Chen, Doped graphene for metal-free catalysis, Chem. Soc. Rev., 2014, 43, 2841–2857 RSC.
- H. Huang, M. Yan, C. Yang, H. He, Q. Jiang, L. Yang, Z. Lu, Z. Sun, X. Xu, Y. Bando and Y. Yamauchi, Graphene Nanoarchitectonics: Recent Advances in Graphene-Based Electrocatalysts for Hydrogen Evolution Reaction, Adv. Mater., 2019, 31(48), e1903415 CrossRef PubMed.
- S. S. Siwal, S. Thakur, Q. B. Zhang and V. K. Thakur, Electrocatalysts for electrooxidation of direct alcohol fuel cell: chemistry and applications, Mater. Today Chem., 2019, 14, 100182 CrossRef CAS.
- Y. Sun, Q. Wu and G. Shi, Graphene based new energy materials, Energy Environ. Sci., 2011, 4, 1113 RSC.
- A. Saadi, M. Becherif, A. Aboubou and M. Y. Ayad, Comparison of proton exchange membrane fuel cell static models, Renewable Energy, 2013, 56, 64–71 CrossRef CAS.
- N. Mahmood, C. Zhang, J. Jiang, F. Liu and Y. Hou, Multifunctional Co3S4/Graphene Composites for Lithium Ion Batteries and Oxygen Reduction Reaction, Chem. – Eur. J., 2013, 19, 5183–5190 CrossRef CAS.
- Z.-Y. Shih, A. P. Periasamy, P.-C. Hsu and H.-T. Chang, Synthesis and catalysis of copper sulfide/carbon nanodots for oxygen reduction in direct methanol fuel cells, Appl. Catal., B, 2013, 132–133, 363–369 CrossRef CAS.
- C. Zhang, R. Hao, H. Yin, F. Liu and Y. Hou, Iron phthalocyanine and nitrogen-doped graphene composite as a novel non-precious catalyst for the oxygen reduction reaction, Nanoscale, 2012, 4, 7326 RSC.
- C. Zhang, R. Hao, H. Yin, F. Liu and Y. Hou, Iron phthalocyanine and nitrogen-doped graphene composite as a novel non-precious catalyst for the oxygen reduction reaction, Nanoscale, 2012, 4, 7326 RSC.
- G. Wu, N. H. Mack, W. Gao, S. Ma, R. Zhong, J. Han, J. K. Baldwin and P. Zelenay, Nitrogen-Doped Graphene-Rich Catalysts Derived from Heteroatom Polymers for Oxygen Reduction in Nonaqueous Lithium–O2 Battery Cathodes, ACS Nano, 2012, 6, 9764–9776 CrossRef CAS.
- J. Hou, Z. Liu and P. Zhang, A new method for fabrication of graphene/polyaniline nanocomplex modified microbial fuel cell anodes, J. Power Sources, 2013, 224, 139–144 CrossRef CAS.
- C. Zhang, R. Hao, H. Liao and Y. Hou, Synthesis of amino-functionalized graphene as metal-free catalyst and exploration of the roles of various nitrogen states in oxygen reduction reaction, Nano Energy, 2013, 2, 88–97 CrossRef CAS.
- L. Zhu, D. Susac, M. Teo, K. Wong, P. Wong, R. Parsons, D. Bizzotto, K. Mitchell and S. Campbell, Investigation of CoS2-based thin films as model catalysts for the oxygen reduction reaction, J. Catal., 2008, 258, 235–242 CrossRef CAS.
- Z.-S. Wu, S. Yang, Y. Sun, K. Parvez, X. Feng and K. Müllen, 3D Nitrogen-Doped Graphene Aerogel-Supported Fe3O4 Nanoparticles as Efficient Electrocatalysts for the Oxygen Reduction Reaction, J. Am. Chem. Soc., 2012, 134, 9082–9085 CrossRef CAS.
- L. Ren, K. S. Hui and K. N. Hui, Self-assembled free-standing three-dimensional nickel nanoparticle/graphene aerogel for direct ethanol fuel cells, J. Mater. Chem. A, 2013, 1, 5689 RSC.
- W. Chen, S. Li, C. Chen and L. Yan, Self-Assembly and Embedding of Nanoparticles by In Situ Reduced Graphene for Preparation of a 3D Graphene/Nanoparticle Aerogel, Adv. Mater., 2011, 23, 5679–5683 CrossRef CAS.
- C. Zhang, R. Hao, H. Liao and Y. Hou, Synthesis of amino-functionalized graphene as metal-free catalyst and exploration of the roles of various nitrogen states in oxygen reduction reaction, Nano Energy, 2013, 2, 88–97 CrossRef CAS.
- S. Wang, D. Yu, L. Dai, D. W. Chang and J.-B. Baek, Polyelectrolyte-Functionalized Graphene as Metal-Free Electrocatalysts for Oxygen Reduction, ACS Nano, 2011, 5, 6202–6209 CrossRef CAS.
- Z. Liu, H. Nie, Z. Yang, J. Zhang, Z. Jin, Y. Lu, Z. Xiao and S. Huang, Sulfur–nitrogen co-doped three-dimensional carbon foams with hierarchical pore structures as efficient metal-free electrocatalysts for oxygen reduction reactions, Nanoscale, 2013, 5, 3283 RSC.
- H. Li, W. Kang, L. Wang, Q. Yue, S. Xu, H. Wang and J. Liu, Synthesis of three-dimensional flowerlike nitrogen-doped carbons by a copyrolysis route and the effect of nitrogen species on the electrocatalytic activity in oxygen reduction reaction, Carbon, 2013, 54, 249–257 CrossRef CAS.
- Z.-W. Liu, F. Peng, H.-J. Wang, H. Yu, W.-X. Zheng and J. Yang, Phosphorus-Doped Graphite Layers with High Electrocatalytic Activity for the O2 Reduction in an Alkaline Medium, Angew. Chem., Int. Ed., 2011, 50, 3257–3261 CrossRef.
- L. Yang, S. Jiang, Y. Zhao, L. Zhu, S. Chen, X. Wang, Q. Wu, J. Ma, Y. Ma and Z. Hu, Boron-Doped Carbon Nanotubes as Metal-Free Electrocatalysts for the Oxygen Reduction Reaction, Angew. Chem., Int. Ed., 2011, 50, 7132–7135 CrossRef CAS PubMed.
- J. Xu, G. Dong, C. Jin, M. Huang and L. Guan, Sulfur and Nitrogen Co-Doped, Few-Layered Graphene Oxide as a Highly Efficient Electrocatalyst for the Oxygen-Reduction Reaction, ChemSusChem, 2013, 6, 493–499 CrossRef CAS PubMed.
- N. Mahmood, C. Zhang, J. Jiang, F. Liu and Y. Hou, Multifunctional Co3S4/Graphene Composites for Lithium Ion Batteries and Oxygen Reduction Reaction, Chem. – Eur. J., 2013, 19, 5183–5190 CrossRef CAS PubMed.
- C. Zhang, N. Mahmood, H. Yin, F. Liu and Y. Hou, Synthesis of Phosphorus-Doped Graphene and its Multifunctional Applications for Oxygen Reduction Reaction and Lithium Ion Batteries, Adv. Mater., 2013, 25, 4932–4937 CrossRef CAS.
- N. Mahmood, C. Zhang, H. Yin and Y. Hou, Graphene-based nanocomposites for energy storage and conversion in lithium batteries, supercapacitors and fuel cells, J. Mater. Chem. A, 2014, 2, 15–32 RSC.
- X. Gong, G. Liu, Y. Li, D. Y. W. Yu and W. Y. Teoh, Functionalized-Graphene Composites: Fabrication and Applications in Sustainable Energy and Environment, Chem. Mater., 2016, 28, 8082–8118 CrossRef CAS.
- M. Liu, R. Zhang and W. Chen, Graphene-Supported Nanoelectrocatalysts for Fuel Cells: Synthesis, Properties, and Applications, Chem. Rev., 2014, 114, 5117–5160 CrossRef CAS PubMed.
- R. Kou, Y. Shao, D. Wang, M. H. Engelhard, J. H. Kwak, J. Wang, V. V. Viswanathan, C. Wang, Y. Lin, Y. Wang, I. A. Aksay and J. Liu, Enhanced activity and stability of Pt catalysts on functionalized graphene sheets for electrocatalytic oxygen reduction, Electrochem. Commun., 2009, 11, 954–957 CrossRef CAS.
- C. Zhu, S. Guo, Y. Zhai and S. Dong, Layer-by-Layer Self-Assembly for Constructing a Graphene/Platinum Nanoparticle Three-Dimensional Hybrid Nanostructure Using Ionic Liquid as a Linker, Langmuir, 2010, 26, 7614–7618 CrossRef CAS PubMed.
- C. Cui, L. Gan, M. Heggen, S. Rudi and P. Strasser, Compositional segregation in shaped Pt alloy nanoparticles and their structural behaviour during electrocatalysis, Nat. Mater., 2013, 12, 765–771 CrossRef CAS PubMed.
- J. Wu, A. Gross and H. Yang, Shape and Composition-Controlled Platinum Alloy Nanocrystals Using Carbon Monoxide as Reducing Agent, Nano Lett., 2011, 11, 798–802 CrossRef CAS.
- Y. Kang and C. B. Murray, Synthesis and Electrocatalytic Properties of Cubic Mn−Pt Nanocrystals (Nanocubes), J. Am. Chem. Soc., 2010, 132, 7568–7569 CrossRef CAS PubMed.
- H. Yang, J. Zhang, K. Sun, S. Zou and J. Fang, Enhancing by Weakening: Electrooxidation of Methanol on Pt3Co and Pt Nanocubes, Angew. Chem., 2010, 122, 7000–7003 CrossRef.
- S. Guo and S. Sun, FePt Nanoparticles Assembled on Graphene as Enhanced Catalyst for Oxygen Reduction Reaction, J. Am. Chem. Soc., 2012, 134, 2492–2495 CrossRef CAS PubMed.
- Y. Si and E. T. Samulski, Exfoliated Graphene Separated by Platinum Nanoparticles, Chem. Mater., 2008, 20, 6792–6797 CrossRef CAS.
- Y. Li, Y. Li, E. Zhu, T. McLouth, C.-Y. Chiu, X. Huang and Y. Huang, Stabilization of High-Performance Oxygen Reduction Reaction Pt Electrocatalyst Supported on Reduced Graphene Oxide/Carbon Black Composite, J. Am. Chem. Soc., 2012, 134, 12326–12329 CrossRef CAS PubMed.
- F. Yang, D. Deng, X. Pan, Q. Fu and X. Bao, Understanding nano effects in catalysis, Natl. Sci. Rev., 2015, 2, 183–201 CrossRef CAS.
- Z. Luo, A. W. Castleman and S. N. Khanna, Reactivity of Metal Clusters, Chem. Rev., 2016, 116, 14456–14492 CrossRef CAS.
- C. Zhang, J. Sha, H. Fei, M. Liu, S. Yazdi, J. Zhang, Q. Zhong, X. Zou, N. Zhao, H. Yu, Z. Jiang, E. Ringe, B. I. Yakobson, J. Dong, D. Chen and J. M. Tour, Single-Atomic Ruthenium Catalytic Sites on Nitrogen-Doped Graphene for Oxygen Reduction Reaction in Acidic Medium, ACS Nano, 2017, 11, 6930–6941 CrossRef CAS.
- C. H. Choi, M. Kim, H. C. Kwon, S. J. Cho, S. Yun, H.-T. Kim, K. J. J. Mayrhofer, H. Kim and M. Choi, Tuning selectivity of electrochemical reactions by atomically dispersed platinum catalyst, Nat. Commun., 2016, 7, 10922 CrossRef CAS.
- M. H. Seo, S. M. Choi, H. J. Kim and W. B. Kim, The graphene-supported Pd and Pt catalysts for highly active oxygen reduction reaction in an alkaline condition, Electrochem. Commun., 2011, 13, 182–185 CrossRef CAS.
- M. Liu, Y. Lu and W. Chen, Electrocatalysts: PdAg Nanorings Supported on Graphene Nanosheets: Highly Methanol-Tolerant Cathode Electrocatalyst for Alkaline Fuel Cells (Adv. Funct. Mater. 10/2013), Adv. Funct. Mater., 2013, 23, 1348 CrossRef CAS.
- M. H. Seo, S. M. Choi, J. K. Seo, S. H. Noh, W. B. Kim and B. Han, The graphene-supported palladium and palladium–yttrium nanoparticles for the oxygen reduction and ethanol oxidation reactions: Experimental measurement and computational validation, Appl. Catal., B, 2013, 129, 163–171 CrossRef CAS.
- H. Yin, H. Tang, D. Wang, Y. Gao and Z. Tang, Facile Synthesis of Surfactant-Free Au Cluster/Graphene Hybrids for High-Performance Oxygen Reduction Reaction, ACS Nano, 2012, 6, 8288–8297 CrossRef CAS.
- S. Jin, M. Chen, H. Dong, B. He, H. Lu, L. Su, W. Dai, Q. Zhang and X. Zhang, Stable silver nanoclusters electrochemically deposited on nitrogen-doped graphene as efficient electrocatalyst for oxygen reduction reaction, J. Power Sources, 2015, 274, 1173–1179 CrossRef CAS.
- J. Liu, Q. Ma, Z. Huang, G. Liu and H. Zhang, Recent Progress in Graphene-Based Noble-Metal Nanocomposites for Electrocatalytic Applications, Adv. Mater., 2019, 31(9), 1800696 CrossRef PubMed.
- X. Lu, S. Xie, H. Yang, Y. Tong and H. Ji, Photoelectrochemical hydrogen production from biomass derivatives and water, Chem. Soc. Rev., 2014, 43, 7581–7593 RSC.
- S. Oh, J. Kim, M. Kim, D. Nam, J. Park, E. Cho and H. Kwon, Synergetic effects of edge formation and sulfur doping on the catalytic activity of a graphene-based catalyst for the oxygen reduction reaction, J. Mater. Chem. A, 2016, 4, 14400–14407 RSC.
- J. Yang, D. Wang, H. Han and C. Li, Roles of Cocatalysts in Photocatalysis and Photoelectrocatalysis, Acc. Chem. Res., 2013, 46, 1900–1909 CrossRef CAS PubMed.
- Z. Fan, M. Bosman, X. Huang, D. Huang, Y. Yu, K. P. Ong, Y. A. Akimov, L. Wu, B. Li, J. Wu, Y. Huang, Q. Liu, C. Eng Png, C. Lip Gan, P. Yang and H. Zhang, Stabilization of 4H hexagonal phase in gold nanoribbons, Nat. Commun., 2015, 6, 7684 CrossRef CAS.
- R. Mazzaro, A. Boni, G. Valenti, M. Marcaccio, F. Paolucci, L. Ortolani, V. Morandi, P. Ceroni and G. Bergamini, Uniform Functionalization of High-Quality Graphene with Platinum Nanoparticles for Electrocatalytic Water Reduction, ChemistryOpen, 2015, 4, 268–273 CrossRef CAS.
- R. Ojani, R. Valiollahi and J.-B. Raoof, Comparison between graphene supported Pt hollow nanospheres and graphene supported Pt solid nanoparticles for hydrogen evolution reaction, Energy, 2014, 74, 871–876 CrossRef CAS.
- M. G. Walter, E. L. Warren, J. R. McKone, S. W. Boettcher, Q. Mi, E. A. Santori and N. S. Lewis, Solar Water Splitting Cells, Chem. Rev., 2010, 110, 6446–6473 CrossRef CAS.
- S. Bai, C. Wang, M. Deng, M. Gong, Y. Bai, J. Jiang and Y. Xiong, Back Cover: Surface Polarization Matters: Enhancing the Hydrogen-Evolution Reaction by Shrinking Pt Shells in Pt-Pd-Graphene Stack Structures (Angew. Chem. Int. Ed. 45/2014), Angew. Chem., Int. Ed., 2014, 53, 12264 CrossRef.
- J. Mahmood, F. Li, S.-M. Jung, M. S. Okyay, I. Ahmad, S.-J. Kim, N. Park, H. Y. Jeong and J.-B. Baek, An efficient and pH-universal ruthenium-based catalyst for the hydrogen evolution reaction, Nat. Nanotechnol., 2017, 12, 441–446 CrossRef CAS PubMed.
- G. Mamba, G. Gangashe, L. Moss, S. Hariganesh, S. Thakur, S. Vadivel, A. K. Mishra, G. D. Vilakati, V. Muthuraj and T. T. I. Nkambule, State of the art on the photocatalytic applications of graphene based nanostructures: From elimination of hazardous pollutants to disinfection and fuel generation, J. Environ. Chem. Eng., 2020, 8, 103505 CrossRef CAS.
- J. Li, P. Zhou, F. Li, R. Ren, Y. Liu, J. Niu, J. Ma, X. Zhang, M. Tian, J. Jin and J. Ma, Ni@Pd/PEI–rGO stack structures with controllable Pd shell thickness as advanced electrodes for efficient hydrogen evolution, J. Mater. Chem. A, 2015, 3, 11261–11268 RSC.
- Z. Cao, Q. Chen, J. Zhang, H. Li, Y. Jiang, S. Shen, G. Fu, B. Lu, Z. Xie and L. Zheng, Platinum-nickel alloy excavated nano-multipods with hexagonal close-packed structure and superior activity towards hydrogen evolution reaction, Nat. Commun., 2017, 8, 15131 CrossRef PubMed.
- P. Wang, X. Zhang, J. Zhang, S. Wan, S. Guo, G. Lu, J. Yao and X. Huang, Precise tuning in platinum-nickel/nickel sulfide interface nanowires for synergistic hydrogen evolution catalysis, Nat. Commun., 2016, 8, 14580 CrossRef.
- T. Yang, H. Zhu, M. Wan, L. Dong, M. Zhang and M. Du, Highly efficient and durable PtCo alloy nanoparticles encapsulated in carbon nanofibers for electrochemical hydrogen generation, Chem. Commun., 2016, 52, 990–993 RSC.
- S. J. Yoo, H. Park, T. Jeon, I. Park, Y. Cho and Y. Sung, Promotional Effect of Palladium on the Hydrogen Oxidation Reaction at a PtPd Alloy Electrode, Angew. Chem., Int. Ed., 2008, 47, 9307–9310 CrossRef CAS.
- T. Chao, X. Luo, W. Chen, B. Jiang, J. Ge, Y. Lin, G. Wu, X. Wang, Y. Hu, Z. Zhuang, Y. Wu, X. Hong and Y. Li, Atomically Dispersed Copper–Platinum Dual Sites Alloyed with Palladium Nanorings Catalyze the Hydrogen Evolution Reaction, Angew. Chem., Int.
Ed., 2017, 56, 16047–16051 CrossRef CAS.
- X. Zhong, L. Wang, Z. Zhuang, X. Chen, J. Zheng, Y. Zhou, G. Zhuang, X. Li and J. Wang, Double Nanoporous Structure with Nanoporous PtFe Embedded in Graphene Nanopores: Highly Efficient Bifunctional Electrocatalysts for Hydrogen Evolution and Oxygen Reduction, Adv. Mater. Interfaces, 2017, 4(5), 1601029 CrossRef.
- L. Jiao, F. Li, X. Li, R. Ren, J. Li, X. Zhou, J. Jin and R. Li, Ultrathin PdTe nanowires anchoring reduced graphene oxide cathodes for efficient hydrogen evolution reaction, Nanoscale, 2015, 7, 18441–18445 RSC.
- Y. Jiang, Y. Yan, Y. Han, H. Zhang and D. Yang, Core–shell and alloy integrating PdAu bimetallic nanoplates on reduced graphene oxide for efficient and stable hydrogen evolution catalysts, RSC Adv., 2017, 7, 43373–43379 RSC.
- H. Zhang, G. Liu, L. Shi and J. Ye, Single-Atom Catalysts: Emerging Multifunctional Materials in Heterogeneous Catalysis, Adv. Energy Mater., 2018, 8(1), 1701343 CrossRef.
- H. Chen, M. B. Müller, K. J. Gilmore, G. G. Wallace and D. Li, Mechanically Strong, Electrically Conductive, and Biocompatible Graphene Paper, Adv. Mater., 2008, 20, 3557–3561 CrossRef CAS.
- C. Shan, H. Yang, J. Song, D. Han, A. Ivaska and L. Niu, Direct Electrochemistry of Glucose Oxidase and Biosensing for Glucose Based on Graphene, Anal. Chem., 2009, 81, 2378–2382 CrossRef CAS PubMed.
- Z. Wang, X. Zhou, J. Zhang, F. Boey and H. Zhang, Direct Electrochemical Reduction of Single-Layer Graphene Oxide and Subsequent Functionalization with Glucose Oxidase, J. Phys. Chem. C, 2009, 113, 14071–14075 CrossRef CAS.
- R. L. McCreery, Advanced Carbon Electrode Materials for Molecular Electrochemistry, Chem. Rev., 2008, 108, 2646–2687 CrossRef CAS PubMed.
- J. Wang, Contents: Electroanalysis 17/2005, Electroanalysis, 2005, 17, 1499–1500 CrossRef.
- X. Kang, J. Wang, H. Wu, I. A. Aksay, J. Liu and Y. Lin, Glucose Oxidase–graphene–chitosan modified electrode for direct electrochemistry and glucose sensing, Biosens. Bioelectron., 2009, 25, 901–905 CrossRef CAS PubMed.
- H. Wu, J. Wang, X. Kang, C. Wang, D. Wang, J. Liu, I. A. Aksay and Y. Lin, Glucose biosensor based on immobilization of glucose oxidase in platinum nanoparticles/graphene/chitosan nanocomposite film, Talanta, 2009, 80, 403–406 CrossRef CAS PubMed.
- J. Lu, L. T. Drzal, R. M. Worden and I. Lee, Simple Fabrication of a Highly Sensitive Glucose Biosensor Using Enzymes Immobilized in Exfoliated Graphite Nanoplatelets Nafion Membrane, Chem. Mater., 2007, 19, 6240–6246 CrossRef CAS.
- G. Liu and Y. Lin, Amperometric glucose biosensor based on self-assembling glucose oxidase on carbon nanotubes, Electrochem. Commun., 2006, 8, 251–256 CrossRef CAS.
- L. Wu, X. Zhang and H. Ju, Amperometric glucose sensor based on catalytic reduction of dissolved oxygen at soluble carbon nanofiber, Biosens. Bioelectron., 2007, 23, 479–484 CrossRef CAS.
- M. D. Rubianes and G. A. Rivas, Carbon nanotubes paste electrode, Electrochem. Commun., 2003, 5, 689–694 CrossRef CAS.
- Y. Lin, F. Lu, Y. Tu and Z. Ren, Glucose Biosensors Based on Carbon Nanotube Nanoelectrode Ensembles, Nano Lett., 2003, 4, 191–195 CrossRef.
- M. Zhou, L. Shang, B. Li, L. Huang and S. Dong, Highly ordered mesoporous carbons as electrode material for the construction of electrochemical dehydrogenase- and oxidase-based biosensors, Biosens. Bioelectron., 2008, 24, 442–447 CrossRef CAS PubMed.
- C. Shan, H. Yang, D. Han, Q. Zhang, A. Ivaska and L. Niu, Graphene/AuNPs/chitosan nanocomposites film for glucose biosensing, Biosens. Bioelectron., 2010, 25, 1070–1074 CrossRef CAS PubMed.
- A. Sassolas, B. D. Leca-Bouvier and L. J. Blum, DNA Biosensors and Microarrays, Chem. Rev., 2007, 108, 109–139 CrossRef.
- T. G. Drummond, M. G. Hill and J. K. Barton, Electrochemical DNA sensors, Nat. Biotechnol., 2003, 21, 1192–1199 CrossRef CAS.
- J. Li, S. Guo, Y. Zhai and E. Wang, High-sensitivity determination of lead and cadmium based on the Nafion-graphene composite film, Anal. Chim. Acta, 2009, 649, 196–201 CrossRef CAS PubMed.
- J. Li, S. Guo, Y. Zhai and E. Wang, Nafion–graphene nanocomposite film as enhanced sensing platform for ultrasensitive determination of cadmium, Electrochem. Commun., 2009, 11, 1085–1088 CrossRef CAS.
- G. Kefala, A. Economou and A. Voulgaropoulos, A study of Nafion-coated bismuth-film electrodes for the determination of trace metals by anodic stripping voltammetry, Analyst, 2004, 129, 1082 RSC.
- L. Zhu, C. Tian, R. Yang and J. Zhai, Anodic Stripping Voltammetric Determination of Lead in Tap Water at an Ordered Mesoporous Carbon/Nafion Composite film Electrode, Electroanalysis, 2008, 20, 527–533 CrossRef CAS.
- H. Xu, L. Zeng, S. Xing, Y. Xian, G. Shi and L. Jin, Ultrasensitive Voltammetric Detection of Trace Lead(II) and Cadmium(II) Using MWCNTs-Nafion/Bismuth Composite Electrodes, Electroanalysis, 2008, 20, 2655–2662 CrossRef CAS.
- H. Chen, M. B. Müller, K. J. Gilmore, G. G. Wallace and D. Li, Mechanically Strong, Electrically Conductive, and Biocompatible Graphene Paper, Adv. Mater., 2008, 20, 3557–3561 CrossRef CAS.
- Y. Shao, J. Wang, H. Wu, J. Liu, I. A. Aksay and Y. Lin, Graphene Based Electrochemical Sensors and Biosensors: A Review, Electroanalysis, 2010, 22, 1027–1036 CrossRef CAS.
- H. A. Rahman, M. Rafi, B. R. Putra and W. T. Wahyuni, Electrochemical Sensors Based on a Composite of Electrochemically Reduced Graphene Oxide and PEDOT:PSS for Hydrazine Detection, ACS Omega, 2023, 8, 3258–3269 CrossRef CAS PubMed.
- C. Rogers, W. S. Perkins, G. Veber, T. E. Williams, R. R. Cloke and F. R. Fischer, Synergistic Enhancement of Electrocatalytic CO2 Reduction with Gold Nanoparticles Embedded in Functional Graphene Nanoribbon Composite Electrodes, J. Am. Chem. Soc., 2017, 139, 4052–4061 CrossRef CAS PubMed.
- G. He, H. Tang, H. Wang and Z. Bian, Highly Selective and Active Pd-In/three-dimensional Graphene with Special Structure for Electroreduction CO2 to Formate, Electroanalysis, 2017, 30, 84–93 CrossRef.
- V. Tripkovic, M. Vanin, M. Karamad, M. E. Björketun, K. W. Jacobsen, K. S. Thygesen and J. Rossmeisl, Electrochemical CO2 and CO Reduction on Metal-Functionalized Porphyrin-like Graphene, J. Phys. Chem. C, 2013, 117, 9187–9195 CrossRef CAS.
- H. He and Y. Jagvaral, Electrochemical reduction of CO2 on graphene supported transition metals – towards single atom catalysts, Phys. Chem. Chem. Phys., 2017, 19, 11436–11446 RSC.
- Y. Shao, J. Wang, H. Wu, J. Liu, I. A. Aksay and Y. Lin, Graphene Based Electrochemical Sensors and Biosensors: A Review, Electroanalysis, 2010, 22, 1027–1036 CrossRef CAS.
- G. Chen, S. Ren, L. Zhang, H. Cheng, Y. Luo, K. Zhu, L. Ding and H. Wang, Nitrogen Reduction Reactions: Advances in Electrocatalytic N2 Reduction—Strategies to Tackle the Selectivity Challenge (Small Methods 6/2019), Small Methods, 2019, 3, 1970016 CrossRef.
- Y. Tanabe and Y. Nishibayashi, Developing more sustainable processes for ammonia synthesis, Coord. Chem. Rev., 2013, 257, 2551–2564 CrossRef CAS.
- L. Xia, J. Yang, H. Wang, R. Zhao, H. Chen, W. Fang, A. M. Asiri, F. Xie, G. Cui and X. Sun, Sulfur-doped graphene for efficient electrocatalytic N2-to-NH3 fixation, Chem. Commun., 2019, 55, 3371–3374 RSC.
- Y. Tian, D. Xu, K. Chu, Z. Wei and W. Liu, Metal-free N, S co-doped graphene for efficient and durable nitrogen reduction reaction, J. Mater. Sci., 2019, 54, 9088–9097 CrossRef CAS.
- Y. Yang, J. Liu, Z. Wei, S. Wang and J. Ma, Transition Metal-dinitrogen Complex Embedded Graphene for Nitrogen Reduction Reaction, ChemCatChem, 2019, 11, 2821–2827 CrossRef CAS.
- K. Wang, H. Wu, Y. Meng and Z. Wei, Conducting Polymer Nanowire Arrays for High Performance Supercapacitors, Small, 2013, 10, 14–31 CrossRef PubMed.
- P. Song, L. Kang, H. Wang, R. Guo and R. Wang, Nitrogen (N), Phosphorus (P)-Codoped Porous Carbon as a Metal-Free Electrocatalyst for N2 Reduction under Ambient Conditions, ACS Appl. Mater. Interfaces, 2019, 11, 12408–12414 CrossRef CAS PubMed.
- T. Wang, L. Xia, J.-J. Yang, H. Wang, W.-H. Fang, H. Chen, D. Tang, A. M. Asiri, Y. Luo, G. Cui and X. Sun, Electrocatalytic N2-to-NH3 conversion using oxygen-doped graphene: experimental and theoretical studies, Chem. Commun., 2019, 55, 7502–7505 RSC.
- X. Yu, P. Han, Z. Wei, L. Huang, Z. Gu, S. Peng, J. Ma and G. Zheng, Boron-Doped Graphene for Electrocatalytic N2 Reduction, Joule, 2018, 2, 1610–1622 CrossRef CAS.
- J. Zhao, J. Yang, L. Ji, H. Wang, H. Chen, Z. Niu, Q. Liu, T. Li, G. Cui and X. Sun, Defect-rich fluorographene nanosheets for artificial N2 fixation under ambient conditions, Chem. Commun., 2019, 55, 4266–4269 RSC.
- M. Zhang, C. Choi, R. Huo, G. H. Gu, S. Hong, C. Yan, S. Xu, A. W. Robertson, J. Qiu, Y. Jung and Z. Sun, Reduced graphene oxides with engineered defects enable efficient electrochemical reduction of dinitrogen to ammonia in wide pH range, Nano Energy, 2020, 68, 104323 CrossRef CAS.
- Y. Jia, L. Zhang, A. Du, G. Gao, J. Chen, X. Yan, C. L. Brown and X. Yao, Defect Graphene as a Trifunctional Catalyst for Electrochemical Reactions, Adv. Mater., 2016, 28, 9532–9538 CrossRef CAS.
- M. Majumder, H. Saini, I. Dědek, A. Schneemann, N. R. Chodankar, V. Ramarao, M. S. Santosh, A. K. Nanjundan, Š. Kment, D. Dubal, M. Otyepka, R. Zbořil and K. Jayaramulu, Rational Design of Graphene Derivatives for Electrochemical Reduction of Nitrogen to Ammonia, ACS Nano, 2021, 15, 17275–17298 CrossRef CAS PubMed.
- F. Wang, H. Wang and J. Mao, Broken holey graphene oxide for electrocatalytic N2-to-NH3 fixation at ambient condition, Colloids Surf., A, 2020, 605, 125345 CrossRef CAS.
- Y. Song, T. Wang, J. Sun, Z. Wang, Y. Luo, L. Zhang, H. Ye and X. Sun, Enhanced Electrochemical N2 Reduction to NH3 on Reduced Graphene Oxide by Tannic Acid Modification, ACS Sustainable Chem. Eng., 2019, 7, 14368–14372 CrossRef CAS.
- M. Zhang, C. Choi, R. Huo, G. H. Gu, S. Hong, C. Yan, S. Xu, A. W. Robertson, J. Qiu, Y. Jung and Z. Sun, Reduced graphene oxides with engineered defects enable efficient electrochemical reduction of dinitrogen to ammonia in wide pH range, Nano Energy, 2020, 68, 104323 CrossRef CAS.
- Y. Du, C. Jiang, W. Xia, L. Song, P. Li, B. Gao, C. Wu, L. Sheng, J. Ye, T. Wang and J. He, Electrocatalytic reduction of N2 and nitrogen-incorporation process on dopant-free defect graphene, J. Mater. Chem. A, 2020, 8, 55–61 RSC.
- C. Li, Y. Fu, Z. Wu, J. Xia and X. Wang, Sandwich-like reduced graphene oxide/yolk–shell-structured Fe@Fe3O4/carbonized paper as an efficient freestanding electrode for electrochemical synthesis of ammonia directly from H2O and nitrogen, Nanoscale, 2019, 11, 12997–13006 RSC.
- W. Fang, J. Zhao, T. Wu, Y. Huang, L. Yang, C. Liu, Q. Zhang, K. Huang and Q. Yan, Hydrophilic engineering of VOx-based nanosheets for ambient electrochemical ammonia synthesis at neutral pH, J. Mater. Chem. A, 2020, 8, 5913–5918 RSC.
- Y. Liu, Y. Li, D. Huang, H. Zhang and K. Chu, ZnO Quantum Dots Coupled with Graphene toward Electrocatalytic N2 Reduction: Experimental and DFT Investigations, Chem. – Eur. J., 2019, 25, 11933–11939 CrossRef CAS PubMed.
- M. Majumder, H. Saini, I. Dědek, A. Schneemann, N. R. Chodankar, V. Ramarao, M. S. Santosh, A. K. Nanjundan, Š. Kment, D. Dubal, M. Otyepka, R. Zbořil and K. Jayaramulu, Rational Design of Graphene Derivatives for Electrochemical Reduction of Nitrogen to Ammonia, ACS Nano, 2021, 15, 17275–17298 CrossRef CAS.
- C. Xiang, M. Li, M. Zhi, A. Manivannan and N. Wu, A reduced graphene oxide/Co3O4 composite for supercapacitor electrode, J. Power Sources, 2013, 226, 65–70 CrossRef CAS.
- Q. Qiu, M. Zhu, Z. Li, K. Qiu, X. Liu, J. Yu and B. Ding, Highly flexible, breathable, tailorable and washable power generation fabrics for wearable electronics, Nano Energy, 2019, 58, 750–758 CrossRef CAS.
- H. Kao, C.-H. Chuang, L.-C. Chang, C.-L. Cho and H.-C. Chiu, Inkjet-printed silver films on textiles for wearable electronics applications, Surf. Coat. Technol., 2019, 362, 328–332 CrossRef CAS.
- C. Lim, Y. Shin, J. Jung, J. H. Kim, S. Lee and D.-H. Kim, Stretchable conductive nanocomposite based on alginate hydrogel and silver nanowires for wearable electronics, APL Mater., 2007, 7(3), 031502 CrossRef.
- A. Soam and R. Kumar, Preparation Of Mno2 Nanoparticles By A Solution Based Approach For Electrochemical Capacitor, Surf. Rev. Lett., 2020, 27, 1950199 CrossRef CAS.
- M. Zhi, C. Xiang, J. Li, M. Li and N. Wu, Nanostructured carbon–metal oxide composite electrodes for supercapacitors: a review, Nanoscale, 2013, 5, 72–88 RSC.
- Z. Ren, Y. Li and J. Yu, A Flexible Supercapacitor with High True Performance, iScience, 2018, 9, 138–148 CrossRef CAS PubMed.
-
B. E. Conway, Electrochemical Supercapacitors: Scientific Fundamentals and Technological Applications, Springer, NY, New York, 1st edn, 2013 Search PubMed.
- Y. Zhang, H. Feng, X. Wu, L. Wang, A. Zhang, T. Xia, H. Dong, X. Li and L. Zhang, Progress of electrochemical capacitor electrode materials: A review, Int. J. Hydrogen Energy, 2009, 34, 4889–4899 CrossRef CAS.
- M. Mazloum-Ardakani, H. Mohammadian-Sarcheshmeh, H. Naderi, F. Farbod and F. Sabaghian, Fabrication of a high-performance hybrid supercapacitor using a modified graphene aerogel/cerium oxide nanoparticle composite, J. Energy Storage, 2019, 26, 100998 CrossRef.
- T.-F. Yi, Y. Li, Y.-M. Li, S. Luo and Y.-G. Liu, ZnS nanoparticles as the electrode materials for high-performance supercapacitors, Solid State Ionics, 2019, 343, 115074 CrossRef CAS.
- R. Lakra, R. Kumar, P. K. Sahoo, D. Thatoi and A. Soam, A mini-review: Graphene based composites for supercapacitor application, Inorg. Chem. Commun., 2021, 133, 108929 CrossRef CAS.
- Z. Wu, Y. Zhu and X. Ji, NiCo2O4-based materials for electrochemical supercapacitors, J. Mater. Chem. A, 2014, 2, 14759–14772 RSC.
- S. W. Bokhari, A. H. Siddique, P. C. Sherrell, X. Yue, K. M. Karumbaiah, S. Wei, A. V. Ellis and W. Gao, Advances in graphene-based supercapacitor electrodes, Energy Rep., 2020, 6, 2768–2784 CrossRef.
- F. Li, X. Jiang, J. Zhao and S. Zhang, Graphene oxide: A promising nanomaterial for energy and environmental applications, Nano Energy, 2015, 16, 488–515 CrossRef CAS.
- L. L. Zhang and X. S. Zhao, Carbon-based materials as supercapacitor electrodes, Chem. Soc. Rev., 2009, 38, 2520 RSC.
- M. F. El-Kady, Y. Shao and R. B. Kaner, Graphene for batteries, supercapacitors and beyond, Nat. Rev. Mater., 2016, 1, 16033 CrossRef CAS.
- M. Fu, Z. Zhu, Z. Zhang, Q. Zhuang, W. Chen and Q. Liu, Microwave deposition synthesis of Ni(OH)2/sorghum stalk biomass carbon electrode materials for supercapacitors, J. Alloys Compd., 2020, 846, 156376 CrossRef CAS.
- K. S. Novoselov, A. K. Geim, S. V. Morozov, D. Jiang, Y. Zhang, S. V. Dubonos, I. V. Grigorieva and A. A. Firsov, Electric Field Effect in Atomically Thin Carbon Films, Science, 2004, 306, 666–669 CrossRef CAS.
- B. Yao, S. Chandrasekaran, H. Zhang, A. Ma, J. Kang, L. Zhang, X. Lu, F. Qian, C. Zhu, E. B. Duoss, C. M. Spadaccini, M. A. Worsley and Y. Li, 3D-Printed Structure Boosts the Kinetics and Intrinsic Capacitance of Pseudocapacitive Graphene Aerogels, Adv. Mater., 2020, 32(8), 1906652 CrossRef CAS.
- Z. Lei, J. Zhang, L. L. Zhang, N. A. Kumar and X. S. Zhao, Functionalization of chemically derived graphene for improving its electrocapacitive energy storage properties, Energy Environ. Sci., 2016, 9, 1891–1930 RSC.
- M. Fu, Z. Zhu, W. Chen, H. Yu and Q. Liu, Microwave-assisted synthesis of MoS2/graphene composites for supercapacitors, J. Mater. Sci., 2020, 55, 16385–16393 CrossRef CAS.
- M. Fu, W. Chen, X. Zhu and Q. Liu, One-step preparation of one dimensional nickel ferrites/graphene composites for supercapacitor electrode with excellent cycling stability, J. Power Sources, 2018, 396, 41–48 CrossRef CAS.
-
A. Soam, Ferrites – Synthesis and Applications: Application of Ferrites as Electrodes for Supercapacitor, IntechOpen, 2021, p. 144 Search PubMed.
- R. Kumar, A. Soam and V. Sahajwalla, Sucrose-derived carbon-coated nickel oxide (SDCC-NiO) as an electrode material for supercapacitor applications, Mater. Adv., 2020, 1, 609–616 RSC.
- R. Kumar, A. Soam, R. Hossain, I. Mansuri and V. Sahajwalla, Carbon coated iron oxide (CC-IO) as high performance electrode material for supercapacitor applications, J. Energy Storage, 2020, 32, 101737 CrossRef.
- R. Kumar, B. K. Singh, A. Soam, S. Parida and P. Bhargava, In-situ carbon coated manganese oxide nanorods (ISCC-MnO2NRs) as an electrode material for supercapacitors, Diamond Relat. Mater., 2019, 94, 110–117 CrossRef CAS.
- Y. Gao, Graphene and Polymer Composites for Supercapacitor Applications: a Review, Nanoscale Res. Lett., 2017, 12, 387 CrossRef.
- H. Gómez, M. K. Ram, F. Alvi, P. Villalba, E. L. Stefanakos and A. Kumar, Graphene-conducting polymer nanocomposite as novel electrode for supercapacitors, J. Power Sources, 2011, 196, 4102–4108 CrossRef.
- H. Zhang, X. Tang, D. Zhao, N. Zheng, L. Huang, T. Sun, C. Gu and Y. Ma, Suppressing charge trapping effect in ambipolar conducting polymer with vertically standing graphene as the composite electrode for high performance supercapacitor, Energy Storage Mater., 2020, 29, 281–286 CrossRef.
- N. Macherla, K. Singh, M. S. Santosh, K. Kumari and R. G. R. Lekkala, Heat assisted facile synthesis of nanostructured polyaniline/reduced crumbled graphene oxide as a high-performance flexible electrode material for supercapacitors, Colloids Surf., A, 2021, 612, 125982 CrossRef CAS.
- A. Moyseowicz and G. Gryglewicz, Hydrothermal-assisted synthesis of a porous polyaniline/reduced graphene oxide composite as a high-performance electrode material for supercapacitors, Composites, Part B, 2019, 159, 4–12 CrossRef CAS.
- X. Hong, B. Zhang, E. Murphy, J. Zou and F. Kim, Three-dimensional reduced graphene oxide/polyaniline nanocomposite film prepared by diffusion driven layer-by-layer assembly for high-performance supercapacitors, J. Power Sources, 2017, 343, 60–66 CrossRef CAS.
- M. K. Chini and S. Chatterjee, Hydrothermally reduced nano porous graphene–polyaniline nanofiber composites for supercapacitor, FlatChem, 2017, 1, 1–5 CrossRef CAS.
- Z.-F. Li, H. Zhang, Q. Liu, L. Sun, L. Stanciu and J. Xie, Fabrication of High-Surface-Area Graphene/Polyaniline Nanocomposites and Their Application in Supercapacitors, ACS Appl. Mater. Interfaces, 2013, 5, 2685–2691 CrossRef CAS.
- N. A. Kumar, H.-J. Choi, Y. R. Shin, D. W. Chang, L. Dai and J.-B. Baek, Polyaniline-Grafted Reduced Graphene Oxide for Efficient Electrochemical Supercapacitors, ACS Nano, 2012, 6, 1715–1723 CrossRef CAS.
- L. Li, A.-R. O. Raji, H. Fei, Y. Yang, E. L. G. Samuel and J. M. Tour, Nanocomposite of Polyaniline Nanorods Grown on Graphene Nanoribbons for Highly Capacitive Pseudocapacitors, ACS Appl. Mater. Interfaces, 2013, 5, 6622–6627 CrossRef CAS.
- K. Li, X. Liu, S. Chen, W. Pan and J. Zhang, A flexible solid-state supercapacitor based on graphene/polyaniline paper electrodes, J. Energy Chem., 2019, 32, 166–173 CrossRef.
- S. Palsaniya, H. B. Nemade and A. K. Dasmahapatra, Hierarchical Nylon-6/reduced graphene oxide/polyaniline nanocomposites with enhanced dielectric properties for energy storage applications, J. Energy Storage, 2020, 32, 101821 CrossRef.
- F. Alvi, M. K. Ram, P. A. Basnayaka, E. Stefanakos, Y. Goswami and A. Kumar, Graphene–polyethylenedioxythiophene conducting polymer nanocomposite based supercapacitor, Electrochim. Acta, 2011, 56, 9406–9412 CrossRef CAS.
- Q. Zhou, D. Zhu, X. Ma, J. Xu, W. Zhou and F. Zhao, High-performance capacitive behavior of layered reduced graphene oxide and polyindole nanocomposite materials, RSC Adv., 2016, 6, 29840–29847 RSC.
- Z. Xu, Z. Zhang, H. Yin, S. Hou, H. Lin, J. Zhou and S. Zhuo, Investigation on the role of different conductive polymers in supercapacitors based on a zinc sulfide/reduced
graphene oxide/conductive polymer ternary composite electrode, RSC Adv., 2020, 10, 3122–3129 RSC.
- S. Li, Y. Chen, X. He, X. Mao, Y. Zhou, J. Xu and Y. Yang, Modifying Reduced Graphene Oxide by Conducting Polymer Through a Hydrothermal Polymerization Method and its Application as Energy Storage Electrodes, Nanoscale Res. Lett., 2019, 14, 226 CrossRef PubMed.
- K. Hareesh, S. R. Rondiya, N. Y. Dzade, S. D. Dhole, J. Williams and S. Sergey, Polymer-wrapped reduced graphene oxide/nickel cobalt ferrite nanocomposites as tertiary hybrid supercapacitors: insights from experiment and simulation, J. Sci.: Adv. Mater. Devices, 2021, 6, 291–301 CAS.
- S. N. J. Syed Zainol Abidin, S. Mamat, S. Abdul Rasyid, Z. Zainal and Y. Sulaiman, Fabrication of poly(vinyl alcohol)-graphene quantum dots coated with poly(3,4-ethylenedioxythiophene) for supercapacitor, J. Polym. Sci., Part A: Polym. Chem., 2017, 56, 50–58 CrossRef.
- Z. Zhao, H. Wang, H. Huang, L. Li and X. Yu, Graphene oxide/polypyrrole/polyaniline composite hydrogel synthesized by vapor-liquid interfacial method for supercapacitors, Colloids Surf., A, 2021, 626, 127125 CrossRef CAS.
- Z. Khanam, J. Liu and S. Song, Flexible graphene paper electrode prepared via polyvinyl alcohol-assisted shear-exfoliation for all-solid-state polymer supercapacitor application, Electrochim. Acta, 2020, 363, 137208 CrossRef CAS.
- R. Lakra, R. Kumar, P. K. Sahoo, D. Thatoi and A. Soam, A mini-review: Graphene based composites for supercapacitor application, Inorg. Chem. Commun., 2021, 133, 108929 CrossRef CAS.
- X. Zhao, Z.-B. Liu, W.-B. Yan, Y. Wu, X.-L. Zhang, Y. Chen and J.-G. Tian, Ultrafast carrier dynamics and saturable absorption of solution-processable few-layered graphene oxide, Appl. Phys. Lett., 2011, 98(12), 121905 CrossRef.
- K. Thakur and B. Kandasubramanian, Graphene and Graphene Oxide-Based Composites for Removal of Organic Pollutants: A Review, J. Chem. Eng. Data, 2019, 64, 833–867 CrossRef CAS.
- K. C. Kemp, H. Seema, M. Saleh, N. H. Le, K. Mahesh, V. Chandra and K. S. Kim, Environmental applications using graphene composites: water remediation and gas adsorption, Nanoscale, 2013, 5, 3149 RSC.
- D. Li, M. B. Müller, S. Gilje, R. B. Kaner and G. G. Wallace, Processable aqueous dispersions of graphene nanosheets, Nat. Nanotechnol., 2008, 3, 101–105 CrossRef CAS.
- B. Yang, Z. Tian, L. Zhang, Y. Guo and S. Yan, Enhanced heterogeneous Fenton degradation of Methylene Blue by nanoscale zero valent iron (nZVI) assembled on magnetic Fe3O4/reduced graphene oxide, J. Water Process Eng., 2015, 5, 101–111 CrossRef.
- C.-E. Boström, P. Gerde, A. Hanberg, B. Jernström, C. Johansson, T. Kyrklund, A. Rannug, M. Törnqvist, K. Victorin and R. Westerholm, Cancer Risk Assessment, Indicators, and Guidelines for Polycyclic Aromatic Hydrocarbons in the Ambient Air, Environ. Health Perspect., 2002, 110, 451–489 Search PubMed.
- C. Cheng, Z. Liu, X. Li, B. Su, T. Zhou and C. Zhao, Graphene oxide interpenetrated polymeric composite hydrogels as highly effective adsorbents for water treatment, RSC Adv., 2014, 4, 42346–42357 RSC.
- X. Zhou, Y. Zhang, C. Wang, X. Wu, Y. Yang, B. Zheng, H. Wu, S. Guo and J. Zhang, Photo-Fenton Reaction of Graphene Oxide: A New Strategy to Prepare Graphene Quantum Dots for DNA Cleavage, ACS Nano, 2012, 6, 6592–6599 CrossRef CAS.
- I. Ali, O. M. L. Alharbi, A. Tkachev, E. Galunin, A. Burakov and V. A. Grachev, Water treatment by new-generation graphene materials: hope for bright future, Environ. Sci. Pollut. Res., 2018, 25, 7315–7329 CrossRef CAS.
- H. Sun, S. Liu, G. Zhou, H. M. Ang, M. O. Tadé and S. Wang, Reduced Graphene Oxide for Catalytic Oxidation of Aqueous Organic Pollutants, ACS Appl. Mater. Interfaces, 2012, 4, 5466–5471 CrossRef CAS PubMed.
- X. Fan, G. Zhang and F. Zhang, Multiple roles of graphene in heterogeneous catalysis, Chem. Soc. Rev., 2015, 44, 3023–3035 RSC.
- G. K. Ramesha, A. Vijaya Kumara, H. B. Muralidhara and S. Sampath, Graphene and graphene oxide as effective adsorbents toward anionic and cationic dyes, J. Colloid Interface Sci., 2011, 361, 270–277 CrossRef CAS PubMed.
- Y. Liu, C. Liu and Y. Liu, Investigation on fluorescence quenching of dyes by graphite oxide and graphene, Appl. Surf. Sci., 2011, 257, 5513–5518 CrossRef CAS.
- Q. Wu, G. Zhao, C. Feng, C. Wang and Z. Wang, Preparation of a graphene-based magnetic nanocomposite for the extraction of carbamate pesticides from environmental water samples, J. Chromatogr. A, 2011, 1218, 7936–7942 CrossRef CAS.
- Y. Gao, Y. Li, L. Zhang, H. Huang, J. Hu, S. M. Shah and X. Su, Adsorption and removal of tetracycline antibiotics from aqueous solution by graphene oxide, J. Colloid Interface Sci., 2012, 368, 540–546 CrossRef CAS.
- Y. Li, Q. Du, T. Liu, X. Peng, J. Wang, J. Sun, Y. Wang, S. Wu, Z. Wang, Y. Xia and L. Xia, Comparative study of methylene blue dye adsorption onto activated carbon, graphene oxide, and carbon nanotubes, Chem. Eng. Res. Des., 2013, 91, 361–368 CrossRef CAS.
- L. A. Loeb and C. C. Harris, Advances in Chemical Carcinogenesis: A Historical Review and Prospective, Cancer Res., 2008, 68, 6863–6872 CrossRef CAS.
- H. M. Korashy and A. O. S. El-Kadi, The Role of Aryl Hydrocarbon Receptor in the Pathogenesis of Cardiovascular Diseases, Drug Metab. Rev., 2006, 38, 411–450 CrossRef CAS PubMed.
- R. H. McKee, J. H. C. M. Lammers, H. Muijser, D. E. Owen and B. M. Kulig, Neurobehavioral Effects of Acute Exposure to Aromatic Hydrocarbons, Int. J. Toxicol., 2010, 29, 277–290 CrossRef CAS.
- M. Husain and Q. Husain, Applications of Redox Mediators in the Treatment of Organic Pollutants by Using Oxidoreductive Enzymes: A Review, Crit. Rev. Environ. Sci. Technol., 2007, 38, 1–42 CrossRef.
- I. Michael, L. Rizzo, C. S. McArdell, C. M. Manaia, C. Merlin, T. Schwartz, C. Dagot and D. Fatta-Kassinos, Urban wastewater treatment plants as hotspots for the release of antibiotics in the environment: A review, Water Res., 2013, 47, 957–995 CrossRef CAS.
- O. A. H. Jones, N. Voulvoulis and J. N. Lester, Human Pharmaceuticals in the Aquatic Environment a Review, Environ. Technol., 2001, 22, 1383–1394 CrossRef CAS.
- F. Marahel, M. A. Khan, E. Marahel, I. Bayesti and S. Hosseini, Kinetics, thermodynamics, and isotherm studies for the adsorption of BR2 dye onto avocado integument, Desalin. Water Treat., 2013, 53, 826–835 CrossRef.
- Q. Liu, J. Shi, J. Sun, T. Wang, L. Zeng and G. Jiang, Graphene and Graphene Oxide Sheets Supported on Silica as Versatile and High-Performance Adsorbents for Solid-Phase Extraction, Angew. Chem., Int. Ed., 2011, 50, 5913–5917 CrossRef CAS PubMed.
- W. Zhang, C. Zhou, W. Zhou, A. Lei, Q. Zhang, Q. Wan and B. Zou, Fast and Considerable Adsorption of Methylene Blue Dye onto Graphene Oxide, Bull. Environ. Contam. Toxicol., 2011, 87, 86–90 CrossRef CAS.
- L. Sun, H. Yu and B. Fugetsu, Graphene
oxide adsorption enhanced by in situ reduction with sodium hydrosulfite to remove acridine orange from aqueous solution, J. Hazard. Mater., 2012, 203–204, 101–110 CrossRef CAS PubMed.
- Z. Hosseinabadi-Farahani, H. Hosseini-Monfared and N. M. Mahmoodi, Graphene oxide nanosheet: preparation and dye removal from binary system colored wastewater, Desalin. Water Treat., 2014, 56, 2382–2394 CrossRef.
- H. Yan, X. Tao, Z. Yang, K. Li, H. Yang, A. Li and R. Cheng, Effects of the oxidation degree of graphene oxide on the adsorption of methylene blue, J. Hazard. Mater., 2014, 268, 191–198 CrossRef CAS.
- W. Konicki, M. Aleksandrzak, D. Moszyński and E. Mijowska, Adsorption of anionic azo-dyes from aqueous solutions onto graphene oxide: Equilibrium, kinetic and thermodynamic studies, J. Colloid Interface Sci., 2017, 496, 188–200 CrossRef CAS.
- S. S. Gupta, T. S. Sreeprasad, S. M. Maliyekkal, S. K. Das and T. Pradeep, Graphene from Sugar and its Application in Water Purification, ACS Appl. Mater. Interfaces, 2012, 4, 4156–4163 CrossRef CAS.
- K. Thakur and B. Kandasubramanian, Graphene and Graphene Oxide-Based Composites for Removal of Organic Pollutants: A Review, J. Chem. Eng. Data, 2019, 64, 833–867 CrossRef CAS.
- V. Ganesan, C. Louis and S. P. Damodaran, Graphene oxide-wrapped magnetite nanoclusters: A recyclable functional hybrid for fast and highly efficient removal of organic dyes from wastewater, J. Environ. Chem. Eng., 2018, 6, 2176–2190 CrossRef CAS.
- T. R. Das, S. Patra, R. Madhuri and P. K. Sharma, Bismuth oxide decorated graphene oxide nanocomposites synthesized via sonochemical assisted hydrothermal method for adsorption of cationic organic dyes, J. Colloid Interface Sci., 2018, 509, 82–93 CrossRef CAS PubMed.
- J. Bockris, The origin of ideas on a Hydrogen Economy and its solution to the decay of the environment, Int. J. Hydrogen Energy, 2002, 27, 731–740 CrossRef CAS.
- N. Han, P. Liu, J. Jiang, L. Ai, Z. Shao and S. Liu, Recent advances in nanostructured metal nitrides for water splitting, J. Mater. Chem. A, 2018, 6, 19912–19933 RSC.
- J. Song, C. Wei, Z.-F. Huang, C. Liu, L. Zeng, X. Wang and Z. J. Xu, A review on fundamentals for designing oxygen evolution electrocatalysts, Chem. Soc. Rev., 2020, 49, 2196–2214 RSC.
- G. Zhao, K. Rui, S. X. Dou and W. Sun, Boosting electrochemical water oxidation: the merits of heterostructured electrocatalysts, J. Mater. Chem. A, 2020, 8, 6393–6405 RSC.
- K. Kannimuthu, K. Sangeetha, S. Sam Sankar, A. Karmakar, R. Madhu and S. Kundu, Investigation on nanostructured Cu-based electrocatalysts for improvising water splitting: a review, Inorg. Chem. Front., 2021, 8, 234–272 RSC.
- T. Audichon, T. W. Napporn, C. Canaff, C. Morais, C. Comminges and K. B. Kokoh, IrO2 Coated on RuO2 as Efficient and Stable Electroactive Nanocatalysts for Electrochemical Water Splitting, J. Phys. Chem. C, 2016, 120, 2562–2573 CrossRef CAS.
- Y. Zhu, X. Ji, C. Pan, Q. Sun, W. Song, L. Fang, Q. Chen and C. E. Banks, A carbon quantum dot decorated RuO2 network: outstanding supercapacitances under ultrafast charge and discharge, Energy Environ. Sci., 2013, 6, 3665 RSC.
- R. R. Rao, M. J. Kolb, N. B. Halck, A. F. Pedersen, A. Mehta, H. You, K. A. Stoerzinger, Z. Feng, H. A. Hansen, H. Zhou, L. Giordano, J. Rossmeisl, T. Vegge, I. Chorkendorff, I. E. L. Stephens and Y. Shao-Horn, Towards identifying the active sites on RuO2(110) in catalyzing oxygen evolution, Energy Environ. Sci., 2017, 10, 2626–2637 RSC.
- A. K. Tareen, G. S. Priyanga, K. Khan, E. Pervaiz, T. Thomas and M. Yang, Nickel-Based Transition Metal Nitride Electrocatalysts for the Oxygen Evolution Reaction, ChemSusChem, 2019, 12, 3941–3954 CrossRef CAS PubMed.
- L. Wang, Z. Sofer and M. Pumera, Will Any Crap We Put into Graphene Increase Its Electrocatalytic Effect?, ACS Nano, 2020, 14, 21–25 CrossRef CAS.
- H. Cui, Z. Zhou and D. Jia, Heteroatom-doped graphene as electrocatalysts for air cathodes, Mater. Horiz., 2017, 4, 7–19 RSC.
- H. Cui, M. Jiao, Y. Chen, Y. Guo, L. Yang, Z. Xie, Z. Zhou and S. Guo, Molten-Salt-Assisted Synthesis of 3D Holey N-Doped Graphene as Bifunctional Electrocatalysts for Rechargeable Zn–Air Batteries, Small Methods, 2018, 2(10), 1800144 CrossRef.
- P. Joshi, H.-H. Huang, R. Yadav, M. Hara and M. Yoshimura, Boron-doped graphene as electrocatalytic support for iridium oxide for oxygen evolution reaction, Catal. Sci. Technol., 2020, 10, 6599–6610 RSC.
- K. Kumar, I. Abidat, C. Canaff, A. Habrioux, C. Morais, T. W. Napporn and K. B. Kokoh, Metal Loading Effect on the Activity of Co3O4/N-Doped Reduced Graphene Oxide Nanocomposites as Bifunctional Oxygen Reduction/Evolution Catalysts, ChemElectroChem, 2017, 5, 483–493 CrossRef.
- J. M. Luque-Centeno, M. V. Martínez-Huerta, D. Sebastián, J. I. Pardo and M. J. Lázaro, CoTiO3/NrGO nanocomposites for oxygen evolution and oxygen reduction reactions: Synthesis and electrocatalytic performance, Electrochim. Acta, 2020, 331, 135396 CrossRef CAS.
- Y. Tong, P. Chen, T. Zhou, K. Xu, W. Chu, C. Wu and Y. Xie, A Bifunctional Hybrid Electrocatalyst for Oxygen Reduction and Evolution: Cobalt Oxide Nanoparticles Strongly Coupled to B,N-Decorated Graphene, Angew. Chem., Int. Ed., 2017, 56, 7121–7125 CrossRef CAS.
- V. S. Sapner, B. B. Mulik, R. V. Digraskar, S. S. Narwade and B. R. Sathe, Enhanced oxygen evolution reaction on amine functionalized graphene oxide in alkaline medium, RSC Adv., 2019, 9, 6444–6451 RSC.
- H. Jung, A. Karmakar, A. Adhikari, R. Patel and S. Kundu, Graphene-based materials as electrocatalysts for the oxygen evolution reaction: a review, Sustainable Energy Fuels, 2022, 6, 640–663 RSC.
- J. Sun, W. Zhang, S. Wang, Y. Ren, Q. Liu, Y. Sun, L. Tang, J. Guo and X. Zhang, Ni-Co-B nanosheets coupled with reduced graphene oxide towards enhanced electrochemical oxygen evolution, J. Alloys Compd., 2019, 776, 511–518 CrossRef CAS.
- X. Xing, R. Liu, K. Cao, U. Kaiser, G. Zhang and C. Streb, Manganese Vanadium Oxide–N-Doped Reduced Graphene Oxide Composites as Oxygen Reduction and Oxygen Evolution Electrocatalysts, ACS Appl. Mater. Interfaces, 2018, 10, 44511–44517 CrossRef CAS.
- S. Li, Y. Gao, N. Li, L. Ge, X. Bu and P. Feng, Transition metal-based bimetallic MOFs and MOF-derived catalysts for electrochemical oxygen evolution reaction, Energy Environ. Sci., 2021, 14, 1897–1927 RSC.
- K. Wang, K. N. Hui, K. San Hui, S. Peng and Y. Xu, Recent progress in metal–organic framework/graphene-derived materials for energy storage and conversion: design, preparation, and application, Chem. Sci., 2021, 12, 5737–5766 RSC.
- Y. Wang, B. Liu, X. Shen, H. Arandiyan, T. Zhao, Y. Li, M. Garbrecht, Z. Su, L. Han, A. Tricoli and C. Zhao, Oxygen Evolution Reaction: Engineering the Activity and Stability of MOF-Nanocomposites for Efficient Water Oxidation, Adv. Energy
Mater., 2021, 11(16), 2170063 CrossRef CAS.
- L. Yan, Y. Xu, P. Chen, S. Zhang, H. Jiang, L. Yang, Y. Wang, L. Zhang, J. Shen, X. Zhao and L. Wang, Heterostructure Films: A Freestanding 3D Heterostructure Film Stitched by MOF-Derived Carbon Nanotube Microsphere Superstructure and Reduced Graphene Oxide Sheets: A Superior Multifunctional Electrode for Overall Water Splitting and Zn–Air Batteries, Adv. Mater., 2020, 32(48) DOI:10.1002/adma.202003313.
- A. Xie, J. Du, F. Tao, Y. Tao, Z. Xiong, S. Luo, X. Li and C. Yao, Three-dimensional graphene surface-mounted nickel-based metal organic framework for oxygen evolution reaction, Electrochim. Acta, 2019, 305, 338–348 CrossRef CAS.
- W. Fang, J. Wang, Y. Hu, X. Cui, R. Zhu, Y. Zhang, C. Yue, J. Dang, W. Cui, H. Zhao and Z. Li, Metal-organic framework derived Fe-Co-CN/reduced graphene oxide for efficient HER and OER, Electrochim. Acta, 2021, 365, 137384 CrossRef CAS.
- Y. Wang, S. Wang, D. Liu, L. Zhou, R. Du, T.-T. Li, T. Miao, J. Qian, Y. Hu and S. Huang, Normal-pulse-voltage-assisted in situ fabrication of graphene-wrapped MOF-derived CuO nanoflowers for water oxidation, Chem. Commun., 2020, 56, 8750–8753 RSC.
- H. Jung, A. Karmakar, A. Adhikari, R. Patel and S. Kundu, Graphene-based materials as electrocatalysts for the oxygen evolution reaction: a review, Sustainable Energy Fuels, 2022, 6, 640–663 RSC.
- W. Liu, X. Qin, X. Zhang, Z. Shao and B. Yi, Wormholelike mesoporous carbon supported PtRu catalysts toward methanol electrooxidation, J. Energy Chem., 2017, 26, 200–206 CrossRef.
- A. Cavallin, M. Pozzo, C. Africh, A. Baraldi, E. Vesselli, C. Dri, G. Comelli, R. Larciprete, P. Lacovig, S. Lizzit and D. Alfè, Local Electronic Structure and Density of Edge and Facet Atoms at Rh Nanoclusters Self-Assembled on a Graphene Template, ACS Nano, 2012, 6, 3034–3043 CrossRef CAS PubMed.
- Y. Li, L. Tang and J. Li, Preparation and electrochemical performance for methanol oxidation of pt/graphene nanocomposites, Electrochem. Commun., 2009, 11, 846–849 CrossRef CAS.
- S. Wu, J. Liu, Z. Tian, Y. Cai, Y. Ye, Q. Yuan and C. Liang, Highly Dispersed Ultrafine Pt Nanoparticles on Reduced Graphene Oxide Nanosheets: In Situ Sacrificial Template Synthesis and Superior Electrocatalytic Performance for Methanol Oxidation, ACS Appl. Mater. Interfaces, 2015, 7, 22935–22940 CrossRef CAS PubMed.
- J. Liu, Q. Ma, Z. Huang, G. Liu and H. Zhang, Recent Progress in Graphene-Based Noble-Metal Nanocomposites for Electrocatalytic Applications, Adv. Mater., 2019, 31(9), 1800696 CrossRef.
- X. Zhang, J. Zhu, C. S. Tiwary, Z. Ma, H. Huang, J. Zhang, Z. Lu, W. Huang and Y. Wu, Palladium Nanoparticles Supported on Nitrogen and Sulfur Dual-Doped Graphene as Highly Active Electrocatalysts for Formic Acid and Methanol Oxidation, ACS Appl. Mater. Interfaces, 2016, 8, 10858–10865 CrossRef CAS PubMed.
- L. Li, Y. Wu, J. Lu, C. Nan and Y. Li, Synthesis of Pt–Ni/graphene via in situ reduction and its enhanced catalyst activity for methanol oxidation, Chem. Commun., 2013, 49, 7486 RSC.
- S. Guo, S. Li, T. Hu, G. Gou, R. Ren, J. Huang, M. Xie, J. Jin and J. Ma, Graphene decorated with Pd nanoparticles via electrostatic self-assembly: A highly active alcohol oxidation electrocatalyst, Electrochim. Acta, 2013, 109, 276–282 CrossRef CAS.
- L. Rao, Y.-X. Jiang, B.-W. Zhang, Y.-R. Cai and S.-G. Sun, High activity of cubic PtRh alloys supported on graphene towards ethanol electrooxidation, Phys. Chem. Chem. Phys., 2014, 16, 13662 RSC.
- C.-T. Hsieh, P.-Y. Yu, D.-Y. Tzou, J.-P. Hsu and Y.-R. Chiu, Bimetallic Pd–Rh nanoparticles onto reduced graphene oxide nanosheets as electrocatalysts for methanol oxidation, J. Electroanal. Chem., 2016, 761, 28–36 CrossRef CAS.
- M. S. Ahmed and S. Jeon, Highly Active Graphene-Supported NixPd100–xBinary Alloyed Catalysts for Electro-Oxidation of Ethanol in an Alkaline Media, ACS Catal., 2014, 4, 1830–1837 CrossRef CAS.
- S. Liu, N. Tian, A.-Y. Xie, J.-H. Du, J. Xiao, L. Liu, H.-Y. Sun, Z.-Y. Cheng, Z.-Y. Zhou and S.-G. Sun, Electrochemically Seed-Mediated Synthesis of Sub-10 nm Tetrahexahedral Pt Nanocrystals Supported on Graphene with Improved Catalytic Performance, J. Am. Chem. Soc., 2016, 138, 5753–5756 CrossRef CAS PubMed.
- W. Huang, H. Wang, J. Zhou, J. Wang, P. N. Duchesne, D. Muir, P. Zhang, N. Han, F. Zhao, M. Zeng, J. Zhong, C. Jin, Y. Li, S.-T. Lee and H. Dai, Highly active and durable methanol oxidation electrocatalyst based on the synergy of platinum–nickel hydroxide–graphene, Nat. Commun., 2015, 6, 10035 CrossRef CAS.
- Y. Kang, Q. Xue, P. Jin, J. Jiang, J. Zeng and Y. Chen, Rhodium Nanosheets–Reduced Graphene Oxide Hybrids: A Highly Active Platinum-Alternative Electrocatalyst for the Methanol Oxidation Reaction in Alkaline Media, ACS Sustainable Chem. Eng., 2017, 5, 10156–10162 CrossRef CAS.
- L. Li, Y. Wu, J. Lu, C. Nan and Y. Li, Synthesis of Pt–Ni/graphene via in situ reduction and its enhanced catalyst activity for methanol oxidation, Chem. Commun., 2013, 49, 7486 RSC.
Footnote |
† Both authors (RA & SH) contributed equally to this work. |
|
This journal is © The Royal Society of Chemistry and the Centre National de la Recherche Scientifique 2024 |