DOI:
10.1039/D4NH00385C
(Review Article)
Nanoscale Horiz., 2025,
10, 56-77
Semiconductor nanowire heterodimensional structures toward advanced optoelectronic devices
Received
7th August 2024
, Accepted 22nd October 2024
First published on 22nd October 2024
Abstract
Semiconductor nanowires are considered as one of the most promising candidates for next-generation devices due to their unique quasi-one-dimensional structures and novel physical properties. In recent years, advanced heterostructures have been developed by combining nanowires with low-dimensional structures such as quantum wells, quantum dots, and two-dimensional materials. Those heterodimensional structures overcome the limitations of homogeneous nanowires and show great potential in high-performance nano-optoelectronic devices. In this review, we summarize and discuss recent advances in fabrication, properties and applications of nanowire heterodimensional structures. Major heterodimensional structures including nanowire/quantum well, nanowire/quantum dot, and nanowire/2D-material are studied. Representative optoelectronic devices including lasers, single photon sources, light emitting diodes, photodetectors, and solar cells are introduced in detail. Related prospects and challenges are also discussed.

Xin Yan
| Xin Yan received the PhD degree in electronic science and technology from Beijing University of Posts and Telecommunications (BUPT) in 2014. He is currently an associate professor with the State Key Laboratory of Information Photonics and Optical Communications, BUPT. He has published more than 150+ research papers (100+ journal papers), holds 8 patents, and authored 2 books. His research interests include low-dimensional semiconductor materials and nano-optoelectronic devices. He served as an Associate Editor of Micro & Nano Letters (2019–2022), and is currently serving as an Associate Editor of Materials Express. He has been named among the Nanoscale 2023 Emerging Investigators by the journal Nanoscale. |

Yao Li
| Yao Li is studying for the PhD degree in electronic science and technology from Beijing University of Posts and Telecommunications (BUPT). She has published 3 journal papers and 2 conferences. Her research interests mainly include semiconductor nanowire solar cells and integrated photovoltaic antenna. |

Xia Zhang
| Xia Zhang received the PhD degree in electromagnetic fields and microwave techniques from Beijing University of Posts and Telecommunications (BUPT) in 2003. She is currently a professor with the State Key Laboratory of Information Photonics and Optical Communications, BUPT. She has published more than 200+ research papers and authored 2 books. Her research interests include low-dimensional materials and next-generation optoelectronic devices. She was selected into “the New Century Excellent Talents Program” by the Ministry of Education of China in 2008. |
1. Introduction
As current chips gradually approach the physical limit of Moore's Law, the development of next-generation chips based on low-dimensional semiconductors has currently become a focus of industry and scientific research. The quasi-one-dimensional semiconductor nanowires (NWs) are considered as one of the most promising candidates for next-generation chips due to their unique advantages of high material quality, large lattice-mismatch tolerance, three-dimensional integration capability, and excellent electrical and optical properties. The research on semiconductor nanowires (NWs) can be dated from the first demonstration of Si nanowhiskers grown via the bottom-up vapor–liquid–solid (VLS) mechanism in 1964, and really started to flourish in the 1990s marked by the realization of GaAs and InAs quantum wires.1–3 Over the past decades, great strides have been made in the fundamental physics, material growth, heterostructure formation, and device fabrication of NWs. To date, single-crystalline NWs of different materials, such as IV, II–VI, and III–V group semiconductors, have been successfully grown by carefully controlling the growth conditions as well as the NW parameters.4–6 Position-controlled uniform NW arrays have also been realized by the widely-known selective-area epitaxy (SAE) method.7–9 Based on these high-quality single NWs and NW arrays, a variety of high-performance nanoscale devices including field effect transistors (FETs), lasers, light emitting diodes (LEDs), photodetectors, single photon devices, and solar cells, have been demonstrated, showing great potential in wide applications of on-chip information emission and reception, lighting and display, as well as energy conversion.10–16
With the prosperity of NW research, some issues are also emerging, of which one practical fact is that the performance of many NW devices is still less than ideal, without showing outstanding advantages compared with conventional devices. For example, NW lasers are one of the most attractive nanoscale devices showing great potential in high-density on-chip/inter-chip optical communication and interconnection. However, the threshold of pure NW lasers is still very high, particularly at high temperature with a small diameter.17 Most importantly, electrically-pumped NW lasers have been rarely reported, seriously restricting the practical applications.18–20 In early studies, the material quality and fabrication technology are the main factors restricting the device performance. After the development of decades, the NW growth technique and device preparing process have become gradually mature. To date, defect-free phase-pure zinc blende (ZB) and wurtzite (WZ) NWs have been demonstrated in different materials, which substantially suppresses the carrier trap and scattering, resulting in enhanced carrier mobility and improved optical properties. Key preparation process such as micromanipulation, surface passivation, and ohmic contact have also been basically solved by different methods. This means that development of current NW devices is mainly hindered by the limitations of physical properties. In a broad sense, NWs are categorized as one type of low-dimensional materials. However, strictly speaking, most of the NWs currently studied still belong to the bulk material category, as the diameter of NWs is usually too large to generate quantum confinement effect, which is the most prominent feature of low-dimensional materials like quantum well (QW) and quantum dot (QD). For typical III–V NWs (such as GaAs, InP, InAs), a maximum diameter of ∼50 nm is required to obtain radial quantum confinement.21–23 Such thin NWs are difficult to grow reliably and hard to be applied in devices due to the poor mechanical stability, rich surface state states, and weak light confinement, etc. This means that in most cases, NW-based devices can take advantage of its structure feature including small size, large lattice-mismatch tolerance, smooth surface and high reflectivity end face, without benefiting from the quantum-confinement-induced performance leap such as ballistic transport, strong carrier confinement, high temperature stability, and fine wavelength control.
Thanks to the flexible quasi-one-dimensional structure, it is easy to form various advanced heterostructures on the NW platform by combining different functional structures together to improve the performance and expand the functionality. The most popular NW heterostructures are axial and core–shell structures, which are formed by growing different materials along the axial and radial direction of NWs, respectively. By introducing low-dimensional structures into the NW along the axial or radial direction, different types of NW heterodimensional structures have been reported, mainly including axial NW/QW, radial NW/QW, axial NW/QD, and radial NW/QD, etc. Benefiting from the quantum confinement effect of the introduced low-dimensional structures, NW heterodimensional structures exhibit significantly improved performance and show great potential in low-threshold multi-wavelength lasers, high-detectivity infrared photodetectors, high-performance single photon sources (SPSs), etc.24–26 In recent years, two-dimensional (2D) materials, such as graphene, MoS2, black phosphorus, and hBN, are emerging as a new type of promising low-dimensional structures which can build van der Waals heterostructures for novel optoelectronic devices.27 The ultra-small thickness (only few atomic layers) make the 2D materials have high flexibility, but at the same time, poor absorption efficiency. By combining semiconductor NWs and 2D materials, all-flexible optoelectronic devices with excellent optoelectronic performance have been demonstrated.28,29
This paper aims to give a comprehensive review of the fabrication, properties and optoelectronic applications of NW heterodimensional structures. Major heterodimensional structures, including NW/QW, NW/QD, and NW/2D-material based on IV, III–V, and II–VI group semiconductor materials, are studied. Schematic of those heterodimensional structures are presented in Fig. 1. For the NW/QW structure, we will summarize the efforts for the controllable growth of axial NW QW (including NW superlattice) and core–(multi)shell NW/QW structures. In the NW/QD section, we will discuss the growth and properties of QD-in-NW and QD-on-NW structures formed by different mechanisms including VLS, strain-driven, phase segregation, and thermally-driven. In the NW/2D-material case, we will focus on NW/graphene structures formed either by direct growth of NWs on graphene, or by contacting graphene with NWs. Representative optoelectronic devices based on NW heterodimensional structures, including lasers, SPSs, LEDs, photodetectors, and solar cells will be introduced in detail. Related prospects and challenges will also be discussed.
 |
| Fig. 1 NW heterodimensional structures. (A) Axial NW/QW structure. (B) Core–multishell NW/QW structure. (C) QD-in-NW structure. (D) QD-on-NW structure. (E) Graphene/vertical NW array structure. (F) Graphene/lateral single NW structure. | |
2. NW heterodimensional structures
2.1. NW/QW structures
QW is one of the most popular low-dimensional structures which has been widely used in telecommunication lasers and infrared photodetectors. Compared with bulk structures, QW possesses quantized discrete energy levels in the direction perpendicular to the plane, leading to strong carrier confinement, high efficiency, and good temperature stability. For conventional planar QW structures, the lattice constant of well and barrier material should be matched or close to avoid lattice-mismatch dislocations which severe degrade the device performance. While for NWs, strain relaxation through the sidewalls allows the combination of lattice mismatched materials axially without creating dislocations.30 Axial NW/QWs are typically fabricated by controlling the growth time to form a thin layer in the axial direction, which have been widely reported in nitride and arsenide material system.31–39Fig. 2A shows the high angle annular dark field (HAADF) STEM and bright field high resolution transmission electron microscopy (HRTEM) images of axial AlN/GaN NW/QW heterostructures.31 The GaN QWs could be clearly identified by the bright contrast. In the axial GaN/(In,Ga)N and AlN/GaN NW/QW heterostructures, weaker quantum-confined Stark effect has been observed, which is attributed to the strain relaxation ability of NW that reduces the piezoelectric component of the polarization difference.32,33 By changing the composition or thickness of the well, the emission wavelength of QW could be tuned for different applications. Ra et al. reported high-quality axial InxGa1−xN/GaN multiple QW NWs on Si substrate grown by metal–organic chemical vapor deposition (MOCVD), whose emission wavelength could be tuned from 415.3 nm to 520.4 nm at room temperature as the In content increases from 0.10 to 0.28.39 It should be noted that for very thin NWs, the inserted QWs behave like QDs due to the strong axial and radial confinement, which will be discussed in detail in the NW/QD section.
 |
| Fig. 2 NW/QW heterodimensional structures. (A) High angle annular dark field (HAADF) STEM and bright field high resolution transmission electron microscopy (HRTEM) images of axial GaN QWs in a GaN/AlN NW. The GaN QWs appear in bright contrast. Reproduced with permission.31 Copyright 2011, American Physical Society. (B) Schematics, SEM, STEM intensity profile, and HAADF-STEM images of the GaAs/GaP NW superlattice. Reproduced with permission.40 Copyright 2023, Royal Society of Chemistry. (C) Cross-sectional HAADF STEM images of core–multishell GaAs/AlGaAs NW/QW structures with different GaAs QW thickness of 8 nm and 4 nm. Bright regions correspond to GaAs and dark regions correspond to AlGaAs. (D) False-color images of the ground state for the electrons in the 8 nm and 4 nm QWs calculated by finite element calculation. Reproduced with permission.41 Copyright 2013, American Chemical Society. | |
Different from the planar QW growth, the catalyst droplet driving the NW growth plays a critical role in the axial NW/QW heterostructure characteristics. Dick et al. presented an extensive investigation of Au-catalyzed axial heterostructure NWs composed of group IV and III–V materials and found that the interface energies between the two materials and the particle plays a dominant role in the heterostructure morphology (straight or kinked).42 For any combination of two materials, straight growth typically occurs in only one interface direction (e.g. GaAs on InAs). While in the opposite direction (e.g. InAs on GaAs), kinked or branched morphology is obtained. The reservoir effect of the catalyst has a strong impact on the heteointerface sharpness. Take the III–V material as an example. The group III species typically have much higher solubilities in many seed metals than the group V species, resulting in sharper interfaces in group V modulation while grading interfaces in group III modulation.43 Many theoretical and experimental works have been done on the mechanism and approach of achieving straight axial NW heterostructures with sharp interfaces.44–56 Messing et al. demonstrated the growth of straight InAs-on-GaAs NW heterostructures with a sharp interface by a judicious choice of NW diameter and by tailoring the growth parameters that control crystal structure.44 Zannier et al. found that the nanoparticle composition played a dominant role in the NW morphology, and straight InAs segments on GaAs required high group III/Au ratios in the nanoparticle.45 Straight axial GaAs/InxGa1−xAs/GaAs NW heterostructures was also achieved by catalyst-free growth method, avoiding the foreign-catalyst-induced kinking.47 Several models have been built to elucidate the reservoir effect. Glas compared two models predicting the composition distribution through axial heterostructures in III–V NWs grown in the VLS mode.48 Dubrovskii presented a self-consistent growth model of VLS III–V NW heterostructures based on group V interchange, and showed that sharp interfaces were not guaranteed in the general case, and their optimization required fine tuning of the growth parameters.49 Leshchenko et al. presented a model based on mass balance of atoms in the catalyst droplet and explained how and why the decrease of growth temperature and increase of the flux improved the interface abruptness.50 Different methods have been developed to control the abruptness of axial heterojunctions in NWs. Dick et al. showed that the InAs–GaAs interface sharpness could be significantly improved by pulsing the Ga source during heterojunction formation which lowered the In solubility.51 GaP/GaAs NW interface abruptness was drastically improved by switching off all the molecular beam fluxes for a short time at the group V commutation.52 Priante et al. demonstrated that interfaces of self-catalyzed NW heterostructures were generally sharper than those Au-catalyzed, and the size of the droplet was a major factor controlling the abruptness of the junction.53
NW superlattice is a special kind of axial NW/QW structures. A typical NW superlattice is formed by alternate stacking of two materials inside a single NW. Benefiting from the excellent strain relaxation ability, materials with large lattice-mismatch could be grown along the NW axis without dislocations, such as GaAs/GaP, InAs/GaAs, InAs/InP, and Si/(Si)Ge.40,57–61Fig. 2B shows a GaAs/GaP NW superlattice with layer thicknesses of 3 nm for GaAs and 3 nm for GaP. Despite the flexible band gap engineering capability, NW superlattices exhibit unique physical properties, such as ultralow thermal conductivity in Si/Ge, and peculiar electron–phonon interactions in GaAs/AlAs.61,62 In addition to the alternate stacking of different materials, NW superlattices can also be formed by periodically changing the crystal phase along the NW direction of one material, which can not be realized in conventional planar structures. This novel structure is achievable as the NW crystal phase can be precisely controlled with atomic layer accuracy by adjusting the growth conditions, such as temperature and III–V ratio.63–65 As this type of superlattice is composed of the same material, the interface-energy-related NW kinking and reservoir-effect-induced interface grading can be avoided. Due to the different energy band structure between ZB and WZ, periodic quantum wells and barriers are formed along a single NW, often exhibiting a “type II” band-alignment with electrons on ZB and holes on WZ for most III–V compounds.66–68 The presence of type II heterojunctions, on one hand, leads to a long radiative recombination lifetime, but on the other hand, has been demonstrated to reduce the charge-carrier cooling rate in NWs, and hence both reduce recombination rates and limit energy losses by allowing hot-carrier harvesting.68 Another promising NW superlattices is the twinning superlattice (TSL), which is formed in a single compound with periodically spaced twin boundaries and sharp interface junctions. The interface between the two orientations in a TSL is perfectly lattice-matched but at the same time highly wavefunction-symmetry-mismatched. This gives rise to large electron scattering at the interface and leads to the appearance of prominent miniband structures. Compared with other types of superlattices such as polytype superlattices, the TSL has the advantages of chemical simplicity and wide choice of materials.69 Theoretical studies have suggested that TSLs offer almost as much versatility in tailoring the electronic miniband structure as there exists in ordinary heterostructure-based superlattices, and the intraband absorption between the valence-band states has rather high values.70,71 Luca et al. experimentally demonstrated the continuous tuning of the phononic properties of a TSL GaP NW by controlling its periodicity.72 The most common way of TSL formation in NWs is introducing impurity dopants during growth.73–75 Dopant-free TSL formation has also been observed in InSb, InP, GaP, InAs, ZnSe, and Zn3P2 NWs by carefully controlling the growth parameters.76–80 Compared with the twin-free NWs, the TSL introduces promising physical properties such as drastic reduction of the thermal conductivity, inter-sub-band transition, and flexible phonon engineering, showing great application potential in thermoelectric, infrared, and phononic devices.81–84
Unlike the axial NW/QW structure that allows the combination of large-lattice-mismatched materials, the core–(multi)shell NW/QW structure has a relatively rigorous requirement for the lattice match as the stress relaxation ability is weak due to a large interface area. Nevertheless, theoretical studies have shown that NW core–shell structure can sufficiently reduce the strain in the shell via passing the strain into the core as well as a self-releasing mechanism.85 This means that compared with planar structures, thicker lattice-mismatched QWs can be coaxially grown on NW without dislocations. To date, core–(multi)shell NW/QW structures have been widely reported not only in lattice-matched AlGaAs/GaAs, InP/InGaAs, and AlN/GaN, but also in lattice-mismatched GaAs/InGaAs and GaN/InGaN.41,86–89 For both catalyst-assisted and catalyst-free NWs, QW shells can be formed by switching the growth from axial direction to radial direction via changing the growth temperature. It should be noted that in the core–(multi)shell structures, QW tubes are formed rather than QW planes in conventional planar structures, as shown in Fig. 2C.41 This tubular QW form is expected to confine the electrons and holes to extremely localized one-dimensional filaments along the corners of the hexagonally symmetric structure and extend along the length of NW, leading to ultra high quantum efficiency and intense emission, as theoretically demonstrated in Fig. 2D. The NW-based tubular QW has also been demonstrated to be an ideal platform for studies of the Aharonov–Bohm effect of neutral and charged excitons.90 In the core–(multi)shell NW/QW structures, the emission wavelength of QWs can be flexibly tuned by adjusting the thickness or composition. Fonseka et al. reported a large emission wavelength shift from ∼1200 nm to ∼1550 nm by increasing the QW thickness from 2.12 nm to 10.19 nm in the InP/InxGa1−xAs core–(multi)shell NW/QW structures.41 By increasing the In composition in the QW, the emission wavelength also exhibits an obvious blue shift.
Group IV core–(multi)shell NW heterostructures have attracted increasing attention in recent years due to their compatibility with Si technology. SiGe coaxial QWs on ordered Si NWs have been fabricated by a combination of nanosphere lithography, metal assisted chemical etching and epitaxial growth, which exhibited significantly enhanced emission in comparison with that from the planar SiGe QW due to the strong coupling between the emissions of the QW and the resonant modes of the NWs.91 Among group IV materials, GeSn has gained particular interest for its potential to achieve a direct band gap transition. Direct band gap core–shell Ge/GeSn NW heterostructures have been demonstrated by different groups, showing great promise in low-cost and high-efficiency infrared devices.92–94 Although haven’t been reported yet, the Ge/GeSn core–(multi)shell NW/QW structures are expected to have significantly improved performance, inferred from the recently reported planar Ge/GeSn QW devices.95,96 Another interesting NW core–(multi)shell heterostructure is the hybrid III–V/IV NW structure, which integrates optically-active III–V and electronic-suitable IV materials on the same NWs.97,98 Direct-bandgap hexagonal Ge and SiGe shells have been obtained on WZ GaAs cores, providing great potential for the integration of photonics and electronics in the nanoscale.99
Compared with the widely reported vertical-aligned NWs, in-plane NWs show unique potential in highly integrated electronic and photonic devices due to their compatibility with planar Si technology.100 Template-assisted selective epitaxy (TASE) is one of the most popular approaches to obtain NW-based heterostructures and devices co-planarly integrated with Si.101 With the TASE method, Brugnolotto et al. demonstrated the growth of in-plane III–V NW/QW heterostructures directly on Si(110) substrate.102 No propagating dislocations or antiboundary defects are detected in these structures, suggesting that TASE is promising for integrating electronic and photonic devices.
2.2. NW/QD structures
QD is a zero-dimensional structure with strong three-dimensional quantum confinement, which has shown great potential in ultra-low-threshold lasers, high-quality SPSs, normal-incidence infrared photodetectors, intermediate band solar cells, etc., due to its excellent optical properties. Self-assembled QDs on a planar substrate follows the well-known Stranski–Krastanov (SK) growth mode, which appers only when the lattice mismatch between the epitaxial layer and substrate materials is relatively large, while the surface energy and interface energy are not very big. Formation of QDs on the NW platform, however, is not limited to the SK growth mode, and the combination of materials is vastly extended. According to the position relationship between NW and QD, NW/QD structures can be divided into “QD-in-NW” and “QD-on-NW”.103 In the VLS growth case, QD-in-NW structure is easy to be formed by inserting a thin slice of a smaller band gap material during the process of growth of a NW of a high band gap material, which typically adopts the same diameter as the NW.104–109 This is very similar to the axial NW/QW structure, except that the NW diameter should be small enough to achieve radial quantum confinement. This type of QD is usually referred to as “quantum disk” due to the low aspect ratio, which results a strong quantum confinement in the axial direction and weak confinement in the radial direction. As the QD is grown via the VLS mode rather than SK, the QD-in-NW structure can also be realized in lattice-matched materials, such as AlGaAs/GaAs, as shown in Fig. 3A.108 Compared with self-assembled QDs on a planar substrate, one major advantage of the QD-in-NW structure is the high controllability of the QD properties as the QD width is defined by the NW diameter, while the QD height at a specific temperature is controlled by flux and growth time. QD-in-NW structures can also be fabricated by catalyst-free growth. F. Glas et al. argued that in this case, deposition of a mismatched material on the top facet of a NW may lead to the formation of a cylindrical island narrower than the NW stem, similar to Volmer–Weber (VW) or SK growth, which has been observed in GaN/InGaN NW/QD structures.110,111 With the SAE method, AlGaAs/GaAs, GaAs/In(Ga)As, and AlGaN/GaN NW/QD structures have been successfully fabricated.112–117 In these structures, the position of each QD can be well controlled as the lateral position could be defined by the NW location (the aperture position), and the distance from the substrate could be controlled by the NW height. Position-controlled InP/InAsP NW/QD structures have also been achieved via a combined SAE and VLS epitaxy approach.118–120 Position selection opens up the possibility for deterministic integration of QDs with photonic structures for obtaining high efficiency optoelectronic devices.
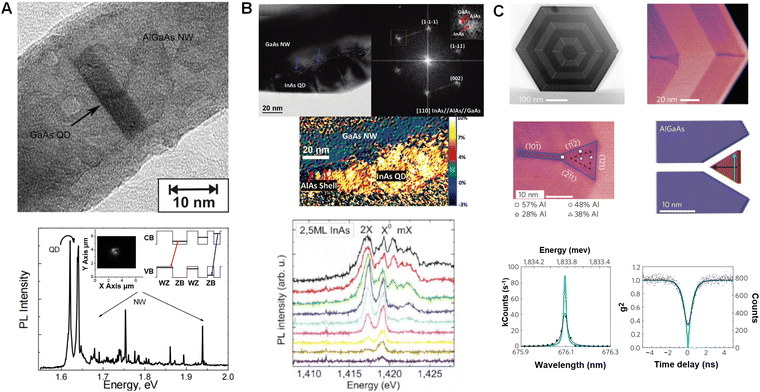 |
| Fig. 3 NW/QD structures. (A) TEM image and micro-photoluminescence spectrum (T = 6 K) of the AlGaAs/GaAs QD-in-NW structure. The single GaAs QD is identified by the dark contrast. Reproduced with permission.108 Copyright 2012, Institute of Physics. (B) TEM, fast Fourier transform (FFT), geometrical phase analysis (GPA), and excitation-power-dependent photoluminescence of the SK InAs QD on the sidewalls of core–shell GaAs/AlAs NW. Reproduced with permission.121 Copyright 2010, American Chemical Society. (C) HAADF STEM image, three-dimensional atomic model, photoluminescence (T = 4.2 K), and g2 measurement of the AlGaAs NW/QD structure formed by phase segregation. Reproduced with permission.122 Copyright 2013, Nature Publishing Group. | |
Compared with the QD-in-NW structure, the fabrication of QD-on-NW structure is relatively more complicated. As the lateral surface area of NW is large, it is possible to grow nanoscale islands on the side walls following the traditional SK growth method. However, it is found that for a very thin NW core, a smooth shell rather than QDs is formed, indicating that the existing theory on the growth on planar substrates is no longer suitable for the growth on the surface with nanoscale curvature.123 To elucidate the SK transition in the strain-induced growth on NW surface, Li et al. established a theoretical model and found that the SK mode can change to the Frank–van der Merwe (FM) mode due to the limit of the nanoscale curved surface.124 The formation of SK QDs on NW surface was firstly demonstrated by Pan et al.125 In their experiment, irregularly truncated pyramidal shaped Ge islands are observed around the Si NW, and a thin Ge layer is seen surrounding the core, which is consistent with SK type growth. However, no subsequent electrical or optical properties have been reported related to this structure, perhaps due to the low quality of Ge QDs with dense misfit dislocations and stacking faults. The first III–V QD-on-NW structure is built by decoration of the GaAs NW facet with S–K InAs QDs, as shown in Fig. 3B.121 Here, the GaAs NWs with {110} side facets are firstly grown by molecular beam epitaxy (MBE) with the Ga-assisted VLS method. As the low-surface-energy {110} surface is unfavorable to the formation of InAs QDs, a thin AlAs layer was deposited around the GaAs NW to modify the surface properties. As a result, high quality InAs QDs are successfully formed. Moreover, the excitation of single and double excitons in the QDs are demonstrated by low-temperature photoluminescence spectroscopy, opening new possibilities for the development of quantum optical devices, lasers, and intermediate-band solar cells. SK InAs QDs have also been directly grown on the surface of GaAs and InP NWs by Au-catalyzed MOCVD method.126–131 The III–V NWs grown by MOCVD typically have high-surface-energy {112} facets, which are favorable to the formation of SK QDs. It is found that the QDs are formed by adatom diffusion from the substrate as well as the sidewalls of the NWs, and the formation and distribution of QDs are strongly influenced by the deposition time of QDs as well as the diameter of NWs. Particularly, photoluminescence emission from the InAs QDs was observed at room temperature for the first time, showing great potential in practical optoelectronic devices. By changing the QD composition and NW material, the morphology, size, and strain of QDs can be tuned to achieve different emission wavelength.132–135 Compared with the QD-in-NW structure usually contains a few number of QDs, hundreds and thousands of SK QDs can be formed on the NW surface to provide much higher gain, which is particularly promising for lasers and photodetectors. Isolated SK QDs on NW have also been fabricated by different methods of using branched NWs, introducing quantum rings, and surface modification.136–138 Generally speaking, the control of size and position of SK QDs on NW is still difficult due to the self-assembled growth mode and adatom diffusion mechanism. In addition, it is observed that the emission wavelength of QD on NW is much shorter than on the planar substrate, limiting its applications in optical telecommunications.121,127,132 At present, the blueshift still remains unclear and needs further investigation.
The formation of QDs on NWs has also been realized by non-strain-driven mechanisms. Heiss et al. reported a special NW/QD structure which contains a few QDs at the edge of NW, as shown in Fig. 3C.122 The structure consists of Al-poor AlxGa1−xAs QDs in an Al-rich AlxGa1−xAs barrier wrapped in an intermediate Al-content matrix. The formation is driven by the different Ga and Al adatom mobilities on the NW surface, leading to Al segregation. The QDs exhibit excellent optical properties even when they are very close to the surface. Inhomogeneities in the indium concentration of InGaAs NWs also lead to the formation of In-rich QDs.139 Recently, lattice-matched Ge QDs have been grown on the sidewall facets of GaAs NWs using thermally-driven mechanism.140 Without the requirement of large-lattice-mismatch, NW/QD structures covering a broad class of materials can be fabricated for various applications.
2.3. NW/2D-material structures
The combination of NWs and 2D materials is attractive for the realization of all-flexible devices with excellent performance. The NW/2D-material structures can be built by direct growth of NWs on a flexible 2D-material substrate, or forming heterostructures through van der Waals interactions. Graphene is one of the most promising 2D materials which can serve as a low-cost high-flexible substrate for epitaxial growth of NWs and simultaneously act as a transparent electrode.141 Theoretical calculations have been employed to investigate the adatom adsorption on graphene, which predict the possibility of epitaxy for growing semiconductor materials on graphene.142,143 To date, group II–VI, IV, and III–V semiconductor NWs have been grown on the graphene substrate. Kim et al. demonstrated the growth vertically aligned ZnO NWs on graphene layers using catalyst-free MOCVD method.144 Few-layer-graphene sheets were firstly transferred onto the SiO2/Si substrates using a simple mechanical exfoliation technique, and then ZnO NWs were grown with the reactants diethylzinc (DEZn) and oxygen. The epitaxy of ZnO NWs on graphene has also been achieved by using Au-catalyzed growth method, and the morphology of NWs strongly depends on the Au film thickness.145 Growth of Si NWs has been demonstrated on graphene by Au-catalyzed VLS mechanism.146 Graphene was firstly transferred onto a SiO2/Si substrate using chemical exfoliation method, and Si NWs were then grown using Au as a catalyst. However, the Si NWs were randomly oriented with no preferred growth direction. Growth of III–V NWs on graphene has been widely investigated due to the potential in all-flexible optoelectronic devices. Munshi et al. proposed a generic atomic model to describe the possible epitaxial growth of III–V semiconductor NWs on graphene, which was demonstrated experimentally with the growth of GaAs NWs on graphitic substrate via the Ga-catalyzed VLS MBE method.143 Hong et al. demonstrated the growth of InAs NWs on graphene for the first time by catalyst-free MOCVD technique, as shown in Fig. 4A.147 Before growth, a 20 nm thick SiOx mask layer was deposited on the graphene by sputtering to achieve SAE. By optimizing the growth conditions, non-tapered vertical InAs NWs with uniform dimension and high yield is obtained. Kang et al. reported the self-assisted growth of InAs NWs on graphene by MBE.148 They studied the influence of substrate on the morphological and structural properties of the NWs and found that the buffer layer is more suitable for InAs NWs growth. The InAs NWs exhibited a semi-ballistic behavior in the conductance measurements, and multiple Andreev reflections in the non-linear regime in Josephson junction measurements, showing great potential in electronic devices. Epitaxial growth of GaN NWs on graphene was demonstrated by using catalyst-free MBE.149 Both the density and height of NWs decrease with increasing number of graphene layers. The NWs exhibit defect-free nature and present excellent optical properties, demonstrating that the graphene substrate is promising for the epitaxy of high quality GaN NWs. Morassi et al. reported the SAE growth of vertically aligned GaN NWs on graphene nanodots by plasma-assisted MBE (PA-MBE), which was the first demonstration of NW growth on a regular array of graphene nanodomains.150 The graphene nanodots were patterned by electron beam lithography (EBL) from polycrystalline graphene patches which were transferred to SiO2 substrates. Vertically aligned GaN NW array was then selectively grown on graphene nanodomains.
 |
| Fig. 4 NW/graphene structures. (A) FEM, TEM, FFT, HRTEM, and atomic model images of InAs NWs directly grown on graphene by catalyst-free MOCVD technique. Reproduced with permission.147 Copyright 2012, American Chemical Society. (B) Schematic, top-view and side-view SEM images of graphene/Si NW array Schottky junctin. Reproduced with permission.151 Copyright 2011, American Chemical Society. | |
Compared with the widely-reported elemental and binary NWs grown on graphene, the reports of ternary NWs grown on graphene are limited, probably due to the difficulty in controling the composition and morphology of NWs. Mohseni et al. compared the growth of InAs and InGaAs NWs on graphene by MOCVD.152 They found that unlike the vertically-aligned single-crystalline InAs NWs on graphene, the growth of InGaAs NWs on graphene results in a spontaneous phase separation, yielding an InAs/InxGa1−xAs core–shell structure. They attribute this phenomenon to that the lack of dangling bonds on graphene makes it unable to share the strain through elastic deformation between InGaAs and graphene. Different groups have demonstrated the growth of GaAs1−xSbx NWs on graphene by self-catalyzed MBE technique, showing great potential in near-infrared lasers and photodetectors.153,154 Vertically-aligned ultrahigh-aspect-ratio InAsSb NWs have also been grown on graphite by In-assisted MBE, which exhibited bright band-to-band related emission.155
It is worth mentioning that besides graphene, other 2D materials can also act as substrates for NW growth. For example, Mohseni et al. reported the growth of InxGa1−xAs NWs on MoS2, and found no InGaAs phase segregation, which was observed in the growth of InxGa1−xAs NWs on graphene.152 The growth of InP NWs on MoS2 was also demonstrated by VLS growth technique.156 With optimized growth parameters, high-quality high-density high-vertical-yield InP NWs were obtained, which exhibited strong linear and nonlinear optical responses.
Another type of NW/2D-material heterodimensional structure is formed by simply contacting 2D materials with as-grown vertical NW array or lateral NWs separated from the substrate. In this case, the NW/2D-material heterointerface characteristics play a dominate role in the structure properties. It has been demonstrated from the theory and experiment that Schottky junction could be formed when contacting graphene with some n-type semiconductors, which is particularly promising for high-efficiency solar cells and ultrafast self-powered photodetectors due to the high optical transparency, electrical conductivity, and surface area of graphene.157 The formation of NW/graphene Schottky junction was firstly demonstrated in II–VI and IV semiconductors. Ye et al. reported a single CdS NW Schottky junction with Au/Graphene Schottky electrodes.158 In their experiment, both the n-CdS NWs and the graphene were synthesized via the CVD method. The graphene was transferred onto a NW covered by 5 nm Au layer in between two In/Au ohmic contact electrodes on a Si/SiO2 substrate. The structure exhibited excellent photovoltaic behavior due to the high-performance Schottky combined electrode with low series resistance, high transparency, and good contact to the NW. Fan et al. demonstrated the first graphene/NW-array Schottky junction by directly coating graphene films on an n-type Si NW array, as shown in Fig. 4B.151 Si NW arrays were fabricated with top–down metal-assisted etching method, and graphene films were synthesized by a CVD method. Graphene was then transferred directly to the top of the Si NW array to form a junction. Thanks to the unique structure of NW arrays, the junction shows enhanced light trapping and faster carrier transport compared to the graphene/planar Si structure. Graphene/GaAs NW Schottky junctions are particularly promising for near-infrared photodetectors and high-efficiency solar cells. To date, both GaAs NW arrays and single GaAs NWs have been contacted with graphene to form Schottky junctions.159,160 The n-doped GaAs NWs are grown by Au-catalyzed MOCVD method. To achieve close contact between graphene and GaAs NW array top, NWs of equal height were obtained by optimizing the growth conditions, and the Au droplets were removed by wet etching before contact, to guarantee a flat top surface.159 Relatively low Schottky barrier heights of 0.58 eV and 0.36 eV were obtained from the NW array and single NW junctions, respectively, probably attributed to the rich surface states and defects at the GaAs NW/graphene interfaces, as well as the shift of the graphene Fermi level induced by the charge carrier transfer. Nevertheless, both the two types of Schottky junctions exhibited excellent photoelectric conversion characteristics.
In the graphene/NW heterostructures, the Schottky barrier height plays a dominate role in the performance of devices. A low barrier height leads to a large dark current, small on/off ratio, low responsivity, and small open-circuit voltage. Different methods have been developed to enhance the Schottky barrier height of graphene/NW heterostructures. Both theoretical and experimental results have demonstrated that the Fermi energy shift of graphene can be achieved by applying gate voltage, making it possible to modulate the Schottky barrier height of graphene/NW structures.161–163 Liu et al. reported the modulation of the transport properties of graphene/ZnO NW Schottky diode by applying gate voltage.164 They found that the barrier height increased quickly with applying gate voltage from 0 to −80 V, while decreased slowly with applying the gate voltage from 0 to 80 V. By applying a small gate voltage from 0 to −0.06 V, the Schottky barrier height of graphene/GaAs NW also slightly increased from 0.244 to 0.251 eV.165 It could be concluded that to achieve effective Schottky barrier height modulation, a sufficient high gate voltage should be applied. Chemical doping of graphene is another promising way to modulate the Schottky barrier height of graphene/NW heterostructures by shifting the Fermi energy of graphene. Shi et al. demonstrated that by AuCl3 doping, the work function of graphene could be up-shifted within the range of 0.5 eV.166 Luo et al. reported a graphene/GaAs NW Schottky junction, whose Schottky barrier height was increased from 0.29 to 0.35 eV after treated with nitric acid vapor, resulting in an enhanced built-in electric field for effective carrier segregation.167
3. NW heterodimensional devices
3.1. NW heterodimensional lasers
Semiconductor NWs are considered as one of the most promising candidates for next-generation lasers due to the ultra-small size, natural cavity structure, high end facet reflectivity, and strong light confinement. Since the first demonstration of lasing from ZnO NWs by Huang et al., NW lasers have been realized in a wide variety of materials, such as GaN, CdS, GaAs, InP, InGaAs, and InAs.168–174 However, the current homogeneous NW lasers typically exhibit a rather high threshold of tens to hundreds kW cm−2 due to the strong self-absorption energy loss. In addition, the emission wavelength of homogeneous NW lasers is limited by the material band gap. By reviewing the evolution history of semiconductor lasers, it could be naturally thought that the introduction of low dimensional quantum structures, e.g. QWs and QDs, is the guaranteed path to achieving high-performance NW lasers. Experiments have demonstrated that the introduction of quantum structures significantly improves the performance of NW lasers, characterized by a lower threshold and high temperature stability. Saxena et al. reported an AlGaAs/GaAs core–multishell NW/MQW laser grown by Au-catalyzed MOCVD, which exhibits a threshold fluence two times lower than that previously reported for room-temperature GaAs NW lasers.175 AlGaAs/GaAs core–multishell NW laser with a single GaAs QW was also demonstrated, which exhibits a relatively low threshold of 600 W cm−2 at 80 K, and an ultrasmall temperature dependent wavelength shift of 0.045 nm K−1.176 The pioneering work by Tatebayashi et al. was the first successful demonstration of room-temperature lasing from a single NW containing stacked QDs, as shown in Fig. 5A.177 The NW consists of a GaAs core containing 50 stacked In0.22Ga0.78As QDs along the axial direction. A low threshold of 25 μJ cm−2 per pulse was achieved at 7 K, and room-temperature lasing was achieved with a threshold of 179 μJ cm−2 per pulse. The characteristic temperature was ∼33 K, higher than the best value reported for bulk GaAs NW lasers. By placing the NW containing InGaAs QD stacks structure on a silver film, the first QD-based plasmonic laser was demonstrated.178 A high characteristic temperature of ∼300 ± 50 K was obtained, much higher than that of a bulk GaAs plasmonic NW laser. With the selective area (SA) MOCVD technique, Zhang et al. demonstrated an ultralow-threshold single-mode InGaAs/GaAs multiple quantum disk NW lasers.179 An ultralow threshold of 1.6 μJ cm−2 per pulse was achieved at 5 K with a quality factor exceeding 6400, and single-mode lasing was obtained with a threshold of 48 μJ cm−2 per pulse at room temperature with a high characteristic temperature of 223 K and power output of ∼0.9 μW.
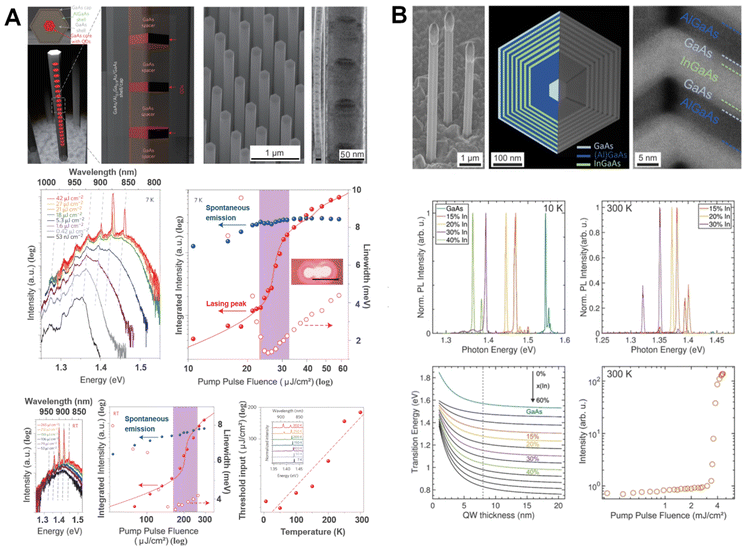 |
| Fig. 5 NW heterodimensional lasers. (A) Schematic illustrations, SEM image, STEM images, low-temperature and room-temperature lasing characteristics of GaAs/Al0.1Ga0.9As/GaAs NWs with stacked In0.22Ga0.78As QDs. Reproduced with permission.177 Copyright 2015, Nature Publishing Group. (B) SEM, cross-sectional HAADF STEM, high-resolution STEM images and lasing characteristics of GaAs/(InGaAs/AlGaAs) core/shell NWs with InGaAs MQWs. Reproduced with permission.180 Copyright 2018, American Chemical Society. | |
By adjusting the composition or thickness (size) of embeded quantum structures, the emission wavelength of NW lasers could be tuned for different applications. Qian et al. reported the first core–multishell NW/MQW laser based on III-nitride materials.24 By changing the In composition of InxGa1−xN QWs, the lasing wavelength can be tuned in a broad range of 365–494 nm. Lasing wavelengths tunable from ∼0.8 to ∼1.1 μm were obtained from GaAs/(InGaAs/AlGaAs) core/shell NWs with InGaAs MQWs by controling the InGaAs composition in the active region, as shown in as shown in Fig. 5B.180 Fonseka et al. reported a telecom-band laser based on InP/InxGa1−xAs core–multishell NW/QW structure.89 The tunability of lasing wavelength in the 1.3–1.55 μm wavelength range was achieved by varying the QW thickness and in the 1.3 μm range by varying the QW composition. By introducing QWs, NW lasers with Si-transparent emisson wavelength have been demonstrated on Si substrate, paving the way for low-consumption on-chip optical communications and interconnects. Schuster et al. firstly demostrated the Si-transparent-wavelength NW lasers on Si substrate based on InP/InGaAs nanopillar MQW structures grown by catalyst-free MOCVD technique.181 Different from the widely-used VLS and high-temperature SAE methods, this unique technique allows low temperature catalyst-free growth of NWs on a large-lattice-mismatched substrate mechanically roughened, which is compatible with the standard CMOS tool process flows.182 By adjusting the MQW width and the ternary InxGa1−xAs composition, a single lasing mode centered at 1.21 μm was obtained at 4 K, with a threshold of 36 kW cm−2. In addition, based on the Si-based InP/InGaAs nanopillar MQW structure, continuous wave lasing at a Si-transparent wavelength was observed for the first time.183
One key issue to achieve high performance NW heterodimensional lasers is the high controllability of the growth of high-quality uniform-dimension quantum structures. This is still challenging in some cases. Due to the difference in the polarity of some III–V NW sidewalls, the radial growth rate on different facets (e.g. {112}A and {112}B) is different, resulting in non-uniform QW thickness or uneven distribution of QDs.184 Compared with QD-in-NW structures, the QD-on-NW structures are more promising in nanolasers due to the high density of QDs which can provide much higher gain.185 However, lasers based on QD-on-NW structures have not been reported yet, probably due to the high non-uniformity of QD size originating from the self-assembled SK growth mode as well as the adatom diffusion mechanism. Another problem is the large variation between bottom-up NWs, leading to differences in yield and performance between individual NWs.186,187
3.2 NW heterodimensional SPSs
SPS, which generates single photons on demand, is one of the most important devices for quantum information technologies.188 Among the various types of SPSs, QD has attracted particular attention due to the excellent atom-like emission properties, easy integration, and electrically pumped possibility. To obtain ideal SPSs with high emission directionality and high extraction efficiency, QDs typically need to be integrated with microcavities, such as distributed Bragg reflector (DBR) pillar microcavity, elliptical Bragg grating (EBG) cavity, and photonic crystal cavity.189–191 These conventional top–down etching approaches limit the light-extraction efficiencies by fabrication imperfections as well as etching-induced defects. The bottom-up NWs are natural optical cavities with the advantages of high crystal quality, atomically smooth surface, and high controllability of cavity shape and dimension, allowing for the realization of single, on-axis QD emiters with a broadband spontaneous emission control and high light-extraction efficiency. Sharp exciton emission from single QDs in NWs was firstly demonstrated by Panev et al.192 The structure was built by inserting a thin InAs or possibly InGaAs slice into the GaAs NW along the growth direction. Exciton emission with a full width at half maximum of ∼200 μeV was observed, which is as narrow as those for high-quality SK QDs. By embeding a 15 nm QD segment in the indirect band gap GaP NW with the Au-catalyzed MOCVD technique, Borgström et al. demonstrated strong photon antibunching and single photon emission from the NW/QD structure for the first time, at a low temperature of 5.2 K.193 After that, NW/QD SPSs have been reported in different materials covering the emission wavelength from ultraviolet to near infrared.112,113,194–197 The purity is one of the most important performance indicators of SPSs, which can be characterized by the second-order intensity correlation function g2(0) measured using a Hanbury Brown and Twiss set-up. A light source with no more than one photon results in g2(0) = 0 whereas a laser gives g2(0) = 1. Dalacu et al. reported ultra-clean emission from InAsP QDs in InP NWs grown by combing SAE and VLS epitaxy.196 Due to the absence of nearby traps in defect-free NWs, an ultra-low g2(0) < 0.005 and ultra-narrow line width of 30 μeV were obtained, which were not possible in previous structures with stacking faults. High-efficiency coupling of the NW/QD emission into a single-mode optical fiber was also demonstrated by Bulgarini et al.198 In their experiment, a single InAsP QD was embeded into a bottom-up grown InP NW with an ultrasmooth tapered tip. At a small diameter, the NW supports only the fundamental waveguide mode, characterized by a Gaussian profile, which was maintained in the far field due to the tapering toward the NW tip. The emission was coupled into a single-mode optical fiber with a record efficiency of 93%, which was a big step towards the practical implementation of SPSs.
Room-temperature operation and electrical pumping are key prerequisites for a practical use of SPSs. For earlier reported NW/QD SPSs, the single photon emission was limited to a temperature of ∼220 K. Room temperature single photon emission from NW/QD structures was firstly demonstrated by Bounouar et al.199 In their experiment, a CdSe slice was inserted in a ZnSe NW by Au-catalyzed MBE technique. Photon antibunching was observed up to 300 K, with a second order correlation function of the peak at zero time delay g2(0) = 0.48 and high repetition rate potentially lager than 1 GHz. Holmes et al. demonstrated a room temperature single photon emission from a sit-controlled AlGaN/GaN NW/QD structure, with a low g2(0) of 0.13, as shown in Fig. 6A.200 Up to date, electrically driven NW/QD SPSs have already been demonstrated in III-nitrides, probably due to their advantages of large bandgap offsets, improved zero dimensional confinement of carriers, as well as low surface recombination velocity. Pioneering work by Deshpande et al. was the first demonstration of electrically driven NW/QD SPS, as presented in as shown in Fig. 6B.201 The InGaN/GaN QD-in-NW structure was grown on Si substrate by catalyst-free PA-MBE. A single In0.25Ga0.75N QD was grown on top of Si-doped GaN (n-GaN) surrounded by undoped GaN barriers, and Mg-doped GaN (p-GaN) was then grown on top to form a p–n junction. Single photon emission in the blue spectral range was achieved by the recombination of injected electrons and holes in the In0.25Ga0.75N QD. At an injection current density of ∼142 A cm−2, g2(0) was calculated to be 0.3 at 10 K. In addition, the single photons were found to be linearly polarized along the c axis of the NW with a degree of ∼70%. Later, based on the InGaN/GaN QD-in-NW structure, the same group extended the single emission wavelength to the green spectral range (λ ∼ 520 nm), and raised the operating temperature up to 150 K.202 Nevertheless, the development of electrically driven NW/QD SPSs is still in very preliminary status. Electrically driven NW/QD SPSs have not been demonstrated in non-nitride materials, and even for nitride materials, room-temperature operation is still a big challenge. Some fundamental issues, such as effective doping and good electrode contact, need to be further studied.
 |
| Fig. 6 NW/QD SPSs. (A) SEM, TEM, schematic, temperature-dependent photoluminescence, and temperature-dependent coincidence counts histograms of a sit-controlled AlGaN/GaN NW/QD SPS. Reproduced with permission.200 Copyright 2014, American Chemical Society. (B) Schematic, SEM, TEM, energy-dispersive X-ray (EDS) spectroscopy, electroluminescence, and electrically injected single-photon emission of a Si-based InGaN/GaN QD-in-NW SPS. Reproduced with permission.201 Copyright 2013, Nature Publishing Group. | |
3.3 NW heterodimensional LEDs
NWs are promising candidates for nanoLEDs due to their ultrasmall footprint and excellent optoelectronic properties. It is particularly worth mentioning that NW LEDs have already reached commercialisation levels by e.g. glo AB (now part of Nanosys). However, LEDs based on simple homogeneous of heterogeneous NWs were single color sources without efficient confinement of injected carriers.203–205 Studies have suggested that the introduction of QWs can significantly enhance the emission efficiency by confining injected carriers in the recombination region. In addition, by adjusting the composition and thickness of quantum structures, the emission wavelength could be tuned to realize multicolor LEDs. To date, LEDs based on NW/QW and NW/QD heterodimensional structures have been widely reported in III-nitride materials.206–212 F. Qian reported multicolor, high-efficiency LEDs based on n-GaN/InxGa1−xN QW/GaN/p-AlGaN/p-GaN core–multishell heterostructures grown by MOCVD, as shown in Fig. 7A.206 This structure was demonstrated to exhibit improved emission properties, including narrower peaks and enhanced quantum efficiencies, compared with homogeneous NWs. By changing the In composition from 1 to 40%, tunable emission from 365 to 600 nm was achieved. Ultraviolet emission was achieved from nonpolar AlGaN core–shell NW/MQW LED by MOCVD.207 At room temperature, the device exhibited high luminescence efficiency ∼74% in the ultraviolet wavelength due to the dramatically reduced dislocation density, suppressed polarization field, and improved carrier injection efficiency. Nguyen et al. demonstrated phosphor-free white LEDs based on the GaN/InGaN QD-in-NW structure grown on Si substrate.208 The active region contained 10 vertically stacked InGaN/GaN QDs. Remarkably stable white light emission was observed, without efficiency degradation for injection current density up to ∼2200 A cm−2. The stable white light emission was attributed to the large inhomogeneous broadening of QDs, uniform carrier distribution in the active region, and the reduced quantum-confined Stark effect (QCSE). NW-based near-infrared LEDs have been demonstrated by introducing InGaAs QWs into the III–V compound NWs such as InP and GaAs.213–215 Herranz et al. demonstrated infrared light generation from coaxial GaAs/(In,Ga)As dot-in-a-well NW heterostructures grown on Si substrate, as shown in Fig. 7B.213 Room temperature emission was achieved at 1.27 μm in the telecommunication O band. Yang et al. reported a multiwavelength near-infrared InGaAs/InP NW/QW LED grown by SA-MOCVD technique.214 It was observed that the QW embedded in the NW consisted of three components with different chemical compositions, leading to the electroluminescence emission with multiple wavelengths. To further extend the emission wavelength to mid-infrared, narrow-bandgap materials such as antimonide needs to be introduced. By embedding type II InAsSb/InAs MQWs within InAs NWs, mid-infrared photoluminescence at 2.83 μm has already been observed room temperature, demonstrating the possibility of mid-infrared LEDs based on NW/QW heterodimentional structures.216
 |
| Fig. 7 NW/QW LEDs. (A) Schematic and corresponding energy band diagram, STEM, EDS, and electroluminescence properties of a single n-GaN/InxGa1−xN QW/GaN/p-AlGaN/p-GaN core–multishell heterostructure. Reproduced with permission.206 Copyright 2005, American Chemical Society. (B) Schematic, SEM image, and room-temperature current–voltage (I–V) characteristics and electroluminescence of a coaxial GaAs/(In,Ga)As dot-in-a-well NW array. Reproduced with permission.213 Copyright 2020, American Chemical Society. | |
3.4 NW heterodimensional photodetectors and photovoltaics
Due to the large surface-to-volume ratio and small size of NWs, NW photodetectors can yield much higher photoresponse than their bulk counterparts.217 In addition to the high responsivity, small dark current, fast speed, low consumption, and large detection wavelength range are also important performance indicators for an ideal photodetector, which are constrained by structure limitations of homogeneous NWs. By introducing low-dimensional structures into the NW, the overall performance of NW photodetectors have been improved, and the potential applications have been significantly expanded. Rigutti et al. reported an ultraviolet photodetector based on GaN/AlN quantum disks in a single NW.218 It was found that the insertion of axial quantum disks drastically reduced the dark current as the band tilting across the quantum disks region created an additional barrier. The responsivity was as high as 2 × 103 A W−1 at λ = 300 nm at room temperature, and the ratio between the photocurrent and the dark current was up to 5 × 102. Erhard et al. demonstrated ultrafast photodetection in a core–shell AlGaAs/GaAs NW/QW structure.219 The NWs were grown on a Si(111) substrate by molecular beam epitaxy (MBE), and each NW consisted of a GaAs core surrounded by an Al0.3Ga0.7As shell, followed by a 2 nm thick GaAs QW layer and Al0.3Ga0.7As shell. A capping layer 10 nm GaAs was deposited outside the Al0.3Ga0.7As shell to prevent oxidation. The QW states exhibited a picosecond optoelectronic response at low temperature, which was attributed to the lateral photo-Dember effect. The effect originates from the different diffusivity of electrons and holes in III–V semiconductor materials, and the diffusivities are enhanced in low-dimensional structures, such as QWs. The low-dimensional structures have also been demonstrated to extend the detection wavelength of NW photodetectors via interband or intersubband transition. Kuyanov et al. compared the performance of GaP NW p–i–n photodetectors without and with GaAs QDs along the NW.220 The device containing QDs showed absorption beyond the bandgap of GaP in comparison to the device without QDs, which was attributed to the interband absorption of QDs. Similar results have been obtained in InP/InAsP NW/QD photodetectors.221 By introducing 20 InAs0.40P0.60 quantum discs into the InP NW, a broad photoresponse range from about 0.70 eV to 2.5 eV was obtained due to the interband transition between the electron and hole ground states in the quantum disks.
Karimi et al. reported the first intersubband NW array photodetectors based on InP/InAsP NW/QD heterostructures, as shown in Fig. 8A.222 The NW photodetectors consisted of InP NWs grown by MOCVD on InP substrates, with 20 InAsP quantum discs grown axially within the NW. The photodetector exhibited a broadband photoresponse from the visible to long-wavelength infrared, and the infrared response from 3–20 μm was attributed to intersubband transitions in low-bandgap InAsP quantum discs.
 |
| Fig. 8 NW heterodimensional photodetectors. (A) SEM, STEM, and EDS images of InP/InAsP QD-in-NW heterostructures, and schematic and spectrally-resolved intersubband photocurrent of the photodetector. Reproduced with permission.222 Copyright 2018, American Chemical Society. (B) Schematic, SEM image, I–V characteristics, and response time of the graphene/GaAs NW self-powered photodetector. Reproduced with permission.223 Copyright 2016, American Institute of Physics. | |
NW heterodimensional structures have also shown great potential in avalanche photodiodes (APDs), which provide high internal gain and have the ability to detect single photons. Chuang et al. reported a core–shell GaAs/InGaAs nanoneedle/QW APD monolithically integrated on a Si dubstrate.224 The current gain was 29 at a very low voltage of −2 V, and further increased to ∼263 at −8 V bias, which were very large in comparison with the current planar Ge/Si APD and InGaAs/Si APD. Avalanche amplification of a single exciton was demonstrated in an InP/InAsP QD-in-NW photodiode.225 InP NWs p–n junction containing single InAsP QDs were grown using a Au-catalyzed MOCVD method. A single photon incident on the device with a energy equal to the QD band gap was absorbed and created a single exciton. The electron and hole separated and tunnelled into the NW depletion region, and then accelerated under reverse bias. Once the carriers got enough energy, additional electron–hole pairs were created by impact ionization, resulting in multiplication of photocurrent. A large multiplication gain of 1.3 × 104 was obtained, and a single exciton was detected using a low excitation rate.
Self-powered photodetectors have wide application prospect in distributing sensor networks, internet of things, and self-powered imaging. NW/graphene Schottky junctions have shown great potential in high-responsivity ultra-fast self-powered photodetectors due to the optical transparency and high electron mobility of graphene. Pioneering work were done on the fabrication of CdSe nanobelt/graphene Schottky junctions, opening the way for the development of self-powered photodetectors based on nanostructure/graphene structures.226,227 A monolayer graphene/GaAs NW self-powered photodetector was demonstrated by Wu et al., as shown in Fig. 8B.223 The device was fabricated by transferring a monolayer graphene onto an n-doped GaAs NW array grown by Au-catalyzed MOCVD. Due to the different work functions of graphene and GaAs, a Schottky junction was formed between them, with an ideality factor of 6.18 and the Schottky barrier height of 0.58 eV at room temperature. Under zero bias and 532 nm laser illumination, the device exhibited a remarkable responsivity of 1.54 mA W−1 and short response/recover time of 71/194 μs, which could be attributed to the strong built-in electric field at the graphene/GaAs NW interface that promotes the separation of carriers. Self-powered fast-responsive ultraviolet photodetectors have been demonstrated based on graphene/ZnO NW array, reduced graphene oxide (r-GO)/GaN NW array, and (Al,Ga)N NW array/graphene/polyvinylidene fluoride (PVDF) structures.228–231 Self-powered near infrared photodetectors were demonstrated by transferring the graphene onto a Si NW array which was fabricated by Ag metal-assisted electroless chemical etching (MACE) technique.232
NW/graphene heterodimensional structures have also shown great potential in solar cells. NWs are considered as one of the most promising photovoltaics structure due to their strong light trapping effect and more appealing, the potential of exceeding the Shockley–Queisser limit.16,233–236 Benefiting from the large absorption cross section, a high short-circuit current of 180 mA cm−2 has been obtained from a single standing NW solar cell at 1 sun illumination, more than one order of magnitude higher than that predicted from the Lambert–Beer law.234 Mann et al. argued that the increase in absorption cross section could not help break the Shockley–Queisser limit. Alternatively, the absorption directivity of NWs provides open-circuit voltage enhancements and larger overall efficiencies.236 It is known that the open-circuit voltage in a solar cell is dominated by the equilibrium recombination rate (R0) and the generation rate of carriers (G). The asymmetric shape of NWs leads to anisotropy in absorptance, which reduces R0 while maintains G. It should also be noted that the homogeneity of NW properties plays an important role in the whole NW array solar cell performance. For example, Mikulik et al. demonstrated the inhomogeneity in the electrical properties of individual GaAs NWs in a NW array lead to a lowered device performance.237 Up to date, the highest reported conversion efficiencies for bottom-up grown NWs and top–down fabricated NWs are 15.3% and 17.8%, respectively.238,239 Recently, axially defined GaInP/InP/InAsP triple-junction NW solar cell was demonstrated, which exhibited a large open-circuit voltage of up to 2.37 V.240 Most of the reported NW solar cells are based on the p(i)n junctions which have been widely used in planar solar cells. However, high-quality p(i)n junctions are difficult to fabricate in NWs due to the non-uniform distribution of doping concentration along both the axial and radial directions, unintentional doping in intrinsic regions, as well as the memory effect of catalyst that leads to an unsharp junction interface.241–244 Compared with p(i)n junctions, Schottky juncions reduce the requirements of doping level and fabrication process, opening up a simple and effective way for NW solar cells. Fan et al. demonstrated a Schottky junction solar cell by directly coating graphene films on n-type Si NW arrays, which showed a conversion efficiencies of up to 2.86% at AM 1.5 condition.151 Schottky junctions solar cells based on graphene/ZnO NW array and graphene/CdS nanobelt have also been demonstrated, with relatively low efficiency <5%.158,245 The low efficiencies are attributed to the mismatch between the incident solar spectrum and materials band gap. Based on a single GaAs NW/graphene Schottky junction, Luo et al. demonstrated a high efficiency solar cell with small dimension, as shown in Fig. 9A.160 The Schottky junction was fabricated by covering a single layer graphene onto an n-doped GaAs NW. Under AM 1.5 G solar illumination, the device exhibited a remarkable conversion efficiency of 8.8%. By further improving the Schottky barrier height through chemical doping of graphene, the efficiency of three samples has been promoted from 8.00%, 5.51%, and 8.95% to 11.10%, 10.66%, and 15.88%, respectively, as shown in Fig. 9B.165 The optimal efficiency (15.88%) is close to the best value obtained in NW-based solar cells.
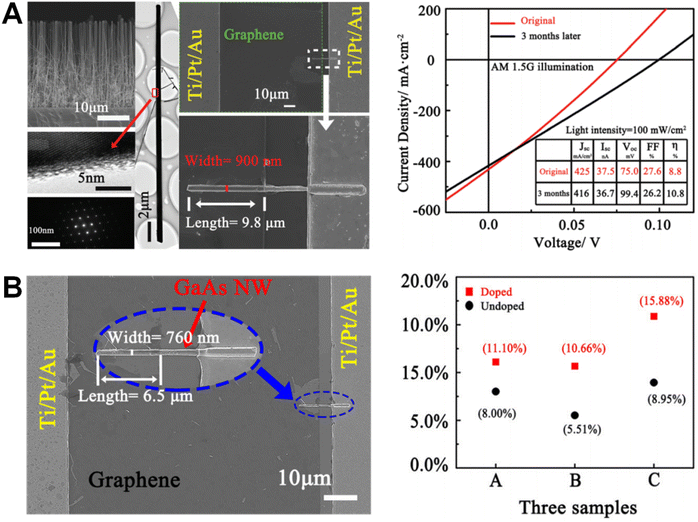 |
| Fig. 9 GaAs NW/graphene Schottky junction solar cells. (A) SEM, STEM, HRTEM, and FFT images of GaAs NWs, and SEM images and photovoltaic performance of GaAs NW/graphene solar cells. Reproduced with permission.160 Copyright 2018, Royal Society of Chemistry. (B) SEM image of the GaAs NW/graphene solar cell after nitric acid treatment, and the efficiency comparison before and after doping of graphene. Reproduced with permission.165 Copyright 2019, American Institute of Physics. | |
4. Conclusions
In this paper, we reviewed the recent progress on the fabrication, characterization and optoelectronic applications of NW heterodimensional structures. Representative heterodimensional structures, including NW/QW, NW/QD, and NW/2D-materials are studied in detail. Owing to the unique properties of quantum structures such as quantized discrete energy levels, strong carrier confinement, and dimension-dependent band gap, NWs embedded with QWs and QDs show great potential in low-threshold high-temperature-stability wavelength-tunable nanolasers, high-efficiency SPSs and LEDs, as well as high-speed intersubband-transition photodetectors. The high optical transparency, electrical conductivity, and flexibility of graphene make the NW/graphene structure particularly promising in self-powered ultra-fast photodetectors and high-efficiency flexible solar cells.
Despite the progress, considerable challenges are emerging in the development of NW heterodimensional structures. For example, the precisely growth of NW-based quantum structures, particularly self-organized QDs, is still challenging due to the specific growth mechanism and surface properties of NWs. The non-ideal NW/graphene interface which typically contains high-density surface states and defects severely degrades the Schottky barrier height and carrier transfer. Most of the reported lasers and SPSs based on NW heterodimensional structures are optically pumped, while electrically-driven light sources for practical optical communications and quantum information have been rarely reported, due to the difficulty in effective doping and good electrode contact. Although the fabrication process of NW/graphene Schottky junction photoelectric conversion devices is simple and flexible, the performance still needs to be improved. We believe that with further exploration of the fundamental physics and processing technologies, NW heterodimensional structures will serve as one of the most promising building blocks for future advanced optoelectronic devices.
Author contributions
X. Yan: methodology and writing – original draft. X. Yan and Y. Li: writing – review and editing. X. Zhang: supervision of the entire project.
Data availability
No primary research results, software or code have been included and no new data were generated or analysed as part of this review.
Conflicts of interest
There are no conflicts to declare.
Acknowledgements
This work was funded by the National Natural Science Foundation of China (62474022 and 61935003), and the State Key Laboratory of Information Photonics and Optical Communications (Beijing University of Posts and Telecommunications), P. R. China (IPOC2022ZT02).
Notes and references
- R. S. Wagner and W. C. Ellis, Appl. Phys. Lett., 1964, 4, 89–90 CrossRef CAS
.
- M. Yazawa, M. Koguchi and K. Hiruma, Appl. Phys. Lett., 1991, 58, 1080–1082 CrossRef CAS
.
- K. Hiruma, T. Katsuyama, K. Ogawa, M. Koguchi, H. Kakibayashi and G. P. Morgan, Appl. Phys. Lett., 1991, 59, 431–433 CrossRef CAS
.
- B. S. Kim, T. W. Koo, J. H. Lee, D. S. Kim, Y. C. Jung, S. W. Hwang, B. L. Choi, E. K. Lee, J. M. Kim and D. Whang, Nano Lett., 2009, 9, 864–869 CrossRef CAS PubMed
.
- V. G. Dubrovskii, W. Kim, V. Piazza, L. Güniat and A. F. Morral, Nano Lett., 2021, 21, 3139–3145 CrossRef CAS PubMed
.
- G. Cheng, C. Miao, Q. Qin, J. Li, F. Xu, H. Haftbaradaran, E. C. Dickey, H. Gao and Y. Zhu, Nat. Nanotechnol., 2015, 10, 687–691 CrossRef CAS PubMed
.
- X. Yuan, D. Pan, Y. Zhou, X. Zhang, K. Peng, B. Zhao, M. Deng, J. He, H. H. Tan and C. Jagadish, Appl. Phys. Rev., 2021, 8, 021302 CAS
.
- A. M. Munshi, D. L. Dheeraj, V. T. Fauske, D. C. Kim, J. Huh, J. F. Reinertsen, L. Ahtapodov, K. D. Lee, B. Heidari, A. T. J. van Helvoort, B. O. Fimland and H. Weman, Nano Lett., 2014, 14, 960–966 CrossRef CAS PubMed
.
- G. N. Parsons and R. D. Clark, Chem. Mater., 2020, 32, 4920–4953 CrossRef CAS
.
- C. Jia, Z. Lin, Y. Huang and X. Duan, Chem. Rev., 2019, 119, 9074–9135 CrossRef CAS PubMed
.
- S. W. Eaton, A. Fu, A. B. Wong, C. Z. Ning and P. Yang, Nat. Rev. Mater., 2016, 1, 16028 CrossRef CAS
.
- K. Behrman and I. Kymissis, Nat. Electron., 2022, 5, 564–573 CrossRef
.
- Z. Li, Z. He, C. Xi, F. Zhang, L. Huang, Y. Yu, H. Hoe Tan, C. Jagadish and L. Fu, Adv. Mater. Technol., 2023, 8, 2202126 CrossRef CAS
.
- I. Aharonovich, D. Englund and M. Toth, Nat. Photonics, 2016, 10, 631–641 CrossRef CAS
.
- S. J. Gibson, B. van Kasteren, B. Tekcan, Y. Cui, D. van Dam, J. E. M. Haverkort, E. P. A. M. Bakkers and M. E. Reimer, Nat. Nanotechnol., 2019, 14, 473–479 CrossRef CAS PubMed
.
- G. Otnes and M. T. Borgström, Nano Today, 2017, 12, 31–45 CrossRef CAS
.
- Y. Zhang, D. Saxena, M. Aagesen and H. Liu, Nanotechnology, 2019, 30, 192002 CrossRef CAS
.
- X. Duan, Y. Huang, R. Agarwal and C. M. Lieber, Nature, 2003, 421, 241–245 CrossRef CAS PubMed
.
- S. Chu, G. Wang, W. Zhou, Y. Lin, L. Chernyak, J. Zhao, J. Kong, L. Li, J. Ren and J. Liu, Nat. Nanotechnol., 2011, 6, 506–510 CrossRef CAS
.
- K. H. Li, X. Liu, Q. Wang, S. Zhao and Z. Mi, Nat. Nanotechnol., 2015, 10, 140–144 CrossRef CAS PubMed
.
- G. Zhang, K. Tateno, H. Sanada, T. Tawara, H. Gotoh and H. Nakano, Appl. Phys. Lett., 2009, 95, 123104 CrossRef
.
- M. S. Gudiksen, J. Wang and C. M. Lieber, J. Phys. Chem. B, 2002, 106, 4036–4039 CrossRef CAS
.
- E. Halpern, A. Henning, H. Shtrikman, R. Rurali, X. Cartoixa and Y. Rosenwaks, Nano Lett., 2015, 15, 481–485 CrossRef CAS
.
- F. Qian, Y. Li, S. Gradečak, H. G. Park, Y. Dong, Y. Ding, Z. L. Wang and C. M. Lieber, Nat. Mater., 2008, 7, 701–706 CrossRef CAS PubMed
.
- M. Karimi, M. Heurlin, S. Limpert, V. Jain, X. Zeng, I. Geijselaers, A. Nowzari, Y. Fu, L. Samuelson, H. Linke, M. T. Borgström and H. Pettersson, Nano Lett., 2018, 18, 365–372 CrossRef CAS PubMed
.
- I. Aharonovich, D. Englund and M. Toth, Nat. Photonics, 2016, 10, 631–641 CrossRef CAS
.
- F. Xia, H. Wang, D. Xiao, M. Dubey and A. Ramasubramaniam, Nat. Photonics, 2014, 8, 899–907 CrossRef CAS
.
- P. K. Mohseni, A. Behnam, J. D. Wood, X. Zhao, K. J. Yu, N. C. Wang, A. Rockett, J. A. Rogers, J. W. Lyding, E. Pop and X. Li, Adv. Mater., 2014, 26, 3755–3760 CrossRef CAS PubMed
.
- X. W. Fu, Z. M. Liao, Y. B. Zhou, H. C. Wu, Y. Q. Bie, J. Xu and D. P. Yu, Appl. Phys. Lett., 2012, 100, 223114 CrossRef
.
- F. Glas, Phys. Rev. B: Condens. Matter Mater. Phys., 2006, 74, 121302(R) CrossRef
.
- F. Furtmayr, J. Teubert, P. Becker, S. Conesa-Boj, J. R. Morante, A. Chernikov, S. Schäfer, S. Chatterjee, J. Arbiol and M. Eickhoff, Phys. Rev. B: Condens. Matter Mater. Phys., 2011, 84, 205303 CrossRef
.
- M. Wölz, V. M. Kaganer, O. Brandt and L. Geelhaar, Appl. Phys. Lett., 2011, 98, 261907 CrossRef
.
- J. Lahnemann, O. Brandt, C. Pfüller, T. Flissikowski, U. Jahn, E. Luna, M. Hanke, M. Knelangen, A. Trampert and H. T. Grahn, Phys. Rev. B: Condens. Matter Mater. Phys., 2011, 84, 155303 CrossRef
.
- M. T. Björk, B. J. Ohlsson, T. Sass, A. I. Persson, C. Thelander, M. H. Magnusson, K. Deppert, L. R. Wallenberg and L. Samuelson, Appl. Phys. Lett., 2002, 80, 1058–1060 CrossRef
.
- L. Rigutti, J. Teubert, G. Jacopin, F. Fortuna, M. Tchernycheva, A. De Luna Bugallo, F. H. Julien, F. Furtmayr, M. Stutzmann and M. Eickhoff, Phys. Rev. B: Condens. Matter Mater. Phys., 2010, 82, 235308 CrossRef
.
- L. F. Zagonel, S. Mazzucco, M. Tencé, K. March, R. Bernard, B. Laslier, G. Jacopin, M. Tchernycheva, L. Rigutti, F. H. Julien, R. Songmuang and M. Kociak, Nano Lett., 2011, 11, 568–573 CrossRef CAS
.
- A. M. Graham, P. Corfdir, M. Heiss, S. Conesa-Boj, E. Uccelli, A. Fontcuberta i Morral and R. T. Phillips, Phys. Rev. B: Condens. Matter Mater. Phys., 2013, 87, 125304 CrossRef
.
- Y. H. Ra, R. Navamathavan, J. H. Park and C. R. Lee, ACS Appl. Mater. Interfaces, 2013, 5, 2111–2117 CrossRef CAS PubMed
.
- M. Heiß, A. Gustafsson, S. Conesa-Boj, F. Peiró, J. R. Morante, G. Abstreiter, J. Arbiol, L. Samuelson and A. Fontcuberta i Morral, Nanotechnology, 2009, 20, 075603 CrossRef
.
- O. Arif, V. Zannier, F. Rossi, D. De Matteis, K. Kress, M. De Luca, I. Zardo and L. Sorba, Nanoscale, 2023, 15, 1145–1153 CAS
.
- M. Fickenscher, T. Shi, H. E. Jackson, L. M. Smith, J. M. Yarrison-Rice, C. Zheng, P. Miller, J. Etheridge, B. M. Wong, Q. Gao, S. Deshpande, H. H. Tan and C. Jagadish, Nano Lett., 2013, 13, 1016–1022 CrossRef CAS PubMed
.
- K. A. Dick, S. Kodambaka, M. C. Reuter, K. Deppert, L. Samuelson, W. Seifert, L. R. Wallenberg and F. M. Ross, Nano Lett., 2007, 7, 1817–1822 CAS
.
- K. A. Dick, J. Bolinsson, B. M. Borg and J. Johansson, Nano Lett., 2012, 12, 3200–3206 CrossRef CAS PubMed
.
- M. E. Messing, J. Wong-Leung, Z. Zanolli, H. J. Joyce, H. H. Tan, Q. Gao, L. R. Wallenberg, J. Johansson and C. Jagadish, Nano Lett., 2011, 11, 3899–3905 CAS
.
- V. Zannier, D. Ercolani, U. P. Gomes, J. David, M. Gemmi, V. G. Dubrovskii and L. Sorba, Nano Lett., 2016, 16, 7183–7190 CAS
.
- M. Ek, B. M. Borg, J. Johansson and K. A. Dick, ACS Nano, 2013, 7, 3668–3675 CAS
.
- M. Heiß, A. Gustafsson, S. Conesa-Boj, F. Peiro, J. R. Morante, G. Abstreiter, J. Arbiol, L. Samuelson and A. F. i Morral, Nanotechnology, 2009, 20, 075603 CrossRef PubMed
.
- F. Glas, Cryst. Growth Des., 2017, 17, 4785–4794 CAS
.
- V. G. Dubrovskii, Phys. Rev. Mater., 2024, 8, 076003 CAS
.
- E. D. Leshchenko and J. Johansson, CrystEngComm, 2022, 24, 8052–8059 RSC
.
- K. A. Dick, J. Bolinsson, B. M. Borg and J. Johansson, Nano Lett., 2012, 12, 3200–3206 CrossRef CAS
.
- G. Priante, G. Patriarche, F. Oehler, F. Glas and J.-C. Harmand, Nano Lett., 2015, 15, 6036–6041 CrossRef CAS PubMed
.
- G. Priante, F. Glas, G. Patriarche, K. Pantzas, F. Oehler and J.-C. Harmand, Nano Lett., 2016, 16, 1917–1924 CrossRef CAS PubMed
.
- H. Kauko, T. Grieb, R. Bjørge, M. Schowalter, A. M. Munshi, H. Weman, A. Rosenauer and A. T. J. van Helvoort, Micron, 2013, 44, 254–260 CrossRef CAS PubMed
.
- A. D. Bolshakov, V. V. Fedorov, N. V. Sibirev, M. V. Fetisova, E. I. Moiseev, N. V. Kryzhanovskaya, O. Y. Koval, E. V. Ubyivovk, A. M. Mozharov, G. E. Cirlin and I. S. Mukhin, Phys. Status Solidi R, 2019, 13, 1900350 CrossRef CAS
.
- Y. Zhang, A. V. Velichko, H. A. Fonseka, P. Parkinson, J. A. Gott, G. Davis, M. Aagesen, A. M. Sanchez, D. Mowbray and H. Liu, Nano Lett., 2021, 21, 5722–5729 CrossRef CAS PubMed
.
- M. S. Gudiksen, L. J. Lauhon, J. Wang, D. C. Smith and C. M. Lieber, Nature, 2002, 415, 617–620 CrossRef CAS
.
- A. K. Sivan, B. Abad, T. Albrigi, O. Arif, J. Trautvetter, A. R. Caridad, C. Arya, V. Zannier, L. Sorba, R. Rurali and I. Zardo, Appl. Nano Mater., 2023, 6, 18602–18613 CrossRef
.
- J. Eymery, F. Rieutord, V. Favre-Nicolin, O. Robach, Y. M. Niquet, L. Fröberg, T. Mårtensson and L. Samuelson, Nano Lett., 2007, 7, 2596–2601 CrossRef CAS
.
- D. Li, Y. Wu, R. Fan, P. Yang and A. Majumdar, Appl. Phys. Lett., 2003, 83, 3186–3188 CrossRef CAS
.
- M. Hu and D. Poulikakos, Nano Lett., 2012, 12, 5487–5494 CrossRef CAS PubMed
.
- S. Mizuno and N. Nishiguchi, J. Phys.: Condens. Matter, 2009, 21, 195303 CrossRef
.
- P. Caroff, K. A. Dick, J. Johansson, M. E. Messing, K. Deppert and L. Samuelson, Nat. Nanotechnol., 2009, 4, 50–55 CrossRef CAS PubMed
.
- K. A. Dick, C. Thelander, L. Samuelson and P. Caroff, Nano Lett., 2010, 10, 3494–3499 CrossRef CAS
.
- J. Bolinsson, P. Caroff, B. Mandl and K. A. Dick, Nanotechnology, 2011, 22, 265606 CrossRef PubMed
.
- K. Pemasiri, M. Montazeri, R. Gass, L. M. Smith, H. E. Jackson, J. Yarrison-Rice, S. Paiman, Q. Gao, H. H. Tan, C. Jagadish, X. Zhang and J. Zou, Nano Lett., 2009, 9, 648–654 CrossRef CAS
.
- L. Zhang, J. W. Luo, A. Zunger, N. Akopian, V. Zwiller and J. C. Harmand, Nano Lett., 2010, 10, 4055–4060 CrossRef CAS PubMed
.
- C. K. Yong, J. Wong-Leung, H. J. Joyce, J. Lloyd-Hughes, Q. Gao, H. H. Tan, C. Jagadish, M. B. Johnston and L. M. Herz, Nano Lett., 2013, 13, 4280–4287 CrossRef CAS PubMed
.
- Z. Ikonic, G. P. Srivastava and J. C. Inkson, Solid State Commun., 1993, 86, 799–802 CrossRef CAS
.
- Z. Ikonić, G. P. Srivastava and J. C. Inkson, Phys. Rev. B: Condens. Matter Mater. Phys., 1993, 48, 17181 CrossRef
.
- Z. Ikonic, G. P. Srivastava and J. C. Inkson, Phys. Rev. B: Condens. Matter Mater. Phys., 1995, 52, 14078 CrossRef CAS
.
- M. D. Luca, C. Fasolato, M. A. Verheijen, Y. Ren, M. Y. Swinkels, S. Kölling, E. P. A. M. Bakkers, R. Rurali, X. Cartoixà and I. Zardo, Nano Lett., 2019, 19, 4702–4711 CrossRef PubMed
.
- R. E. Algra, M. A. Verheijen, M. T. Borgström, L. F. Feiner, G. Immink, W. J. P. van Enckevort, E. Vlieg and E. P. A. M. Bakkers, Nature, 2008, 456, 369–372 CrossRef CAS PubMed
.
- T. Burgess, S. Breuer, P. Caroff, J. Wong-Leung, Q. Gao, H. H. Tan and C. Jagadish, ACS Nano, 2013, 7, 8105–8114 CrossRef CAS
.
- N. I. Goktas, A. Sokolovskii, V. G. Dubrovskii and R. R. LaPierre, Nano Lett., 2020, 20, 3344–3351 CrossRef
.
- X. Yuan, Y. Guo, P. Caroff, J. He, H. H. Tan and C. Jagadish, Phys. Status Solidi R, 2017, 11, 1700310 CrossRef
.
- J. Johansson, L. S. Karlsson, C. Patrik, T. Svensson, T. Mårtensson, B. A. Wacaser, K. Deppert, L. Samuelson and W. Seifert, Nat. Mater., 2006, 5, 574–580 CrossRef CAS PubMed
.
- J. Johansson, K. A. Dick, P. Caroff, M. E. Messing, J. Bolinsson, K. Deppert and L. Samuelson, J. Phys. Chem. C, 2010, 114, 3837–3842 CrossRef CAS
.
- Q. Li, X. Gong, C. Wang, J. Wang, K. Ip and S. Hark, Adv. Mater., 2004, 16, 1436–1440 CrossRef CAS
.
- S. E. Steinvall, L. Ghisalberti, R. R. Zamani, N. Tappy, F. S. Hage, E. Z. Stutz, M. Zamani, R. Paul, J.-B. Leran, Q. M. Ramasse, W. C. Carter and A. F. i Morral, Nanoscale, 2020, 12, 22534–22540 RSC
.
- L. Peri, D. Prete, V. Demontis, V. Zannier, F. Rossi, L. Sorba, F. Beltram and F. Rossella, Nano Energy, 2022, 103, 107700 CrossRef CAS
.
- A. Ghukasyan and R. LaPierre, Nanoscale, 2022, 14, 6480–6487 RSC
.
- M. Xue, M. Li, Y. Huang, R. Chen, Y. Li, J. Wang, Y. Xing, J. Chen, H. Yan, H. Xu and J. Chen, Adv. Mater., 2020, 32, 2004120 CrossRef CAS
.
- M. D. Luca, C. Fasolato, M. A. Verheijen, Y. Ren, M. Y. Swinkels, S. Kölling, E. P. A. M. Bakkers, R. Rurali, X. Cartoixà and I. Zardo, Nano Lett., 2019, 19, 4702–4711 CrossRef
.
- X. Yan, S. Fan, X. Zhang and X. Ren, Nanoscale Res. Lett., 2015, 10, 389 CrossRef
.
- H. A. Fonseka, A. S. Ameruddin, P. Caroff, D. Tedeschi, M. De Luca, F. Mura, Y. Guo, M. Lysevych, F. Wang, H. H. Tan, A. Polimenic and C. Jagadish, Nanoscale, 2017, 9, 13554–13562 RSC
.
- V. Grenier, S. Finot, G. Jacopin, C. Bougerol, E. Robin, N. Mollard, B. Gayral, E. Monroy, J. Eymery and C. Durand, ACS Appl. Mater. Interfaces, 2020, 12, 44007–44016 CrossRef CAS
.
- X. Yan, X. Zhang, J. Li, Y. Wu, J. Cui and X. Ren, Nanoscale, 2015, 7, 1110–1115 RSC
.
- J. Segura-Ruiz, D. Salomon, A. Rogalev, J. Eymery, B. Alén and G. Martínez-Criado, Nano Lett., 2021, 21, 9494–9501 CrossRef CAS
.
- P. Corfdir, O. Marquardt, R. B. Lewis, C. Sinito, M. Ramsteiner, A. Trampert, U. Jahn, L. Geelhaar, O. Brandt and V. M. Fomin, Adv. Mater., 2019, 31, e1805645 CrossRef
.
- Z. Wu, H. Lei, T. Zhou, Y. Fan and Z. Zhong, Nanotechnology, 2014, 25, 055204 CrossRef CAS
.
- A. C. Meng, C. S. Fenrich, M. R. Braun, J. P. McVittie, A. F. Marshall, J. S. Harris and P. C. McIntyre, Nano Lett., 2016, 16, 7521–7529 CrossRef CAS
.
- S. Assali, A. Dijkstra, A. Li, S. Koelling, M. A. Verheijen, L. Gagliano, N. von den Driesch, D. Buca, P. M. Koenraad, J. E. M. Haverkort and E. P. A. M. Bakkers, Nano Lett., 2017, 17, 1538–1544 CrossRef CAS
.
- A. C. Meng, M. R. Braun, Y. Wang, S. Peng, W. Tan, J. Z. Lentz, M. Xue, A. Pakzad, A. F. Marshall, J. S. Harris, W. Cai and P. C. McIntyre, Mater. Today, 2020, 40, 101–113 CrossRef CAS
.
- Q. Chen, H. Zhou, S. Xu., Y.-C. Huang, S. Wu, K. H. Lee, X. Gong and C. S. Tan, ACS Nano, 2023, 17, 12151–12159 CrossRef CAS
.
- Q. Chen, Y. Jung, H. Zhou, S. Wu, X. Gong, Y.-C. Huang, K. H. Lee, L. Zhang, D. Nam, J. Liu, J.-W. Luo, W. Fan and C. S. Tan, ACS Photonics, 2023, 10, 1716–1725 CrossRef CAS
.
- H. Zeng, X. Yu, H. A. Fonseka, J. A. Gott, M. Tang, Y. Zhang, G. Boras, J. Xu, A. M. Sanchez and H. Liu, Nano Lett., 2018, 18, 6397–6403 CrossRef CAS
.
- I. Dudko, T. Dursap, A. D. Lamirand, C. Botella, P. Regreny, A. Danescu, S. Brottet, M. Bugnet, S. Walia, N. Chauvin and J. Penuelas, Cryst. Growth Des., 2022, 22, 32–36 CrossRef CAS
.
- E. M. T. Fadaly, A. Dijkstra, J. R. Suckert, D. Ziss, M. A. J. van Tilburg, C. Mao, Y. Ren, V. T. van Lange, K. Korzun, S. Kölling, M. A. Verheijen, D. Busse, C. Rödl, J. Furthmüller, F. Bechstedt, J. Stangl, J. J. Finley, S. Botti, J. E. M. Haverkort and E. P. A. M. Bakkers, Nature, 2020, 580, 205–209 CrossRef CAS
.
- S. Mauthe, Y. Baumgartner, M. Sousa, Q. Ding, M. D. Rossell, A. Schenk, L. Czornomaz and K. E. Moselund, Nat. Commun., 2020, 11, 4565 CrossRef CAS PubMed
.
- H. Schmid, M. Borg, K. Moselund, L. Gignac, C. M. Breslin, J. Bruley, D. Cutaia and H. Riel, Appl. Phys. Lett., 2015, 106, 233101 CrossRef
.
- E. Brugnolotto, H. Schmid, V. Georgiev and M. Sousa, Cryst. Growth Des., 2023, 23, 8034–8042 CrossRef CAS
.
- J. Arbiol, M. de la Mata, M. Eickhoff and A. Fontcuberta i Morral, Mater. Today, 2013, 6, 213–219 CrossRef
.
- G. Bulgarini, M. E. Reimer, T. Zehender, M. Hocevar, E. P. A. M. Bakkers, L. P. Kouwenhoven and V. Zwiller, Appl. Phys. Lett., 2012, 100, 121106 CrossRef
.
- K. Tateno, G. Zhang, H. Gotoh and T. Sogawa, Nano Lett., 2012, 12, 2888–2893 CrossRef CAS
.
- D. Dalacu, K. Mnaymneh, J. Lapointe, X. Wu, P. J. Poole, G. Bulgarini, V. Zwiller and M. E. Reimer, Nano Lett., 2012, 12, 5919–5923 CrossRef CAS
.
- J. Wu, A. Ramsay, A. Sanchez, Y. Zhang, D. Kim, F. Brossard, X. Hu, M. Benamara, M. E. Ware., Y. I. Mazur, G. J. Salamo, M. Aagesen, Z. Wang and H. Liu, Nano Lett., 2016, 16, 504–511 CrossRef CAS
.
- V. N. Kats, V. P. Kochereshko, A. V. Platonov, T. V. Chizhova, G. E. Cirlin, A. D. Bouravleuv, Y. B. Samsonenko, I. P. Soshnikov, E. V. Ubyivovk and J. Bleuse, Semicond. Sci. Technol., 2012, 27, 015009 CrossRef
.
- G. E. Cirlin, R. R. Reznik, I. V. Shtrom, A. I. Khrebtov, I. P. Soshnikov, S. A. Kukushkin, L. Leandro, T. Kasama and N. Akopian, J. Phys. D: Appl. Phys., 2017, 50, 484003 CrossRef
.
- F. Glas and B. Daudin, Phys. Rev. B: Condens. Matter Mater. Phys., 2012, 86, 174112 CrossRef
.
- G. Tourbot, C. Bougerol, F. Glas, L. F. Zagonel, Z. Mahfoud, S. Meuret, P. Gilet, M. Kociak, B. Gayral and B. Daudin, Nanotechnology, 2012, 23, 135703 CrossRef CAS
.
- J. Heinrich, A. Huggenberger, T. Heindel, S. Reitzenstein, S. Höfling, L. Worschech and A. Forchel, Appl. Phys. Lett., 2010, 96, 211117 CrossRef
.
- J. Tatebayashi, Y. Ota, S. Ishida, M. Nishioka, S. Iwamoto and Y. Arakawa, Appl. Phys. Lett., 2012, 100, 263101 CrossRef
.
- A. P. Foster, J. P. Bradley, K. Gardner, A. B. Krysa, B. Royall, M. S. Skolnick and L. R. Wilson, Nano Lett., 2015, 15, 1559–1563 CrossRef CAS
.
- K. Choi, S. Kako, M. J. Holmes, M. Arita and Y. Arakawa, Appl. Phys. Lett., 2013, 103, 171907 CrossRef
.
- M. J. Holmes, K. Choi, S. Kako, M. Arita and Y. Arakawa, Nano Lett., 2014, 14, 982–986 CrossRef CAS
.
- J. Tatebayashi, Y. Ota, S. Ishida, M. Nishioka, S. Iwamoto and Y. Arakawa, Appl. Phys. Lett., 2014, 105, 103104 CrossRef
.
- D. Dalacu, K. Mnaymneh, X. Wu, J. Lapointe, G. C. Aers, P. J. Poole and R. L. Williams, Appl. Phys. Lett., 2011, 98, 251101 CrossRef
.
- S. Haffouz, K. D. Zeuner, D. Dalacu, P. J. Poole, J. Lapointe, D. Poitras, K. Mnaymneh, X. Wu, M. Couillard, M. Korkusinski, E. Schöll, K. D. Jöns, V. Zwiller and R. L. Williams, Nano Lett., 2018, 18, 3047–3052 CrossRef CAS
.
- A. Fiset-Cyr, D. Dalacu, S. Haffouz, P. J. Poole, J. Lapointe, G. C. Aers and R. L. Williams, Appl. Phys. Lett., 2018, 113, 053105 CrossRef
.
- E. Uccelli, J. Arbiol, J. R. Morante and A. Fontcuberta i Morral, ACS Nano, 2010, 4, 5985–5993 CrossRef CAS PubMed
.
- M. Heiss, Y. Fontana, A. Gustafsson, G. Wüst, C. Magen, D. D. O’Regan, J. W. Luo, B. Ketterer, S. Conesa-Boj, A. V. Kuhlmann, J. Houel, E. Russo-Averchi, J. R. Morante, M. Cantoni, N. Marzari, J. Arbiol, A. Zunger, R. J. Warburton and A. Fontcuberta i Morral, Nat. Mater., 2013, 12, 439–444 CrossRef CAS PubMed
.
- R. Popovitz-Biro, A. Kretinin, P. Von Huth and H. Shtrikman, Cryst. Growth Des., 2011, 11, 3858–3865 CrossRef CAS
.
- X. Li and G. Yang, Nanotechnology, 2014, 25, 435605 CrossRef PubMed
.
- L. Pan, K. K. Lew, J. M. Redwing and E. C. Dickey, Nano Lett., 2005, 5, 1081–1085 CrossRef CAS PubMed
.
- X. Yan, X. Zhang, X. Ren, H. Huang, J. Guo, X. Guo, M. Liu, Q. Wang, S. Cai and Y. Huang, Nano Lett., 2011, 11, 3941–3945 CrossRef CAS PubMed
.
- X. Yan, X. Zhang, X. Ren, X. Lv, J. Li, Q. Wang, S. Cai and Y. Huang, Nano Lett., 2012, 12, 1851–1856 CrossRef CAS PubMed
.
- X. Yan, X. Zhan, X. Ren, J. Li, J. Cui, S. Wang, S. Fan, Q. Wang and Y. Huang, Appl. Phys. Lett., 2013, 103, 172102 CrossRef
.
- X. Yan, X. Zhang, J. Li, J. Cui and X. Ren, J. Appl. Phys., 2015, 117, 054301 CrossRef
.
- F. Cheng, B. Li, L. Li, X. Wang, S. Shen, W. Liu, H. Zheng, S. Jia, X. Yan, X. Zhang, J. Wang and Y. Gao, J. Phys. Chem. C, 2019, 123, 4228–4234 CrossRef CAS
.
- T. Qi, Y. Cheng, F. Cheng, L. Li, C. Li, S. Jia, X. Yan, X. Zhang, J. Wang and Y. Gao, Nanotechnology, 2020, 31, 245701 CrossRef CAS PubMed
.
- X. Yan, X. Zhang, X. Ren, J. Li, X. Lv, Q. Wang and Y. Huang, Appl. Phys. Lett., 2012, 101, 023106 CrossRef
.
- X. Yan, X. Zhang, X. Ren, J. Li, J. Cui, L. Li, S. Wang, Q. Wang and Y. Huang, J. Mater. Chem. C, 2013, 1, 7914–7919 RSC
.
- X. Yan, X. Zhang, J. Li, J. Cui and X. Ren, J. Appl. Phys., 2014, 116, 214304 CrossRef
.
- X. Yan, X. Zhang, J. Li, Y. Wu, B. Li and X. Ren, Phys. Status Solidi R, 2016, 10, 168–171 CrossRef CAS
.
- Y. Yu, M. F. Li, J. F. He, Y. M. He, Y. J. Wei, Y. He, G. W. Zha, X. J. Shang, J. Wang, L. J. Wang, G. W. Wang, H. Q. Ni, C. Y. Lu and Z. C. Niu, Nano Lett., 2013, 13, 1399–1404 CrossRef CAS PubMed
.
- Y. Yu, X. M. Dou, B. Wei, G. W. Zha, X. J. Shang, L. Wang, D. Su, J. X. Xu, H. Y. Wang, H. Q. Ni, B. Q. Sun, Y. Ji, X. D. Han and Z. C. Niu, Adv. Mater., 2014, 26, 2710–2717 CrossRef CAS
.
- X. Yan, F. Tang, Y. Wu, B. Li, X. Zhang and X. Ren, J. Cryst. Growth, 2017, 468, 185–187 CrossRef CAS
.
- M. Heiss, B. Ketterer, E. Uccelli, J. R. Morante, J. Arbiol and A. F. i Morral, Nanotechnology, 2011, 22, 195601 CrossRef PubMed
.
- Y. Zhang, H. A. Fonseka, H. Yang, X. Yu, P. Jurczak, S. Huo, A. M. Sanchez and H. Liu, Nanoscale Horiz., 2022, 7, 311–318 RSC
.
- A. M. Munshi and H. Weman, Phys. Status Solidi R, 2013, 10, 713–726 CrossRef
.
- K. Nakada and A. Ishii, Solid State Commun., 2011, 151, 13–16 CrossRef CAS
.
- A. M. Munshi, L. Dheeraj, V. T. Fauske, D. C. Kim, A. T. J. van Helvoort, B. O. Fimland and H. Weman, Nano Lett., 2012, 12, 4570–4576 CrossRef CAS PubMed
.
- Y. J. Kim, J. H. Lee and G. C. Yi, Appl. Phys. Lett., 2009, 95, 213101 CrossRef
.
- B. Kumar, K. Y. Lee, H. K. Park, S. J. Chae, Y. H. Lee and S. W. Kim, ACS Nano, 2011, 5, 4197–4204 CrossRef CAS
.
- J. P. Alper, A. Gutes, C. Carraro and R. Maboudian, Nanoscale, 2013, 5, 4114–4118 RSC
.
- Y. J. Hong, W. H. Lee, Y. Wu, R. S. Ruoff and T. Fukui, Nano Lett., 2012, 12, 1431–1436 CrossRef CAS
.
- J. H. Kang, Y. Ronen, Y. Cohen, D. Convertino, A. Rossi, C. Coletti, S. Heun, L. Sorba, P. Kacman and H. Shtrikman, Semicond. Sci. Technol., 2016, 31, 115005 CrossRef
.
- V. Kumaresan, L. Largeau, A. Madouri, F. Glas, H. Zhang, F. Oehler, A. Cavanna, A. Babichev, L. Travers, N. Gogneau, M. Tchernycheva and J. C. Harmand, Nano Lett., 2016, 16, 4895–4902 CrossRef CAS
.
- M. Morassi, N. Guan, V. G. Dubrovskii, Y. Berdnikov, C. Barbier, L. Mancini, L. Largeau, A. V. Babichev, V. Kumaresan, F. H. Julien, L. Travers, N. Gogneau, J. C. Harmand and M. Tchernycheva, Cryst. Growth Des., 2020, 20, 552–559 CrossRef CAS
.
- G. Fan, H. Zhu, K. Wang, J. Wei, X. Li, Q. Shu, N. Guo and D. Wu, ACS Appl. Mater. Interfaces, 2011, 3, 721–725 CrossRef CAS
.
- P. K. Mohseni, A. Behnam, J. D. Wood, C. D. English, J. W. Lyding, E. Pop and X. Li, Nano Lett., 2013, 13, 1153–1161 CrossRef CAS
.
- S. Nalamati, M. Sharma, P. Deshmukh, J. Kronz, R. Lavelle, D. Snyder, C. L. Reynolds, Y. Liu and S. Iyer, ACS Appl. Nano Mater., 2019, 2, 4528–4537 CrossRef CAS
.
- D. Ren, T. A. Nilsen, J. S. Nilsen, L. Ahtapodov, A. Mukherjee, Y. Li, A. T. J. van Helvoort, H. Weman and B. O. Fimland, ACS Appl. Nano Mater., 2024, 7, 6797–6803 CrossRef CAS
.
- E. A. Anyebe, A. M. Sanchez, S. Hindmarsh, X. Chen, J. Shao, M. K. Rajpalke, T. D. Veal, B. J. Robinson, O. Kolosov, F. Anderson, R. Sundaram, Z. M. Wang, V. Falko and Q. Zhuang, Nano Lett., 2015, 15, 4348–4355 CrossRef CAS PubMed
.
- A. M. Shafi, S. Das, V. Khayrudinov, E. X. Ding, M. G. Uddin, F. Ahmed, Z. Sun and H. Lipsanen, Chem. Mater., 2022, 34, 9055–9061 CrossRef CAS
.
- S. Tongay, M. Lemaitre, X. Miao, B. Gila, B. R. Appleton and A. F. Hebard, Phys. Rev. X, 2012, 2, 011002 Search PubMed
.
- Y. Ye, Y. Dai, L. Dai, Z. Shi, N. Liu, F. Wang, L. Fu, R. Peng, X. Wen, Z. Chen, Z. Liu and G. Qin, ACS Appl. Mater. Interfaces, 2010, 2, 3406–3410 CrossRef CAS
.
- Y. Wu, X. Yan, X. Zhang and X. Ren, Appl. Phys. Lett., 2016, 109, 183101 CrossRef
.
- Y. Luo, X. Yan, J. Zhang, B. Li, Y. Wu, Q. Lu, C. Jin, X. Zhang and X. Ren, Nanoscale, 2018, 10, 9212–9217 RSC
.
- Y. J. Yu, Y. Zhao, S. Ryu, L. E. Brus, K. S. Kim and P. Kim, Nano Lett., 2009, 9, 3430–3434 CrossRef CAS
.
- S. M. Song, J. K. Park, O. J. Sul and B. J. Cho, Nano Lett., 2012, 12, 3887–3892 CrossRef CAS
.
- G. Fiori and G. Iannaccone, IEEE Electron Device Lett., 2009, 30, 261–264 CAS
.
- R. Liu, X. C. You, X. W. Fu, F. Lin, J. Meng, D. P. Yu and Z. M. Liao, Sci. Rep., 2015, 5, 10125 CrossRef CAS PubMed
.
- Y. Luo, X. Yan, W. Wei, M. Zhang, J. Zhang, B. Li, J. Zheng, Q. Lu, Q. Lin, W. Liu, X. Zhang and X. Ren, J. Vac. Sci. Technol., B, 2018, 36, 051205 CrossRef
.
- Y. Shi, K. K. Kim, A. Reina, M. Hofmann, L. J. Li and J. Kong, ACS Nano, 2010, 5, 2689–2694 CrossRef
.
- Y. Luo, X. Yan, X. Zhang, M. Zhang, B. Li, J. Zheng, Q. Lu, Q. Lin, Q. Zeng and X. Ren, J. Vac. Sci. Technol., B, 2019, 37, 051202 CrossRef
.
- M. H. Huang, S. Mao, H. Feick, H. Yan, Y. Wu, H. Kind, E. Weber, R. Russo and P. Yang, Science, 2001, 292, 1897–1899 CrossRef CAS PubMed
.
- J. C. Johnson, H. J. Choi, K. P. Knutsen, R. D. Schaller, P. Yang and R. J. Saykally, Nat. Mater., 2002, 1, 106–110 CrossRef CAS
.
- R. Agarwal, C. J. Barrelet and C. M. Lieber, Nano Lett., 2005, 5, 917–920 CrossRef CAS PubMed
.
- D. Saxena, S. Mokkapati, P. Parkinson, N. Jiang, Q. Gao, H. H. Tan and C. Jagadish, Nat. Photonics, 2013, 7, 963–968 CrossRef CAS
.
- W. Z. Xu, W. Z. Xu, F. F. Ren, D. Jevtics, A. Hurtado, L. Li, Q. Gao, J. Ye, F. Wang, B. Guilhabert, L. Fu, H. Lu, R. Zhang, H. H. Tan, M. D. Dawson and C. Jagadish, Nano Lett., 2018, 18, 3414–3420 CrossRef CAS PubMed
.
- H. Kim, W. J. Lee, A. C. Farrell, J. S. D. Morales, P. Senanayake, S. V. Prikhodko, T. J. Ochalski and D. L. Huffaker, Nano Lett., 2017, 17, 3465–3470 CrossRef
.
- H. Sumikura, G. Zhang, M. Takiguchi, N. Takemura, A. Shinya, H. Gotoh and M. Notomi, Nano Lett., 2019, 19, 8059–8065 CrossRef CAS PubMed
.
- D. Saxena, N. Jiang, X. Yuan, S. Mokkapati, Y. Guo, H. H. Tan and C. Jagadish, Nano Lett., 2016, 16, 5080–5086 CrossRef CAS
.
- X. Yan, W. Wei, F. Tang, X. Wang, L. Li, X. Zhang and X. Ren, Appl. Phys. Lett., 2017, 110, 061104 CrossRef
.
- J. Tatebayashi, S. Kako, J. Ho, Y. Ota, S. Iwamoto and Y. Arakawa, Nat. Photonics, 2015, 9, 501–505 CrossRef CAS
.
- J. Ho, J. Tatebayashi, S. Sergent, C. F. Fong, Y. Ota, S. Iwamoto and Y. Arakawa, Nano Lett., 2016, 16, 2845–2850 CrossRef CAS
.
- X. Zhang, R. Yi, N. Gagrani, Z. Li, F. Zhang, X. Gan, X. Yao, X. Yuan, N. Wang, J. Zhao, P. Chen, W. Lu, L. Fu, H. H. Tan and C. Jagadish, ACS Nano, 2021, 15, 9126–9133 CrossRef CAS PubMed
.
- T. Stettner, A. Thurn, M. Döblinger, M. O. Hill, J. Bissinger, P. Schmiedeke, S. Matich, T. Kostenbader, D. Ruhstorfer, H. Riedl, M. Kaniber, L. J. Lauhon, J. J. Finley and G. Koblmüller, Nano Lett., 2018, 18, 6292–6300 CrossRef CAS PubMed
.
- F. Schuster, J. Kapraun, G. N. Malheiros-Silveira, S. Deshpande and C. J. Chang-Hasnain, Nano Lett., 2017, 17, 2697–2702 CrossRef CAS
.
- M. Moewe, L. C. Chuang, S. Crankshaw, C. Chase and C. Chang-Hasnain, Appl. Phys. Lett., 2008, 93, 023116 CrossRef
.
- F. Lu, I. Bhattacharya, H. Sun, T. T. D. Tran, K. W. Ng, G. N. Malheiros-Silveira and C. Chang-Hasnain, Optica, 2017, 4, 717–723 CrossRef CAS
.
- M. Paladugu, J. Zou, Y. Guo, X. Zhang, H. J. Joyce, Q. Gao, H. H. Tan, C. Jagadish and Y. Kim, Appl. Phys. Lett., 2008, 93, 201908 CrossRef
.
- Y. Li, X. Yan, X. Zhang, C. Wu, J. Zheng, C. Zha, T. Fu, L. Gong and X. Ren, Opt. Laser Technol., 2022, 152, 108150 CrossRef CAS
.
- S. A. Church, N. Patel, R. Al-Abri, N. Al-Amairi, Y. Zhang, H. Liu and P. Parkinson, Adv. Opt. Mater., 2023, 11, 2202476 CrossRef CAS
.
- J. A. Alanis, D. Saxena, S. Mokkapati, N. Jiang, K. Peng, X. Tang, L. Fu, H. H. Tan, C. Jagadish and P. Parkinson, Nano Lett., 2017, 17, 4860–4865 CrossRef CAS PubMed
.
- P. Senellart, G. Solomon and A. White, Nat. Nanotechnol., 2017, 12, 1026–1039 CrossRef CAS PubMed
.
- M. Moczała-Dusanowska, Ł. Dusanowski, S. Gerhardt, Y. M. He, M. Reindl, A. Rastelli, R. Trotta, N. Gregersen, S. Höfling and C. Schneider, ACS Photonics, 2019, 6, 2025–2031 CrossRef
.
- Z. Ge, T. Chung, Y. M. He, M. Benyoucef and Y. Huo, Nano Lett., 2024, 24, 1746–1752 CrossRef CAS PubMed
.
- P. Yao, V. S. C. M. Rao and S. Hughes, Laser Photon. Rev., 2010, 4, 499–516 CrossRef CAS
.
- N. Panev, A. I. Persson, N. Sköld and L. Samuelson, Appl. Phys. Lett., 2003, 83, 2238–2240 CrossRef CAS
.
- M. T. Borgström, V. Zwiller, E. Müller and A. Imamoglu, Nano Lett., 2005, 5, 1439–1443 CrossRef
.
- A. Tribu, G. Sallen, T. Aichele, R. André, J. P. Poizat, C. Bougerol, S. Tatarenko and K. Kheng, Nano Lett., 2008, 8, 4326–4329 CrossRef CAS
.
- S. N. Dorenbos, H. Sasakura, M. P. van Kouwen, N. Akopian, S. Adachi, N. Namekata, M. Jo, J. Motohisa, Y. Kobayashi, K. Tomioka, T. Fukui, S. Inoue, H. Kumano, C. M. Natarajan, R. H. Hadfield, T. Zijlstra, T. M. Klapwijk, V. Zwiller and I. Suemune, Appl. Phys. Lett., 2010, 97, 171106 CrossRef
.
- S. Deshpande, L. Zhang, T. Hill, A. Das, H. Deng and P. Bhattacharya, IEEE Photonics Conf., 2012, 678–679 Search PubMed
.
- M. E. Reimer, G. Bulgarini, N. Akopian, M. Hocevar, M. B. Bavinck, M. A. Verheijen, E. P. A. M. Bakkers, L. P. Kouwenhoven and V. Zwiller, Nat. Commun., 2012, 3, 737 CrossRef
.
- G. Bulgarini, M. E. Reimer, M. B. Bavinck, K. D. Jöns, D. Dalacu, P. J. Poole, E. P. A. M. Bakkers and V. Zwiller, Nano Lett., 2014, 14, 4102–4106 CrossRef CAS PubMed
.
- S. Bounouar, M. Elouneg-Jamroz, M. den Hertog, C. Morchutt, E. Bellet-Amalric, R. André, C. Bougerol, Y. Genuist, J.-Ph Poizat, S. Tatarenko and K. Kheng, Nano Lett., 2012, 12, 2977–2981 CrossRef CAS
.
- M. J. Holmes, K. Choi, S. Kako, M. Arita and Y. Arakawa, Nano Lett., 2014, 14, 982–986 CrossRef CAS
.
- S. Deshpande, J. Heo, A. Das and P. Bhattacharya, Nat. Commun., 2013, 4, 1675 CrossRef
.
- S. Deshpande and P. Bhattacharya, Appl. Phys. Lett., 2013, 103, 241117 CrossRef
.
- O. Hayden, A. B. Greytak and D. C. Bell, Adv. Mater., 2005, 17, 701–704 CrossRef CAS
.
- K. Tomioka, J. Motohisa, S. Hara, K. Hiruma and T. Fukui, Nano Lett., 2010, 10, 1639–1644 CrossRef CAS PubMed
.
- C. P. T. Svensson, T. Mårtensson, J. Trägårdh, C. Larsson, M. Rask, D. Hessman, L. Samuelson and J. Ohlsson, Nanotechnology, 2008, 19, 305201 CrossRef
.
- F. Qian, S. Gradecak, Y. Li, C. Y. Wen and C. M. Lieber, Nano Lett., 2005, 5, 2287–2291 CrossRef CAS PubMed
.
- Y. H. Ra, S. Kang and C. R. Lee, Adv. Opt. Mater., 2018, 6, 1701391 CrossRef
.
- H. P. T. Nguyen, K. Cui, S. Zhang, M. Djavid, A. Korinek, G. A. Botton and Z. Mi, Nano Lett., 2012, 12, 1317–1323 CrossRef CAS PubMed
.
- H. P. T. Nguyen, K. Cui, S. Zhang, S. Fathololoumi and Z. Mi, Nanotechnology, 2011, 22, 445202 CrossRef
.
- Y.-H. Ra, R. Navamathavan, J. H. Park and C. R. Lee, Nano Lett., 2013, 13, 3506–3516 CrossRef
.
- Y.-H. Ra, R. Navamathavan, H. I. Yoo and C. R. Lee, Nano Lett., 2014, 14, 1537–1545 CrossRef CAS PubMed
.
- B. Jain, R. T. Velpula, H. Q. Thang Bui, H. D. Nguyen, T. R. Lenka, T. K. Nguyen and H. P. T. Nguyen, Opt. Express, 2020, 28, 665–675 CrossRef CAS
.
- J. Herranz, P. Corfdir, E. Luna, U. Jahn, R. B. Lewis, L. Schrottke, J. Lähnemann, A. Tahraoui, A. Trampert, O. Brandt and L. Geelhaar, ACS Appl. Nano Mater., 2020, 3, 165–174 CrossRef CAS
.
- I. Yang, Z. Li, J. Wong-Leung, Y. Zhu, Z. Li, N. Gagrani, L. Li, M. N. Lockrey, H. Nguyen, Y. Lu, H. H. Tan, C. Jagadish and L. Fu, Nano Lett., 2019, 19, 3821–3829 CrossRef CAS PubMed
.
- I. Yang, S. Kim, M. Niihori, A. Alabadla, Z. Li, L. Li, M. N. Lockrey, D. Choi, I. Aharonovich, J. Wong-Leung, H. H. Tan, C. Jagadish and L. Fu, Nano Energy, 2020, 71, 104576 CrossRef CAS
.
- A. Alhodaib, Y. J. Noori, P. J. Carrington, A. M. Sanchez, M. D. Thompson, R. J. Young, A. Krier and A. R. J. Marshall, Nano Lett., 2018, 18, 235–240 CrossRef CAS
.
- C. Soci, A. Zhang, X. Y. Bao, H. Kim, Y. Lo and D. Wang, J. Nanosci. Nanotechnol., 2010, 10, 1430–1449 CrossRef CAS
.
- L. Rigutti, M. Tchernycheva, A. D. L. Bugallo, G. Jacopin, F. H. Julien, L. F. Zagonel, K. March, O. Stephan, M. Kociak and R. Songmuang, Nano Lett., 2010, 10, 2939–2943 CrossRef CAS PubMed
.
- N. Erhard, S. Zenger, S. Morkötter, D. Rudolph, M. Weiss, H. J. Krenner, H. Karl, G. Abstreiter, J. J. Finley, G. Koblmüller and A. W. Holleitner, Nano Lett., 2015, 15, 6869–6874 CrossRef CAS PubMed
.
- P. Kuyanov, S. A. McNamee and R. R. LaPierre, Nanotechnology, 2018, 29, 124003 CrossRef CAS PubMed
.
- M. Karimi, X. Zeng, B. Witzigmann, L. Samuelson, M. T. Borgstrom and H. Pettersson, Nano Lett., 2019, 19, 8424–8430 CrossRef CAS PubMed
.
- M. Karimi, M. Heurlin, S. Limpert, V. Jain, X. Zeng, I. Geijselaers, A. Nowzari, Y. Fu, L. Samuelson, H. Linke, M. T. Borgström and H. Pettersson, Nano Lett., 2018, 18, 365–372 CrossRef CAS PubMed
.
- Y. Wu, X. Yan, X. Zhang and X. Ren, Appl. Phys. Lett., 2016, 109, 183101 CrossRef
.
- L. C. Chuang, F. G. Sedgwick, R. Chen, W. S. Ko, M. Moewe, K. W. Ng, T. T. D. Tran and C. Chang-Hasnain, Nano Lett., 2011, 11, 385–390 CrossRef CAS PubMed
.
- G. Bulgarini, M. E. Reimer, M. Hocevar, E. P. A. M. Bakkers, L. P. Kouwenhoven and V. Zwiller, Nat.
Photonics, 2012, 6, 455–458 CrossRef CAS
.
- W. Jin, Y. Ye, L. Gan, B. Yu, P. Wu, Y. Dai, H. Meng, X. Guo and L. Dai, J. Mater. Chem., 2012, 22, 2863–2867 RSC
.
- Z. Gao, W. Jin, Y. Zhou, Y. Dai, B. Yu, C. Liu, W. Xu, Y. Li, H. Peng, Z. Liu and L. Dai, Nanoscale, 2013, 5, 5576–5581 RSC
.
- B. D. Boruah, A. Mukherjee and A. Misra, Nanotechnology, 2016, 27, 095205 CrossRef PubMed
.
- L. Duan, F. He, Y. Tian, B. Sun, J. Fan, X. Yu, L. Ni, Y. Zhang, Y. Chen and W. Zhang, ACS Appl. Mater. Interfaces, 2017, 9, 8161–8168 CrossRef CAS PubMed
.
- N. Prakash, M. Singh, G. Kumar, A. Barvat, K. Anand, P. Pal, S. P. Singh and S. P. Khanna, Appl. Phys. Lett., 2016, 109, 242102 CrossRef
.
- M. Zhou, Y. Zhao, Q. Zhang, X. Gu, J. Zhang, M. Jiang and S. Lu, Opt. Lett., 2024, 49, 338–341 CrossRef CAS
.
- H. Chaliyawala, N. Aggarwal, Z. Purohit, R. Patel, G. Gupta, A. Jaffre, S. L. Gall, A. Ray and I. Mukhopadhyay, Nanotechnology, 2020, 31, 225208 CrossRef CAS PubMed
.
- N. Anttu, ACS Photonics, 2015, 2, 446–453 CrossRef CAS
.
- P. Krogstrup, H. I. Jørgensen, M. Heiss, O. Demichel, J. V. Holm, M. Aagesen, J. Nygard and A. F. i Morral, Nat. Photonics, 2013, 7, 306–310 CrossRef CAS
.
- Y. Xu, T. Gong and J. N. Munday, Sci. Rep., 2015, 5, 13536 CrossRef PubMed
.
- S. A. Mann, R. R. Grote, R. M. Osgood, Jr., A. Alu and E. C. Garnett, ACS Nano, 2016, 10, 8620–8631 CrossRef CAS
.
- D. Mikulik, M. Ricci, G. Tutuncuoglu, F. Matteini, J. Vukajlovic, N. Vulic, E. Alarcon-Llado and A. F. i Morral, Nano Energy, 2017, 41, 566–572 CrossRef CAS
.
- I. Åberg, G. Vescovi, D. Asoli, U. Naseem, J. P. Gilboy, C. Sundvall, A. Dahlgren, K. E. Svensson, N. Anttu, M. Björk and L. Samuelson, IEEE J. Photovoltaics, 2015, 6, 185–190 Search PubMed
.
- D. van Dam, N. J. J. van Hoof, Y. Cui, P. J. van Veldhoven, E. P. A. M. Bakkers, J. G. Rivas and J. E. M. Haverkort, ACS Nano, 2016, 10, 11414–11419 CrossRef CAS PubMed
.
- L. Hrachowina, Y. Chen, E. Barrigón, R. Wallenberg and M. T. Borgström, Mater. Today Energy, 2022, 27, 101050 CrossRef CAS
.
- M. H. T. Dastjerdi, E. M. Fiordaliso, E. D. Leshchenko, A. Akhtari-Zavareh, T. Kasama, M. Aagesen, V. G. Dubrovskii and R. R. LaPierre, Nano Lett., 2017, 17, 5875–5882 CrossRef CAS PubMed
.
- E. Koren, N. Berkovitch and Y. Rosenwaks, Nano Lett., 2010, 10, 1163–1167 CrossRef CAS
.
- A. Troian, G. Otnes, X. Zeng, L. Chayanun, V. Dagyte, S. Hammarberg, D. Salomon, R. Timm, A. Mikkelsen, M. T. Borgstrom and J. Wallentin, Nano Lett., 2018, 18, 6461–6468 CrossRef CAS
.
- C. Gutsche, R. Niepelt, M. Gnauck, A. Lysov, W. Prost, C. Ronning and F. Tegude, Nano Lett., 2012, 12, 1453–1458 CrossRef CAS PubMed
.
- H. Park, S. Chang, J. Jean, J. J. Cheng, P. T. Araujo, M. Wang, M. G. Bawendi, M. S. Dresselhaus, V. Bulovic, J. Kong and S. Gradečak, Nano Lett., 2013, 13, 233–239 CrossRef CAS
.
|
This journal is © The Royal Society of Chemistry 2025 |
Click here to see how this site uses Cookies. View our privacy policy here.