DOI:
10.1039/D4NA00327F
(Paper)
Nanoscale Adv., 2024,
6, 4693-4703
A field-portable electrochemical immunosensor based on a multifunctional Ag2O/g-C3N4@MA-DBB covalent organic framework receptor interface for single-step detection of aflatoxin M1 in raw milk samples†
Received
19th April 2024
, Accepted 12th July 2024
First published on 31st July 2024
Abstract
Aflatoxin M1 (AFM1), a hydroxylated metabolite of aflatoxin B1 (AFB1), contaminates milk and dairy products from livestock via ingestion of feed contaminated with a species of Aspergillus. As AFM1 is a Group-II B carcinogen, it is indispensable to develop novel, easy-to-handle, sensitive, portable and cost-effective strategies for its detection. Herein, a covalent organic framework (COF)-based electroactive nanocomposite, Ag2O/g-C3N4-COOH@MA-DBB-COF (silver oxide/carboxy-functionalized graphitic carbon nitride@melamine-dibromo butane COF), is designed to serve as a multifunctional receptor surface. The Ag2O/g-C3N4-COOH@MA-DBB-COF formation was characterized through X-ray diffraction (XRD), Fourier transform infrared (FTIR) spectroscopy, micro-Raman spectroscopy (RAMAN), dynamic light scattering (DLS) and thermogravimetric analysis (TGA), and each step of the sensor fabrication was monitored using field emission scanning electron microscopy (FE-SEM), cyclic voltammetry (CV) and electrochemical impedance spectroscopy (EIS). Under the optimal conditions, the designed immunosensor permitted the detection of AFM1 in the linear range of 0.03–1000 fg mL−1, with a 0.01 fg mL−1 limit of detection (LOD). The selectivity of the designed immunosensor was validated via an anti-interference study. The practical applicability of the immunosensor was demonstrated by the detection of AFM1 in real milk samples, and good recovery values (97.28–102.62%) were obtained. Furthermore, the developed immunosensor and high-performance liquid chromatography (HPLC) were employed in parallel to detect AFM1 in local market milk samples from twenty different sites to validate the performance of the newly designed immunosensor. Additionally, the designed immunosensor was stable over an extended period of time. This work reports a single-step field-portable multifunctional innovative electrochemical immunoreceptor design for on-site and label-free detection of AFM1 in milk samples. Hence, the present study is the first report on the fabrication of a multifunctional innovative electrochemical immunoreceptor based on PGE/Ag2O/g-C3N4-COOH@MA-DBB-COF for the detection of AFM1 in milk samples.
1. Introduction
Milk is an essential dietary element that is rich in micro- and macronutrients. It plays a vital role in growth and development, especially in infants and children.1,2 The quality of milk is deteriorated by contaminants such as aflatoxins, particularly AFM1.3 Aflatoxins are structurally related toxic secondary metabolites produced by species of Aspergillus in corn, rice, nuts, peanuts, coconut, dried fruits and spices.4–8 Among the more than 20 different aflatoxins, AFB1 is the most toxic.9 AFM1 is a hydroxylated metabolite of AFB1.10 AFB1 from contaminated animal feed is metabolized in the liver by cytochrome P-450 to AFM1 and excreted in the milk directly, contaminating milk and dairy products.11 Its toxicity profile includes growth retardation, immunosuppression, genotoxicity, and carcinogenicity.12,13 AFM1 was initially classified as a group IIB human carcinogen by IARC and has recently been moved to group I.14 Due to its extreme toxicity, maximum permissible limits for AFM1 in milk ranging from 0.025 to 0.5 μg L−1 have been set by food regulatory agencies such as FAO and EC.15
AFM1 is highly thermostable and resistant to conventional cooking and food processing practices such as irradiation, pasteurization, and ultraheat treatment (UHT). Prolonged exposure to AFM1 is very dangerous, even at low concentrations.16 In order to maintain food safety and human health, accurate monitoring AFM1 of is of fundamental importance. The established methods for the detection of AFM1 include thin layer chromatography (TLC) modified to high performance HPTLC, high-performance liquid chromatography-fluorescence detection (HPLC-FLD), liquid chromatography coupled tandem mass spectrometry (LC-MS/MS) and enzyme-linked immunosorbent assay (ELISA).17–20 Although chromatographic techniques are accurate, promising high sensitivity, they require tedious and costly sample clean-up procedures, highly expensive and massive equipment, and skilled personnel to attain good accuracy and precision.21 The downsides of ELISA are extended incubation times, frequent inaccurate positive or negative screening reports, less sensitivity and inadequate detection range.22,23 Hence, it is indispensable to develop novel, easy-to-handle, sensitive, portable, compact-sized and cost-effective strategies for AFM1 detection.
With the advent of nanotechnology, currently, immunosensors with improved sensitivity and selectivity represent an attractive field of analytical research for aflatoxin detection.24 A number of AFM1 immunosensors have been reported using discrete nanoparticles (NPs) such as Au NPs, quantum dots, Fe2O3 NPs, Ni NPs, Fe3O4 NPs, and countless others to perform one particular function to improve the analytical figure of merit.25–29 Recently, using ELISA with N-doped photoluminescent carbon dots, ultrasensitive detection of AFM1 was achieved with a high quantum yield.30 Further, a strong anti-idiotypic nanobody was used on a screen-printed carbon electrode to replace a highly toxic synthetic antibody for quantitative AFM1 detection through chronoamperometry.31 However, multiphasic nanocomposite materials prepared by combining two or more different constituents in one material can perform multifunctional roles. Each constituent offers exceptional tunable properties due to its small size, large surface-to-volume ratio, and, particularly, due to the interfacial interaction among the phases.32,33 An immunosensor for robust AFM1 detection was designed using graphene oxide-chitosan and cerium oxide-chitosan on a screen-printed electrode.34 A nanocomposite consisting of metal–organic-framework-incorporated dichalcogenide quantum dots was fabricated on a screen-printed electrode for the monitoring of AFM1.35 Herein, the synthesis of a novel COF-based multifunctional nanocomposite material is reported to improve the analytical performance of a single-step-operation immunosensor for the ultrasensitive detection of AFM1 in milk samples.
The application of graphitic carbon nitride (g-C3N4) in analytical disciplines is attributed to its exceptional surface properties such as biocompatibility, ease of surface functionalization and hydrogen bonding.36,37 Moreover, its restricted electrochemical performance due to its chemical inertness and poor conductivity38,39 has been improved by customization with nanomaterials.40–43 The present study reports the design and fabrication of a novel electrochemical immunosensor based on a binary metal oxide (Ag2O) incorporated in g-C3N4 and modified with a porous crystalline polymer matrix. Nanomaterials are frequently modified by means of direct functionalization during synthesis or coating with functional polymers without affecting their specific properties.44 Such functionalization not only allows reproducible immobilization of bioreceptor units, but can also increase the biocompatibility of these materials.45 In the present study, Ag2O/g-C3N4 was acidified to Ag2O/g-C3N4-COOH to offer a covalent immobilization site for the probe as well as signal magnification to develop a label-free electrochemical sensor.
The tunable properties of COFs, such as their high surface area, variable porosity, thermal and chemical stability, and high electrical conductivity, has made them materials of choice for diverse applications.46 In the present study, a melamine- and 1,4-dibromobutane-based synthesized MA-DBB-COF was ligated with Ag2O/g-C3N4-COOH through van der Waals interactions. This developed via the lone pairs available at the N of g-C3N4 and –N–H of the COF and was conducive for charge transfer. Hence, the incorporation of MA-DBB-COF improved the electrical conductivity of the immunosensor, leading to further signal amplification. In the designed nanocomposite, the Ag in Ag2O served as a signal-generating probe to produce a precise electric readout of the presence of the analyte with high sensitivity. g-C3N4-COOH, which exhibits high biocompatibility, served as a covalent immobilization site for the probe and bio-recognition unit. g-C3N4-COOH can develop an amide linkage with the amino group of bio-receptors or can be functionalized as g-C3N4-NH2 to develop a similar linkage with the –COOH group of the bioreceptors. Thus, functionalized g-C3N4 offers wide-scale biocompatibility as an immobilization support. The ability of the nanocomposite material to be electrochemically oxidized is the basis of the sensing of the analyte by generating a direct oxidation read-out signal. Thus, the intrinsic oxidation signal of the synthesized nanocomposite served not only as an analytical signal, but also as a label to detect the analyte. The designed hybrid nanocomposite material offers: (i) automated signal generation, (ii) immobilization of the probe and bioreceptor on the transducer platform, and (iii) synergistic conductivity for signal amplification. The designed nanocomposite material is applied on a pencil graphite electrode (PGE) to fabricate the immunosensor for the detection of AFM1. To the best of our knowledge, the present study is the first report on the fabrication of a multifunctional innovative electrochemical immunoreceptor based on PGE/Ag2O/g-C3N4-COOH@MA-DBB-COF for AFM1 detection in milk samples.
2. Methodology
2.1. Chemicals & apparatus
All the reagents used in the experimental section were of analytical grade. Melamine (C3N3(NH2)3) (Dae-Jung), 1,4-dibromobutane (C4H8Br2), dimethyl sulfoxide (C2H6OS), sodium hydroxide (NaOH), N-hydroxy succinimide (C4H5NO3) (NHS), N-(3-dimethylaminopropyl)-N′-ethyl-carbodiimide hydrochloride (C8H17N3) (EDC), AFM1 (C17H12O7) and anti-AFM1 monoclonal antibody were purchased from Alfa Aesar. Chloroacetic acid (C2H3ClO2), potassium ferricyanide (K3Fe(CN)6), potassium ferrocyanide (K4Fe(CN)6), and phosphate buffer saline tablets (Cl2H3K2Na3O8P2) pH 7.4 were purchased from UNI-CHEM. Bovine serum albumin (BSA, MW 66 kDa) was from Sigma Aldrich, France. All solutions were prepared in distilled water.
An electrochemical workstation (Potentiostat Interface GAMRY 1010E, USA), equipped with a platinum counter electrode, Ag/AgCl as a reference electrode and PGE as the working electrode was used for electrochemical measurements. The immunosensor was designed as PGE/Ag2O-g-C3N4-COOH@MA-DBB-COF. The surface morphology of the gradual fabrication of PGE was studied using FE-SEM (Apreo S, Thermo Fisher Scientific), CV and EIS. The synthesized COF-based hybrid nanocomposite and constituent materials were subjected to FTIR in the scan range of 600–4000 cm−1 using a Thermo Fischer Scientific (Nicolet 6700, USA) spectrometer. Polymorphism, crystallinity, and molecular dynamics were analyzed using an In-Via RAMAN microscope (Renishaw, UK) at an excitation wavelength of 514 nm for 10 s. The XRD patterns were obtained using a PXRD PANalytical Xpert powder diffractometer (Malvern) at a scan range of 0–80. The thermostability of the synthesized material was examined via TGA (BXT-DSC-TGA-1250) at 25–800 °C under nitrogen atmosphere (heating rate: 10 °C min−1). The particle size measurements were performed using a Zetasizer Nano ZS90 (Malvern, UK).
2.2. Synthesis of Ag2O/g-C3N4-COOH@MA-DBB-COF nanocomposite
The g-C3N4 was synthesized following Ahmed et al., 2020 with slight modification.47 Melamine (15 g) was heated to 550 °C at a ramp rate of 5 °C min−1 for 4 h. Afterwards, the obtained amber-colored product was ground into a fine powder. To prepare Ag2O/g-C3N4, g-AgNO3 (8.2 g) and C3N4 (250 mg) were sonicated in 40 mL distilled water for 10 min, followed by the addition of 6 mg of K2HPO4 at 80 °C. The product was dried in an oven at 60 °C for 24 h. The functionalization of Ag2O/g-C3N4 was carried out following Rauf et al., 2018 (ref. 48) with slight modification. The Ag2O/g-C3N4 (1 mg mL−1 distilled H2O) dispersion was obtained through sonication. Subsequently, to obtain Ag2O/g-C3N4-COOH, NaOH (0.3 g) and C2H3ClO2 (0.2 g) were added to the above dispersion, and the mixture was used for electrochemical assay.
MA-DBB-COF was synthesized following Sahiner et al., 2016 (ref. 49) with slight modification. Melamine (0.0939 g/5 mL DMSO) and 1,4-dibromobutane (136 μL) were heated with magnetic stirring at 150 °C for 2 h. The obtained tangerine-colored precipitate of MA-DBB-COF was dried in an incubator at 37 °C for 45 min and stored for subsequent use.
2.3. Fabrication of electrochemical immunosensor (PGE/Ag2O/g-C3N4-COOH@MA-DBB-COF)
The COF-based electroactive nanocomposite Ag2O/g-C3N4-COOH@MA-DBB-COF was prepared by adding Ag2O/g-C3N4-COOH (1 mg) and MA-DBB-COF (0.4 mg) to distilled water (1 mg Ag2O/g-C3N4-COOH + 0.4 mg COF mL−1), followed by sonication for 3–4 min. The surface of the modified working electrode (PGE/Ag2O/g-C3N4-COOH@MA-DBB-COF) was fabricated by incubating PGE in 10 μL of the nanocomposite (Ag2O/g-C3N4-COOH@MA-DBB-COF) for 30 min and then allowing it to dry. Following the same procedure, PGE/Ag2O/g-C3N4 and PGE/Ag2O/g-C3N4-COOH were also fabricated, and DPV of all fabricated electrodes was performed (−0.2–0.5 V). As the designed immunosensor offers immobilization support to the bioreceptors through its functionalization feature, anti-AFM1 antibody was conjugated to the carboxylic group on the electrode surface. This was carried out by incubating a mixture of 10 μL NHS (25 mM), EDC (100 mM), and the optimized anti-AFM1 antibody concentration (1/4000 unit in PBS from a stock solution (6.6 mg mL−1)) was used in further experimentation. Subsequently, 1% BSA was used as a blocking protein for 30 min at the surface of the anti-AFM1-antibody-immobilized fabricated PGE.
2.4. Milk sample analysis using the designed electrochemical immunosensor and HPLC
A stock solution of AFM1 (1 μL mL−1 PBS) from 1.54 μM AFM1 in acetonitrile was prepared and stored at −20 °C. The working standard solutions of 0.03–1000 fg mL−1 prepared from the stock solution were used, and calibration curve was obtained by plotting the peak currents vs. the concentration of AFM1. The LOD was calculated using the standard deviation of the response and the slope of the calibration curve, expressed as LOD = 3.3σ/S, where σ represents the standard deviation and S is the slope of the calibration curve.
The milk sample (5 mL) was centrifuged at 2000 rpm/20 min to remove fats. Subsequently, different concentrations of AFM1 standards (6, 420 and 910 fg mL−1) were spiked into the skimmed milk sample. Finally, the designed immunosensor was utilized to analyze the extract to study the viability of the proposed sensor in the food matrix.
To cross-validate the analytical performance of the designed immunosensor, HPLC (ProminanceTM, Shimadzu®, Kyoto, Japan) analysis of AFM1 was also performed. The method was established with standard solution of AFM1. A spectrofluorometer detector RF-10AXL ® (Shimadzu, Japan) set at 365 nm excitation and 425 nm emission wavelengths was used. The isocratic mobile phase (20
:
60
:
20) of acetonitrile
:
water
:
methanol was used at a flow rate of 1.5 mL min−1 10 μL was the injection volume using a Rheodyne® manual sample injector. Sample cleanup was carried out using immunoaffinity columns (Afla star company, Australia). Milk samples from twenty different sites of local markets were collected and tested for the presence/absence of AFM1 through both HPLC and the designed immunosensor.
3. Results and discussion
3.1. Surface characterization
Fig. 1A shows the comparative FTIR spectra of the synthesized materials, g-C3N4 and Ag2O/g-C3N4. The sharp peak at 807 cm−1 can be assigned to breathing mode vibrations, which are a characteristic feature of triazine rings, and the peaks at 1530 and 1640 cm−1 can be attributed to C
N stretching.50,51 In the absorption spectra of Ag2O/g-C3N4 (b), relatively sharp peaks were observed between 1400–1600 cm−1. The broad peak in the region 2500–3500 cm−1 was less intense and wider due to Ag2O loading in the Ag2O/g-C3N4 nanocomposite. As is evident in the FTIR spectrum of Ag2O/g-C3N4 in Fig. 1A, the absorption spectrum of g-C3N4 showed peak shifts in the region 800–1700 cm−1, which were attributed to the coordination linking of Ag and N atoms, indicating successful incorporation of Ag2O in g-C3N4.47,52 Furthermore, the FTIR spectra of MA-DBB-COF (c), and Ag2O/g-C3N4-COOH@MA-DBB-COF (d) are presented in Fig. 1A, and the details of the peaks are given in Table S1.† In the spectrum of Ag2O/g-C3N4-COOH@MA-DBB-COF (d), the peaks (700–1550 cm−1) were less sharp, indicating loading of Ag2O/g-C3N4 on the MA-DBB-COF surface. Sharp peaks at 770, 1022 and 1130 cm−1 in the absorption spectrum of MA-DBB-COF (c) are representative of ring-out-of-plane deformations, and were broader and shifted to higher intensity for Ag2O/g-C3N4-COOH@MA-DBB-COF (d). To confirm the successful synthesis, structural changes were observed through Raman spectrographs (Fig. 1B). The graphitic and defect behavior is represented by the characteristic G and D bands, and the intensities of the disorder and graphite band (ID/IG) of each reacting component and nanocomposite material (Table S2†). g-C3N4 displayed a D-band at 800 cm−1 of the s-triazine unit. In the case of Ag2O/g-C3N4-COOH, the relatively intense peak at 430 cm−1 indicates Ag2O incorporated on the surface. The numerous newly observed peaks at 790, 990, 1260, 1470, 1803 and 1950 cm−1 represent interfacial interaction between Ag2O and g-C3N4-COOH.47,53 In the spectra of MA-DBB-COF, the peak at 1705 cm−1 can be assigned to aromatic C–N stretching vibrations, whereas the peaks at 2130 cm−1 and 2515 cm−1 indicate C
N bending modes. In the final COF-based nanocomposite, Ag2O/g-C3N4-COOH@MA-DBB-COF, the incorporation of Ag2O is represented by the peak at 468 cm−1 and that of triazine subunit by the peak at 812 cm−1. There is a gradual increase in the ID/IG ratio from g-C3N4 to Ag2O/g-C3N4-COOH@MA-DBB-COF, exhibiting more defects and less graphitic behavior in the final composite material, as shown in Table S2.†
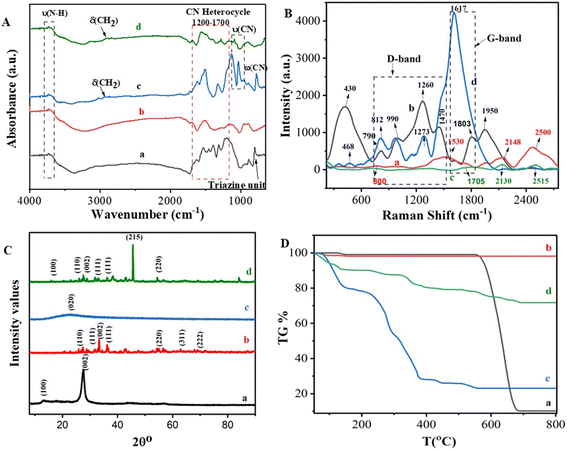 |
| Fig. 1 (A) FTIR spectra, (B) Raman spectra, (C) XRD patterns, and (D) thermogravimetric analysis of (a) g-C3N4, (b) Ag2O/g-C3N4-COOH, (c) MA-DBB-COF and (d) Ag2O/g-C3N4-COOH@MA-DBB-COF. | |
The crystallinity and phases of the g-C3N4 (a), Ag2O/g-C3N4 (b), MA-DBB-COF (c) and Ag2O/g-C3N4-COOH@MA-DBB-COF (d) were characterized using XRD (Fig. 1C). In g-C3N4, the two diffraction peaks appearing at 13.1° and 27.5° were suggested to correspond to the (100) and (002) planes of hexadic g-C3N4 (JCPDS 87-1526), respectively.54 In the Ag2O/g-C3N4 (b) nanocomposites, both the peaks were less intense, and a few additional peaks observed at various 2θ° values of were attributed to the (110), (111), (220), (311) and (222) diffraction planes of the cubic crystal phase (JCPDS 41-1104).55 This shows the successful incorporation of Ag2O in g-C3N4. In the XRD pattern of the synthesized COF (c), there was a broad peak extending from 14° to 34°, which was suggested to be (020). The final composite material, Ag2O/g-C3N4-COOH@MA-DBB-COF (d) was found to be crystalline. It showed peaks referring to the (100), (110), (002), (111) and (220) refractive angles. Thermogravimetric analysis was performed to analyze the thermal stability of the synthesized composite material (Fig. 1D). g-C3N4 exhibits (a) an onset 1% weight loss between 123–149 °C. This may be attributed to loss of water trapped in the interstices. g-C3N4 remained intact between 150–559 °C, and an 87.7% decrease in weight was observed between 599 and 681 °C with only 0.1% further weight loss between 681 and 800 °C. As shown in Fig. 1D(b), Ag2O/g-C3N4-COOH showed 2% weight loss at 48–98 °C followed by only 0.34% decrease in weight between 129 and 154 °C. The entirely different TGA profiles of g-C3N4 and Ag2O/g-C3N4 indicate the successful synthesis of Ag2O/g-C3N4. MA-DBB-COF (c) showed three-phase degradation: a first phase at 72–150 °C, a second at 150–393 °C and a third phase between 390–561 °C. Finally, as shown in Table S3,† the Ag2O/g-C3N4-COOH@MA-DBB-COF (d) displayed a different TGA profile, with a 10% weight loss in the range 100–150 °C, followed by 11% weight loss between 232 and 454 °C. Lastly, between 506 and 692 °C, only 7.3% weight loss was observed. Afterwards, the composite remained intact at up to 800 °C. Thus, a highly thermostable composite was synthesized, as 72% of the composite did not degrade even at very high temperature.
Zeta potential measurements were performed to study the surface modifications of the synthetic materials. The particle size of the final composite material Ag2O/g-C3N4-COOH@MA-DBB-COF was found to be 442.6 nm. All the contributing materials intrinsically had negative zeta potentials. However, upon acidification, the zeta potential of Ag2O/g-C3N4-COOH increased to −78.1 mV from −0.421 mV for g-C3N4 due to the development of the negatively charged surface attributed to –COO−. MA-DBB-COF and Ag2O/g-C3N4-COOH@MA-DBB-COF showed zeta potentials of −7.02 mV and −80.4 mV, respectively. Particles with a zeta potential >+30 mV or >−30 mV are considered stable; thus, a stable nanocomposite was prepared.
3.2. Principle design of electrochemical immunosensor
The ability of the synthesized COF-based electroactive nanocomposite material to undergo electrooxidation, and to offer immobilization support to the bioreceptor and signal amplification through enhanced electrical conductivity, are the basic principles of the designed label-free electrochemical immunosensor. The presence of electroactive Ag in the nanocomposite generates a sharp oxidation signal response in an electrochemically inactive PBS solution. The working principle of the designed electrochemical immunosensor and electrochemical response of the designed sensing platform are displayed in Scheme 1. The unmodified PGE did not generate any electrical signal. Upon customization of the PGE with the Ag2O/g-C3N4 hybrid nanocomposite, an oxidation peak at a potential of +0.14 V was observed, as is evident in Fig. S1(a),† which affirmed the electroactive nature of the integrated nanocomposite.56,57 Acidification of Ag2O/g-C3N4 to Ag2O/g-C3N4-COOH not only resulted in a decrease in the electrical signal, but also added the feature of biocompatibility. Ag2O/g-C3N4-COOH was integrated with MA-DBB-COF, and the resulting modified PGE/Ag2O/g-C3N4-COOH@MA-DBB-COF showed significant signal amplification of the electro-oxidation signal of Ag+, as shown in Fig. S1(c).† Herein, the material modification to fabricate the transducer surface, particularly with COF, was relatively conductive in nature.58 The –COOH group in Ag2O/g-C3N4-COOH of the modified PGE was activated by the cross-linker EDC/NHS to develop an amide linkage with the anti-AFM1 antibody for aligned orientation. The scope of the study can be extended in terms of material modification and wide-scale sensing application, as Ag2O/g-C3N4 can be functionalized to Ag2O/g-C3N4-NH2, as well as the Ag2O/g-C3N4-COOH presented in this report. The –COOH in the modified nanocomposite can develop an amide linkage with the –NH2 terminal of various biorecognition molecules; the antibody anti-AFM1 was chosen to establish the working principle in the present study. Subsequently, BSA was used as a blocking agent to avoid any nonspecific adsorption at the surface of the anti-AFM1-antibody-bound fabricated PGE. The decrease in the electrical signal due to complexation between the bioreceptor and target analyte is the basis of detection. PGE was customized with Ag2O/g-C3N4-COOH@MA-DBB-COF to serve as a multifunctional electrochemical transduction platform. AFM1 was used as a target analyte to validate the working principle of the designed transducer. The DPV response of the modified PGE decreased in the presence of AFM1, indicating that the anti-AFM1-antibody-immobilized nano-transducer interface was electroreceptive, complexing with AFM1. There was a proportional decrease in the electrooxidation signal of Ag+ of the designed modified PGE with increasing concentrations of AFM1. This decrease can be used for quantitative estimation of AFM1.
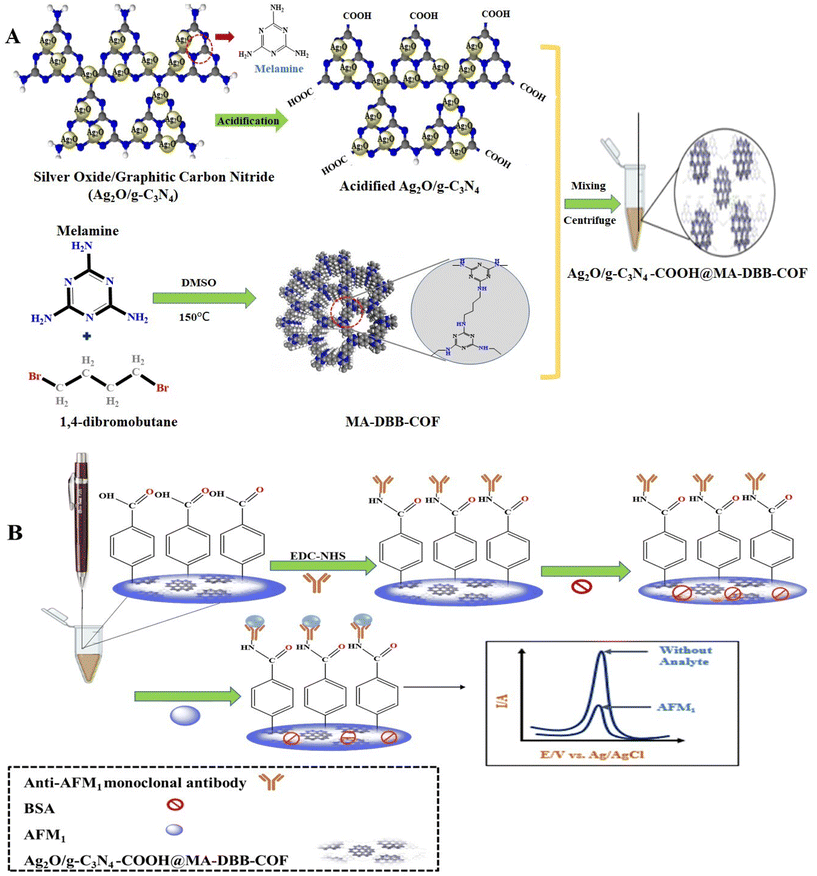 |
| Scheme 1 (A) Preparation of acidified Ag2O/g-C3N4-COOH@MA-DBB-COF; (B) immunosensor fabrication. | |
3.3. Electrochemical surface characterization of the receptor surface
CV, EIS and FE-SEM images were used to characterize each step of fabrication for the development of the immunosensor. The step-wise consecutive measurements were carried out against 0.1 mM ferri/ferrocyanide as a reversible redox probe, at a 50 mV s−1 scan rate, as shown in Fig. 2A. The peak-to-peak separation and variation in peak current at each step of PGE fabrication relate to the resistance and heterogenous electron transfer rate. The bare PGE showed a couple of anodic and cathodic peaks. After the deposition of Ag2O/g-C3N4-COOH@MA-DBB-COF/EDC-NHS on PGE, there was an increase in the electron transfer rate, as indicated by the decrease in the peak-to-peak separation value. The fabricated PGE showed an increased current response compared to unmodified PGE. Upon the covalent binding of anti-AFM1 antibody on the modified PGE, there was a decrease in the CV response of the redox couple, as the bulky anti-AFM1 antibody molecule may create hindrance in electron transfer. The next fabrication step was BSA treatment to block nonspecific adsorption, which further decreased the CV response. The hindered electron-transfer kinetics of ferri/ferrocyanide were observed in the interaction of AFM1 with the modified PGE, causing blockage of the redox peak. The EIS results are consistent with CV surface characterization, as displayed in Fig. 2A and B. There was an increase in electron-transfer resistance, confirming that designed approach is suitable for the detection of AFM1. The data regarding the electroactive surface area and peak potential difference ΔEp are presented in Table S4.† The bare PGE had an electroactive surface area of 0.024 cm2, whereas modification of the electrode with Ag2O/g-C3N4-COOH@MA-DBB-COF/EDC-NHS increased the electroactive surface to 0.031 cm2. Further, the deposition of anti-AFM1 antibody on PGE/Ag2O/g-C3N4-COOH@MA-DBB-COF decreased the electroactive surface area (0.021 cm2) compared to that of the modified immunosensor.
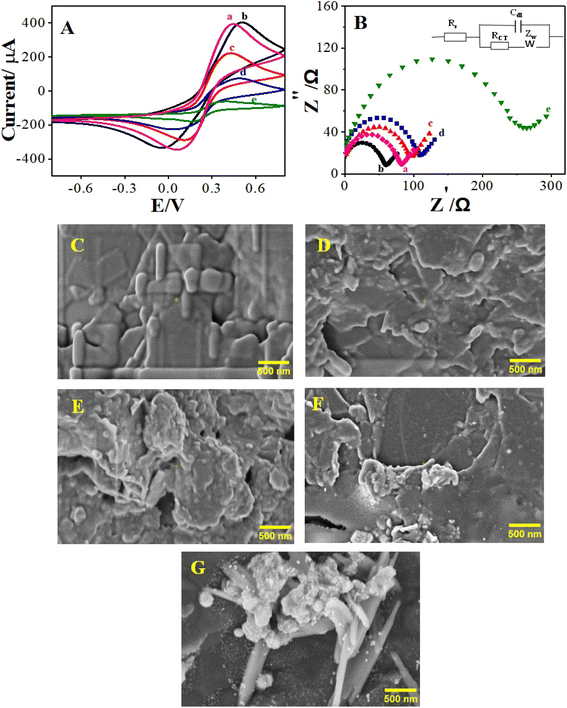 |
| Fig. 2 (A) Cyclic voltammograms and (B) electrochemical impedance spectroscopy (Nyquist plot) of 0.1 M [Fe(CN)6]3−/4− at a scan rate of 50 mV s−1 for the (a) bare electrode, (b) EDC-NHS/Ag2O/g-C3N4-COOH@MA-DBB-COF/PGE, (c) anti-AFM1 antibody/EDC-NHS/Ag2O/g-C3N4-COOH@MA-DBB-COF/PGE, (d) BSA/anti-AFM1 antibody/EDC-NHS/Ag2O/g-C3N4-COOH@MA-DBB-COF/PGE and (e) AFM1/BSA/anti-AFM1 antibody/EDC-NHS/Ag2O/g-C3N4-COOH@MA-DBB-COF/PGE. FE-SEM images of the step-wise modification of the pencil graphite electrode for the (C) bare electrode, (D) EDC-NHS/Ag2O/g-C3N4-COOH@MA-DBB-COF/PGE, (E) anti-AFM1 antibody/EDC-NHS/Ag2O/g-C3N4-COOH@MA-DBB-COF/PGE, (F) BSA/anti-AFM1 antibody/EDC-NHS/Ag2O/g-C3N4-COOH@MA-DBB-COF/PG and (G) AFM1/BSA/anti-AFM1 antibody/EDC-NHS/Ag2O/g-C3N4-COOH@MA-DBB-COF/PGE. | |
The electrochemical signal of Ag+ in the designed nanocomposite material was monitored through DPV in 0.1 M PBS (pH = 7.0) as shown in Fig. S1 & S2.† Anti-AFM1 antibody deposition followed by AFM1 binding resulted in successive decreases in the electrical signal, which formed the basis for quantitative determination.
In order to examine the structural changes at molecular level during the different fabrication steps of the immunosensor, the surface morphology was studied using FE-SEM to show the modification in the surface nature. It can be observed from Fig. 2C that the unmodified PGE has distinct morphology in comparison to the other stages, i.e., when PGE was modified with EDC-NHS and BSA anti-AFM1 antibody and AFM1 were used to test the performance of the designed sensor. The distinct morphological changes are further evident in the FE-SEM images of the fabrication steps in Fig. S3.† The surface modification via their step-wise application on the designed immunosensor was also compared using FE-SEM. It can also be observed that the surface roughness of PGE increased after the deposition of EDC-NHS/Ag2O/g-C3N4-COOH@MA-DBB-COF and was further enhanced upon the application of anti-AFM1 antibody/EDC-NHS/Ag2O/g-C3N4-COOH@MA-DBB-COF. Similarly, the surface morphology of the designed immunosensor changed upon the addition of anti-AFM1 antibody followed by AFM1.
3.4. Optimization of experimental parameters
To improve the analytical performance of the developed immunosensor, the optimization of several factors is critical. Analytical variables like incubation time, concentration of the composite material, bioreceptor concentration and analyte incubation time can substantially affect the performance of the sensor. The analytical performance of the designed immunosensor can be affected by the incubation time of the materials constituting the COF-based nanocomposite material. Different time intervals (5–60 min) for the incubation of Ag2O/g-C3N4-COOH on PGE were observed. There was a proportional increase in the electrical response with gradual increase in incubation time. After 30 min, there was a decrease in the electrical signal, as shown in Fig. S4(A).† At 30 min, the maximum electro-oxidation of Ag was accomplished; the subsequent decrease in the electrical signal indicates the reversibility of the reaction. Fig. S4(B)† represents the effect of the concentration of MA-DBB-COF on the peak current in the 0.1 M PBS. Different concentrations (0.1–0.5 mg mL−1) of MA-DBB-COF were used, and the highest electrical response was observed at 0.4 mg mL−1 of MA-DBB-COF. Afterwards, the excessive deposition of MA-DBB-COF on the electrode surface led to a steady electron transfer rate.
The electrical signal is directly influenced by the variation in bioreceptor concentration. Therefore, different concentrations (1/1000 to 1/10
000 units in PBS) of anti-AFM1 antibody were immobilized at the modified PGE/Ag2O/g-C3N4-COOH@MA-DBB-COF electrode, and DPVs were recorded to determine the optimum response. The optimum working dilution of anti-AFM1 antibody was found to be 1/4000 unit in PBS, which caused the maximum decrease in the electrical signal, as shown in Fig. S4D.† Further non-specific adsorption of anti-AFM1 antibody on PGE/Ag2O/g-C3N4-COOH@MA-DBB-COF was prevented by using 1% BSA solution for 30 min. The incubation time for anti-AFM1 antibody and AFM1 were optimized by considering different time intervals (5, 15, 30, 45 and 60 min). The optimum incubation time for anti-AFM1 antibody was 30 min (Fig. S4C†). AFM1 exhibited maximum immobilization of anti-AFM1 antibody at 30 min, as shown in Fig. S4E.†
3.5. Electrochemical detection of AFM1
Control experiments were performed by gradually integrating Ag2O/g-C3N4, Ag2O/g-C3N4-COOH and Ag2O/g-C3N4-COOH@MA-DBB-COF on PGE, as shown in Fig. S1.† The output signal at +0.14 V attributed to Ag in Ag2O/g-C3N4 decreased upon functionalization due to the repulsion caused by the negative charge of the carboxylic group. In contrast, a substantial increase in the output signal was observed after the deposition of MA-DBB-COF on PGE/Ag2O/g-C3N4-COOH, as shown in Fig. S1(c).† Subsequently, the cross-linker EDC-NHS was used to activate the –COOH group, followed by covalent immobilization of anti-AFM1 antibody, as shown in Fig. S2.†,59
The designed PGE/Ag2O/g-C3N4-COOH@MA-DBB-COF/EDC-NHS/anti-AFM1 antibody immunosensor was exposed to different concentrations of AFM1 to monitor the binding between the anti-AFM1-antibody-modified probe and AFM1. Upon the addition of AFM1, there was a substantial decrease in the oxidation signal representing AFM1 binding on the transducer surface. The results confirmed that the greater the amount of AFM1 bound to immunosensor, the smaller the oxidation signal of the designed immunosensor. Hence, there was a decrease in the number of active sites, and the oxidation current peak at 0.13 V was decreased by increasing the amount of AFM1, as shown in Fig. 3A. Thus, there exists a correlation between the concentration of AFM1 and the variation in the oxidation peak intensity. Different known concentrations of AFM1 (0.03–1000 fg mL−1) were assessed, and DPV was employed for recording the response. A substantial decrease in the electrical response with increasing concentration is shown in Fig. 3A. The decrease in the oxidation peak is proportional to the increasing concentration of AFM1. Therefore, the designed immunosensor can be employed for quantitative analysis. The obtained LOD was 0.01 fg mL−1 with a linear range of 0.03–1000 fg mL−1 for AFM1, as depicted in Fig. 3A and B, representing the excellent viability of the designed sensor. A comparison of the analytical performance of the BSA/anti-AFM1 antibody/EDC-NHS/Ag2O/g-C3N4-COOH@MA-DBB-COF/PGE immunosensor with other electrochemical sensors recently reported in the literature for the detection of AFM1 is presented in Table S5.†
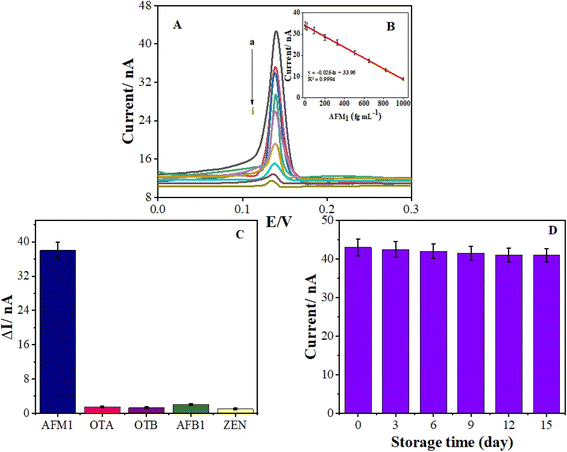 |
| Fig. 3 (A) Differential voltammograms for different concentrations of the analyte: (a) 0.03, (b) 16, (c) 90, (d) 200, (e) 328 (f) 505, (g) 650, (h) 820, and (i) 1000 fg mL−1. (B) Calibration curve for AFM1 concentration. (C) Specificity of the immunosensor and (D) stability of the immunosensor. | |
3.6. Specificity of the AFM1 immunosensor
Intense false electrochemical signals can be generated by nonspecific interactions. Label-free detection methods are affected by these interfering nonspecific interactions. It was necessary to ensure that the change in the oxidation signal was caused entirely by the specific interaction between anti-AFM1 antibody on the transducer surface and AFM1, and not by the non-specific adsorption of AFM1. Thus, the specificity of the designed immunosensor for AFM1 was assessed versus selected interfering mycotoxins such as OTA, OTB, AFB1 and ZEN, as shown in Fig. 3C. The experiment was carried out by incubating the developed immunosensor in concentrations of the interfering analytes that were 10 times higher than the concentration of AFM1. The findings clearly indicate the selective response of the immunosensor PGE/Ag2O/g-C3N4-COOH@MA-DBB-COF/EDC-NHS/anti-AFM1 antibody toward AFM1 analysis.
3.6.1. Reproducibility, accuracy and stability study of AFM1 immunosensor.
As the proposed COF-based electroactive probe is cost effective, the designed immunosensor will be disposable and easy to use. Inter-assay accuracy and precision were used to estimate the reproducibility of the designed immunosensor. Six fabricated electrodes were used to estimate the accuracy and reproducibility of the COF-based nanocomposite probe with a constant concentration of AFM1 under similar experimental conditions. The calculated percentage relative standard deviation (R. S. D. %) values of 1.8% and 1.9% demonstrated the fabrication stability and adequate precision, respectively. With a negligible loss in activity (S. D. 1.6%), the designed immunosensor maintained its electrochemical response over an extended period of time (stored at 4 °C) to serve as a ready-to-use device for single-step analysis of AFM1. Fig. 3D shows the response of the sensor over two weeks (representative); however, response stability was observed up to six months without significant loss in the signal. The extended stability can be attributed to the amide linkage between the –COOH group of the nanocomposite and the terminal –NH2 group of the anti-AFM1 antibody. These findings indicate the excellent stability and reproducibility of the designed transducer surface.
3.7. Milk sample analysis
As an in-field application, the proposed probe was used to detect AFM1 in milk samples. To study the matrix effect, different concentrations (6
420
910 fg mL−1) of AFM1 were spiked into milk samples, and the corresponding recovery values were calculated, as presented in Table 1. The observed recovery percentages were <97%, with R. S. D. values of approximately >2.0% (n = 3), which represents the acceptable accuracy of the proposed immunosensor, as shown in Table 1.
Table 1 Accuracy obtained with the proposed electrochemical immunosensora
Milk sample |
AFM1 added (fg mL−1) |
AFM1 found (fg mL−1) |
R. S. D. % |
R. E. % |
R % |
R. E. % = relative error; R% = recovery percentage.
|
1 |
6 |
5.836 |
1.95 |
2.73% |
97.28% |
2 |
420 |
428.01 |
1.33 |
1.90% |
101% |
3 |
910 |
933.85 |
1.82 |
2.52% |
102.62% |
Furthermore, to validate the performance of designed immunosensor, established HPLC method for the detection of AFM1 was used in parallel. Fig. S5† is the representative chromatogram of standard AFM1 obtained with the established HPLC based method. Twenty different sites were selected in the local market to collect the milk samples for screening of AFM1via immunosensor and HPLC method. Thirteen samples were found AFM1 positive with both the methodologies (0.5–0.8 ng mL−1). While seven were AFM1 negative following HPLC method, however, out of these seven samples, five were AFM1 positive with the designed immunosensor (350–800 fg mL−1). This can be attributed to lower range detection capability of the proposed immunosensor in comparison to that of HPLC based method that permitted to detect lower AFM1 concentrations lower than the range of HPLC based method. The remaining two samples were found AFM1 negative with the proposed immunosensor, and were used for the recovery studies. These results show the successful application of the developed multifunctional electrochemical sensor to screen the contaminated milk samples.
4. Conclusion
This study reports a novel approach for the successful design of an easy to use, one-step, highly sensitive and selective electrochemical immunosensor for the detection of AFM1 in milk samples. The designed sensing platform was composed of a COF-based electroactive hybrid nanocomposite material, Ag2O/g-C3N4-COOH@MA-DBB-COF, fabricated on PGE with an analyte-specific antibody. The COF-based electroactive nanocomposite material served as a multitasking probe offering relative conductivity, immobilization support, and an electrooxidation signal. The principle for the immunosensor design follows the hypothesis of complex formation between anti-AFM1-monoclonal antibody and AFM1 leading to decrease in the oxidation signal through steric crowding. Compared to existing electrochemical immunosensors, the proposed design offers a multifunctional, single-step and highly sensitive sensing platform. The applicability of the immunosensor for AFM1 detection was validated by analysis of local market milk samples. The proposed transducer design can be used as a prototype sensing platform for the inexpensive and specific detection of AFM1 in the screening of milk samples.
Data availability
The data supporting this article have been included as part of the ESI.†
Author contributions
Iram Naz: contributed to writing original draft, study conception, data curation, formal analysis, project administration, validation, and visualization. Akhtar Hayat: contributed to the study conception, formal analysis, funding acquisition, methodology, resources, supervision, writing-review and editing, Farhat Jubeen: contributed to the writing original draft, study conception, data curation, formal analysis, funding acquisition, investigation, project administration, resources, supervision, validation, and visualization. Sadia Asim contributed to the software and formal analysis. All authors read and approved the final manuscript. Abida Kausar: contributed to the software and formal analysis.
Conflicts of interest
The authors declare that they have no known competing interests.
Acknowledgements
The authors of the present study are highly thankful for financial assistance from the (i) Ministry of Science and Technology, Government of Pakistan for the developmental grant entitled as “Establishment of Center for Advance Technologies in Biomedical Material” under its knowledge economy initiative and (ii) Higher Education Commission of Pakistan through 8966/Federal/NRPU/R&D/HEC/2017.
References
- F. Gaucheron, J. Am. Coll. Nutr., 2011, 30, 400s–409s CrossRef CAS PubMed
.
-
B. Grenov, A. Larnkjær, C. Mølgaard and K. F. Michaelsen, in Global Landscape of Nutrition Challenges in Infants and Children: 93rd Nestlé Nutrition Institute Workshop March 2019, ed. K. F. Michaelsen, L. M. Neufeld, A. M. Prentice and S. Karger, AG, Kolkata, 2020, vol. 93 Search PubMed
.
- V. Awasthi, S. Bahman, L. K. Thakur, S. K. Singh, A. Dua and S. Ganguly, Indian J. Public Health, 2012, 56, 95–99 CrossRef PubMed
.
- S. Firdous, A. Ashfaq, S. J. Khan and N. Khan, Food additives & contaminants. Part B, Surveillance, 2014, 7, 95–98 CAS
.
- N. Ali, Toxicol Rep, 2019, 6, 1188–1197 CrossRef CAS PubMed
.
- A. M. Macri, I. Pop, D. Simeanu, D. Toma, I. Sandu, L. L. Pavel and O. S. Mintas, Microorganisms, 2020, 9, 61 CrossRef PubMed
.
- I. Naeem, A. Ismail, A. U. Rehman, Z. Ismail, S. Saima, A. Naz, A. Faraz, C. A. F. de Oliveira, N. Benkerroum, M. Z. Aslam and R. Aslam, Int. J. Environ. Res. Public Health, 2022, 19, 3404 CrossRef PubMed
.
- P. Khazaeli, M. Mehrabani, M. R. Heidari, G. Asadikaram and M. Lari Najafi, Iran. J. Public Health, 2017, 46, 1540–1545 Search PubMed
.
- R. Shadjou, M. Hasanzadeh, M. Heidar-Poor and N. Shadjou, J. Mol. Recognit., 2018, 31, e2699 CrossRef PubMed
.
- S. Marchese, A. Polo, A. Ariano, S. Velotto, S. Costantini and L. Severino, Toxins, 2018, 10, 214 CrossRef PubMed
.
- Q. Chen, M. Meng, W. Li, Y. Xiong, Y. Fang and Q. Lin, Food Chem., 2023, 398, 133848 CrossRef CAS PubMed
.
- P. Malik, R. Gupta, V. Malik and R. K. Ameta, Meas.: Sens., 2021, 16, 100050 Search PubMed
.
- A. A. Ismaiel, N. A. Tharwat, M. A. Sayed and S. A. Gameh, J. Food Sci. Technol., 2020, 57, 2182–2189 CrossRef CAS PubMed
.
- B. D. Abera, A. Falco, P. Ibba, G. Cantarella, L. Petti and P. Lugli, Sensors, 2019, 19, 3912 CrossRef CAS PubMed
.
- S. S. Omar, Ital. J. Food Saf., 2016, 5, 5788 Search PubMed
.
- A. M. Zakaria, Y. A. Amin, O. S. F. Khalil, E. Y. Abdelhiee and M. M. Elkamshishi, J. Adv. Vet. Anim. Res., 2019, 6, 197–201 CrossRef PubMed
.
- J. Ramesh, S. Ghadevaru and V. Sureshkumar, Int. J. Curr. Microbiol. Appl. Sci., 2013, 2, 373–377 Search PubMed
.
- F. Jubeen, N. Zahra, Z. I. Nazli, M. K. Saleemi, F. Aslam, I. Naz, L. B. Farhat, A. Saleh, S. Z. Alshawwa and M. Iqbal, Toxins, 2022, 14, 547 CrossRef CAS PubMed
.
-
A. Mateen, in Aflatoxins, ed. A. U. Lukman Bola, IntechOpen, Rijeka, 2021, Ch. 10, DOI:10.5772/intechopen.98508
.
- S. C. Pei, Y. Y. Zhang, S. A. Eremin and W. J. Lee, Food Control, 2009, 20, 1080–1085 CrossRef CAS
.
- S. F. Ahmadi, M. Hojjatoleslamy, H. Kiani and H. Molavi, Food Chem., 2022, 373, 131321 CrossRef CAS PubMed
.
- Y.-H. Pang, L.-L. Guo, X.-F. Shen, N.-C. Yang and C. Yang, Electrochim. Acta, 2020, 341, 136055 CrossRef CAS
.
- N. Tarannum, M. N. Nipa, S. Das and S. Parveen, Toxicol Rep, 2020, 7, 1339–1343 CrossRef CAS PubMed
.
- X. Zhang, C.-R. Li, W.-C. Wang, J. Xue, Y.-L. Huang, X.-X. Yang, B. Tan, X.-P. Zhou, C. Shao, S.-J. Ding and J.-F. Qiu, Food Chem., 2016, 192, 197–202 CrossRef CAS PubMed
.
- E. C. Dreaden, A. M. Alkilany, X. Huang, C. J. Murphy and M. A. El-Sayed, Chem. Soc. Rev., 2012, 41, 2740–2779 RSC
.
- K. Abhijith, K. Ragavan and M. Thakur, Anal. Methods, 2013, 5, 4838–4845 RSC
.
- M. Tudorache and C. Bala, Sensors, 2008, 8, 7571–7580 CrossRef CAS PubMed
.
- S. Srivastava, V. Kumar, K. Arora, C. Singh, M. A. Ali, N. K. Puri and B. D. Malhotra, RSC Adv., 2016, 6, 56518–56526 RSC
.
- N. Gan, J. Zhou, P. Xiong, F. Hu, Y. Cao, T. Li and Q. Jiang, Toxins, 2013, 5, 865–883 CrossRef CAS PubMed
.
- G. Li, C. Liu, X. Zhang, P. Luo, G. Lin and W. Jiang, Food Chem., 2021, 355, 129443 CrossRef CAS PubMed
.
- X. Tang, G. Catanante, X. Huang, J.-L. Marty, H. Wang, Q. Zhang and P. Li, Food Chem., 2022, 383, 132598 CrossRef CAS PubMed
.
-
S. Mousumi, in Nanotechnology and the Environment, ed. S. Mousumi, IntechOpen, Rijeka, 2020, Ch. 6, DOI:10.5772/intechopen.93047
.
-
J. Hári and B. Pukánszky, in Applied Plastics Engineering Handbook, ed. M. Kutz, William Andrew Publishing, Oxford, 2011, pp. 109–142, DOI:10.1016/B978-1-4377-3514-7.10008-X
.
- X. An, X. Shi, H. Zhang, Y. Yao, G. Wang, Q. Yang, L. Xia and X. Sun, New J. Chem., 2020, 44, 1362–1370 RSC
.
- G. Kaur, S. Sharma, S. Singh, N. Bhardwaj and A. Deep, ACS Omega, 2022, 7, 17600–17608 CrossRef CAS PubMed
.
- J. Zhu, P. Xiao, H. Li and S. A. C. Carabineiro, ACS Appl. Mater. Interfaces, 2014, 6, 16449–16465 CrossRef CAS PubMed
.
- J. Low, B. Cheng, J. Yu and M. Jaroniec, Energy Storage Mater., 2016, 3, 24–35 CrossRef
.
- Q. Zhao, W. Wu, X. Wei, J. Shunli, T. Zhou, Q. Li and Q. Lu, Sens. Actuators, B, 2017, 248, 673–681 CrossRef CAS
.
- N. Mekgoe, N. Mabuba and K. Pillay, Front. sens., 2022, 3, 827954 CrossRef
.
- H. Zhang, Q. Huang, Y. Huang, F. Li, W. Zhang, C. Wei, J. Chen, P. Dai, L. Huang, Z. Huang, L. Kang, S. Hu and A. Hao, Electrochim. Acta, 2014, 142, 125–131 CrossRef CAS
.
- S. Munusamy, K. Sivaranjan, P. Sabhapathy, V. Narayanan, F. Mohammad and S. Sagadevan, Synth. Met., 2021, 272, 116669 CrossRef CAS
.
- M. Afshari, M. Dinari and M. M. Momeni, J. Electroanal. Chem., 2019, 833, 9–16 CrossRef CAS
.
- S. Vinoth, K. S. Shalini Devi and A. Pandikumar, TrAC, Trends Anal. Chem., 2021, 140, 116274 CrossRef CAS
.
- V. Biju, Chem. Soc. Rev., 2014, 43, 744–764 RSC
.
- M. Holzinger, A. Le Goff and S. Cosnier, Front. Chem., 2014, 2, 63 Search PubMed
.
- S. Chen, B. Yuan, G. Liu and D. Zhang, Front. Chem., 2020, 8, 601044 CrossRef CAS PubMed
.
- A. Ahmed, A. Hayat, M. H. Nawaz, P. John and M. Nasir, J. Colloid Interface Sci., 2020, 558, 230–241 CrossRef PubMed
.
- S. Rauf, G. K. Mishra, J. Azhar, R. K. Mishra, K. Y. Goud, M. A. H. Nawaz, J. L. Marty and A. Hayat, Anal. Biochem., 2018, 545, 13–19 CrossRef CAS PubMed
.
- N. Sahiner, S. Demirci and K. Sel, J. Porous Mater., 2016, 23, 1025–1035 CrossRef CAS
.
- J. Liu, T. Zhang, Z. Wang, G. Dawson and W. Chen, J. Mater. Chem., 2011, 21, 14398–14401 RSC
.
- B. Fahimirad, A. Asghari and M. Rajabi, Microchim. Acta, 2017, 184, 3027–3035 CrossRef CAS
.
- A. Ahmed, A. Hayat, M. H. Nawaz, P. John and M. Nasir, J. Colloid Interface Sci., 2020, 558, 230–241 CrossRef PubMed
.
- A. Ahmed, A. Hayat, P. John, M. H. Nawaz and M. Nasir, J. Nanostruct. Chem., 2021, 11, 675–691 CrossRef CAS
.
- H.-T. Ren, S.-Y. Jia, Y. Wu, S.-H. Wu, T.-H. Zhang and X. Han, Ind. Eng. Chem. Res., 2014, 53, 17645–17653 CrossRef CAS
.
- S. Vignesh, P. Eniya, M. Srinivasan, J. Kalyana Sundar, H. Li, S. Jayavel, M. Pandiaraman, M. A. Manthrammel, M. Shkir and B. Palanivel, J. Environ. Chem. Eng., 2021, 9, 105996 CrossRef CAS
.
- L. D. Chakkarapani, Z. Bytešníková, L. Richtera and M. Brandl, Appl. Mater. Today, 2024, 37, 102113 CrossRef
.
- S. I. Khan, A. Hassan, R. Bano, M. A. Gilani, J. L. Marty, H. Zhang and A. Hayat, Talanta, 2024, 267, 125233 CrossRef CAS PubMed
.
- W. Wei, S. Zhou, D. D. Ma, Q. Li, M. Ran, X. Li, X. T. Wu and Q. L. Zhu, Adv. Funct. Mater., 2023, 33, 2302917 CrossRef CAS
.
- A. Hayat, L. Barthelmebs and J.-L. Marty, Sens. Actuators, B, 2012, 171–172, 810–815 CrossRef CAS
.
|
This journal is © The Royal Society of Chemistry 2024 |