DOI:
10.1039/D4NA00285G
(Review Article)
Nanoscale Adv., 2024,
6, 4275-4308
Smart delivery vehicles for cancer: categories, unique roles and therapeutic strategies
Received
4th April 2024
, Accepted 19th June 2024
First published on 20th June 2024
Abstract
Chemotherapy and surgery remain the primary treatment modalities for cancers; however, these techniques have drawbacks, such as cancer recurrence and toxic side effects, necessitating more efficient cancer treatment strategies. Recent advancements in research and medical technology have provided novel insights and expanded our understanding of cancer development; consequently, scholars have investigated several delivery vehicles for cancer therapy to improve the efficiency of cancer treatment and patient outcomes. Herein, we summarize several types of smart therapeutic carriers and elaborate on the mechanism underlying drug delivery. We reveal the advantages of smart therapeutic carriers for cancer treatment, focus on their effectiveness in cancer immunotherapy, and discuss the application of smart cancer therapy vehicles in combination with other emerging therapeutic strategies for cancer treatment. Finally, we summarize the bottlenecks encountered in the development of smart cancer therapeutic vehicles and suggest directions for future research. This review will promote progress in smart cancer therapy and facilitate related research.
1. Introduction
Cancer is the leading cause of mortality worldwide.1 The World Health Organization estimated that the number of cancer-related deaths will increase by 2030.2 Therefore, effective treatment of cancers remains urgently needed.
Surgery, radiotherapy, and chemotherapy are the first-line treatment options for most cancers.3 Conventional chemotherapy, a fundamental approach to cancer treatment, distributes drugs through the bloodstream to various organs, where it interferes with DNA synthesis and mitosis in rapidly proliferating cells and causes cell-cycle arrest.4,5 However, chemotherapy is associated with multidrug resistance (MDR), nonspecific drug distribution, and systemic toxicities.6 Chemotherapeutic drugs are non-selective; their cytotoxic effects can damage healthy tissue cells, leading to adverse toxic effects, such as cardiotoxicity in the case of adriamycin7 or hepatotoxicity in the case of camptothecin.8 In addition, conventional chemotherapeutic drugs are less bioaccessible to cancer tissues; therefore, high dosages are required, which in turn produces toxicity in normal cells and increases the likelihood of multi-drug resistance.9 The efficacy of cancer therapy is influenced by drug tolerance, effective drug delivery, and duration of drug action, among others, which considerably restrict its application.10 Consequently, conventional cancer treatments are associated with disadvantages, such as difficulty in achieving treatment, cancer recurrence, and side effects.11 Despite considerable advances in cancer treatment, cancer-related morbidity and mortality rates continue to increase.12 According to statistics, the age-standardized cancer incidence rate is 201.7/100
000 in China, 319.2/100
000 in the United Kingdom, and 352.2/100
000 in the United States. At the same time, the cancer mortality rate is 130.1 per 100
000 in China, 102.6 per 100
000 in the United Kingdom, and 91.0 per 100
000 in the United States.13 Therefore, highly effective and less toxic strategies that can differentiate between cancer and normal cells, selectively target cancer tissue, and respond “intelligently” to the complex microenvironment of the cancer are warranted.
Precise cancer therapeutic strategies have been developed to improve clinical outcomes. Nanotechnology has great potential to improve the clinical outcomes for various diseases, including cancer.14–16
Among them, the development and application of various smart cancer drug delivery vehicles, including polymers,17 liposomes,18 inorganic carriers,19 and polymeric hydrogels,20 have greatly compensated for the limitations in conventional cancer treatments. For example, smart cancer drug delivery vehicles can increase the targeting of tumors through functionalized modifications, thereby enabling drug accumulation in tumors,21 improving the stability of therapeutic drugs in vivo, and reducing drug resistance.22 In addition, while improving therapeutic efficacy, smart cancer drug delivery vehicles can accurately monitor tumor-related biomarkers, which is conducive to the early diagnosis of tumors.23 More importantly, novel therapeutic strategies, such as photothermal therapy, photodynamic therapy (PDT), gene therapy, and hormone therapy, are minimally invasive, if at all, and have demonstrated good potential for cancer treatment and prevention in preclinical studies.24,25 However, photosensitizers are susceptible to self-extinction during delivery and generate reactive oxygen species (ROS) with a small diffusion radius, thereby hampering the anti-tumor effect of PDT. The application of smart cancer delivery vehicles can overcome these limitations,26 and, when combined with different treatment methods, enhance the ability to kill cancer cells through synergistic effects.27,28 Currently, several nanoparticle-based chemotherapeutic agents have been clinically approved,29,30 and novel chemotherapeutic drugs are at different stages of preclinical development. Therefore, the development of smart cancer delivery systems with better targeting capabilities, longer blood circulation times, and the possibility of combination therapy is important.
In this review, we discuss various smart delivery vehicles currently used in cancer therapy and their advantages, focusing on their role in facilitating cancer immunotherapy and the current challenges in their application (Fig. 1). Our findings will help to facilitate future clinical translation and propose new directions for further advancements in anti-cancer therapy.
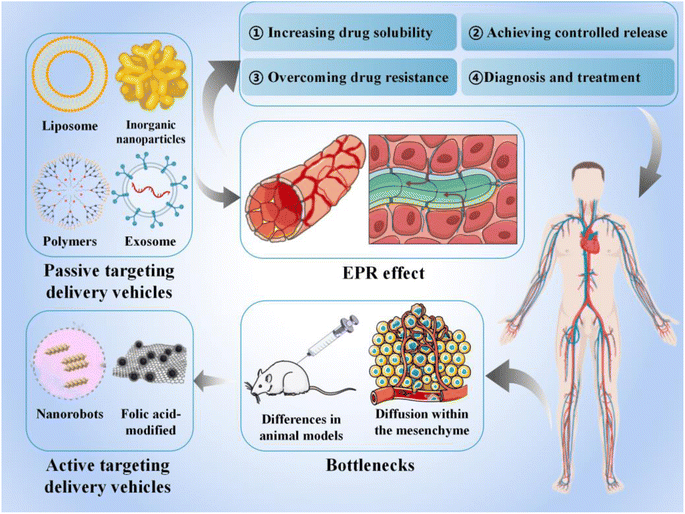 |
| Fig. 1 Schematic diagram of the application of smart delivery vehicles in cancer treatment. | |
2. Status of research on smart delivery vehicles
Traditional administration methods involve non-specific biological distribution and arbitrary drug release. To improve treatment efficiency and reduce related side effects, drugs should be released in a controlled manner at specific sites of action. In this review, smart delivery vehicles are defined as a type of tool that can deliver drugs to the target site and control drug release to “intelligently” exert their effects, thereby improving treatment efficiency and reducing drug toxicity.
2.1 Passive targeting-based delivery vehicles
Passive targeting-based therapeutic vehicles loaded with therapeutic agents are widely used in various cancer treatments (Table 1).
Table 1 Summary of commonly used passive smart therapy vehicles in cancer
Type of delivery vehicles |
Loaded drugs |
Cancer |
Mechanisms |
References |
Liposomes |
Doxorubicin (DOX) |
Colorectal cancer |
Peptide connectors respond to upregulated MMP-2 in the cancer microenvironment and enhance the capture of liposomes |
31
|
|
Oxaliplatin and antisense lncRNA of MDC1 |
Cervical cancer |
Thermosensitive release of OXA with enhanced inhibition of cervical cancer cells by co-delivery |
32
|
|
Anti-STAT3 siRNA |
Melanoma |
Enhances cell internalization and cytotoxicity, induces apoptosis, and significantly inhibits the STAT10 gene |
33
|
|
Oxaliplatin and paclitaxel |
Ovarian cancer |
Better specific targeting ability, anti-tumor proliferative effects and prolonged drug half-life |
34
|
|
Irinotecan (IRI) and doxorubicin |
Breast cancer |
Improves drug loading and stability and promotes drug synergism through co-loading |
35
|
|
DOX |
Increases affinity for the cell membrane, thereby facilitating drug release and entry to the nucleus of the tumor cell and avoiding lysosomal capture |
36
|
Polymeric nanoparticles |
Doxorubicin, 5-fluorouracil, and methotrexate |
Triple-negative breast cancer (TNBC) |
Ruthenium in the dendrimer structure has anti-cancer effects and can form stable nanocomposites with drugs |
37
|
|
— |
Chronic lymphocytic leukemia |
Inhibits the proliferation of leukemia cells and promotes cell apoptosis |
38
|
|
Contrast agents (CAs) |
Murine glioblastoma |
Crosses the blood–brain barrier (BBB), enhances tumor contrast and significantly reduces toxicity |
39
|
|
Gemcitabine (Gem) |
Pancreatic cancer |
Stable formulation with pH-responsive drug release, effective accumulation at the tumor site and rapid cellular uptake |
40
|
Inorganic nanoparticles |
Cancer-penetrating peptide (TPP) |
TNBC |
Induces apoptosis by increasing ROS |
41
|
|
Gemcitabine |
Liver and pancreatic cancer |
Improves targeting and increases synergy |
42
|
|
TK-p53-NTR and microRNA |
Lung cancer |
Improves gene transfection rates |
43
|
|
Methotrexate (MTX) |
A-375 cancer cell line |
Controls drug release and increases selectivity for tumor cells |
44
|
|
— |
Cervical cancer |
Cytotoxicity to tumor cells in a dose-dependent manner and induction of apoptosis |
45
|
Polymeric micelles |
TPL-NSA |
Gastric cancer |
Reduces the expression of collagen, FAP, and α-smooth muscle actin in cancers |
46
|
|
Paclitaxel (PTX), etoposide (ETP), and rapamycin (RAPA) |
The pH-sensitive property was utilized to effectively control drug release in tumor cells and improve the water solubility of the drug |
47
|
|
Taxotere (DTX) |
Hepatocellular carcinoma |
Overcomes solubility and anti-proliferative activity and inhibits ascites production |
48
|
|
Anti-KRAS antibodies (KRAS-Ab) |
Pancreatic and colorectal cancers |
Blocks the overactivation of the KRAS-related cascade and recovers the influence of its mutation |
49
|
|
Containing camphor sulfonamide (DK164) |
Breast and lung cancer |
Higher stability and cellular uptake for improved anti-cancer properties while maintaining drug activity |
50
|
|
2,6-Bis((3-methoxy-4-hydroxyphenyl) methylene) cyclohexanone |
Colon cancer |
Higher selective cytotoxicity against tumor cells, arresting cell growth at the G2/M phase and inducing apoptosis earlier |
51
|
Exosomes |
Paclitaxel |
MDR cancer |
High loading efficiency and sustained drug release, resulting in more than a 50-fold increase in cytotoxicity |
52
|
|
HChrR6-encoding mRNA |
HER2 human breast cancer |
Confines HChrR6 generation and CNOB activation to the cancer |
53
|
|
lncRNA MEG3 |
Osteosarcoma (OS) |
Improves anti-cancer properties |
54
|
|
Rifampicin (RIF) |
Accelerates entry of rifampicin into OS cells, stalls the cell cycle in the G2/M phase and leads to mitochondrial cleavage and apoptosis |
55
|
|
CaCO3NPs and Cur |
Colon cancer |
CaCO3NPs with homologous targeting ability improves drug accumulation, and releases Ca2+ to disrupt mitochondria and induce oxidative stress |
56
|
|
Triptolide (TP) |
Melanoma |
Antiproliferative, anti-invasive, and pro-apoptotic; prolongs half-life of TP |
57
|
|
Hyaluronan (HA) |
Human prostate cancer cell line PC3 |
Reduces the number of associated immunosuppressive immune cells and hyaluronidase-induced tumor cell metastasis |
58
|
|
IL-12 |
B16F10 and MC38 cell lines |
Prolongs IL-12 retention and long-lasting immune memory |
59
|
2.1.1 Liposomes.
Smart delivery systems based on liposomes aid in advanced cancer therapy.60 Owing to their biodegradable nature, they can be loaded with biomolecules with different properties, such as RNA, peptides, and proteins, without altering their original features. Thus, liposomes have become the most widely used carriers in cancer therapy and the first therapeutic nanoparticles approved for clinical treatment.61
Liposomes are spherical lipid vesicles composed of phospholipids with a bilayered structure.62 Since their discovery in 1965, they have become versatile therapeutic carriers owing to their superior biocompatibility and biodegradability, as well as their unique ability to encapsulate hydrophobic drugs. In addition, liposomes offer controlled drug release, low toxicity, and good biocompatibility and can avoid drug leakage.63 However, liposomes exhibit high uptake mainly by the liver and spleen;64 therefore, different surface modifications have been applied to increase the circulation time of liposomes and improve the efficiency of chemotherapeutic drugs.65
Xie et al. used polyethylene glycol-modified liposome surfaces, followed by binding to estrone (ES-SSL), to deliver chemotherapeutic drugs to ovarian cancer cells with high expression of estrogen receptors. The authors reported a prolonged drug half-life, slowed clearance, and 85.24% cancer inhibition.34 Irinotecan (IRI) and DOX are often combined in cancer treatments; Liu et al. constructed a novel liposome carrier for the co-delivery of IRI and DOX using the triethyl octasulfate sucrose gradient loading method. The co-delivery of liposomes maintains the optimal proportion of drug action and increases the distribution of the two drugs in cancer tissues. In addition, co-loaded liposomes exhibited a stronger anti-cancer effect on 4T-1 breast cancer xenotransplantation compared to a mixture of single-loaded liposomes.35
Stealth magnetic liposomes containing calcium-substituted magnesium ferrite NPs have been used as nanocarriers for curcumin delivery, and showed superparamagnetic properties, targeted cancer sites, and offered combined effects, such as magnetic heat and drug release.66 The release of liposome-loaded drugs can be triggered by external factors, such as heat, light, and magnetic fields. Lipid bilayer-loaded protoporphyrin IX (PpIX), a hydrophobic photosensitizer, promotes the nuclear delivery of DOX and has a greater affinity for cytoplasmic membranes than liposome carriers. Such a feature encourages its separation from liposomes upon encountering cancer cells, thereby triggering the effective release of DOX and facilitating its entry into the nucleus of breast cancer cells and avoiding lysosomal degradation (Fig. 2A).36 Based on the well-established use of liposomes in cancer therapy, Chen et al. developed a novel liposome drug delivery system that mimics viruses and used a self-assembled liposome bilayer structure to mimic the viral envelope and a loaded drug to mimic the viral genome. The structure and concentration of the adsorbed polymers were adjusted to control drug release from liposomes. Owing to their ability to bypass the efflux mechanism, enhance the uptake of target cells, and provide effective internal body escape, treatment vehicle systems have demonstrated efficacy against various drug-resistant cancer cells such as HeLa cervical cancer, A549 lung cancer, MES-SA uterine cancer, and MES-SA/DX5 multidrug-resistant cancer cells (Fig. 2B).67 Stereochemistry can affect the biological properties of liposomes. Designing liposomes by stereospecific ionization of lipids can increase the efficiency of their mediated mRNA delivery. A novel C12-200 (stereospecific derivative)-S LNP was designed to deliver mRNA 3.8 times more efficiently than its racemate.68
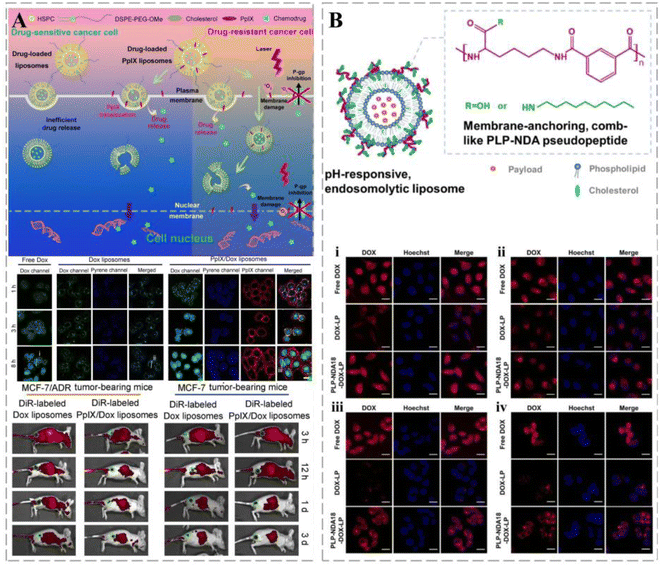 |
| Fig. 2 Liposomes in cancer therapy. (A) Schematic illustration of the plasma membrane-activatable drug release and plasma membrane-based PDT for MDR reversal. PpIX/DOX liposomes appear rapidly in cancer-bearing mice and last for more than three days. Reprinted with permission from ref. 36. (B) Schematic diagram of pH-responsive endolysis of liposomes. The intensity of red fluorescence in HeLa cells (i), A549 cells (ii), MES-SA cells (iii), and MES-SA/DX5 cells (iv) was significantly reduced after internalization of DOX-loaded naked liposomes by endocytosis. Reprinted with permission from ref. 67. | |
2.1.2 Polymeric nanoparticles.
Polymeric nanoparticles (PNPs) are polymeric particles with a size range of 1–1000 nm69 and different structures, such as nanocapsules, nanospheres, micelles, and dendritic polymers.70 Most materials used in PNPs have good biocompatibility and are approved by the United States Food and Drug Administration (FDA).71 Various small molecules, such as RNA and proteins, modify the surface of PNPs to confer different functions. In addition to targeting the delivery of loaded drugs, PNPs prevent phagocytosis by the phagocytic system and adverse reactions at other sites.72
In addition, PNP-based drug-delivery systems can control the release rate of drugs by altering stimulus-responsive systems, such as pH and magnetic thermal environments, to prolong the duration of action in target regions (Fig. 3A).73 Based on the plasticity of PNP surfaces and structures, the development of PNPs with various functions for drug loading and their application in cancer-targeted therapies have been investigated.
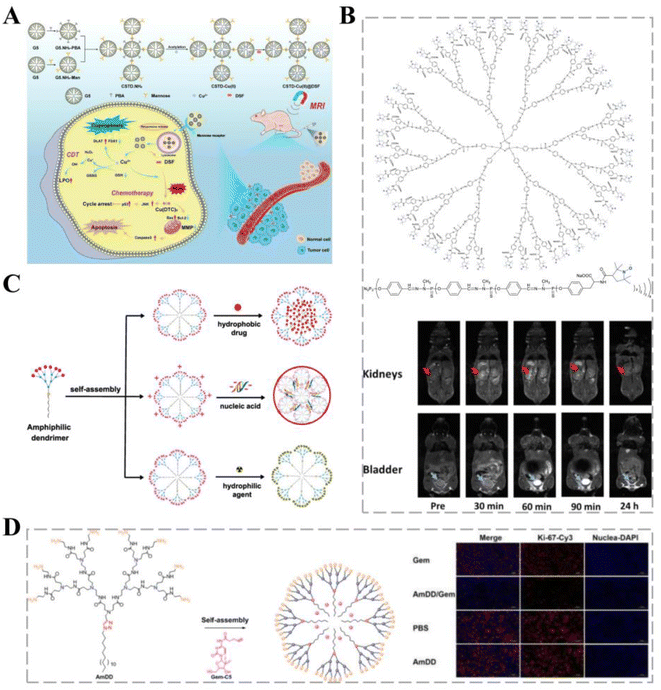 |
| Fig. 3 Dendritic polymers for cancer therapy. (A) A novel pH-responsive formulation consisting of PCAD-DMSN@DOX in which polycarboxylic acid dextran (PCAD) is electrostatically attached to the DMSN@DOX surface. Covalent coupling of CD133-RNA aptamers to the PCAD-DMSN nanoparticle surface results in specific translocation of the encapsulated anti-cancer drugs to CD133-overexpressing cancer cells. Reprinted with permission from ref. 73. (B) Structure of the G3-Tyr-PROXYL-ONa radical dendrimer. Reprinted with permission from ref. 39. (C) Self-assembly of small amphiphilic dendrimers into supramolecular dendrimers mimicking covalent construction of dendrimers. Reprinted with permission from ref. 80. (D) Self-assembling amphiphilic dendritic polymers for drug encapsulation. Immunohistochemical analysis using Ki-67, a tumor cell proliferation marker, showed that Ki-67 expression in tumor cells of AmDD/Gem-treated PC tumor-bearing mice was lower than that in the Gem group. | |
Dendritic polymers are hyperbranched polymers with well-defined structures comprising cores, branches, gaps, and terminal groups.74 Dendritic polymers have better physicochemical behavior than straight or branched polymers, allowing for a wide range of applications, including as adjuvants for vaccine antigens75 or as modified contrast agents (CAs).76 The unique properties of dendritic macromolecules, such as uniform size and size distribution, spherical design, high branching, and functional surfaces, make them effective carriers for drug delivery.77,78
Magnetic resonance imaging (MRI) can be used for the early diagnosis of cancers and is particularly important for brain cancers. Paramagnetic gadolinium-based CAs are the most widely used for MRI acquisition in the brain; however, they are associated with potentially fatal nephrogenic systemic fibrosis.79 Organic free radicals fixed to the surfaces of dendritic macromolecules have paramagnetic properties, thereby reducing the accumulation of toxic metals, and can serve as CAs for T1CA imaging. Zhang et al. investigated a third-generation water-soluble family of poly(phosphorhydrazone) radical dendrimers and developed G3-Tyr-PROXYL-ONa radical dendrimers, offering a viable alternative to metal-based MRI CAs (Fig. 3B). In a mouse glioblastoma model, carriers loaded with less than four times the administered clinical dose showed appropriate contrast enhancement and selective accumulation in the brain cancer tissue, remaining within cancer tissue and allowing image acquisition over a longer period.39
Self-assembled small amphiphilic dendrimers exhibit lipid self-assembly abilities combined with the specific structure and stability of dendrimers, allowing for high drug-loading capacity while maintaining a small size and stable formulation (Fig. 3C).80 The efficacy of gemcitabine (Gem) is mainly limited by its unstable metabolism and poor cellular uptake; therefore, higher doses of Gem are administered to improve efficacy, leading to severe systemic toxicity.81 Zhao et al. first synthesized an aliphatic Gem prodrug and encapsulated it into a small amphiphilic dendritic polymer that could self-assemble into nano-micelles in water. Nano-formulations provide significant advantages, such as excellent stability to protect the loaded drug from early release, maintenance of their small size for effective accumulation at the cancer site, and effective pH-responsive drug release to increase the drug concentration at cancer sites. Dendrimer carriers have shown more potent anti-cancer activity in vitro and in vivo and considerably fewer adverse effects than free Gem (Fig. 3D).40
2.1.3 Inorganic nanoparticles.
Inorganic nanoparticles (INPs), synthesized from inorganic particles and biodegradable polycations, including metals, metal oxides, carbon materials, and mesoporous silica nanoparticles, have various sizes and shapes and a range of physical properties induced by the quantum nature of their core materials. INP-based drug delivery systems have the advantages of wide surface area coupling chemistry and multifunctional surface functionalization, which provide new strategies for designing novel therapeutic and imaging agents.82
Since entering clinical trials, metal NPs have been widely used as probes for observing cell components under electron microscopes to detect markers83 and as carriers for drug delivery.84 Metal–organic framework nanoparticles (MOF-NPs) are crystalline hybrid microporous or mesoporous nanomaterials with significant potential in biomedicine owing to their drug loading and controlled release properties. Porous capsules are prepared from MIL-100 carboxylate iron nanoparticles via low-temperature spray drying, allowing for MTX encapsulation in the pores of MOF-NPs during pod formation at a high loading. Collagenase (COL) was packaged in a specific mesoporous cavity in a pot to enhance cancer treatment. Compared with naked MOF-NPs, this binding offers enhanced controlled release of the active components, MTX and COL, under simulated body fluid conditions. In addition, the selective toxicity of loaded MIL-100 capsules to A-375 cancer cells was nine times higher than that of normal HaCaT cells, indicating that the capsules could be used for the selective treatment of cancer cells.44 Zinc ligand polymers are novel drug delivery vehicles that can protonate the ligand bonds of zinc-based organic ligand polymers in a slightly acidic cancer environment to achieve targeted drug release, exhibiting great potential for application in cancer treatment.85 Green-synthesized ZnO nanoparticles exhibit significant cytotoxicity against SiHa cancer cell lines and improve the efficiency of treatment for cervical cancer.45
Mesoporous silica nanoparticles (MSNPs) are commonly used inorganic non-metallic nanoparticles with superior biosafety to metal nanoparticles, higher drug loading, and a faster dissolution rate.86
Gallbladder cancer (GBC) manifests via non-specific symptoms early in the course of the disease and is often diagnosed at advanced stages. GBC is chemo-resistant, leading to poor clinical outcomes. An electrochemical probe constructed on SiO2 nanoparticles with ENPP1 and EpCAM as dual targets has been shown to specifically detect circulating cancer cells (CTCs) in GBC and enable a more rapid and sensitive diagnosis of GBC and determination of chemoresistance than traditional invasive tissue biopsy.87 mRNA is an unstable large molecule with very low in vitro effectiveness.88 Dong et al. varied the size, porosity, surface topography, length, and width of MSNPs to optimize their effectiveness in delivering mRNA. The vehicle could achieve effective cellular uptake and intracellular escape in animal models, remain stable and active for a long time, and achieve tissue-specific mRNA expression.89
2.1.4 Polymer micelles.
Micelles are nanoscale systems of spherical or globular colloids formed by the self-assembly of amphiphilic block copolymers in aqueous solutions90 with a hydrophobic core and a hydrophilic shell. Hydrophobic cores are used to store hydrophobic drugs, whereas hydrophilic shells enhance the solubility of the polymers and hydrophobic drugs in water.91 Drugs bind to polymer micelles through chemical, physical, or electrostatic interactions. Polymeric micelle nanoparticles (PM-NPs) have been used as paclitaxel (PTX) nanocarrier platforms, showing good clinical performance.92 Genenaxel PM (PEG-poly(D,L-propanediol)-paclitaxel) is the first PM preparation of PTX; it does not contain cremophor and exhibits good therapeutic efficacy and safety in advanced refractory malignant cancers.93 pH-sensitive PMs maintain a stable state in normal tissues; however, upon reaching the cancer site, they become unstable and release the encapsulated drug in response to the low pH in cancer tissue.94 Thus, pH-sensitive PMs can exploit the pH difference between the cancer and normal tissues to trigger drug release. Methoxypolyethylene glycol-b-poly(ε-caprolactone; mPEG-b-PCL) consists of a hydrophilic PEG shell and a hydrophobic PCL core, with the shell connected to the core via citraconic anhydride.95
pH-sensitive mPEG-pH-PCL copolymer micelles exhibit high stability and sustained release as carriers loaded with PTX, etoposide (ETP), and rapamycin (RAPA), exploiting the low pH of the cancer microenvironment to disrupt citraconic amide bonds for rapid drug release.47 By embedding hydrophobic bioactive substances in PEG, Schröder developed a novel micellar form, 113-b-P(CyCL3-co-CL46)-B-PEO113, based on triblock copolymer micelles of ferrocene-containing camphorsulfonamide DK164. The drug-loaded micelles are stable in aqueous media and have high encapsulation efficiency and sustained-release properties.50 Sripetthong prepared nanomicelles loaded with curcumin analogs for colon cancer chemotherapy. CL-NBSCh showed considerable selective cytotoxicity against human colon cancer mucosal epithelial cells (HT-29). In addition, CL-NBSCh micelles more effectively induced cell growth arrest at the G2/M phase and induced apoptosis earlier in HT-29 cells than free CL.51
2.1.5 Exosomes.
Exosomes (Exos), extracellular vesicles (EVs) secreted by mesenchymal stem cells (MSCs), are produced by the vesicle outgrowth of endosomes that mature into multivesicular bodies or by vesicle outgrowth directly from the plasma membrane.96 Exos are 30–150 nm in diameter, secreted by almost all cells,97 and retain the cancer-regulating properties of MSCs.98 Exos can transport biomolecules, such as proteins, lipids, and RNA, to target cells through various physiological barriers, including the blood–brain barrier (BBB),99 thereby increasing the local concentration of therapeutic agents.100 Owing to their lipid bilayers, Exos remain stable in the blood and have low immunogenicity and good biocompatibility and, hence, are used in several in vivo anti-cancer drug delivery strategies.101 Compared with cell therapy, Exos have revolutionized therapy for various diseases and enhanced drug safety.102–104
The use of Exos as a therapeutic vehicle for drug delivery is being actively explored. In animal models, Exos carry anti-cancer drugs into the brain via receptor-mediated endocytosis, promoting the cytotoxicity of anti-cancer drugs in cancer cells, significantly reducing cancer growth.105
Exos loaded with rifampicin (RIF) accelerated its entry into osteosarcoma (OS) cells, and the inhibition of OS proliferation, migration, and invasion by RIF was further enhanced. In mice, kinesin-related protein 1 (Drp1) was activated using EXO-RIF and caused mitochondrial lysis and apoptosis, thereby increasing survival.55 Sonodynamic therapy (SDT) is minimally invasive and exhibits low toxicity and the ability to treat deep tissues; however, low water-soluble acoustic sensitizers can limit its clinical application and the tumor microenvironment (TME) can affect its effectiveness. Exos facilitate communication between cells and regulate specific responses in recipient cells. Li et al. designed a bionanosystem (ECaC) by loading mesoporous calcium carbonate nanoparticles (CaCO3-NPs) and acoustic sensitizer curcumin (Cur) into cancer-derived Exos to synergistically enhance the efficacy of SDT. Exos provided homologous targeting capabilities to CaCO3-NPs and avoided clearance by the immune system. When they reach the cancer site, CaCO3-NPs are degraded into Ca2+ in acidic TME to disrupt the cellular mitochondria. Consequently, cancer cell respiration is disrupted, causing oxidative stress and enhancing Cur-mediated chemotherapy/SDT.56 Gu et al. used Exos derived from human umbilical cord mesenchymal stromal cells (hUCMSCs) and cyclic peptide arginine-glycine-aspartate (cRGD) encapsulated with thujaplicin lactone (TP) to establish a bionic targeted drug delivery system (cRGD-Exo/TP). The delivery system exhibited a drug loading of 10.76 ± 1.21% and significant anti-proliferative, anti-invasive, and pro-apoptotic activities in A375 cells via the cystein cascade and mitochondrial pathway, as well as cell-cycle alterations (Fig. 4A).57
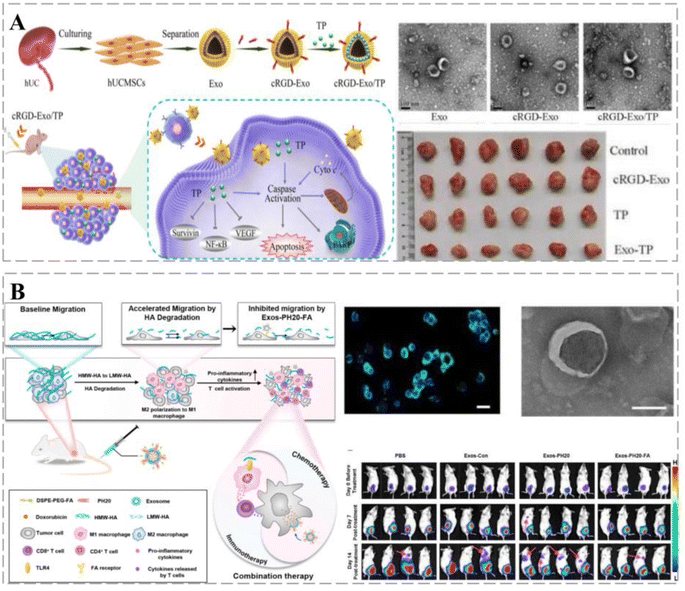 |
| Fig. 4 Exos for cancer therapy. (A) Functionalized Exo vehicles for targeted therapy of malignant melanoma. These vehicles exhibit a distinct bilayer membrane-shaped disc morphology; the cRGD-Exo/TP group significantly inhibits cancer growth. (B) Folic acid-modified self-assembled and genetically engineered Exo vehicles transform the cancer microenvironment from immunosuppressive into immune-supportive and improve the efficacy of combination chemotherapy. PH20 expression can be observed on the surface of transfected 293T cells and used to produce Exos. Furthermore, after FA modification, Exo-PH20-triggered metastasis of cancer cells to the lung was significantly inhibited. | |
Surface modifications can confer additional functions to Exos, such as sensitization of TME, stimulation of immune responses, improved cancer targeting and retention, and in vivo imaging and transport. Feng et al. used genetic engineering and self-assembly techniques to develop Exos-PH20-FA, where Exos were modified with folic acid (FA). Exos-PH20-FA polarized macrophages to the M1 phenotype and reduced the number of associated immunosuppressive immune cells, thereby changing the immune microenvironment from immunosuppressive to immune-supportive. In addition, Exos-PH20-FA directly reduced hyaluronidase-induced cancer cell metastasis (Fig. 4B).58 Interleukin (IL)12 was prepared by fusion with the exosomal surface protein, PTGFRN, to generate ExoIL12. ExoIL12 exhibited longer cancer retention, greater anti-cancer activity, and more potent cancer growth inhibition than recombinant IL12.59 The aforementioned studies demonstrate that Exos play a key role in cancer treatment and can improve prognosis; therefore, Exo-based therapeutic strategies provide alternative options for cancer treatment.
2.2 Active targeting-based delivery vehicles
Active targeting strategies are being developed to enhance tumor therapy (Table 2).
Table 2 Summary of active targeting-based delivery vehicles in cancer
Type of delivery vehicles |
Cancer |
Mechanisms |
References |
Nanorobots |
Hepatocellular carcinoma cells (Hep3B) |
Manipulation of nanorobot movement using an external EMA system; real-time drug release by near-infrared laser irradiation |
106
|
Folic acid (FA) |
Breast cancer |
Enhances cellular internalization and promotes drug uptake |
107
|
|
HepG2 cells |
Promotes intracellular uptake of drugs by tumor cells; co-administration of drugs by chemotherapy and photothermal action for synergistic anti-tumor effects |
108
|
Lactoferrin (Lf) |
Prostate cancer |
Delivery of drugs into drug-resistant cells to avoid drug efflux and prolong nuclear retention time |
109
|
|
Glioma |
Modulates the STAT6 pathway and inhibits Ras/Raf/p-Erk pathway-induced mitochondrial apoptosis |
110
|
|
Breast cancer |
Prolonged drug action; selective cytotoxicity against tumor cells |
111
|
2.2.1 Nanorobots.
Current drug delivery nanocarriers rely on the enhanced permeability and retention (EPR) effects and lack dynamic navigation, which limits their therapeutic efficacy. Nanorobots offer unique features as novel delivery vehicles, including propulsion, controlled navigation, and cargo traction and release, and can potentially penetrate tissues and rapidly transport therapeutic drugs directly to target sites, improving efficacy and reducing systemic side effects of toxic drugs. The Janus nanomotor is a small active targeting delivery system. Scientists have developed micro-robots over the past decade for precise drug delivery and controlled release.112 These mobile microrobots can be used to target deep wounds and narrow blood vessels that are difficult to reach during surgery.112,113 They increase the apparent diffusion coefficient by a factor of two at low H2O2 fuel concentrations (<3 wt%). Owing to their mesoporous structure and small size, mobile microrobots can be loaded with large amounts of cargo molecules and act as active nanocarriers for targeted delivery on chips.114 The speed of motion of nanorobots can be regulated by changing the fuel concentration, and the direction of motion can be controlled using an external magnetic field. These assembled nanorobots can act as both autonomous engines and intelligent carriers for drug loading, targeted transport, and remotely controlled release near cells and tissues in living organisms.115 Helical microrobots with gyroid surfaces exhibit enhanced drug-loading efficiency.106 The microrobots, controlled by an external EMA system and equipped with iron oxide nanoparticles on their surface, can achieve real-time drug release through irradiation with a near-infrared (NIR) laser, combining photothermal and drug treatments for maximum therapeutic effect and minimal side effects (Fig. 5A).
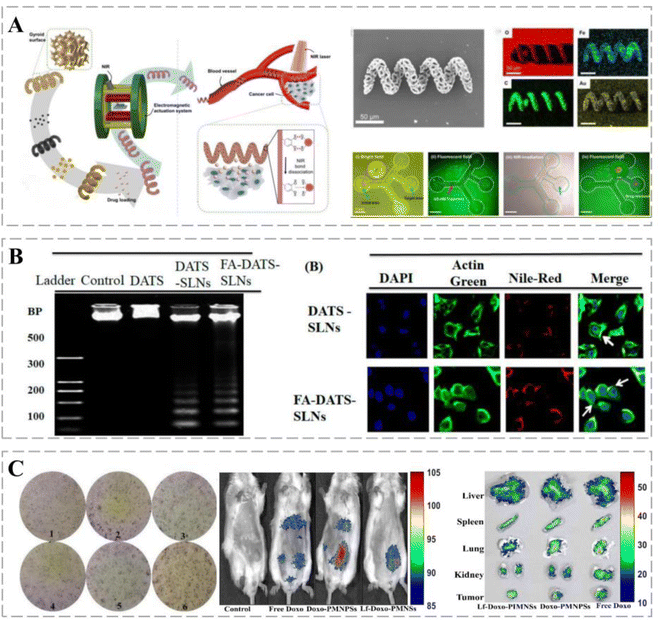 |
| Fig. 5 Applications of active targeting vehicles. (A) Magnetically guided helical microrobots with a gyroid surface coated with magnetic nanoparticles (MNPs) can achieve active motion under magnetic fields, and the use of plasmon resonance (LSPR) to modulate the robot surface coated with star-shaped gold nanoparticles (Au-nanostars) can facilitate multi-step drug release. (B) The MDA-MB-231 cell line treated with FA-DATS-SLNs exhibited more apoptotic DNA fragmentation with superior DATS cell accumulation. This vehicle, with target specificity, promotes and improves the internalization of DATS cells. (C) Lf-Doxo-PMNSs can stimulate 4T1 cell death by generating ROS, resulting in significant changes in cell morphology and targeted delivery of drugs. | |
2.2.2 Folic acid.
Active targeting enhances therapeutic carrier selectivity for cancer cells, minimizing the distribution of drugs in non-specific tissues. Various ligands can be used to functionalize nanocarriers, of which surface modification of vehicles by folic acid (FA) is a promising strategy for active cancer targeting owing to its low cost, easy coupling to nanoparticles, and limited stimulation of the immune system.116 In particular, most solid cancers, including breast cancer, exhibit overexpression of the alpha isoform, whereas its expression in normal tissues is negligible; therefore, FA-nanoparticles are a promising therapeutic strategy. Granja et al. functionalized solid lipid nanoparticle (SLN) vehicles loaded with mitoxantrone (Mito) using disteroyl phosphatidylethanolamine-poly(ethylene glycol)-FA (DSPE-PEG-FA) ligands. Functionalized NPs can improve the anti-cancer effect of free drugs.
In addition, confocal microscopy and flow cytometry revealed the enhanced cellular internalization of functionalized solid lipid nanoparticles (SLNs) via folate receptor (FR)-mediated endocytosis.107 Chen successfully constructed a folic-acid-modified erythrocyte drug delivery system, DOX and ICG-PLGA@RBC nanoparticles (DIRNPs), for simultaneous transport of chemotherapeutic drugs (e.g., DOX) and photothermal agents (indocyanine green, ICG) for application in synergistic chemotherapy. FA modification effectively promoted the capture of DIRNP vehicles by HepG2 cells by promoting the level of ROS that induced apoptosis and limited cell migration. A NIR laser disintegrates DIRNPs by increasing the local temperature of cancer tissues and rapidly releases the loaded drug, which in turn promotes cancer cell apoptosis. Therefore, this combination strategy is an effective method for cancer treatment.108 Compared with non-targeted DATS and DATS-SLNs, FA-DATS-SLNs containing surface-functionalized FA are further selective for invasive TNBC MDA-MB-231 cells and more susceptible to cellular capture, which increases cytotoxicity. FA-DATS-SLNs significantly downregulate the anti-apoptotic protein Bcl2, upregulate the pro-apoptotic protein caspase-9, and enhance the apoptotic potential of functionalized agents by interfering with the intrinsic apoptotic pathway (Fig. 5B).117
2.2.3 Lactoferrin.
Lactoferrin (Lf) is a non-toxic and inexpensive natural iron-binding glycoprotein, the anti-cancer activity of which has been observed in various cell lines, animal models, and clinical trials. The main anti-cancer mechanisms include the downregulation of the anti-apoptotic protein Bcl-2,118 increased expression of the pro-apoptotic Bax protein,119 upregulation of Fas expression,120 and specific activation of the p53 cancer suppressor gene.121 Since 1992, the mode of action of Lf in cancer therapy has attracted increasing attention.122–124 Lf has been used for surface modification of drug nanocarriers since its receptor is overexpressed by cancer cells and other tissues, such as the brain. Lf acts as an excellent functional carrier protein coupled with DOX for delivery to DU145 cells, CD44+/EpCAM+ double-positive enriched DU145 3D prostate cells, and drug-resistant ADR1000-DU145 cells, bypassing the DOX efflux to overcome chemoresistance. Iron-saturated bLf-DOX inhibits cancer development by upregulating the serum levels of anti-cancer molecules (e.g., TNF-α, IFN-γ, CCL4, and CCL17).109 Mo et al. co-encapsulated simvastatin and fenretinide in a TPGS-TAT-embedded Lf nanoparticle system via the LRP-1 receptor for brain-targeted bionic delivery. The Lf-nanoparticles change cancer-associated macrophages from the M2 to M1 phenotypes by modulating the STAT6 pathway and inhibiting the Ras/Raf/p-Erk pathway to induce mitochondrial apoptosis.110
Lf-inorganic nanocarriers exert synergistic anti-cancer effects when used in combination with chemotherapy. Sharifi et al. coated highly homogeneous porous magnetite nanoparticles (PMNs) with Lf for targeted drug delivery to breast cancers. Lf-Doxo-PMNs prolonged the circulation time of DOX in the blood and reduced cancer drug resistance. In addition, combination therapies based on Lf-Doxo-PMNs, such as chemo-MF, chemo-PTT, and chemo-MF-PTT, induced apoptosis through extrinsic (TNF-α) and intrinsic (Bax) pathways and significantly reduced the volume and size of breast cancers (Fig. 5C).111 Lf can cross the BBB, and its receptors, LfRs, are highly expressed on the surface of glioblastoma cells.125 Song et al. used Lf as a targeting ligand to construct an Lf@graphene oxide (GO)@Fe3O4 targeted delivery system via EDC/NHS chemistry. Lf-modified nanocarriers have higher intracellular delivery efficiency and are more cytotoxic to C6 glioma cells than free DOX and DOX@GO@Fe. These results suggest Lf-conjugated GO@Fe as a potential 3O4 nanocomposite for therapeutic applications in glioma treatment.126
2.2.4 Others.
Escherichia coli K1 (EC-K1) can actively penetrate the BBB and induce bacterial meningitis after colonizing the brain. We have referred to the bacteria-based drug delivery system as “Trojan Bacteria.” It is used for photothermal immunotherapy of glioblastoma (GBM). The system consists of a loaded photosensitive ICG bypassing the BBB and targeting the penetration of GBM tissue, after which the ICG converts light energy into heat under laser irradiation to destroy cancer cells.127 In contrast, dead EC-K1 can penetrate the BBB and does not produce bacterial virulence. Based on this feature, EC-K1 was modified by maltodextrin and extinguished under UV irradiation to construct a “dead EC-K1” drug delivery system, which significantly enhanced the accumulation of therapeutic drugs in the brain without toxicity and retained the health of the animal model.128
3. The pharmacokinetics of smart cancer therapy delivery vehicles
Pharmacokinetics is the science of elucidating the relationship between drug concentration in different parts of the body and time by quantitatively studying the dynamic changes in the absorption, distribution, metabolism and excretion of drugs in the organism. Drugs are metabolized by different pathways in different tissues and cells.129 Many factors can affect drug metabolism, such as cellular transporters, metabolic enzymes, pH environment and electrochemical gradients, as well as the drug itself, such as drug polarity, dosage form, and surface charge. Compared with free drugs, smart delivery vehicles have the characteristics of controllability and targeting, which can improve the bioavailability of drugs and reduce the toxicity and side effects, while their cell entry and transmembrane pathways and the mechanism of pharmacological effects are different from those of free drugs.130 The pharmacokinetic study of smart delivery vehicles can more effectively and comprehensively evaluate and predict the efficacy of the loaded drugs and the possible toxic side effects, and can provide more important references for the design of smart delivery vehicles.131
3.1 Absorption and transport
Smart delivery carriers enter cells mainly through endocytosis, and the main endocytosis pathways include giant cell drinking, lattice protein-mediated endocytosis, follicular protein-mediated endocytosis and lattice protein/follicular protein-independent endocytosis. The endocytosis process is closely related to the nature of the carrier itself and the nature of the cell.132,133
The nature of the vehicles itself mainly includes surface charge, particle size and shape. The charge can affect the amount and pathway of the delivery vehicles into the cell. Positively charged vehicles have stronger interactions with cells, and are therefore more likely to be taken up by cells and tend to be endocytosed through the lattice protein-mediated pathway.134 In addition, charge can also affect the organelle localization of the vehicles after they enter the cell, as negatively charged vehicles are more likely to pass through the lysosomal degradation pathway, whereas positively charged vehicles tend to bypass the lysosomal pathway. Particle size can affect the entry and transit of therapeutic carriers. It was found that the larger the particle size, the slower the internalization rate of the vehicles.135 Studies have shown that nanocarriers exhibit excellent dissolution properties due to their smaller particle size and larger surface area. In addition, under simulated in vitro gastrointestinal conditions, nanocarriers showed faster release, higher bioaccessibility and higher permeability.136 In addition, the cell type can also affect the cellular uptake of the delivery vehicles. The cancer cell uptake of arginine–glycine–aspartic acid (RGD)-modified DOX liposomes was higher, which was closely related to the high expression of integrin receptors on the cancer cell surface.137
The in vivo blood concentration of smart delivery vehicles is usually inconsistent with the cellular level of cell entry. Differences in the nature of delivery carriers and target cells may lead to a higher distribution of highly targeted delivery vehicles in target organs and target cells, thus showing more significant drug effects. The study of cellular pharmacokinetics can be used to screen out smart delivery vehicles with high targeting ability, reduce the workload of in vivo experiments, and improve the efficiency of drug screening. According to the results of cellular pharmacokinetics, when designing smart delivery vehicles, we can try to change the absorption and transportation pathways of drugs by changing the charge and particle size of the vehicles, thus affecting the absorption and distribution of drugs in the body.
3.2 Distribution and metabolism
Compared with free drugs, the distribution, metabolism and efficacy of smart delivery vehicles are altered after entering cells. In MCF-7 cells, the concentration of free PTX was higher than that of CMCS-DFNS@PTX at 1 h, which may be attributed to the fact that CMCS-DFNS@PTX needs to be endocytosed to enter the cells and needs a certain sustained release time after entering the cells to exert the drug effect. After 2 h, CMCS-DFNS@ PTX had a higher drug concentration than free PTX after 2 h, and the drug concentration in the cells reached the highest value after 12 h.138
For drugs with different targets and properties, changes in intracellular transport pathways induced by smart delivery vehicles can bring about different therapeutic effects. DOX and PTX, which are physicochemically and chemically stable, can be efficiently delivered into the cell by the vehicles, whereas biomolecule drugs (e.g., peptides, proteins, siRNAs, etc.) are unstable in a lysosomal low-pH and enzyme-rich microenvironment,139 so the lysosomal escape of biomolecule drugs has to be taken into account in designing the vehicles loading drugs. Since the traditional pharmacokinetics based on plasma drug concentration often fails to fully explain the pharmacological effects of drugs on cancers, a more in-depth understanding of the mechanism of drug efficacy can be achieved by analyzing the distribution of smart delivery vehicles in cells. The design of different smart delivery vehicles according to the specific internal environment of the cell can achieve the purpose of controlling the metabolic pathway of drugs in the cell, thus providing a broader idea for cancer therapy.
3.3 Excretion
Free drugs can easily enter normal cells, but it is difficult for them to accumulate in drug-resistant cells due to the role of various exocytosis proteins. The emergence of smart delivery vehicles has solved this problem.
Ideally, the delivery vehicles should be excreted via the renal clearance route. Studies have shown that the dissolved Bi(III) ions in BiNPs can be cleared by metallothionein (a cysteine-rich protein in the kidney, excreted in the urine). Even at high concentrations of 800 μg mL−1, the nanocarriers demonstrated good blood compatibility with a hemolytic effect of less than 2%. No significant weight loss or tissue damage was observed in the animals after administration of BiNPs.140 It was shown that free PTX in cells was significantly eliminated in the first 18 h, while the elimination of CMCS-DFNS@PTX was relatively slow. Throughout the elimination period, the concentration of CMCS-DFNS@PTX in the cells was higher than that of free PTX, indicating that CMCS-DFNS could prolong the circulation time of PTX in the cells and significantly increase the bioavailability of PTX, thus improving the therapeutic effect.138
In conclusion, the study of the cellular exocytosis of delivery vehicles is of great significance in improving the efficacy of drugs and designing new formulations.
Smart delivery vehicles often exhibit different cellular pharmacokinetic behaviors compared to free drugs. The study of the absorption, transport, distribution, metabolism and excretion processes of smart delivery vehicles in tissues and cells plays a crucial role in the effectiveness and safety of delivery systems. It is of great significance for the development, screening and clinical application of smart delivery vehicles to record, analyze and reveal the intracellular kinetic processes and laws of smart delivery vehicles by using analytical techniques and cellular molecular biology research techniques.
4. Advantages of smart cancer therapy delivery vehicles
4.1 Delivery of drugs with different physicochemical properties through improved solubility
The poor solubility of most anti-cancer chemotherapeutic drugs such as adriamycin and methotrexate,141 in aqueous solutions hinders their clinical application, mainly due to the difficulties in passing through the aqueous environment surrounding the cancer cells to cross the cell membrane and act on intracellular targets. In addition, some chemotherapeutic drugs can cause serious toxicity through intravenous administration, such as skin and visceral damage.142 Therefore, the delivery of hydrophobic therapeutic agents to cancer tissues is an important breakthrough in cancer therapy.
Smart drug delivery carriers containing hydrophobic or amphiphilic materials address the problem of poor solubility of hydrophobic drugs, such as PLGA, PLA, chitosan, gelatin, polycaprolactone, and polyalkyl cyanoacrylate.143 The high lipophilicity and low water solubility of the oleanolic acid derivative DKS26 are attributed to its very low oral bioavailability. Liposomal loading of DKS26 significantly enhances the absolute oral bioavailability.144 Gholizadeh et al. prepared immunoliposomal carriers to deliver sepantronium bromide YM155 (a hydrophilic drug with low oral bioavailability and rapid renal elimination). The YM155-loaded liposomes exhibited prolonged circulation and a significantly increased half-life in cancer tissue compared to intravenous free YM155.145 Paclitaxel (PTX) is an effective anti-cancer drug with very low solubility in water. Meanwhile, the complex gastrointestinal environment and epithelial barriers hinder its antitumor effect. PEGylated high-density glycerylcholic acid-decorated micelles (PTX@GNPs) based on PEGylation can encapsulate PTX by π–π stacking, thereby gaining mucus-trapping escape ability and significantly improving drug targeting in the gastrointestinal tract.146 Mitomycin C (MMC) is used for the treatment of various solid cancers; however, the application of MMC via intravenous injection is associated with toxic side effects and non-specific interpolymerization. Yang et al. synthesized PEG2k-Fmoc-ibuprofen (PEG-FIbu) micelle nanocarriers loaded with MMC. PEG-FIbu/MMC micelles exhibited superior stability, higher drug loading efficiency, slower release, longer circulation time, and higher cancer uptake and therapeutic efficiency compared to MMC intravenous injection.147 Zhao et al. developed FA-PLGA/PCADK-lipid NPs (FA-PPLNPs) to encapsulate methotrexate (Mtx). The developed NPs exhibited high cellular uptake rates.148 The amphiphilic CPT-ss-EB prodrug developed by Zhang et al. self-assembles into nanostructures with high solubility in aqueous solution while rapidly transforming into a long-term circulating nanocomplex.149 Therefore, therapeutic carriers can enhance the solubility of hydrophobic drugs and deliver drugs with different physicochemical properties.
4.2 Controlled release of drugs
Kline and French introduced the first controlled-release formulation for the delivery of dextroamphetamine (dexedrine) in 1952. By the late 1970s, no improvements were made in understanding the mechanism underlying controlled drug delivery. Smart therapeutic vehicles are capable of controlled-release of the loaded drug and can improve the presentation of the drug in different temporal spaces in the body, protect the drug from clearance and degradation, and reduce the toxic effects, which can improve patient outcomes. Drug delivery technologies have advanced, from understanding drug release mechanisms to manipulating vehicle size for targeted drug delivery, which has facilitated the development of several nanoparticle-based controlled-release systems with excellent results.150,151
Smart stimuli-responsive nanoparticles (srNPs) have undergone substantial progress as effective drug-delivery vehicles for cancer immunotherapy. srNPs use unique cancer microenvironments or external stimuli, such as weak acidity, high glutathione (GSH) concentrations, overexpression of cancer site enzymes, and ROS, as triggers for the precise delivery and controlled release of drugs. This function can improve the bioavailability of the drug and reduce its toxic effects.152 Stimulus-responsive delivery systems exhibit more dynamic activity than non-stimulus-responsive nanocarriers, allowing for more precise drug release.153
The structure and metabolism of cancers result in an acidic microenvironment,154 which provides favorable conditions for cancer growth, affecting the immune surveillance of cancer cells and possibly leading to the immune escape of the cancer. Cancer-targeting smart nanoparticle carriers can alter this microenvironment based on different pH values: the surface potential of the carrier shifts from negative to neutral (pH 6.5–7.0), which facilitates cellular uptake of the drug, whereas, at pH 4.5–6.5, the carriers dissociate, inducing endosomal escape and releasing the drug into the cytoplasm. Meanwhile, smart nanoparticles modified with the cancer-penetrating peptide iNRG were used as cancer-targeting molecules. In an acidic environment (pH 6.8), this carrier promoted the uptake of the drug by cancer cells (Fig. 6A).155 The polyion complex (PIC) micelles prepared by Hsieh et al. demonstrated good colloidal stability at different pH values. Controlled permeability of the micelles can be achieved by adjusting the degree of cross-linking and accelerating drug release under low pH conditions.156 ROS plays an important role in cancerogenesis; elevated ROS levels have been reported in several cancer cells.157 Wang et al. designed a therapeutic system containing a ROS marker that oxidizes and hydrolyzes TSPBA in the presence of ROS, resulting in sustained release of gemcitabine (GEM) and aPDL1 (anti-PD-L1 blocking antibody) to enhance anti-cancer responses.158 In addition, enzyme-responsive cancer drug delivery systems offer new solutions for cancer therapy. Wang et al. synthesized PEG5k-GL2-IMDQ micelles using imidazoquinoline-like TLR7/8 agonists. The micelles form vesicles in aqueous media that can be specifically degraded by endosomal enzymes and can control drug delivery through an enzymatic response at the tidal junctions. Once micellar vesicles accumulate in the cancer region, effector proteases are depleted, leading to local drug release (Fig. 6B).159 Under hypoxic conditions, macrophages and neutrophils are easily transformed into the cancer-promoting M2 phenotype, thereby inhibiting the killing effects of T and NK cells.160 However, as the levels of anaerobic metabolites increase, the production of interferon γ (IF-γ) is affected, which impairs the function of connective tissue growth factors. Therefore, the design of low-oxygen-responsive nanomaterials will enhance cancer therapy. Nanovesicular carriers were assembled from hypoxia-responsive amphiphilic polymer-grafted manganese ferrite nanoparticles (MFNs), with DOX loaded into an aqueous cavity. Under hypoxic conditions, the nanocarriers rapidly dissociate into individual MFNs, releasing DOX and inducing cancer breakdown of H2O2, relieving cancer hypoxia, and contributing to cancer treatment.161
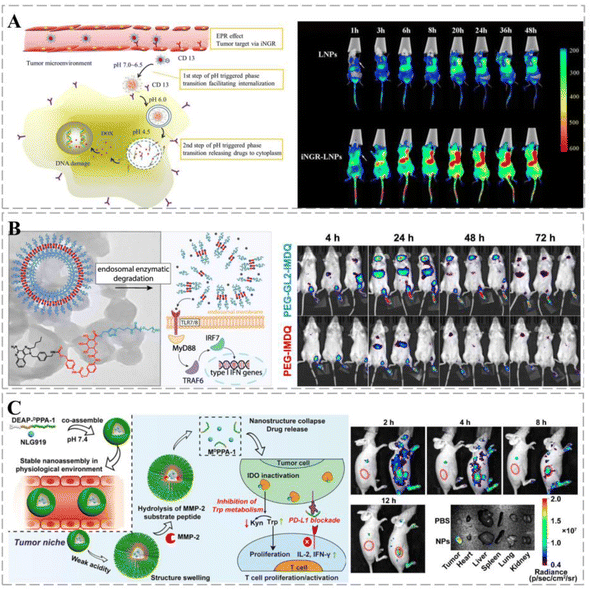 |
| Fig. 6 Smart therapeutic vehicles enabling controlled drug release. (A) Schematic diagram of the two-step phase transition of pH-triggered iNGR-LPNs. iNGR-LPNs alter the biodistribution of free DOX, exhibiting long circulation and cancer-specific distribution properties while avoiding the cardiac distribution. iNGR-LPNs administered to mice first recognize cancer neovascularization, induce high levels of particle accumulation at the cancer site, and promote cancer-specific cellular uptake via CD13 receptor-mediated endocytosis and pH-triggered particle phase transition to promote cancer-specific cellular uptake. Reprinted with permission from ref. 155. (B) Upon endocytosis by antigen-presenting cells, the self-assembled nanovesicles are degraded and release native IMDQ, which bound to TLR7/8 receptors and triggered immune activation. One week after injection, PEG5k-GL2-IMDQ vesicle signals can be detected in mice, suggesting that sustained degradation and release of IMDQ prolong immune stimulation due to IMDQ, thereby reducing the frequency of dosing and inducing a more effective immunomodulatory effect. (C) Schematic diagram of the composition of DEAP-DPPA-1 and the anti-cancer mechanism of NLG919@DEAP-DPPA-1 nanoparticles. The peptide-assembled nanoparticles have a high sensitivity to pH. TRITC fluorescence shows nanoparticles predominantly distributed in tumors. Reprinted with permission from ref. 162. | |
Multi-responsive therapeutic regimens can amplify anti-cancer responses. Nanocarriers containing therapeutic peptide components respond to dual stimuli in the cancer extracellular matrix with targeted delivery to cancer and on-demand release of a short D-peptide antagonist of programmed cell death ligand 1 (DPPA-1) and an isoindolamine 2,3-dioxygenase inhibitor (NLG919). By blocking the immune checkpoint and tryptophan metabolism, the local release of DPPA-1 and NLG919 facilitates cytotoxic T lymphocyte survival and activation, ultimately inhibiting melanoma progression (Fig. 6C).162 Xu et al. designed a drug delivery vehicle that responded sequentially to the triple response of MMP-2, pH, and GSH for co-immunotherapy of TNBC. The therapeutic vehicle undergoes structural transformation to achieve optimal size and shape changes according to therapeutic needs. It synergistically amplified the ROS cascade response, increased H2O2 and ˙OH levels, induced immunogenic cell death (ICD) responses, and promoted anti-TNBC immunity by enhancing the interaction between dendritic cells (DCs) and dying cancer cells.163 Stimuli-responsive drug delivery systems have considerable potential to improve the efficiency of cancer treatment by controlling drug release and degradation and combining them with multiple treatment options to minimize side effects.
4.3 Overcoming multi-drug resistance and synergistic treatment to improve efficacy
The underlying principles of various cancer combination therapies have been investigated to achieve optimal clinical outcomes.164 Compared with monotherapy, combination therapy significantly improves clinical outcomes, effectively overcomes clonal heterogeneity, and reduces drug toxicity in humans.165
MDR affects the efficacy of chemotherapy and leads to chemotherapy failure. When treatment doses are increased to avoid drug resistance, the resulting drug toxicity damages healthy organs and tissues. Smart drug delivery carriers have been proven effective in overcoming MDR using several mechanisms. Degradable poly(lactic acid–glycolic acid) (PLGA) NPs loaded with both DOX and Cur effectively inhibited the growth of DOX-resistant esophageal cancer (Fig. 7A).166 Zhen et al. constructed DEB/TQR@PMP micelles by encapsulating a near-infrared fluorophore (DEB-BDTO) as a photosensitizer with the drug resistance inhibitor tariquidar (TQR) in a polymeric pre-drug (PMP). The micelles exhibited synergistic lethal effects on SKOV-3 and SKOV-3/MDR cells, significantly enhancing the inhibition of cancer growth.167 Xing et al. dissolved IR780 (a photosensitizer) in D-α-tocopheryl polyethylene glycol succinate (TPGS) micelles and loaded clusters of polydopamine (PDA) NPs on their surface for the combined treatment of drug-resistant breast cancer. Mediated by PDA, the system exhibited significant quenching of fluorescence emission and inhibition of singlet oxygen generation upon exposure to NIR light, facilitating efficient PTT treatment. Furthermore, micellar carriers significantly enhanced the intracellular accumulation of adriamycin hydrochloride, and photothermolysis promoted its release. Such findings suggest that smart therapeutic carrier-loaded drugs can enable complementary interactions between photothermal/photodynamic therapy/chemotherapy, thereby improving the efficiency of combination therapy for multi-drug resistant cancers (Fig. 7B).168
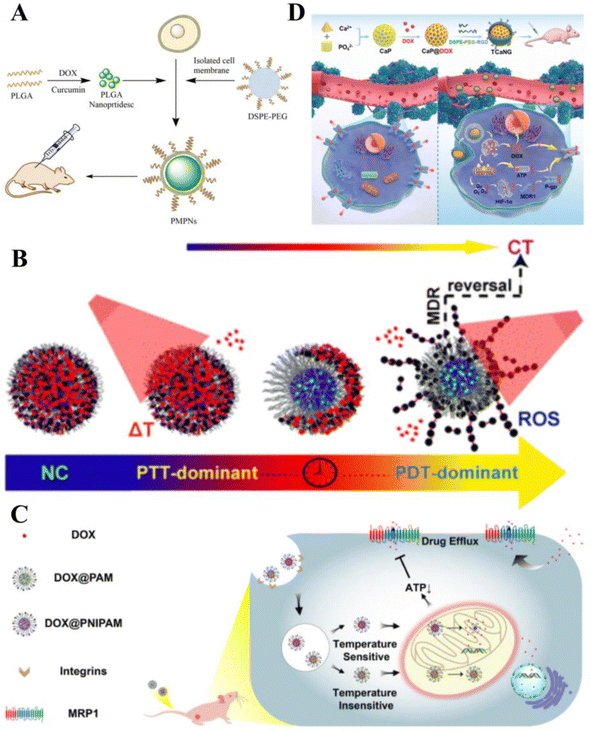 |
| Fig. 7 Smart therapeutic vehicles to overcome MDR. (A) PLGA loaded with DOX and Cur to form PLGA-NP, followed by the addition of isolated TE10 cell membranes and DSPE-PEG and self-assembly on PLGA-NP to form PMPN. Application of bionanodrug PMPN to the in vivo treatment of MDR esophageal cancer. (B) Photoresponsive nanocluster (NC) system enabling combination chemotherapy (CT)/photothermal therapy (PTT)/PDT for drug-resistant breast cancer. Reprinted with permission from ref. 168. (C) Mitochondrial temperature-responsive drug delivery in a DOX-resistant model of small cell cancer. The thermoresponsive nanocarrier PNIPAM can release DOX at high mitochondrial temperatures compared with the non-thermoresponsive nanocarrier PAM, thereby damaging mitochondria and reversing DOX resistance. (D) Cancer-targeted “calcium nano-generator” (TCANG) safely and effectively reverses drug resistance in cancer cells through a nano-activated intracellular calcium explosion resistance. Reprinted with permission from ref. 170. | |
Smart therapeutic carriers can also overcome MDR by inhibiting energy metabolism and blocking ion-mediated signaling pathways. Ruan et al. developed a mitochondrial temperature-responsive drug delivery system that prevents adriamycin efflux and promotes adriamycin accumulation and mitochondrial targeting in drug-resistant cancers using thermally responsive nanocarriers. Thermoresponsive nanocarriers effectively enhanced the cytotoxicity of adriamycin and reversed drug resistance in cancer-bearing mice (Fig. 7C).169 Such effects inhibit cellular respiration and downregulate HIF-1α expression to suppress P-glycoprotein biosynthesis. Additionally, Ca2+ burst-induced respiratory inhibition blocks intracellular ATP production, leading to P-glycoprotein insufficiency. Thus, TCANG enhanced the proliferative effect of IC50DOX on MCF-7/ADR cells by approximately 30-fold and the proliferation of drug-resistant cancers by approximately 13-fold (Fig. 7D).170
4.4 Diagnosis and treatment
Cancer therapeutic carriers can be loaded with various CAs and fluorescent agents to effectively deliver drugs. Owing to their optical properties, these carriers can be used in the diagnosis of cancers.
Zhou et al. developed NRh-G-NPs, which can specifically respond to GGT overexpressed in U87MG cancer cells and selectively illuminate cancers for image-guided therapy. Furthermore, restoring photothermal properties in the cancer region can improve the accuracy of cancer-targeted therapy and reduce side effects (Fig. 8A).171 Pan used circulating EV vehicles to assess cancer-specific drug–target interactions in patient blood samples, such as Exo small molecule chemical occupancy and protein expression monitoring (ExoSCOPE). The use of such technology in cancer diagnosis and treatment monitoring allows for accurate classification of disease status and rapid differentiation of the outcome of targeted therapies within 24 h of treatment.172 Nanodroplets (NDs), a noninvasive delivery strategy, can enhance both ultrasound imaging and therapeutic efficacy. Zhao et al. constructed novel SP94 peptide-modified and doxorubicin-loaded ultrasound nanodroplets (SP94-DOX-NDs) to target and treat castrate-resistant prostate cancer (CRPC). In vitro and in vivo experiments showed that SP94-DOX-NDs could specifically deliver DOX to 22RV1 cells under ultrasound guidance and, therefore, exhibited strong anti-cancer effects (Fig. 8B).173 Narmani et al. used a polyethylene glycol-modified and folate-functionalized PAMAM G4 dendrimer as a smart, low-toxicity nanocarrier. The nanocarrier exhibited excellent potential for delivering 5-FU chemotherapeutic agents to breast cancer cell lines, and cancer accumulation studies demonstrated its targeting ability. In addition, imaging studies of targeted radiotracers confirmed the excellent performance of the nanocomplexes in a cancer-bearing mouse model. In conclusion, novel smart synthetic nanocomplexes are suitable for cancer treatment, tracking, and imaging (Fig. 8C).174 Kong et al. designed a novel nanocarrier based on HA conjugated with Gd3+ and loaded it with therapeutic drugs for combined magnetic resonance imaging (MRI)-guided cancer chemotherapy and MRI-photothermal treatment. HA-Gd-DOX exhibited high photothermal conversion efficiency and photothermal stability; its pH-responsive release properties and photothermal effects allowed for the gradual release of DOX. HA-Gd-DOX was also efficient in MRI-guided cancer monitoring (Fig. 8D).175 Owing to the limitations of the BBB, it is difficult to maintain high concentrations of therapeutic drugs in the brain. The use of Lf-modified dual-target magnetic polydiethylene glycol nanocarriers (PDNCs) can improve BBB crossing efficiency for treating brain cancers. The magnetic Lf-modified PDNCs exhibited MRI and dual-targeting capabilities and could enhance PDNC transport to the BBB to track and target gliomas.176 In addition, highly fluorescent CdTe quantum dots (QDs) were coupled with Lf-targeted nanocapsules. The covalent bond between Lf and QDs prevents Cd from entering the circulatory system and ensures that QDs are released only at the cancer site. Upon conjugation to Lf (OFF state), QDs luminesced in vitro owing to an electron/energy transfer mechanism. Upon intracellular uptake into MCF-7 cells, the luminescence was restored (ON state) as the surface-bound ligand was separated from QDs in the cytoplasm. In vivo, cancer tissue from Lf-QDs–CS–NC-treated mice exhibited higher fluorescence intensity than the liver and kidney tissue, demonstrating the efficient localization of QDs in cancer tissue.177
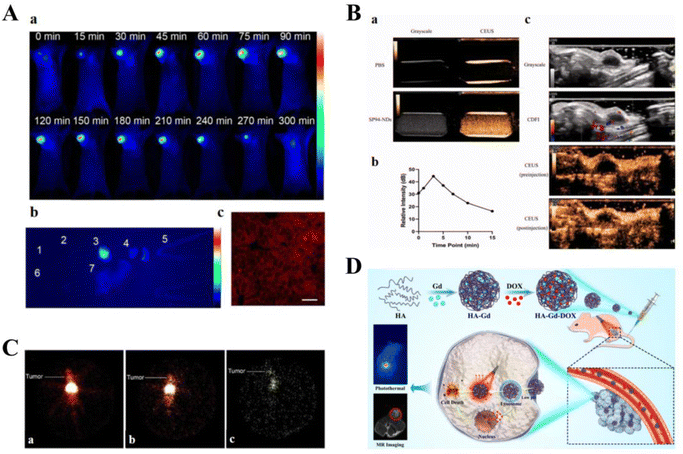 |
| Fig. 8 Smart therapeutic vehicle for simultaneous diagnosis and treatment. (A) NRh-G-NPs indicate the cancer location by passive targeting and can be used for effective real-time non-invasive imaging of GGT in cancers. (B) SP94-NDs achieve significant ultrasound enhancement. (C) P-PA-Suc-99mTc nanocomplex is localized to the specific site of the cancer following intravenous injection. Reprinted with permission from ref. 174. (D) HA-GD-DOX performs photothermal/chemotherapy guided by MRI for cancer treatment. | |
5. Smart cancer therapy delivery systems enhancing immunotherapy
Immunomodulation plays an important role in the treatment of cancers and has given rise to a range of therapeutic modalities for advanced cancers.178 Immunomodulation has several advantages, such as high specificity and few side effects, thereby killing cancer cells by prolonging cancerogenesis, inhibiting cancer growth, preventing recurrence, and suppressing metastasis.179 Current primary cancer immunotherapy strategies include immune checkpoint blockers,180 monoclonal antibody technology,181 and cancer vaccines.182 However, the limited response rate of patients to conventional immunotherapy, poor efficacy in solid cancers, and potentially serious toxic side effects limit the clinical use of immunotherapy in cancer treatment.183 The development of smart cancer therapy delivery systems has optimized cancer immunotherapy strategies to overcome the shortcomings of conventional immunotherapy184 while working synergistically with established immunotherapies to improve cancer response rates to drugs and patient survival.185,186
5.1 Elimination of immune escape
Cancers can use various immune-escape mechanisms to weaken or even silence the body's anti-cancer immunity187 and are, therefore, prone to invasion and metastasis.188 PD-L1 is a ligand for programmed death 1 (PD-1) protein that enables cancer cells to evade the body's immune system. Thus, blocking the interaction between PD-1 and PD-L1 enhances the immune response and anti-cancer activity,189,190 making PD-L1 an effective target for eliminating cancer immune escape.191 The promoter-specific CRISPR/Cas9 system (F-PC/pHCP) proposed by Zhao et al. can achieve permanent disruption of the PD-L1 genome and trigger a multifaceted anti-cancer immune response to enhance immunotherapy (Fig. 9A). The system comprises an encapsulated fluorinated dendrimer containing chlorine e6 and an HSP70 promoter-driven CRISPR/Cas9 system. Under a 660 nm laser, F-PC/pHCP activates HSP70 to specifically express Cas9 protein, thereby disrupting the PD-L1 gene and preventing immune escape, demonstrating excellent anti-cancer efficacy.192
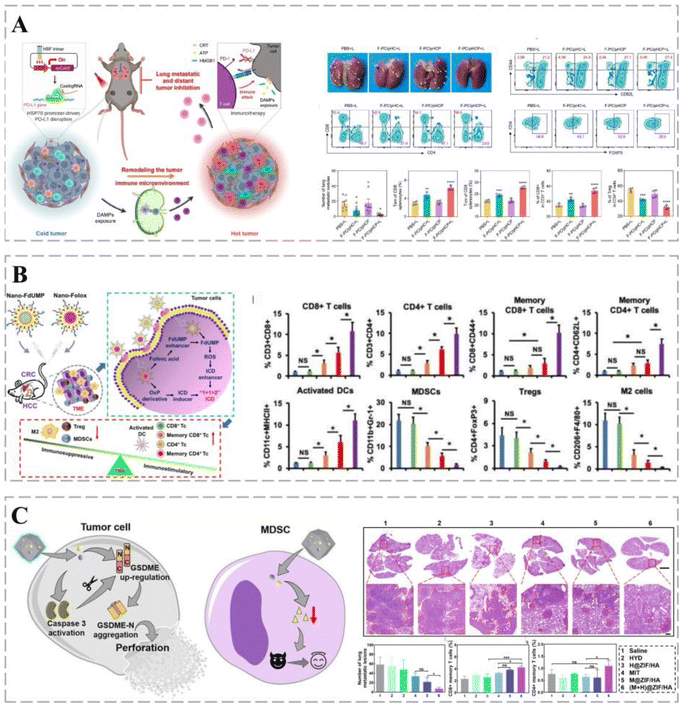 |
| Fig. 9 Smart treatment vehicles eliminate immune escape. (A) HSP70-Promoter-Driven CRISPR/Cas9 system activated by ROS for multifaceted anti-cancer immune response and multifaceted anti-cancer immune response (immunosuppression). The CRISPR/Cas9 system inhibits distant cancer growth and lung metastasis with the highest percentage of TEM and TCM cells and significantly reduces the percentage of CD8 T+ cells in distant cancers by 56.6% following F-PC/pHCP+L treatment. Treg cell numbers (immunosuppression). Reprinted with permission from ref. 192. (B) Nanopreparations containing FdUMP (nano-FdUMP) in combination with nanoformulations containing OxP derivatives and FnA (nano-Folox) for CRC and HCC treatment. The combination of the two nanoformulations shifts the cancer microenvironment from “cold” to “hot”, with CD8 T cells, CD4 T cells, and dendritic cells (DCs) being significantly activated by the combined strategy, and MDSCs, regulatory T cells (Tregs), and cancer-associated macrophages (M2) being significantly downregulated in cancer. (C) Schematic representation of the role of (M + H)@ZIF/HA in cancer cells and MDSCs. (M + H)@ZIF/HA treatment resulted in a significant reduction in metastatic nodules and an increase in the percentage of CD8 TCMs and CD4 TCMs in mice, indicating that this vehicle can stimulate T-cell immune memory responses to suppress cancer metastasis. Reprinted with permission from ref. 197. | |
The event of cancer cell death promoting anti-cancer immune responses is known as ICD.193 Cancer cells undergoing ICD promote the activation of antigen-presenting cells (APCs) by releasing a damage-associated molecular pattern (DAMP), which increases the activation of antigen-specific T-cells, enhancing the anti-cancer effect. The combination of low doses of nano-Folox and free 5-FU significantly promotes CRC cancer regression through OxP-mediated immunogenic cell death (Fig. 9B).194
Most cancer treatment approaches, such as chemotherapy and immunotherapy, enhance the immune response by increasing antigen exposure, mainly by triggering the apoptosis of target cells.195,196 However, apoptosis is considered a form of immune silencing, and the immune effects of chemotherapy may be severely affected by apoptosis. Scorch death is another type of programmed cell death that can be used to enhance the immunogenicity of cancer cells; however, immune evasion involving myeloid-derived suppressor cells (MDSCs) limits the use of immunotherapy based on scorch death. MOF-based nano-delivery systems trigger apoptosis-scarring death transition and counter MDSC-based immune escape. (M + H)@ZIF/HA nano-delivery systems convert cancers into a reservoir of antigens that stimulate a powerful immune response while suppressing immune escape. It triggers a strong cytotoxic T-cell response that eliminates cancers and establishes a long-term immune memory response that prevents further metastasis (Fig. 9C).197
5.2 Improvement of the cancer immunosuppressive microenvironment
The TME consists of various cell types (e.g., immune cells, fibroblasts, endothelial cells, and lymphocytes), extracellular matrices, blood vessels, and chemokines and directly affects immunotherapy efficacy.198 The TME affects the penetration of therapeutic agents into cancer cells and is associated with MDR and low response rates in the organism; therefore, smart therapeutic vehicles targeting the TME can enable cancer-specific therapy.199,200
Immunosuppressive cells, such as tumor-associated macrophages (TAMs), regulatory cells (Tregs), and MDSCs, can promote cancer development and resist immunotherapy by providing nutrition to cancer cells. However, they can also exert anti-cancer effects by enhancing phagocytic and oxidative functions.201 Currently, cancer drug development is shifting from targeting the intrinsic properties of cancer cells to the cancer immune microenvironment and the body's immune system.202 Multiple delivery vehicles have been designed to target TAMs and MDSCs to deliver drugs that improve the therapeutic impact of the cancer immunosuppressive microenvironment by inducing apoptosis, inhibiting cell infiltration activation, or modulating cancer cell differentiation.203 In addition, T-cell hypofunction can lead to poor outcomes in cancer immunotherapy. Therefore, activating T-cell function in the cancer microenvironment enhances anti-cancer effects.204 Tang et al. proposed using protein nanogels (NGs) to “package” large amounts of drugs onto T-cells and selectively release them upon T-cell receptor activation. Compared with the systemic use of free cytokines, the release of NGs resulted in a 16-fold expansion of T-cell numbers, whereas no significant cytotoxicity at increasing drug doses was observed.205
Smart cancer therapy delivery systems have also been applied to enhance the infiltration of immune cells into the TME, specifically promoting the infiltration of effector T-cells with anti-cancer effects into the cancer tissue, thereby improving the cancer immune microenvironment. The cancer acid-reactive nanoparticle delivery system NP-siCD47/CCL25 significantly increased CCR9+CD8+ T-cell infiltration and downregulated CD47 expression in cancers. It sequentially released CCL25 protein and CD47 siRNAs, thereby downregulating cancer development and metastasis through T-cell-dependent immunosuppression. The combination of NP-siCD47/CCL25 with the PD-L1 antibody synergistically enhanced their anti-cancer effect.206
Cancers are associated with fibroblasts at all stages of development, including metastasis.207 In addition to immune cells in the TME, smart cancer therapy delivery systems have been designed to target non-immune cells, such as cancer-associated fibroblasts (CAFs). CAFs improve immunosuppression by increasing the proportion of ECM in the TME to reconstitute the microenvironment.208 CAFs contribute to the evolution of MDR cancer phenotypes through various mechanisms.209 Kovács et al. developed Au@Ag NPs and demonstrated their indirect effect on the metastatic activity of cancer by weakening the pro-cancer capacity of CAFs and regulating their secretion (Fig. 10A).210 In addition, gold-nucleated nanoparticles (AuNPs) can reduce the density of fibroblasts within cancers and improve the chemotherapeutic effects of cisplatin (Fig. 10B).211
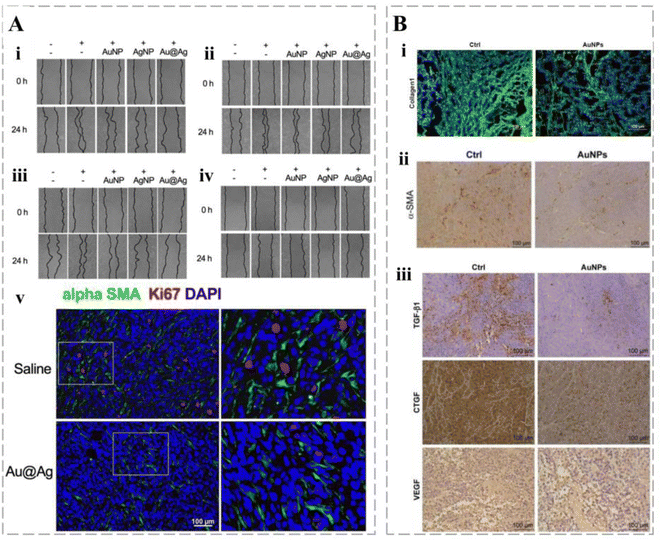 |
| Fig. 10 Smart treatment vehicles improve the cancer immunosuppressive microenvironment. (A) AgNP and Au@Ag treatments significantly inhibit the cancer cell-promoting activity of fibroblasts, characterized by reduced wound closure, thereby slowing the migration of adenocarcinoma cells. In addition, vehicles reduce the number of proliferating cancer cells in fibroblast-rich cancer microdomains. (B) (i) AuNPs rapidly inhibit collagen I expression in cancers, decreasing pericancer blood flow; (ii) AuNPs reduced α-SMA-positive CAF density in SW620 cancers; (iii) AuNPs reduced pro-fibroblast cytokine levels in plasma and SW620 cancers. | |
5.3 Enhancement of the anti-cancer effect of the peripheral immune system
The successful clinical use of immune checkpoint inhibitors has led to the establishment of immunotherapy as a key component of cancer treatment.212 Immune checkpoint blockade therapy has been applied in various cancers, and its applications are broadening.213 Preclinical and clinical data suggest that the antibody blockade of immune checkpoints significantly enhances the anti-cancer effects of immunotherapy.214 The activated immune system promotes immunosurveillance and eliminates cancers while establishing an immune protection mechanism against cancer recurrence.215 Therapeutic vehicles can target immune cells such as effector T-cells, DCs, natural killer (NK) cells, and TAMs to significantly enhance their anti-cancer effects.185 Several immune checkpoint-based therapeutic strategies have been developed, such as PD-1/PD-L1 and CTLA-4, to promote T-cell activation and control cancer progression.216,217 Mao et al. reported a biomacromolecular delivery system that delivers monocyte chemotactic molecules to cancer tissue and attenuates the acidic microenvironment surrounding cancer tissues. This nanodrug carrier significantly inhibits cancer growth through the anti-cancer immune action of T cells, facilitating a cascade amplification of peripheral anti-cancer effects.218 Therapeutic vehicles can enhance the activation of cancer antigen-specific T-cells by the targeted delivery of cancer antigens to antigen-presenting cells in the peripheral lymph nodes or spleen. The use of antigen-capturing nanocarrier particles (AC-NPs) can improve cancer immunotherapy. AC-NPs can be enriched in lymph nodes, enhance the presentation of cancer antigens by APCs, and increase the activation of CD8+ T-cells. AC-NPs cause the expansion of CD8+ cytotoxic T-cells and significantly increase the ratio of CD4T+/Tregs and CD8T+/Tregs. Targeted delivery of mRNA encoding cancer antigens to splenic APCs was achieved by altering the surface charge of the vehicle, which enhanced the activation of antigen-specific CD8+ T-cells and improved the inhibition of aggressively growing murine cancers.219 PLE-IL-12-NPs, prepared using cytokine therapy, selectively bind to cancer cells and remain stable on the cell surface, releasing IL-12 over the course of 24 h to activate T-cells.220 Sun et al. developed an immunostimulatory delivery system for STING agonists that enhanced coordination and promotes nanoparticle self-assembly by exploiting the unique coordination kinetics between the drug and metal ions, supplemented with polyhistidine. The resulting Zinc-Mn-CDN Particle (ZMCP) elicits strong cellular and humoral immune responses, leading to a robust anti-tumor immune response and inhibition of tumor growth.221
The development of cancer immunotherapy has brought hope for more patients and significantly improved their prognosis and survival rate. However, the clinical efficacy of traditional immunotherapy still faces challenges, prompting scientists to explore better solutions. The smart delivery vehicles solve the current challenges of cancer immunotherapy by eliminating immune escape, improving the tumor immunosuppressive microenvironment, and enhancing the anti-tumor effect of the peripheral immune system. The widespread application of smart delivery vehicles in cancer immunotherapy still needs to address some issues, such as the immunogenicity and biocompatibility issues mediated by therapeutic vectors. In addition, the excessive activation of the immune system by immunotherapy drugs can affect the activity of normal cells, so it is necessary to design delivery vehicles reasonably to control the release of therapeutic drugs. In summary, the use of smart delivery vehicles for cancer immunotherapy is of great significance.
6. Smart therapy vehicles in other cancer treatments
Cancer immunotherapy has progressed rapidly; however, its widespread use is hindered by low patient response rates.222 Therefore, scientists have developed several therapeutic modalities to combine with immunotherapy, such as chemotherapy, radiotherapy, and phototherapy, and enhance anti-cancer immune responses.223 Furthermore, the combination of smart therapeutic vehicles loaded with multiple therapeutic agents offers targeted delivery and controlled release, which can enhance the efficiency of combined immunotherapy.224,225
6.1 PDT
PDT has been used clinically to treat various cancers as a local treatment modality that activates photosensitizers in the target organ under light and induces chemical damage through ROS.226 However, the current clinical application of PDT suffers from poor blood circulation, limited cancer accumulation, and the inability of the photosensitizer excitation wavelength to reach the target in vivo.227 Nanoparticle-based PDT employs photosensitizers that generate toxic ROS after laser irradiation, acting as in situ vaccines to destroy cancer cells and enhance cancer immunogenicity.228 Therefore, PDT combined with immunotherapy is an effective strategy for cancer treatment.
Bai et al. successfully developed a nano-interference vehicle for small interfering RNA (siRNA) blocking Cdk4 (siCdk4) delivery in combination with photodynamic therapy. siCdk4y blocked the cell cycle, inhibited cancer cell proliferation, and interfered with PD-L1 expression, promoting cancer antigen presentation. Upon laser irradiation, immunogenic cancer antigens are released under PCN-mediated PDT, enhancing the anti-cancer immune response and the binding of anti-PD-L1 antibodies. This strategy enhances the synergistic effects of PDT and immunotherapy and delays cancer progression (Fig. 11A).229 Based on the hypoxic cancer microenvironment, oxygen nanobubbles were protein-modified to enhance their cancer-targeting and apoptosis-inducing abilities. Copper phthalocyanine has been used as a photosensitizer for cancer combination therapy with anti-HER2 scFv-nCytcl, which exhibited superior z-treatment and alleviated cancer hypoxia in vivo (Fig. 11B).230 NPs were produced using clinically approved human serum albumin as a nanoreactor to encapsulate the photosensitizer chlorin e6 (CA-NPs). CA-NPs produced more ROS and exhibited excellent resistance to photobleaching. Furthermore, CA-NPs were efficiently internalized and localized in lysosomes by cancer cells, and upon irradiation, they rapidly translocated into the cytoplasm, inducing significant cytotoxicity. More importantly, ROS generation and apoptosis experiments demonstrated that the vehicle induced positive PDT effects.231
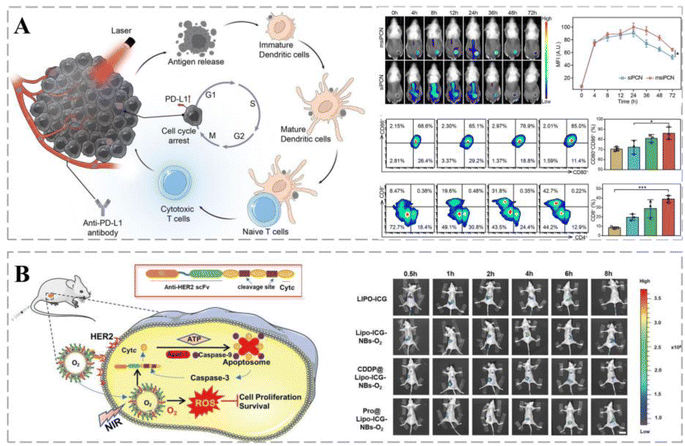 |
| Fig. 11 Smart therapeutic vehicles mediate cancer immune-PDT therapy. (A) Schematic diagram of a nano-interferon combined with anti-PD-L1 antibody to promote cancer photoimmunotherapy. siPCN and msiPCN rapidly target cancer sites for a long duration following administration. Cdk4 inhibition in synergy with PDT can induce the release of strong immunogenic antigens through ICD, allowing for full DC penetration. Reprinted with permission from ref. 229. (B) Pro@Lipo-PS-NBs-O2 combined with NIR irradiation can be concentrated in cancers following endocytosis. Pro@Lipo-ICG-NBs-O2 has long-term cycling characteristics in mice and is highly concentrated in the cancer region at 8 h post-injection. Reprinted with permission from ref. 230. | |
6.2 Gene therapy
Cancers are closely related to genetic alternation, and the emergence of gene therapy is a major breakthrough in the treatment of gene-related diseases.232 Currently, several gene therapy drugs have been clinically approved.233 Owing to their short half-life, naked nucleic acids are susceptible to rapid degradation in circulation in vivo. In addition, both DNA and cell membranes carry negative charges, which impede DNA from approaching the cell membrane,234 leading to a low nucleic acid capture rate by the target cells. Therefore, effective and safe gene delivery systems are urgently needed. Ideal vehicles should protect nucleic acids from degradation and maintain their long-term stability in circulation; however, they should also improve the recognition of target cells and promote their uptake efficiency.235
The cationic polymer polyethylene glycol (PEI) plays a key role in gene delivery. The molecular weight of PEI can affect its transfection efficiency and cytotoxicity; therefore, by effective chemical modification, it is possible to improve PEI transfection activity and reduce its toxicity. ROS-responsive PEI-based fluoropolymers (TKPV) with different degrees of fluorination have excellent therapeutic properties: (1) fluorinated PEI-based fluoropolymers reduce the positive charge density and impart hydrophobic and lipophilic properties to the carrier to resist the effects of serum; (2) the fluorophilic effect makes cellular uptake more effective; (3) ROS-responsive TK linkers allow for the decomposition of polymorphic forms to reduce their cytotoxicity and improve drug release from targets.236 Debele et al. encapsulated polyplexes in methoxy glycol (mPEG)-modified liposomes loaded with DOX in combination with siRNA. The lipid polymer successfully released DOX at low pH, inducing cancer cell death and the siRNA to leave the endosome and inhibit the translation of the FAK protein (Fig. 12A).237 Liu et al. developed a charge conversion biological platform with a three-layer core–shell structure, which effectively resolved the problem of siRNA delivery to glioblastoma (GBM). The resulting nanocomposites can prolong the blood circulation of nucleic acids, have high BBB transmembrane performance, effectively accumulate in cancers, and can be specifically ingested by target cells. In addition, further destruction of the red blood cell membrane (RBCm) and the effective release of siRNA can trigger negative to positive charge transfer in cancer cells, silencing highly effective target genes with strong anti-GBM effects (Fig. 12B).238
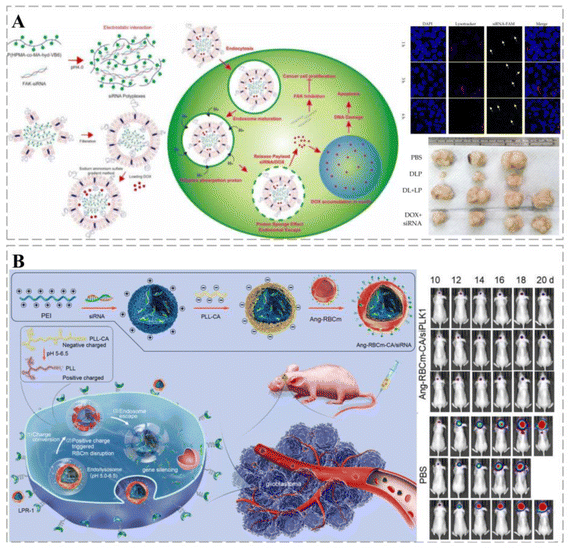 |
| Fig. 12 Smart therapeutic vehicles mediate cancer immune-gene therapy. (A) Schematic diagram of lipopolyplexes loaded with DOX for cancer chemotherapy-gene therapy. siRNA and DOX labeled by fluorescence can be observed in tumor cells, suggesting that lipopolyplexes can deliver drugs into tumor cells and release them. The co-delivery of DOX and siRNA through lipopolyplexes significantly inhibits tumor growth through a synergistic effect and was significantly more effective than free drugs. (B) Schematic diagram of RNAi therapy using a pH-responsive charge-switching bionanocomplex (Ang-RBCm-CA/siRNA) to promote in situ glioblastoma. Ang-RBCm-CA/siRNA with long circulation time and high BBB penetration in vivo is effective in treating glioblastoma in nude mice through charge conversion, low pH-induced membrane disruption, and siRNA release. Reprinted with permission from ref. 238. | |
The long-term safety of vehicle-mediated gene therapy for cancers has not been fully assessed. For example, the autoimmune system may recognize the new substances produced by gene therapy as “foreign” substances, leading to autoimmune diseases. Furthermore, the widespread use of emerging gene-editing therapies has led to related legal issues and adverse consequences. Therefore, the adoption of vehicle-mediated gene therapy for cancers requires long-term follow-up of patients to assess its long-term effect on suppressing cancer progression.
7. Bottlenecks of smart cancer treatment vehicles in cancer therapy
Smart therapeutic carriers can overcome the limitations of traditional therapeutic modalities, including low bioavailability, poor specificity, and drug resistance. Furthermore, they exhibit advantages, such as targeted and controlled drug release and rich and variable drug loading strategies, which are promising for applications in cancer therapy. However, despite the numerous promising cancer therapeutic strategies, the application of smart cancer therapeutic delivery vehicles remains challenging, and only a few studies have successfully applied them clinically.
7.1 EPR effect
Matsumura and Maeda first proposed the EPR effect in cancer targeting in 1986; solid cancers exhibit such an effect.239 Owing to the dysfunction of lymphatic drainage in the cancer TME, carriers with a particle size of <200 nm can enter the interstitial tissue through the blood vessel wall; therefore, drug-loaded carriers exhibit preferential accumulation in solid cancers. It is believed that passive processes dominate the accumulation of nanocarriers in cancer tissues. Current drug-loaded therapeutic vehicles that accumulate in cancers rely on the EPR effect, which is the basis for the passive targeting of vehicles.240,241 Their therapeutic effectiveness is influenced by the different stages of the cancer, intensity of the EPR effect, and physiological barriers.242
Despite their widespread clinical use, passive targeting strategies have many limitations owing to the heterogeneous distribution of blood vessels in solid cancers, resulting in heterogeneous permeability. For small cancers not characterized by angiogenesis or those that metastasize, the efficiency of passive targeting based on the EPR effect is limited.243 In addition, drug delivery vehicles that are based on the EPR effect have limited accumulation in cancers and are highly heterogeneous.244 Therefore, treatment of patients with multiple cancers may fail owing to the lack of the EPR effect. Notably, compared with clinical cancers, it is believed that experimental animal cancer models exhibit more significant EPR effects245 and that nanoparticles have very low delivery efficiency in human cancers.
Other routes have been suggested to address the limited accumulation of drugs in cancers based on the EPR effect (Table 3), such as increasing cancer vascular permeability through physical strategies or using drugs to achieve an increased EPR effect, such as thalidomide.
Table 3 Bottlenecks of smart cancer treatment vehicles and the corresponding solution measures
Treatment bottleneck |
Specific methods |
Examples |
References |
EPR effect |
Physical strategy |
Thermotherapy, ultrasound, and photodynamic therapy |
246–248
|
Regulation of cancer vascular permeability |
Thalidomide and NO |
249–251
|
Increase carrier penetration |
iRGD |
137
|
Intra-stromal spread of cancer |
Reduces cancer interstitial pressure |
Water splitting and decomposition of cancer mesenchyme |
252, and 253 |
Rational drug distribution |
Ultrasmall gold nanosatellite |
254
|
7.2 Diffusion of therapeutic vehicles within the cancer mesenchyme
For smart carriers to deliver drugs to cancer tissues through extravascular penetration, they must be near and enter the cancer cells before exerting their anti-cancer effects. The cancer mesenchyme determines the efficiency of passive targeting. Unlike normal tissues, cancers exhibit high interstitial pressure, especially in their central part, whereas the elevated interstitial fluid pressure (TIP) may create physiological barriers to drug delivery in solid cancers.255 Both the absence of lymphatic vessels and the lack of lymphatic return result in the retention of tissue fluid in the cancer interstitium.256 Excessive TIP can impede the flow of blood and drugs from blood vessels; therefore, only a few nanocarriers may reach the site of action in solid cancers.257 This low delivery efficiency hinders the use of cancer nanodelivery systems in solid cancer treatment (Table 3).
NPs are transported into the cancer mesenchyme via a net force between mesenchymal pressure and drug concentration. Fu et al. grew Ag2S NPs in situ on the ultrathin WS2 surface and designed a “nanomotor” to fabricate the Z-type photocatalytic drug AWS@M. AWS@M can reduce TIP by splitting the water in the interstitium to produce O2, which rapidly enters cancer tissues. At the same time, O2 can be converted into ROS and combined with thermal therapy, increasing the local temperature of the cancer and eliminating deep cancer cells. Upon irradiation with a 660 nm laser, the “nanomotor” can effectively reduce TIP levels in cervical and pancreatic cancers, enhancing intra-cancer drug delivery and inhibiting cancer growth. This nanomotor successfully addresses the issue of blocked intracancer drug delivery and provides a multifunctional strategy for effective cancer growth inhibition.258 The self-assembly of a thermosensitive polymer, superparamagnetic MnFe2O4 nanoparticles, and DOX produced DOX-MTM, which exhibited high drug loading efficiency. Owing to the high content of superparamagnetic nanoparticles, DOX-MTM exhibited good SAR and magneto-thermally sensitive drug release, with a suitable LCST of 42.3 °C. Therefore, the in vitro cytotoxicity was substantially increased through magneto-thermal dependent DOX release and endocytosis. Subsequently, DOX was effectively released under the influence of MH and enhanced the penetration depth into the cancer tissue.259
7.3 Differences between animal models and clinical cancers
Most current studies on the performance of smart vehicles in cancer therapy are based on data from animal models, whereas their translation into human applications remains unexplored. In vivo animal models and clinical cancer patients present a large variability;260 for example, cancer patients often have a combination of underlying diseases and are influenced by psychiatric factors and conditions that cannot be replicated in animal models. Although some preclinical and clinical studies have examined the pharmacokinetics (PK) of different species, detailed data for predicting the safety and efficacy of therapeutic vehicles in clinical settings using animal models are lacking. In addition, even in animal models, drugs do not fully exploit the EPR effect to achieve positive efficacy.261 The efficiency of smart carrier drug delivery based on the EPR effect is correlated with the individual size, which in turn affects the distribution and stability of the carrier in vivo. Previous studies have used the weight of animal models to determine the amount of drug loaded onto the therapeutic vehicle. However, the timing of drug delivery and treatment duration should be adjusted according to the specific clinical condition of the patient. The drug clearance rate in animal models fundamentally differs from that in humans, leading to inconsistencies in treatment duration compared with clinical practice. Therefore, the results obtained from animal models do not accurately reflect the potential effects on clinical patients, limiting the translation of therapeutic vehicles from animal models to clinical practice.
8. Conclusion and outlook
Smart cancer delivery systems have been under development, and scientists have made several advances to actively develop and apply them to promote the effectiveness of cancer treatments. Smart cancer therapeutic delivery vehicles exhibit numerous advantages over traditional drug delivery methods; an essential advantage is their modifiability, which allows them to be designed in various sizes, shapes, and functions as needed. They can be modified or loaded with various drugs to enable the targeted delivery of therapeutic agents. Furthermore, smart carriers can exploit the EPR effect, facilitating the accumulation of drugs within cancerous tissues. Moreover, the complex TME during cancer development necessitates precise drug release at specific locations and periods. Smart therapeutic carriers possess unique properties that prevent drug degradation while releasing them in response to specific stimuli.
However, the efficacy of smart delivery systems has not met expectations, and their clinical application remains limited. The field of clinical translation is in its early stages. Furthermore, the EPR effects in cancer tissues of different patients can be inconsistent, and active targeting systems remain underdeveloped, rendering cancer drug delivery systems unstable for cancer treatment applications. Meanwhile, animal cancer models are not representative of clinical cancers, leading to discrepancies between the preclinical and clinical trial efficacies of smart drug delivery systems, thereby reducing the success rate of clinical translation.
Therefore, to improve the clinical translation, the development of multifunctional biomaterials that can overcome most biological barriers, such as humanized animal models, is required. At the same time, appropriate smart delivery vehicles should be selected for cancer treatment, and FDA-approved drugs, such as liposomes or polymers, should be used first in clinical practice to accelerate clinical translation and immunomodulation. In addition to controlling the toxicity of immune drugs themselves, the physical and chemical properties of smart delivery vehicles, including size, shape, structure, and surface charge, should also be adjusted to reduce the side effects of nanoparticles and enhance their potential in cancer treatment.262 In addition, the surface functionalization of smart delivery vehicles is another important factor affecting cancer treatment. Surface functionalization can affect intracellular uptake of delivery vehicles, and even affect their processing.263 Therefore, appropriate surface functionalization should also be considered in the future for the development of smart delivery vehicles. Smart delivery vehicles can combine immunotherapy with other therapies such as PDT and gene therapy, significantly enhancing treatment effectiveness and reducing toxic side effects. Further exploration is needed to determine the rationale for combining different treatment regimens and optimize the timing and sequence of drug release. For instance, the timing of PTX or cyclophosphamide injections can impact the anti-cancer T-cell response induced by the CD47 blockade. Additionally, the effectiveness of therapeutic vehicles can vary among patients due to individual heterogeneity. Therefore, personalizing the design of therapeutic vehicles according to each patient's needs is necessary to achieve optimal therapeutic performance. Furthermore, more extensive screening of cancer therapeutic targets should be conducted to identify suitable regimens and optimize personalized combination protocols. The development of smart cancer therapeutic vehicles holds great potential for addressing challenges in cancer treatment. These vehicles can substantially reduce the adverse events associated with immunotherapy. Current advances in diagnostic imaging, immune response monitoring, and prognosis have enhanced the versatility of smart therapeutic vehicles in cancer treatment.
Smart delivery vehicles compensate for the shortcomings of conventional cancer therapies, and their application to all types of cancers has yielded excellent therapeutic results. The study and optimization of biomaterials have expanded the prospects for the broader application of smart delivery carriers in cancer therapy.
List of abbrevisations
AC-NPs | Antigen-capturing nanocarrier particles |
APC | Antigen-presenting cells |
aPDL1 | Anti-PD-L1 blocking antibody |
AuNPs | Gold nucleated nanoparticles |
BBB | Blood–brain barrier |
CA | Contrast agent |
CAF | Cancer-associated fibroblasts |
CA-NPs | Chlorin e6 nanoparticles |
COL | Collagenase |
CPT | Camptothecin |
CRPC | Castrate prostate cancer |
CT | Combination chemotherapy |
Cur | Curcumin |
DAMP | Damage associated molecular pattern |
DCs | Dendritic cells |
DOX | Doxorubicin |
ePC | Egg phosphatidylcholine |
EPR | Enhanced permeability and retention |
ER | Estrogen receptor |
ES | Estrone |
ETP | Etoposide |
Exos | Exosomes |
FA | Folic acid |
FR | Folate receptor |
GBM | Glioblastoma |
GEM | Gemcitabine |
GSH | Glutathione |
GS-HM | Gyroid surface helical microrobot |
HA | Hyaluronic acid |
HD | Hydrodynamic diameter |
HMON | Hollow mesoporous organosilicon nanoparticles |
HYD | Hydrazine |
ICD | Immunogenic cell death |
ICG | Indocyanine green |
IF-γ | Interferon γ |
IMDQ | Imidazoquinoline |
IRI | Irinotecan |
Lf: | Lactoferrin |
MDR | Multidrug resistance |
MDSCs | Myeloid-derived suppressor cells |
MFN | Manganese ferrite nanoparticles |
Mito | Mitoxantrone |
MMC | Mitomycin C |
MNP | Magnetic nanoparticles |
mPEG | Methoxy glycol |
MRI | Magnetic resonance imaging |
MSCs | Mesenchymal stem cells |
Mtx | Methotrexate |
NC | nanocluster |
NDs | Nanodroplets |
NGs | Nanogels |
NIR | Near infra-red |
NK | Natural killer |
NPs | Nanoparticles |
OS | Osteosarcoma |
PAA | Polyacrylic acid |
PCAD | Polycarboxylic acid dextran |
PDA | Polydopamine |
PDNCs | Polydiacetylene nanocarriers |
PDT | Photodynamic therapy |
PEG-FIbu | PEG2k-Fmoc-ibuprofen |
PEI | Cationic polyethylene glycol |
PIC | Polyion complex |
PK | Pharmacokinetics |
PLGA | Poly(lactic acid–glycolic acid) |
PLL | Poly-L-lysine |
PM | Polymeric micelles |
PMNs | Porous magnetite nanoparticles |
PMP | Polymeric pre-drug |
PNPs | Polymeric nanoparticles |
PpIX | Protoporphyrin IX |
PTT | Photothermal therapy |
PTX | Paclitaxel |
QDs | Quantum dots |
RAPA | Rapamycin |
RBCm | Red blood cell membrane |
RIF | Rifampicin |
ROS | Reactive oxygen species |
SDT | Sonodynamic therapy |
siRNA | Small interfering RNA |
SLNs | Solid lipid nanoparticles |
srNPs | Smart stimuli-responsive nanoparticles |
TAMs | Tumor-associated macrophages |
TIP | Interstitial fluid pressure |
TME | Tumor microenvironment |
TNBC | Triple-negative breast cancer |
TPGS |
D-α-tocopheryl polyethylene glycol succinate |
TPP | Two-photon polymerization |
TQR | Tariquidar |
Tregs | Regulatory cells |
ZIF-8 | Zeolite imidazolate framework-8 |
Author contributions
XX: carried out the conceptualization and designed the manuscript. YZ and YG: manuscript writing. LH and WG: collated and produced relevant figures for the manuscript. XW and TM: participated in discussions. All authors read and approved the final manuscript.
Conflicts of interest
The authors declare that they have no known competing financial interests or personal relationships.
Acknowledgements
This study was funded by the Natural Science Foundation of Hunan Province, China (2023JJ60089).
References
- A. Erez and R. J. DeBerardinis, Metabolic dysregulation in monogenic disorders and cancer—finding method in madness, Nat. Rev. Cancer, 2015, 15, 440–448 CrossRef CAS PubMed.
- M. Fane and A. T. Weeraratna, How the ageing microenvironment influences tumour progression, Nat. Rev. Cancer, 2020, 20, 89–106 CrossRef CAS PubMed.
- R. L. Siegel, K. D. Miller, N. S. Wagle and A. Jemal, Cancer statistics, Ca-Cancer J. Clin., 2023, 73, 17–48 CrossRef PubMed.
- Q. Huang, C. Pu, L. Tan, S. Wang, H. Zhang and S. Yu,
et al., Synthesis and biological evaluation of a novel c-Myc inhibitor against colorectal cancer via blocking c-Myc/Max heterodimerization and disturbing its DNA binding, Eur. J. Med. Chem., 2022, 243, 114779 CrossRef CAS PubMed.
- X. Cai, J. Gao, C. Shi, W. z. Guo, D. Guo and S. Zhang, The role of NCAPG in various of tumors, Biomed. Pharmacother., 2022, 155, 113635 CrossRef CAS PubMed.
- K. M. Islam, T. Anggondowati, P. E. Deviany, J. E. Ryan, A. Fetrick and D. Bagenda,
et al., Patient preferences of chemotherapy treatment options and tolerance of chemotherapy side effects in advanced stage lung cancer, BMC Cancer, 2019, 19, 1–9 CrossRef PubMed.
- A. Y. Higgins, T. D. O'Halloran and J. D. Chang, Chemotherapy-induced cardiomyopathy, Heart Failure Rev., 2015, 20, 721–730 CrossRef CAS PubMed.
- H. Zhu, C. Lu, F. Gao, Z. Qian, Y. Yin and S. Kan,
et al., Selenium-enriched Bifidobacterium longum DD98 attenuates irinotecan-induced intestinal and hepatic toxicity in vitro and in vivo, Biomed. Pharmacother., 2021, 143, 112192 CrossRef CAS PubMed.
- R. Pisa and T. M. Kapoor, Chemical strategies to overcome resistance against targeted anticancer therapeutics, Nat. Chem. Biol., 2020, 16, 817–825 CrossRef CAS PubMed.
- Q. Yang, M. Li, X. Yang, Z. Xiao, X. Tong and A. Tuerdi,
et al., Flourishing tumor organoids: History, emerging technology, and application, Bioeng. Transl. Med., 2023, 8, e10559 CrossRef PubMed.
- F. Casaluce and C. Gridelli, Combined chemo-immunotherapy in advanced non-small cell lung cancer: feasible in the elderly?, Expert Opin. Emerging Drugs, 2023, 28, 121–127 CrossRef PubMed.
- A. Aldin, B. Besiroglu, A. Adams, I. Monsef, V. Piechotta and E. Tomlinson,
et al., First-line therapy for adults with advanced renal cell carcinoma: a systematic review and network meta-analysis, Cochrane Database Syst. Rev., 2023, 5, Cd013798 Search PubMed.
- R. M. Feng, Y. N. Zong, S. M. Cao and R. H. Xu, Current cancer situation in China: good or bad news from the 2018 Global Cancer Statistics?, Cancer Commun., 2019, 39, 22 Search PubMed.
- R. Shi, R. Lv, Z. Dong, Q. Cao, R. Wu and S. Liu,
et al., Magnetically-targetable outer-membrane vesicles for sonodynamic eradication of antibiotic-tolerant bacteria in bacterial meningitis, Biomaterials, 2023, 302, 122320 CrossRef CAS PubMed.
- A. Bakrania, Y. Mo, G. Zheng and M. Bhat, RNA nanomedicine in liver diseases, Hepatology, 2023 DOI:10.1097/HEP.0000000000000606.
- S. Sun, W. Lv, S. Li, Q. Zhang, W. He and Z. Min,
et al., Smart Liposomal Nanocarrier Enhanced the Treatment of Ischemic Stroke through Neutrophil Extracellular Traps and Cyclic Guanosine Monophosphate-Adenosine Monophosphate Synthase-Stimulator of Interferon Genes (cGAS-STING) Pathway Inhibition of Ischemic Penumbra, ACS Nano, 2023, 17, 17845–17857 CrossRef CAS PubMed.
- S. Kumar, S. Singh, S. Senapati, A. P. Singh, B. Ray and P. Maiti, Controlled drug release through regulated biodegradation of poly(lactic acid) using inorganic salts, Int. J. Biol. Macromol., 2017, 104, 487–497 CrossRef CAS PubMed.
-
S. A. Dilliard, Q. Cheng, and D. J. Siegwart, On the Mechanism of Tissue-specific mRNA Delivery by Selective Organ Targeting Nanoparticles, Proceedings of the National Academy of Sciences, 2021, vol. 118, p. e2109256118 Search PubMed.
- Y. Wang, D. Zhong, F. Xie, S. Chen, Z. Ma and X. Yang,
et al., Manganese Phosphate-Doxorubicin-Based Nanomedicines Using Mimetic Mineralization for Cancer Chemotherapy, ACS Biomater. Sci. Eng., 2022, 8, 1930–1941 CrossRef CAS PubMed.
- F. Andrade, M. M. Roca-Melendres, M. Llaguno, D. Hide, I. Raurell and M. Martell,
et al., Smart and eco-friendly N-isopropylacrylamide and cellulose hydrogels as a safe dual-drug local cancer therapy approach, Carbohydr. Polym., 2022, 295, 119859 CrossRef CAS PubMed.
- J. Zou, Site-specific delivery of cisplatin and paclitaxel mediated by liposomes: A promising approach in cancer chemotherapy, Environ. Res., 2023, 117111 CrossRef CAS.
- D. Li, T. Ren, Y. Ge, X. Wang, G. Sun and N. Zhang,
et al., A multi-functional hypoxia/esterase dual stimulus responsive and hyaluronic acid-based nanomicelle for targeting delivery of chloroethylnitrosouea, J. Nanobiotechnol., 2023, 21, 291 CrossRef CAS PubMed.
- K. Ratajczak, H. Grel, P. Olejnik, S. Jakiela and M. Stobiecka, Current progress, strategy, and prospects of PD-1/PDL-1 immune checkpoint biosensing platforms for cancer diagnostics, therapy monitoring, and drug screening, Biosens. Bioelectron., 2023, 240, 115644 CrossRef CAS PubMed.
- T. G. Nguyen Cao, J. H. Kang, S. J. Kang, Q. Truong Hoang, H. C. Kang and W. J. Rhee,
et al., Brain endothelial cell-derived extracellular vesicles with a mitochondria-targeting photosensitizer effectively treat glioblastoma by hijacking the blood–brain barrier, Acta Pharm. Sin. B, 2023, 13, 3834–3848 CrossRef PubMed.
- C. Zhang, H. Wang, Q. Liu, S. Dai, G. Tian and X. Wei,
et al., LncRNA CCAT1 facilitates the progression of gastric cancer via PTBP1-mediated glycolysis enhancement, J. Exp. Clin. Cancer Res., 2023, 42, 246 CrossRef CAS.
- B. Sun, J. Liu, H. J. Kim, J. N. B. Rahmat, K. G. Neoh and Y. Zhang, Light-responsive smart nanocarriers for wirelessly controlled photodynamic therapy for prostate cancers, Acta Biomater., 2023, 171, 553–564 CrossRef CAS PubMed.
- Y. Mou, P. Zhang, W.-F. Lai and D. Zhang, Design and applications of liposome-in-gel as carriers for cancer therapy, Drug Delivery, 2022, 29, 3245–3255 CrossRef CAS PubMed.
- H. Ding, J. Zhang, F. Zhang, Y. Xu, W. Liang and Y. Yu, Nanotechnological approaches for diagnosis and treatment of ovarian cancer: a review of recent trends, Drug Delivery, 2022, 29, 3218–3232 CrossRef CAS.
- S. I. Bukhari, S. S. Imam, M. Z. Ahmad, P. R. Vuddanda, S. Alshehri and W. A. Mahdi,
et al., Recent Progress in Lipid Nanoparticles for Cancer Theranostics: Opportunity and Challenges, Pharmaceutics, 2021, 13, 840 CrossRef CAS PubMed.
- S. Zeng, Q. Tang, M. Xiao, X. Tong, T. Yang and D. Yin,
et al., Cell membrane-coated nanomaterials for cancer therapy, Mater. Today Bio, 2023, 20, 100633 CrossRef CAS PubMed.
- A. Askarizadeh, M. Mashreghi, E. Mirhadi, F. Mirzavi, V. H. Shargh and B. Ali,
et al., Doxorubicin-loaded liposomes surface engineered with the matrix metalloproteinase-2 cleavable polyethylene glycol conjugate for cancer therapy, Cancer Nanotechnol., 2023, 14, 1–26 CrossRef.
- H. Ye, X. Chu, Z. Cao, X. Hu, Z. Wang and M. Li,
et al., A novel targeted therapy system for cervical cancer: co-delivery system of antisense LncRNA of MDC1 and oxaliplatin magnetic thermosensitive cationic liposome drug carrier, Int. J. Nanomed., 2021, 16, 1051 CrossRef PubMed.
- E. Khabazian, F. Vakhshiteh, P. Norouzi, Y. Fatahi, R. Dinarvand and F. Atyabi, Cationic liposome decorated with cyclic RGD peptide for targeted delivery of anti-STAT3 siRNA to melanoma cancer cells, J. Drug Targeting, 2022, 30, 522–533 CrossRef CAS PubMed.
- Y. Xie, Z. Ren, H. Chen, H. Tang, M. Zhu and Z. Lv,
et al., A novel estrogen-targeted PEGylated liposome co-delivery oxaliplatin and paclitaxel for the treatment of ovarian cancer, Biomed. Pharmacother., 2023, 160, 114304 CrossRef CAS.
- J. Liu, D. Chi, S. Pan, L. Zhao, X. Wang and D. Wang,
et al., Effective co-encapsulation of doxorubicin and irinotecan for synergistic therapy using liposomes prepared with triethylammonium sucrose octasulfate as drug trapping agent, Int. J. Pharm., 2019, 557, 264–272 CrossRef CAS PubMed.
- Y.-X. Zhu, H.-R. Jia, Q.-Y. Duan, X. Liu, J. Yang and Y. Liu,
et al., Photosensitizer-doped and plasma membrane-responsive liposomes for nuclear drug delivery and multidrug resistance reversal, ACS Appl. Mater. Interfaces, 2020, 12, 36882–36894 CrossRef CAS PubMed.
- S. Michlewska, M. Maly, D. Wójkowska, K. Karolczak, E. Skiba and M. Hołota,
et al., Carbosilane ruthenium metallodendrimer as alternative anti-cancer drug carrier in triple negative breast cancer mouse model: a preliminary study, Int. J. Pharm., 2023, 122784 CrossRef CAS.
- I. Franiak-Pietryga, B. Ziemba, H. Sikorska, M. Jander, W. Kuncman and M. Danilewicz,
et al., Maltotriose-modified poly(propylene imine) Glycodendrimers as a potential novel platform in the treatment of chronic lymphocytic Leukemia. A proof-of-concept pilot study in the animal model of CLL, Toxicol. Appl. Pharmacol., 2020, 403, 115139 CrossRef CAS.
- S. Zhang, V. Lloveras, S. Lope-Piedrafita, P. Calero-Pérez, S. Wu and A. P. Candiota,
et al., Metal-free radical dendrimers as MRI contrast agents for glioblastoma diagnosis: Ex vivo and in vivo approaches, Biomacromolecules, 2022, 23, 2767–2777 CrossRef CAS PubMed.
- W. Zhao, S. Yang, C. Li, F. Li, H. Pang and G. Xu,
et al., Amphiphilic dendritic nanomicelle-mediated delivery of gemcitabine for enhancing the specificity and effectiveness, Int. J. Nanomed., 2022, 3239–3249 CrossRef PubMed.
- Z. Wu, S. Stangl, A. Hernandez-Schnelzer, F. Wang, M. H. Kafshgari and B. D. Ali,
et al., Functionalized Hybrid Iron Oxide–Gold Nanoparticles Targeting Membrane Hsp70 Radiosensitize Triple-Negative Breast Cancer Cells by ROS-Mediated Apoptosis, Cancers, 2023, 15, 1167 CrossRef CAS.
- N. González-Ballesteros, I. Maietta, R. Rey-Méndez, M. Carmen Rodríguez-Argüelles, M. Lastra-Valdor and A. Cavazza,
et al., Gold Nanoparticles Synthesized by an Aqueous Extract of Codium tomentosum as Potential Antitumoral Enhancers of Gemcitabine, Mar. Drugs, 2023, 21, 20 CrossRef PubMed.
- Y. Liu, U. K. Sukumar, N. Jugniot, S. M. Seetharam, A. Rengaramachandran and N. Sadeghipour,
et al., Inhaled Gold Nano-Star Carriers for Targeted Delivery of Triple Suicide Gene Therapy and Therapeutic MicroRNAs to Lung Metastases: Development and Validation in a Small Animal Model, Adv. Ther., 2022, 5, 2200018 CrossRef CAS PubMed.
- M. Paiva Abuçafy, R. C. G. Frem, G. Polinario, F. R. Pavan, H. Zhao and A. Mielcarek,
et al., MIL-100 (Fe) Sub-Micrometric Capsules as a Dual Drug Delivery System, Int. J. Mol. Sci., 2022, 23, 7670 CrossRef PubMed.
- N. Rani, K. Rawat, M. Saini, S. Yadav, S. Syeda and K. Saini,
et al., Comparative In Vitro Anticancer Study of Cisplatin Drug with Green Synthesized ZnO Nanoparticles on Cervical Squamous Carcinoma (SiHa) Cell Lines, ACS Omega, 2023, 8, 14509–14519 CrossRef CAS PubMed.
- S. Zheng, J. Wang, N. Ding, W. Chen, H. Chen and M. Xue,
et al., Prodrug polymeric micelles integrating cancer-associated fibroblasts deactivation and synergistic chemotherapy for gastric cancer, J. Nanobiotechnol., 2021, 19, 1–18 CrossRef PubMed.
- M. J. Jo, H. Ji Shin, M. S. Yoon, S. Y. Kim, C. E. Jin and C.-W. Park,
et al., Evaluation of pH-Sensitive Polymeric Micelles Using Citraconic Amide Bonds for the Co-Delivery of Paclitaxel, Etoposide, and Rapamycin, Pharmaceutics, 2023, 15, 154 CrossRef CAS PubMed.
- L. Guo, X. Qin, L. Xue, J. Y. Yang, Y. Zhang and S. Zhu,
et al., A novel form of docetaxel polymeric micelles demonstrates anti-tumor and ascites-inhibitory activities in animal models as monotherapy or in combination with anti-angiogenic agents, Front. Pharmacol, 2022, 13 DOI:10.3389/fphar.2022.964076.
- D. Rafael, S. Montero, P. Carcavilla, F. Andrade, J. German-Cortés and Z. V. Diaz-Riascos,
et al., Intracellular Delivery of Anti-Kirsten Rat Sarcoma Antibodies Mediated by Polymeric Micelles Exerts Strong In Vitro and In Vivo Anti-Tumorigenic Activity in Kirsten Rat Sarcoma-Mutated Cancers, ACS Appl. Mater. Interfaces, 2023, 15, 10398–10413 CrossRef CAS PubMed.
- M. Schröder, M. Petrova, G. M. Dobrikov, G. Grancharov, D. Momekova and P. D. Petrov,
et al., Micellar Form of a Ferrocene-Containing Camphor Sulfonamide with Improved Aqueous Solubility and Tumor Curing Potential, Pharmaceutics, 2023, 15, 791 CrossRef PubMed.
- S. Sripetthong, F. N. Eze, W. Sajomsang and C. Ovatlarnporn, Development of pH-Responsive N-benzyl-N-O-succinyl Chitosan Micelles Loaded with a Curcumin Analog (Cyqualone) for Treatment of Colon Cancer, Molecules, 2023, 28, 2693 CrossRef CAS PubMed.
- M. S. Kim, M. J. Haney, Y. Zhao, V. Mahajan, I. Deygen and N. L. Klyachko,
et al., Development of exosome-encapsulated paclitaxel to overcome MDR in cancer cells, Nanomed. Nanotechnol. Biol. Med., 2016, 12, 655–664 CrossRef CAS PubMed.
- J.-H. Wang, A. V. Forterre, J. Zhao, D. O. Frimannsson, A. Delcayre and T. J. Antes,
et al., Anti-HER2 scFv-directed extracellular vesicle-mediated mRNA-based gene delivery inhibits growth of HER2-positive human breast tumor xenografts by prodrug activation, Mol. Cancer Ther., 2018, 17, 1133–1142 CrossRef CAS PubMed.
- X. Huang, W. Wu, D. Jing, L. Yang, H. Guo and L. Wang,
et al., Engineered exosome as targeted lncRNA MEG3 delivery vehicles for osteosarcoma therapy, J. Controlled Release, 2022, 343, 107–117 CrossRef CAS PubMed.
- W. Chen, W. Lin, N. Yu, L. Zhang, Z. Wu and Y. Chen,
et al., Activation of Dynamin-Related Protein 1 and Induction of Mitochondrial Apoptosis by Exosome-Rifampicin Nanoparticles Exerts Anti-Osteosarcoma Effect, Int. J. Nanomed., 2022, 5431–5446 CrossRef CAS PubMed.
- Y. Li, C. Huang and Y. Xu, Colon cancer exosome-derived biomimetic nanoplatform for curcumin-mediated sonodynamic therapy and calcium overload, Front. bioeng. biotechnol., 2022, 10 DOI:10.3389/fbioe.2022.1069676.
- Y. Gu, Y. Du, L. Jiang, X. Tang, A. Li and Y. Zhao,
et al., αvβ3 integrin-specific exosomes engineered with cyclopeptide for targeted delivery of triptolide against malignant melanoma, J. Nanobiotechnol., 2022, 20, 1–20 CrossRef PubMed.
- C. Feng, Z. Xiong, C. Wang, X. Wen, H. Xiao and K. Xie,
et al., Folic acid-modified Exosome-PH20 enhances the efficiency of therapy via modulation of the tumor microenvironment and directly inhibits tumor cell metastasis, Bioact. Mater., 2021, 6, 963–974 CAS.
- N. D. Lewis, C. L. Sia, K. Kirwin, S. Haupt, G. Mahimkar and Z. Tong,
et al., Exosome Surface Display of IL12 Results in Tumor-Retained Pharmacology with Superior Potency and Limited Systemic Exposure Compared with Recombinant IL12Exosomes Expressing IL12 Promote Antitumor Immunity, Mol. Cancer Ther., 2021, 20, 523–534 CrossRef CAS PubMed.
- S. H. El Moukhtari, E. Garbayo, A. Amundarain, S. Pascual-Gil, A. Carrasco-León and F. Prosper,
et al., Lipid nanoparticles for siRNA delivery in cancer treatment, J. Controlled Release, 2023, 361, 130–146 CrossRef CAS PubMed.
- M. LaRose, R. M. Connolly, C. C. O’Sullivan, V. Velcheti, R. Vilimas and K. Gano,
et al., A Phase I Study of a Combination of Liposomal Irinotecan and Veliparib in Solid Tumors, Oncologist, 2023, 28 DOI:10.1093/oncolo/oyad023.
- Z. Gu, C. G. da Silva, S. Ma, Q. Liu, T. Schomann and F. Ossendorp,
et al., Dual-Targeting Nanoliposome Improves Proinflammatory Immunomodulation of the Tumor Microenvironment, Adv. Healthcare Mater., 2023, e2302046 CrossRef PubMed.
- N. Dhiman, R. Awasthi, B. Sharma, H. Kharkwal and G. T. Kulkarni, Lipid nanoparticles as carriers for bioactive delivery, Front. Chem., 2021, 9, 580118 CrossRef CAS PubMed.
- X. Y. Wong, A. Sena-Torralba, R. Alvarez-Diduk, K. Muthoosamy and A. Merkoçi, Nanomaterials for nanotheranostics: tuning their properties according to disease needs, ACS Nano, 2020, 14, 2585–2627 CrossRef CAS PubMed.
- J. Y. Oh, H. S. Kim, L. Palanikumar, E. M. Go, B. Jana and S. A. Park,
et al., Cloaking nanoparticles with protein corona shield for targeted drug delivery, Nat. Commun., 2018, 9, 4548 CrossRef PubMed.
- D. C. Beatriz, A. R. O. Rodrigues, B. G. Almeida, C. O. Amorim, V. S. Amaral and E. M. S. Castanheira,
et al., Stealth magnetoliposomes based on calcium-substituted magnesium ferrite nanoparticles for curcumin transport and release, Int. J. Mol. Sci., 2020, 21, 3641 CrossRef PubMed.
- S. Chen, G. Morrison, W. Liu, A. Bhamra and R. Chen, A pH-responsive, endosomolytic liposome functionalized with membrane-anchoring, comb-like pseudopeptides for enhanced intracellular delivery and cancer treatment, Biomater. Sci., 2022, 10, 6718–6730 RSC.
- A. J. Da Silva Sanchez, K. Zhao, S. G. Huayamares, M. Z. C. Hatit, M. P. Lokugamage and D. Loughrey,
et al., Substituting racemic ionizable lipids with stereopure ionizable lipids can increase mRNA delivery, J. Controlled Release, 2023, 353, 270–277 CrossRef CAS PubMed.
- B. L. Banik, P. Fattahi and J. L. Brown, Polymeric nanoparticles: the future of nanomedicine, Wiley Interdiscip. Rev.: Nanomed. Nanobiotechnol., 2016, 8, 271–299 Search PubMed.
- J. Nicolas and P. Couvreur, Polymer nanoparticles for the delivery of anticancer drug, Med. Sci., 2017, 33, 11–17 Search PubMed.
- S. Ali, Z. Qamar, S. Md, N. A. Alhakamy, S. Baboota and J. Ali, An insight to brain targeting utilizing polymeric nanoparticles: effective treatment modalities for neurological disorders and brain tumor, Front. bioeng. biotechnol., 2022, 10 DOI:10.3389/fbioe.2022.788128.
- A. Zielińska, F. Carreiró, A. M. Oliveira, A. Neves, B. Pires and D. N. Venkatesh,
et al., Polymeric nanoparticles: production, characterization, toxicology and ecotoxicology, Molecules, 2020, 25, 3731 CrossRef PubMed.
- C. Ni, Z. Ouyang, G. Li, J. Liu, X. Cao and L. Zheng,
et al., A tumor microenvironment-responsive core–shell tecto dendrimer nanoplatform for magnetic resonance imaging-guided and cuproptosis-promoted chemo-chemodynamic therapy, Acta Biomater., 2023, 164, 474–486 CrossRef CAS PubMed.
- M. Mu, F. A. M. Leermakers, J. Chen, M. Holmes and R. Ettelaie, Effect of polymer architecture on the adsorption behaviour of amphiphilic copolymers: A theoretical study, J. Colloid Interface Sci., 2023, 644, 333–345 CrossRef CAS PubMed.
- S. Chowdhury, I. Toth and R. J. Stephenson, Dendrimers in vaccine delivery: Recent progress and advances, Biomaterials, 2022, 280, 121303 CrossRef CAS PubMed.
- Z. Bober, D. Bartusik-Aebisher and D. Aebisher, Application of Dendrimers in Anticancer Diagnostics and Therapy, Molecules, 2022, 27, 3237 CrossRef CAS PubMed.
- H. -J. Hsu, J. Bugno, S. -r. Lee and S. Hong, Dendrimer-based nanocarriers: a versatile platform for drug delivery, Wiley Interdiscip. Rev.: Nanomed. Nanobiotechnol., 2017, 9, e1409 Search PubMed.
- R. Yang, S. Xia, T. Ye, J. Yao, R. Zhang and S. Wang,
et al., Synthesis of a novel polyamidoamine dendrimer conjugating with alkali blue as a lymphatic tracer and study on the lymphatic targeting in vivo, Drug Delivery, 2016, 23, 2298–2308 CrossRef CAS PubMed.
- M. Rogosnitzky and S. Branch, Gadolinium-based contrast agent toxicity: a review of known and proposed mechanisms, BioMetals, 2016, 29, 365–376 CrossRef CAS PubMed.
- Z. Lyu, L. Ding, A. Tintaru and L. Peng, Self-assembling supramolecular dendrimers for biomedical applications: Lessons learned from poly(amidoamine) dendrimers, Acc. Chem. Res., 2020, 53, 2936–2949 CrossRef CAS PubMed.
- S. Paroha, J. Verma, R. Dhar Dubey, R. Prasad Dewangan, N. Molugulu and R. A. Bapat,
et al., Recent advances and prospects in gemcitabine drug delivery systems, Int. J. Pharm., 2021, 592, 120043 CrossRef CAS PubMed.
- Y. Wen, M. Xu, X. Liu, X. Jin, J. Kang and D. Xu,
et al., Magnetofluorescent nanohybrid comprising polyglycerol grafted carbon dots and iron oxides: Colloidal synthesis and applications in cellular imaging and magnetically enhanced drug delivery, Colloids Surf., B, 2019, 173, 842–850 CrossRef CAS PubMed.
- R. Bilan, A. Ametzazurra, K. Brazhnik, S. Escorza, D. Fernández and M. Uríbarri,
et al., Quantum-dot-based suspension microarray for multiplex detection of lung cancer markers: preclinical validation and comparison with the Luminex xMAP® system, Sci. Rep., 2017, 7, 1–10 CrossRef PubMed.
- H. Daraee, E. Ali, E. Abbasi, S. F. Aval, M. Kouhi and A. Akbarzadeh, Application of gold nanoparticles in biomedical and drug delivery, Artif. Cells, Nanomed., Biotechnol., 2016, 44, 410–422 CrossRef CAS PubMed.
- W. Liu, Y. Pan, W. Xiao, H. Xu, D. Liu and F. Ren,
et al., Recent developments on zinc (ii) metal–organic framework nanocarriers for physiological pH-responsive drug delivery, MedChemComm, 2019, 10, 2038–2051 RSC.
- A. M. Abu-Dief, M. Alsehli, A. Al-Enizi and A. Nafady, Recent Advances in Mesoporous Silica Nanoparticles for Targeted Drug Delivery Applications, Curr. Drug Delivery, 2022, 19, 436–450 CrossRef CAS PubMed.
- X. Zhang, L. Li, M. Zhang, L. Zhang, S. Liu and J. Guo,
et al., Intelligent recognition of CTCs from gallbladder cancer by ultrasensitive electrochemical cytosensor and diagnosis of chemotherapeutic resistance, Biosens. Bioelectron., 2023, 228, 115183 CrossRef CAS PubMed.
- Y. Wang, H. Song, M. Yu, C. Xu, Y. Liu and J. Tang,
et al., Room temperature synthesis of dendritic mesoporous silica nanoparticles with small sizes and enhanced mRNA delivery performance, J. Mater. Chem. B, 2018, 6, 4089–4095 RSC.
- S. Dong, Z. Feng, R. Ma, T. Zhang, J. Jiang and Y. Li,
et al., Engineered Design of a Mesoporous Silica Nanoparticle-Based Nanocarrier for Efficient mRNA Delivery in Vivo, Nano Lett., 2023, 23, 2137–2147 CrossRef CAS PubMed.
-
C. Guo, X. Hou, X. Liu, C. Sun, D. Chen, and M. Kong, Novel Dual CAFs and Cancer Cell Targeting Nano-Drug Delivery System for Anti-fibrosis Mechanism of Liver Cancer, 2021 Search PubMed.
- M. J. Jo, Y. H. Jo, Yu J. Lee, C.-W. Park, J.-S. Kim and J. T. Hong,
et al., Physicochemical, pharmacokinetic, and toxicity evaluation of methoxy poly(ethylene glycol)-b-poly(d, l-Lactide) polymeric micelles encapsulating alpinumisoflavone extracted from unripe Cudrania tricuspidata fruit, Pharmaceutics, 2019, 11, 366 CrossRef CAS PubMed.
- J. Lu, A. Gu, W. Wang, A. Huang, B. Han and H. Zhong, Polymeric micellar paclitaxel (pm-Pac) prolonged overall survival for NSCLC patients without pleural metastasis, Int. J. Pharm., 2022, 623, 121961 CrossRef CAS PubMed.
- M. Shi, A. Gu, H. Tu, C. Huang, H. Wang and Z. Yu,
et al., Comparing nanoparticle polymeric micellar paclitaxel and solvent-based paclitaxel as first-line treatment of advanced non-small-cell lung cancer: an open-label, randomized, multicenter, phase III trial, Ann. Oncol., 2021, 32, 85–96 CrossRef CAS PubMed.
- Z. Wang, X. Deng, J. Ding, W. Zhou, Z. Xing and G. Tang, Mechanisms of drug release in pH-sensitive micelles for tumour targeted drug delivery system: A review, Int. J. Pharm., 2018, 535, 253–260 CrossRef CAS PubMed.
- J. Cao, T. Su, L. Zhang, R. Liu, G. Wang and B. He,
et al., Polymeric micelles with citraconic amide as pH-sensitive bond in backbone for anticancer drug delivery, Int. J. Pharm., 2014, 471, 28–36 CrossRef CAS PubMed.
- D. Michiel Pegtel and S. J. Gould, Exosomes, Annu. Rev. Biochem., 2019, 88, 487–514 CrossRef PubMed.
- J. Liu, L. Ren, S. Li, W. Li, X. Zheng and Y. Yang,
et al., The biology, function, and applications of exosomes in cancer, Acta Pharm. Sin. B, 2021, 11, 2783–2797 CrossRef CAS PubMed.
- A. Rezabakhsh, E. Sokullu and R. Rahbarghazi, Applications, challenges and prospects of mesenchymal stem cell exosomes in regenerative medicine, Stem Cell Res. Ther., 2021, 12, 1–8 CrossRef PubMed.
- H. Choi, K. Choi, D.-H. Kim, B.-K. Oh, H. Yim and S. Jo,
et al., Strategies for Targeted Delivery of Exosomes to the Brain: Advantages and Challenges, Pharmaceutics, 2022, 14, 672 CrossRef CAS PubMed.
- D. Yuan, Y. Zhao, W. A. Banks, K. M. Bullock, M. Haney and E. Batrakova,
et al., Macrophage exosomes as natural nanocarriers for protein delivery to inflamed brain, Biomaterials, 2017, 142, 1–12 CrossRef CAS PubMed.
- F. Semcheddine, N. E. I. Guissi, W. Liu, L. Gang, H. Jiang and X. Wang, Rapid and label-free cancer theranostics via in situ bio-self-assembled DNA–gold nanostructures loaded exosomes, Mater. Horiz., 2021, 8, 2771–2784 RSC.
- Gi-H. Nam, Y. Choi, Gi B. Kim, S. Kim, S. A. Kim and I.-S. Kim, Emerging prospects of exosomes for cancer treatment: from conventional therapy to immunotherapy, Adv. Mater., 2020, 32, 2002440 CrossRef CAS PubMed.
- M. Ahmadi and J. Rezaie, Ageing and mesenchymal stem cells derived exosomes: Molecular insight and challenges, Cell Biochem. Funct., 2021, 39, 60–66 CrossRef CAS PubMed.
- M. Babaei and J. Rezaie, Application of stem cell-derived exosomes in ischemic diseases: opportunity and limitations, J. Transl. Med., 2021, 19, 1–11 CrossRef PubMed.
- T. Yang, P. Martin, B. Fogarty, A. Brown, K. Schurman and R. Phipps,
et al., Exosome delivered anticancer drugs across the blood–brain barrier for brain cancer therapy in Danio rerio, Pharm. Res., 2015, 32, 2003–2014 CrossRef CAS PubMed.
- S. Zheng, M. C. Hoang, V. D. Nguyen, G. Go, M. Nan and B. A. Darmawan,
et al., Microrobot with Gyroid Surface and Gold Nanostar for High Drug Loading and Near-Infrared-Triggered Chemo-Photothermal Therapy, Pharmaceutics, 2022, 14, 2393 CrossRef CAS PubMed.
- A. Granja, C. Nunes, C. T. Sousa and S. Reis, Folate receptor-mediated delivery of mitoxantrone-loaded solid lipid nanoparticles to breast cancer cells, Biomed. Pharmacother., 2022, 154, 113525 CrossRef CAS PubMed.
- Z. Chen, W. Wang, Y. Li, W. Cui, P. Zhong and D. He,
et al., Folic acid-modified erythrocyte membrane loading dual drug for targeted and chemo-photothermal synergistic cancer therapy, Mol. Pharm., 2020, 18, 386–402 CrossRef PubMed.
- J. S. Shankaranarayanan, J. R. Kanwar, A. Jalil Abd Al-Juhaishi and R. K. Kanwar, Doxorubicin conjugated to immunomodulatory anticancer lactoferrin displays improved cytotoxicity overcoming prostate cancer chemo resistance and inhibits tumour development in TRAMP mice, Sci. Rep., 2016, 6, 1–16 CrossRef PubMed.
- X. Mo, Z. Zheng, Y. He, H. Zhong, X. Kang and M. Shi,
et al., Antiglioma via regulating oxidative stress and remodeling tumor-associated macrophage using lactoferrin-mediated biomimetic codelivery of simvastatin/fenretinide, J. Controlled Release, 2018, 287, 12–23 CrossRef CAS PubMed.
- M. Sharifi, A. Hasan, N. M. Q. Nanakali, A. Salihi, F. Ali Qadir and H. A. Muhammad,
et al., Combined chemo-magnetic field-photothermal breast cancer therapy based on porous magnetite nanospheres, Sci. Rep., 2020, 10, 1–15 CrossRef PubMed.
- J. Li, B. Esteban-Fernández de Ávila, W. Gao, L. Zhang and J. Wang, Micro/nanorobots for biomedicine: Delivery, surgery, sensing, and detoxification, Sci. Robot., 2017, 2 DOI:10.1126/scirobotics.aam6431.
- H. Ceylan, I. C. Yasa, O. Yasa, A. F. Tabak, J. Giltinan and M. Sitti, 3D-printed biodegradable microswimmer for theranostic cargo delivery and release, ACS Nano, 2019, 13, 3353–3362 CrossRef CAS PubMed.
- X. Ma, K. Hahn and S. Sanchez, Catalytic mesoporous Janus nanomotors for active cargo delivery, J. Am. Chem. Soc., 2015, 137, 4976–4979 CrossRef CAS PubMed.
- Z. Wu, Y. Wu, W. He, X. Lin, J. Sun and Q. He, Self-propelled polymer-based multilayer nanorockets for transportation and drug release, Angew. Chem., Int. Ed., 2013, 52, 7000–7003 CrossRef CAS PubMed.
- S. Comincini, F. Manai, M. Sorrenti, S. Perteghella, C. D’Amato and D. Miele,
et al., Development of Berberine-Loaded Nanoparticles for Astrocytoma Cells Administration and Photodynamic Therapy Stimulation, Pharmaceutics, 2023, 15 DOI:10.3390/pharmaceutics15041078.
- A. De, P. Roychowdhury, N. R. Bhuyan, Y. T. Ko, S. K. Singh and D. Kamal,
et al., Folic Acid Functionalized Diallyl Trisulfide–Solid Lipid Nanoparticles for Targeting Triple Negative Breast Cancer, Molecules, 2023, 28, 1393 CrossRef CAS PubMed.
- Y. Zhang, C. F. Lima and L. R. Rodrigues,
In vitro evaluation of bovine lactoferrin potential as an anticancer agent, Int. Dairy J., 2015, 40, 6–15 CrossRef CAS.
- J. Wang, Q. Li, Y. Ou, K. Li, Z. Han and P. Wang,
et al., Recombination adenovirus-mediated human lactoferrin cDNA inhibits the growth of human MCF-7 breast cancer cells, J. Pharm. Pharmacol., 2012, 64, 457–463 CrossRef CAS PubMed.
- X.-Bo Zhang, Si-Qi Xu, Yi-G. Hui, H.-Yu Zhou, Yi-C. Hu and R.-H. Zhang,
et al., Lactotransferrin promotes intervertebral disc degeneration by regulating Fas and inhibiting human nucleus pulposus cell apoptosis, Aging, 2022, 14, 4572 CrossRef CAS PubMed.
- M. Yuliana Kosim, T. Fukazawa, M. Miyauchi, N. Hirohashi and K. Tanimoto, p53 status modifies cytotoxic activity of lactoferrin under hypoxic conditions, Front. Pharmacol, 2022, 13 DOI:10.3389/fphar.2022.988335.
- R. Jiang and B. Lönnerdal, Bovine lactoferrin and lactoferricin exert antitumor activities on human colorectal cancer cells (HT-29) by activating various signaling pathways, Biochem. Cell Biol., 2017, 95, 99–109 CrossRef CAS PubMed.
- C. Luzi, F. Brisdelli, R. Iorio, A. Bozzi, V. Carnicelli and A. D. Giulio,
et al., Apoptotic effects of bovine apo-lactoferrin on HeLa tumor cells, Cell Biochem. Funct., 2017, 35, 33–41 CrossRef CAS PubMed.
- O. E. Ahmed, M. A. Abdelmoneem, I. A. Hassanin, M. M. Abd Elwakil, M. A. Elnaggar and S. Mokhtar,
et al., Lactoferrin, a multi-functional glycoprotein: Active therapeutic, drug nanocarrier & targeting ligand, Biomaterials, 2020, 263, 120355 CrossRef PubMed.
- H. S. Kim, S. C. Park, H. J. Kim and D. Y. Lee, Inhibition of DAMP actions in the tumoral microenvironment using lactoferrin-glycyrrhizin conjugate for glioblastoma therapy, Biomater. Res., 2023, 27, 52 CrossRef CAS PubMed.
- M.-M. Song, H.-L. Xu, J.-X. Liang, H.-H. Xiang, R. Liu and Yu-X. Shen, Lactoferrin modified graphene oxide iron oxide nanocomposite for glioma-targeted drug delivery, Mater. Sci. Eng., C, 2017, 77, 904–911 CrossRef CAS PubMed.
- R. Sun, M. Liu, J. Lu, B. Chu, Y. Yang and B. Song,
et al., Bacteria loaded with glucose polymer and photosensitive ICG silicon-nanoparticles for glioblastoma photothermal immunotherapy, Nat. Commun., 2022, 13, 5127 CrossRef CAS PubMed.
- J. Lu, J. Ding, B. Chu, C. Ji, Q. Zhang and Y. Xu,
et al., Inactive Trojan Bacteria as Safe Drug Delivery Vehicles Crossing the Blood–Brain Barrier, Nano Lett., 2023, 23, 4326–4333 CrossRef CAS PubMed.
- D. A. Subramanian, R. Langer and G. Traverso, Mucus interaction to improve gastrointestinal retention and pharmacokinetics of orally administered nano-drug delivery systems, J. Nanobiotechnol., 2022, 20, 362 CrossRef PubMed.
- M. A. Rajora, A. Dhaliwal, M. Zheng, V. Choi, M. Overchuk and J. W. H. Lou,
et al., Quantitative Pharmacokinetics Reveal Impact of Lipid Composition on Microbubble and Nanoprogeny Shell Fate, Adv. Sci., 2024, 11, e2304453 CrossRef PubMed.
- H. Cabral, J. Li, K. Miyata and K. Kataoka, Controlling the biodistribution and clearance of nanomedicines, Nat. Rev. Bioeng., 2024, 2, 214–232 CrossRef.
- A. N. Bokatyi, N. V. Dubashynskaya and Y. A. Skorik, Chemical modification of hyaluronic acid as a strategy for the development of advanced drug delivery systems, Carbohydr. Polym., 2024, 337, 122145 CrossRef CAS PubMed.
- A. Narmani, R. Jahedi, E. Bakhshian-Dehkordi, S. Ganji, M. Nemati and R. Ghahramani-Asl,
et al., Biomedical applications of PLGA nanoparticles in nanomedicine: advances in drug delivery systems and cancer therapy, Expert Opin. Drug Delivery, 2023, 20, 937–954 CrossRef CAS PubMed.
- W. S. Yun, J. Kim, D. K. Lim, D. H. Kim, S. I. Jeon and K. Kim, Recent Studies and Progress in the Intratumoral Administration of Nano-Sized Drug Delivery Systems, Nanomaterials, 2023, 13 DOI:10.3390/nano13152225.
- A. Paillard, F. Hindré, C. Vignes-Colombeix, J. P. Benoit and E. Garcion, The importance of endo-lysosomal escape with lipid nanocapsules for drug subcellular bioavailability, Biomaterials, 2010, 31, 7542–7554 CrossRef CAS PubMed.
- L. Mahalakshmi, M. M. Leena, J. A. Moses and C. Anandharamakrishnan, Micro- and nano-encapsulation of β-carotene in zein protein: size-dependent release and absorption behavior, Food Funct., 2020, 11, 1647–1660 RSC.
- W. Dai, Y. Fan, H. Zhang, X. Wang, Q. Zhang and X. Wang, A comprehensive study of iRGD-modified liposomes with improved chemotherapeutic efficacy on B16 melanoma, Drug Delivery, 2015, 22, 10–20 CrossRef CAS PubMed.
- Y. Zhu, M. Wu, X. Miao, B. Wang, J. He and X. Qiu, Delivery of paclitaxel by carboxymethyl chitosan-functionalized dendritic fibrous nano-silica: Fabrication, characterization, controlled release performance and pharmacokinetics, Int. J. Biol. Macromol., 2024, 256, 128431 CrossRef CAS PubMed.
- S. Chattopadhyay, S. S. Sarkar, S. Saproo, S. Yadav, D. Antil and B. Das,
et al., Apoptosis-targeted gene therapy for non-small cell lung cancer using chitosan-poly-lactic-co-glycolic acid-based nano-delivery system and CASP8 and miRs 29A-B1 and 34A, Front. Bioeng. Biotechnol., 2023, 11, 1188652 CrossRef PubMed.
- S. Badrigilan, F. Heydarpanahi, J. Choupani, M. Jaymand, H. Samadian and M. Hoseini-Ghahfarokhi,
et al., A Review on the Biodistribution, Pharmacokinetics and Toxicity of Bismuth-Based Nanomaterials, Int. J. Nanomed., 2020, 15, 7079–7096 CrossRef CAS PubMed.
- A. Mansour, M. Y. Mahmoud, A. F. Bakr, M. G. Ghoniem, F. A. Adam and I. M. El-Sherbiny, Dual-Enhanced Pluronic Nanoformulated Methotrexate-Based Treatment Approach for Breast Cancer: Development and Evaluation of In Vitro and In Vivo Efficiency, Pharmaceutics, 2022, 14 DOI:10.3390/pharmaceutics14122668.
- H. Zhao, X. Wang, Z. Geng, N. Liang, Q. Li and X. Hu,
et al., Dual-function microneedle array for efficient photodynamic therapy with transdermal co-delivered light and photosensitizers, Lab Chip, 2022, 22, 4521–4530 RSC.
- A. Mondal, A. K. Nayak, P. Chakraborty, S. Banerjee and B. C. Nandy, Natural Polymeric Nanobiocomposites for Anti-Cancer Drug Delivery Therapeutics: A Recent Update, Pharmaceutics, 2023, 15 DOI:10.3390/pharmaceutics15082064.
- Y. Ban, Y. Chu, F. Pan, Z. Guo, Y. Yang and X. Wei,
et al., Lipid-Based Nanocarriers Enabled Oral Delivery of Oleanolic Acid Derivative DKS26 for Diabetes Management, Adv. Healthcare Mater., 2023, 12, e2300639 CrossRef PubMed.
- S. Gholizadeh, E. M. Dolman, R. Wieriks, R. W. Sparidans, W. E. Hennink and R. J. Kok, Anti-GD2 immunoliposomes for targeted delivery of the survivin inhibitor sepantronium bromide (YM155) to neuroblastoma tumor cells, Pharm. Res., 2018, 35, 1–15 CrossRef CAS PubMed.
- C. Liu, W. Liu, Y. Liu, H. Duan, L. Chen and X. Zhang,
et al., Versatile flexible micelles integrating mucosal penetration and intestinal targeting for effectively oral delivery of paclitaxel, Acta Pharm. Sin. B, 2023, 13, 3425–3443 CrossRef CAS PubMed.
- H. Yang, M. Wang, Y. Huang, Q. Qiao, C. Zhao and M. Zhao,
In vitro and in vivo evaluation of a novel mitomycin nanomicelle delivery system, RSC Adv., 2019, 9, 14708–14717 RSC.
- J. Zhao, M. Zhao, C. Yu, X. Zhang, J. Liu and X. Cheng,
et al., Multifunctional folate receptor-targeting and pH-responsive nanocarriers loaded with methotrexate for treatment of rheumatoid arthritis, Int. J. Nanomed., 2017, 12, 6735–6746 CrossRef CAS PubMed.
- F. Zhang, G. Zhu, O. Jacobson, Y. Liu, K. Chen and G. Yu,
et al., Transformative Nanomedicine of an Amphiphilic Camptothecin Prodrug for Long Circulation and High Tumor Uptake in Cancer Therapy, ACS Nano, 2017, 11, 8838–8848 CrossRef CAS PubMed.
- J. Zhang, Y. Lin, Z. Lin, W. Qi, J. Qian and R. Ruan,
et al., Stimuli-Responsive Nanoparticles for Controlled Drug Delivery in Synergistic Cancer Immunotherapy, Adv. Sci., 2021, 9 DOI:10.1002/advs.202103444.
- M. Su, L. Ruan, X. Dong, S. Tian, W. Lang and M. Wu,
et al., Current state of knowledge on intelligent-response biological and other macromolecular hydrogels in biomedical engineering: A review, Int. J. Biol. Macromol., 2023, 227, 472–492 CrossRef CAS PubMed.
- Y. Li, X. Zhang, X. Liu, W. Pan, N. Li and B. Tang, Intelligent stimuli-responsive nano immunomodulators for cancer immunotherapy, Chem. Sci., 2021, 12, 3130–3145 RSC.
- S. Peng, F. Xiao, M. Chen and H. Gao, Tumor-Microenvironment-Responsive Nanomedicine for Enhanced Cancer Immunotherapy, Adv. Sci., 2022, 9, 2103836 CrossRef CAS PubMed.
- C. Roma-Rodrigues, R. Mendes, P. V. Baptista and A. R. Fernandes, Targeting Tumor Microenvironment for Cancer Therapy, Int. J. Mol. Sci., 2019, 20 DOI:10.3390/ijms20040840.
- G. Ye, Y. Jiang, X. Yang, H. Hu, B. Wang and L. Sun,
et al., Smart Nanoparticles Undergo Phase Transition for Enhanced Cellular Uptake and Subsequent Intracellular Drug Release in a Tumor Microenvironment, ACS Appl. Mater. Interfaces, 2018, 10, 278–289 CrossRef CAS PubMed.
- Yi-H. Hsieh, Y.-T. Hsiao and J.-S. Jan, Shell and core cross-linked poly(l-lysine)/poly(acrylic acid) complex micelles, Soft Matter, 2014, 10, 9568–9576 RSC.
- F. Weinberg, N. Ramnath and D. Nagrath, Reactive oxygen species in the tumor microenvironment: an overview, Cancers, 2019, 11, 1191 CrossRef CAS PubMed.
- C. Wang, J. Wang, X. Zhang, S. Yu, D. Wen and Q. Hu,
et al., In situ formed reactive oxygen species–responsive scaffold with gemcitabine and checkpoint inhibitor for combination therapy, Sci. Transl. Med., 2018, 10, eaan3682 CrossRef PubMed.
- B. Wang, S. Van Herck, Y. Chen, X. Bai, Z. Zhong and D. Kim,
et al., Potent and prolonged innate immune activation by enzyme-responsive imidazoquinoline TLR7/8 agonist prodrug vesicles, J. Am. Chem. Soc., 2020, 142, 12133–12139 CrossRef CAS PubMed.
- C. A. Corzo, T. Condamine, L. Lu, M. J. Cotter, J.-I. Youn and P. Cheng,
et al., HIF-1α regulates function and differentiation of myeloid-derived suppressor cells in the tumor microenvironment, J. Exp. Med., 2010, 207, 2439–2453 CrossRef CAS PubMed.
- K. Yang, G. Yu, R. Tian, Z. Zhou, H. Deng and L. Li,
et al., Oxygen-Evolving Manganese Ferrite Nanovesicles for Hypoxia-Responsive Drug Delivery and Enhanced Cancer Chemoimmunotherapy, Adv. Funct. Mater., 2021, 31, 2008078 CrossRef CAS.
- K. Cheng, Y. Ding, Y. Zhao, S. Ye, X. Zhao and Y. Zhang,
et al., Sequentially responsive therapeutic peptide assembling nanoparticles for dual-targeted cancer immunotherapy, Nano Lett., 2018, 18, 3250–3258 CrossRef CAS PubMed.
- C. Xu, Y. Yu, Y. Sun, L. Kong, C. Yang and M. Hu,
et al., Transformable Nanoparticle-Enabled Synergistic Elicitation and Promotion of Immunogenic Cell Death for Triple-Negative Breast Cancer Immunotherapy, Adv. Funct. Mater., 2019, 29, 1905213 CrossRef CAS.
- M. Ismail, S. Khan, F. Khan, S. Noor, H. Sajid and S. Yar,
et al., Prevalence and significance of potential drug-drug interactions among cancer patients receiving chemotherapy, BMC Cancer, 2020, 20, 1–9 CrossRef PubMed.
- N. M. Ayoub, Editorial: Novel Combination Therapies for the Treatment of Solid Cancers, Front. Oncol., 2021, 11, 708943 CrossRef PubMed.
- Y. Gao, Y. Zhu, X. Xu, F. Wang, W. Shen and X. Leng,
et al., Surface PEGylated cancer cell membrane-coated nanoparticles for codelivery of curcumin and doxorubicin for the treatment of multidrug resistant esophageal carcinoma, Front. Cell Dev. Biol., 2021, 9, 688070 CrossRef PubMed.
- S. Zhen, X. Yi, Z. Zhao, X. Lou, F. Xia and B. Z. Tang, Drug delivery micelles with efficient near-infrared photosensitizer for combined image-guided photodynamic therapy and chemotherapy of drug-resistant cancer, Biomaterials, 2019, 218, 119330 CrossRef CAS PubMed.
- Y. Xing, T. Ding, Z. Wang, L. Wang, H. Guan and T. Jia,
et al., Temporally controlled photothermal/photodynamic and combined therapy for overcoming multidrug resistance of cancer by polydopamine nanoclustered micelles, ACS Appl. Mater. Interfaces, 2019, 11, 13945–13953 CrossRef CAS PubMed.
- L. Ruan, J. Chen, C. Du, H. Lu, J. Zhang and X. Cai,
et al., Mitochondrial temperature-responsive drug delivery reverses drug resistance in lung cancer, Bioact. Mater., 2022, 13, 191–199 CAS.
- J. Liu, C. Zhu, L. Xu, D. Wang, W. Liu and K. Zhang,
et al., Nanoenabled intracellular calcium bursting for safe and efficient reversal of drug resistance in tumor cells, Nano Lett., 2020, 20, 8102–8111 CrossRef CAS PubMed.
- F. Zhou, S. Yang, C. Zhao, W. Liu, X. Yao and H. Yu,
et al., gamma-Glutamyl transpeptidase-activatable near-infrared nanoassembly for tumor fluorescence imaging-guided photothermal therapy, Theranostics, 2021, 11, 7045–7056 CrossRef CAS PubMed.
- S. Pan, Y. Zhang, A. Natalia, C. Z. J. Lim, N. R. Y. Ho and B. Chowbay,
et al., Extracellular vesicle drug occupancy enables real-time monitoring of targeted cancer therapy, Nat. Nanotechnol., 2021, 16, 734–742 CrossRef CAS PubMed.
- Y. Zhao, D. Shi, M. Shang, X. Sun, L. Guo and M. Dong,
et al., GRP78-targeted and doxorubicin-loaded nanodroplets combined with ultrasound: a potential novel theranostics for castration-resistant prostate cancer, Drug Delivery, 2022, 29, 203–213 CrossRef CAS PubMed.
- A. Narmani, K. Yavari and J. Mohammadnejad, Imaging, biodistribution and in vitro study of smart 99mTc-PAMAM G4 dendrimer as novel nano-complex, Colloids Surf., B, 2017, 159, 232–240 CrossRef CAS PubMed.
- J. Kong, Y. Li, W. Ma, Y. Du, L. Liu and T. Qu,
et al., A novel vector for magnetic resonance imaging-guided chemo-photothermal therapy for cancer, Front. Oncol., 2022, 12 DOI:10.3389/fonc.2022.972082.
- J. -H. Fang, T. -L. Chiu, W. -C. Huang, Y. -H. Lai, S. -H. Hu and Y. -Y. Chen,
et al., Dual-Targeting Lactoferrin-Conjugated Polymerized Magnetic Polydiacetylene-Assembled Nanocarriers with Self-Responsive Fluorescence/Magnetic Resonance Imaging for In Vivo Brain Tumor Therapy, Adv. Healthcare Mater., 2016, 5, 688–695 CrossRef CAS PubMed.
- S. A. E. Ahmed, D. G. Zayed, M. W. Helmy, S. M. Ebrahim, M. Bahey-El-Din and E. A. Zein-El-Dein,
et al., Lactoferrin-tagged quantum dots-based theranostic nanocapsules for combined COX-2 inhibitor/herbal therapy of breast cancer, Nanomedicine, 2018, 13, 2637–2656 CrossRef PubMed.
- J. Wolchok, Putting the immunologic brakes on cancer, Cell, 2018, 175, 1452–1454 CrossRef CAS PubMed.
- Y. Guo, D. Wang, Q. Song, T. Wu, X. Zhuang and Y. Bao,
et al., Erythrocyte membrane-enveloped polymeric nanoparticles as nanovaccine for induction of antitumor immunity against melanoma, ACS Nano, 2015, 9, 6918–6933 CrossRef CAS PubMed.
- M. Lesouhaitier, C. Dudreuilh, M. Tamain, N. Kanaan, E. Bailly and D. Legoupil,
et al., Checkpoint blockade after kidney transplantation, Eur. J. Cancer, 2018, 96, 111–114 CrossRef PubMed.
- S. Najafi, J. Majidpoor and K. Mortezaee, The impact of microbiota on PD-1/PD-L1 inhibitor therapy outcomes: A focus on solid tumors, Life Sci., 2022, 121138 CrossRef CAS PubMed.
- X. Zhang, H. Cui, W. Zhang, Z. Li and J. Gao, Engineered tumor cell-derived vaccines against cancer: The art of combating poison with poison, Bioact. Mater., 2023, 22, 491–517 CAS.
- F. Dai, J. Kloepper, Z. Amoozgar, D. G. Duda and R. K. Jain, Enhancing cancer immunotherapy using antiangiogenics: opportunities and challenges, Nat. Rev. Clin. Oncol., 2018, 15, 325–340 CrossRef PubMed.
- X. Zhuang, T. Wu, Y. Zhao, X. Hu, Y. Bao and Y. Guo,
et al., Lipid-enveloped zinc phosphate hybrid nanoparticles for codelivery of H-2Kb and H-2Db-restricted antigenic peptides and monophosphoryl lipid A to induce antitumor immunity against melanoma, J. Controlled Release, 2016, 228, 26–37 CrossRef CAS PubMed.
- H. Cabral, H. Kinoh and K. Kataoka, Tumor-targeted nanomedicine for immunotherapy, Acc. Chem. Res., 2020, 53, 2765–2776 CrossRef CAS PubMed.
- M. Xiao, Q. Tang, S. Zeng, Q. Yang, X. Yang and X. Tong,
et al., Emerging biomaterials for tumor immunotherapy, Biomater. Res., 2023, 27, 47 CrossRef CAS PubMed.
- X. Wang, H. Bai, J. Zhang, Z. Wang, J. Duan and H. Cai,
et al., Genetic intratumour heterogeneity remodels the immune microenvironment and induces immune evasion in brain metastasis of lung cancer, J. Thorac. Oncol., 2023, 19, 252–272 CrossRef PubMed.
- J. Zhang, Y. Lin, Z. Lin, W. Qi, J. Qian and R. Ruan,
et al., Stimuli-Responsive Nanoparticles for Controlled Drug Delivery in Synergistic Cancer Immunotherapy, Adv. Sci., 2022, 9, 2103444 CrossRef CAS PubMed.
- Y. Zhang, L. Zeng, M. Wang, Z. Yang, H. Zhang and L. Gao,
et al., RIG-I promotes immune evasion of colon cancer by
modulating PD-L1 ubiquitination, J. Immunother. Cancer., 2023, 11 DOI:10.1136/jitc-2023-007313.
- C. W. Wong, C. Evangelou, K. N. Sefton, R. Leshem, W. Zhang and V. Gopalan,
et al., PARP14 inhibition restores PD-1 immune checkpoint inhibitor response following IFNγ-driven acquired resistance in preclinical cancer models, Nat. Commun., 2023, 14, 5983 CrossRef CAS PubMed.
- S. H. Kiaie, H. Salehi-Shadkami, M. J. Sanaei, M. Azizi, M. Shokrollahi Barough and M. S. Nasr,
et al., Nano-immunotherapy: overcoming delivery challenge of immune checkpoint therapy, J. Nanobiotechnol., 2023, 21, 339 CrossRef PubMed.
- L. Zhao, D. Li, Y. Zhang, Q. Huang, Z. Zhang and C. Chen,
et al., HSP70-Promoter-Driven CRISPR/Cas9 System Activated by Reactive Oxygen Species for Multifaceted Anticancer Immune Response and Potentiated Immunotherapy, ACS Nano, 2022, 16, 13821–13833 CrossRef CAS PubMed.
- Y. Wang, Y. Huang, M. Yang, Y. Yu, X. Chen and L. Ma,
et al., Comprehensive Pan-Cancer Analyses of Immunogenic Cell Death as a Biomarker in Predicting Prognosis and Therapeutic Response, Cancers, 2022, 14, 5952 CrossRef CAS PubMed.
- J. Guo, Z. Yu, D. Sun, Y. Zou, Y. Liu and L. Huang, Two nanoformulations induce reactive oxygen species and immunogenetic cell death for synergistic chemo-immunotherapy eradicating colorectal cancer and hepatocellular carcinoma, Mol. Cancer, 2021, 20, 1–17 Search PubMed.
- B. Cui, L. Song, Q. Wang, K. Li, Q. He and X. Wu,
et al., Non-small cell lung cancers (NSCLCs) oncolysis using coxsackievirus B5 and synergistic DNA-damage response inhibitors, Signal Transduction Targeted Ther., 2023, 8, 366 CrossRef CAS PubMed.
- J. F. Yang, X. Xing, L. Luo, X. W. Zhou, J. X. Feng and K. B. Huang,
et al., Mitochondria-ER contact mediated by MFN2-SERCA2 interaction supports CD8(+) T cell metabolic fitness and function in tumors, Sci. Immunol., 2023, 8, eabq2424 CrossRef CAS PubMed.
- S. Zhou, S. Qi, J. Ji and Y. Luan, A Nanoplatform to Amplify Apoptosis-to-Pyroptosis Immunotherapy via Immunomodulation of Myeloid-Derived Suppressor Cells, ACS Appl. Mater. Interfaces, 2021, 13, 47407–47417 CrossRef CAS PubMed.
-
A. E. Denton, E. W. Roberts, and T. F. Douglas, Stromal cells in the tumor microenvironment, Stromal immunology, 2018, pp. 99–114 Search PubMed.
- Y. Huang, S. Wu, L. Zhang, Q. Deng, J. Ren and X. Qu, A Metabolic Multistage Glutathione Depletion Used for Tumor-Specific Chemodynamic Therapy, ACS Nano, 2022, 16, 4228–4238 CrossRef CAS PubMed.
- L. Lei, B. Ma, C. Xu and H. Liu, Emerging tumor-on-chips with electrochemical biosensors, TrAC, Trends Anal. Chem., 2022, 153 DOI:10.1016/j.trac.2022.116640.
- I. Vitale, G. Manic, L. M. Coussens, G. Kroemer and L. Galluzzi, Macrophages and Metabolism in the Tumor Microenvironment, Cell Metab., 2019, 30, 36–50 CrossRef CAS PubMed.
- J. M. Pitt, A. Marabelle, A. Eggermont, J. C. Soria, G. Kroemer and L. Zitvogel, Targeting the tumor microenvironment: removing obstruction to anticancer immune responses and immunotherapy, Ann. Oncol., 2016, 27, 1482–1492 CrossRef CAS PubMed.
- C. Song, H. Phuengkham, Y. S. Kim, V. V. Dinh, I. Lee and I. W. Shin,
et al., Syringeable immunotherapeutic nanogel reshapes tumor microenvironment and prevents tumor metastasis and recurrence, Nat. Commun., 2019, 10, 3745 CrossRef PubMed.
- Y. Choi, Y. Shi, C. L. Haymaker, A. Naing, G. Ciliberto and J. Hajjar, T-cell agonists in cancer immunotherapy, J. Immunother. Cancer., 2020, 8 DOI:10.1136/jitc-2020-000966.
- L. Tang, Y. Zheng, M. B. Melo, L. Mabardi, A. P. Castano and Y. Q. Xie,
et al., Enhancing T cell therapy through TCR-signaling-responsive nanoparticle drug delivery, Nat. Biotechnol., 2018, 36, 707–716 CrossRef CAS PubMed.
- H. Chen, X. Cong, C. Wu, X. Wu, J. Wang and K. Mao,
et al., Intratumoral delivery of CCL25 enhances immunotherapy against triple-negative breast cancer by recruiting CCR9+ T cells, Sci. Adv., 2020, 6, eaax4690 CrossRef CAS PubMed.
- R. Kalluri, The biology and function of fibroblasts in cancer, Nat. Rev. Cancer, 2016, 16, 582–598 CrossRef CAS PubMed.
- E. Hirata and E. Sahai, Tumor microenvironment and differential responses to therapy, Cold Spring Harbor Perspect. Med., 2017, 7, a026781 CrossRef PubMed.
- L. V. Ireland and A. Mielgo, Macrophages and Fibroblasts, Key Players in Cancer Chemoresistance, Front. Cell Dev. Biol., 2018, 6, 131 CrossRef PubMed.
- D. Kovács, N. Igaz, A. Marton, A. Ronavari, P. Belteky and L. Bodai,
et al., Core–shell nanoparticles suppress metastasis and modify the tumour-supportive activity of cancer-associated fibroblasts, J. Nanobiotechnol., 2020, 18, 18 CrossRef PubMed.
- X. Zhao, J. Pan, W. Li, W. Yang, L. Qin and Y. Pan, Gold nanoparticles enhance cisplatin delivery and potentiate chemotherapy by decompressing colorectal cancer vessels, Int. J. Nanomed., 2018, 13, 6207–6221 CrossRef CAS PubMed.
- T. Tang, X. Huang, G. Zhang, Z. Hong, X. Bai and T. Liang, Advantages of targeting the tumor immune microenvironment over blocking immune checkpoint in cancer immunotherapy, Signal Transduction Targeted Ther., 2021, 6, 72 CrossRef PubMed.
- X. Dai, Y. Du, Y. Li and F. Yan, Nanomaterials-based precision sonodynamic therapy enhancing immune checkpoint blockade: A promising strategy targeting solid tumor, Mater. Today Bio, 2023, 23, 100796 CrossRef CAS PubMed.
- S. Serratì, R. Di Fonte, L. Porcelli, S. De Summa, I. De Risi and L. Fucci,
et al., Circulating extracellular vesicles are monitoring biomarkers of anti-PD1 response and enhancer of tumor progression and immunosuppression in metastatic melanoma, J. Exp. Clin. Cancer Res., 2023, 42, 251 CrossRef PubMed.
- S. T. Chuang, B. Conklin, J. B. Stein, G. Pan and K. B. Lee, Nanotechnology-enabled immunoengineering approaches to advance therapeutic applications, Nano Convergence, 2022, 9, 19 CrossRef CAS PubMed.
- N. McGranahan, A. J. Furness, R. Rosenthal, S. Ramskov, R. Lyngaa and S. K. Saini,
et al., Clonal neoantigens elicit T cell immunoreactivity and sensitivity to immune checkpoint blockade, Science, 2016, 351, 1463–1469 CrossRef CAS PubMed.
- D. T. Le, J. N. Durham, K. N. Smith, H. Wang, B. R. Bartlett and L. K. Aulakh,
et al., Mismatch repair deficiency predicts response of solid tumors to PD-1 blockade, Science, 2017, 357, 409–413 CrossRef CAS PubMed.
- K. Mao, X. Cong, L. Feng, H. Chen, J. Wang and C. Wu,
et al., Intratumoral delivery of M-CSF by calcium crosslinked polymer micelles enhances cancer immunotherapy, Biomater. Sci., 2019, 7, 2769–2776 RSC.
- Y. Min, K. C. Roche, S. Tian, M. J. Eblan, K. P. McKinnon and J. M. Caster,
et al., Antigen-capturing nanoparticles improve the abscopal effect and cancer immunotherapy, Nat. Nanotechnol., 2017, 12, 877–882 CrossRef CAS PubMed.
- A. E. Barberio, S. G. Smith, I. S. Pires, S. Iyer, F. Reinhardt and M. B. Melo,
et al., Layer-by-layer interleukin-12 nanoparticles drive a safe and effective response in ovarian tumors, Bioeng. Transl. Med., 2023, 8, e10453 CrossRef CAS PubMed.
- X. Sun, X. Huang, K. S. Park, X. Zhou, A. A. Kennedy and C. D. Pretto,
et al., Self-Assembled STING-Activating Coordination Nanoparticles for Cancer Immunotherapy and Vaccine Applications, ACS Nano, 2024, 18, 10439–10453 CrossRef CAS PubMed.
- P. Sharma, S. Hu-Lieskovan, J. A. Wargo and A. Ribas, Primary, adaptive, and acquired resistance to cancer immunotherapy, Cell, 2017, 168, 707–723 CrossRef CAS PubMed.
- J. Nam, S. Son, K. S. Park, W. Zou, L. D. Shea and J. J. Moon, Cancer nanomedicine for combination cancer immunotherapy, Nat. Rev. Mater., 2019, 4, 398–414 CrossRef.
- J. Shi, P. W. Kantoff, R. Wooster and O. C. Farokhzad, Cancer nanomedicine: progress, challenges and opportunities, Nat. Rev. Cancer, 2017, 17, 20–37 CrossRef CAS PubMed.
- S. Zanganeh, G. Hutter, R. Spitler, O. Lenkov, M. Mahmoudi and A. Shaw,
et al., Iron oxide nanoparticles inhibit tumour growth by inducing pro-inflammatory macrophage polarization in tumour tissues, Nat. Nanotechnol., 2016, 11, 986–994 CrossRef CAS PubMed.
- T. C. Pham, V.-N. Nguyen, Y. Choi, S. Lee and J. Yoon, Recent strategies to develop innovative photosensitizers for enhanced photodynamic therapy, Chem. Rev., 2021, 121, 13454–13619 CrossRef CAS PubMed.
- L. Menilli, C. Milani, E. Reddi and F. Moret, Overview of nanoparticle-based approaches for the combination of photodynamic therapy (PDT) and chemotherapy at the preclinical stage, Cancers, 2022, 14, 4462 CrossRef CAS PubMed.
- X. Li, J. F. Lovell, J. Yoon and X. Chen, Clinical development and potential of photothermal and photodynamic therapies for cancer, Nat. Rev. Clin. Oncol., 2020, 17, 657–674 CrossRef PubMed.
- X.-F. Bai, Y. Chen, M.-Z. Zou, C.-X. Li, Y. Zhang and M.-J. Li,
et al., Homotypic Targeted Photosensitive Nanointerferer for Tumor Cell Cycle Arrest to Boost Tumor Photoimmunotherapy, ACS Nano, 2022, 16, 18555–18567 CrossRef CAS PubMed.
- J. Tian, S. Wan, J. Tian, L. Liu, J. Xia and Y. Hu,
et al., Anti-HER2 scFv-nCytc-Modified Lipid-Encapsulated Oxygen Nanobubbles Prepared with Bulk Nanobubble Water for Inducing Apoptosis and Improving Photodynamic Therapy, Small, 2023, 2206091 CrossRef CAS PubMed.
- J. Luo, Z. Miao, X. Huang, Y. Yang, M. Liu and G. Shen,
et al., Translational albumin nanocarrier caging photosensitizer for efficient cancer photodynamic therapy, Front. bioeng. biotechnol., 2023, 11 DOI:10.3389/fbioe.2023.1132591.
- Y. Yu, Y. Gao, L. He, B. Fang, W. Ge and P. Yang,
et al., Biomaterial-based gene therapy, MedComm, 2023, 4, e259 CrossRef CAS PubMed.
- A. Shahryari, M. Saghaeian Jazi, S. Mohammadi, H. R. Nikoo, Z. Nazari and E. Sadat Hosseini,
et al., Development and clinical translation of approved gene therapy products for genetic disorders, Front. Genet., 2019, 10, 868 CrossRef CAS PubMed.
- Z. Jia, J. Choi and S. Park, Surface Charge Density-Dependent DNA Capture through Polymer Planar Nanopores, ACS Appl. Mater. Interfaces, 2018, 10, 40927–40937 CrossRef CAS PubMed.
- M. Liu, L. Wang, Y. Lo, S. C.-C. Shiu, A. B. Kinghorn and J. A. Tanner, Aptamer-enabled nanomaterials for therapeutics, drug targeting and imaging, Cells, 2022, 11, 159 CrossRef CAS PubMed.
- H. Peng, D. Yang, R. Chen, P. Qiu and M. Chen, ROS responsive polyethylenimine-based fluorinated polymers for enhanced transfection efficiency and lower cytotoxicity, Bosnian J. Basic Med. Sci., 2022, 22, 593 Search PubMed.
- T. A. Debele, C.-K. Chen, L.-Y. Yu and C.-L. Lo, Lipopolyplex-Mediated Co-Delivery of Doxorubicin and FAK siRNA to Enhance Therapeutic Efficiency of Treating Colorectal Cancer, Pharmaceutics, 2023, 15, 596 CrossRef CAS PubMed.
- Y. Liu, Y. Zou, C. Feng, A. Lee, J. Yin and R. Chung,
et al., Charge conversional biomimetic nanocomplexes as a multifunctional platform for boosting orthotopic glioblastoma RNAi therapy, Nano Lett., 2020, 20, 1637–1646 CrossRef CAS PubMed.
- Y. Matsumura and H. Maeda, A new concept for macromolecular therapeutics in cancer chemotherapy: mechanism of tumoritropic accumulation of proteins and the antitumor agent smancs, Cancer Res., 1986, 46, 6387–6392 CAS.
- H. Maeda, Toward a full understanding of the EPR effect in primary and metastatic tumors as well as issues related to its heterogeneity, Adv. Drug Delivery Rev., 2015, 91, 3–6 CrossRef CAS PubMed.
- Y. Shi, R. Van der Meel, X. Chen and T. Lammers, The EPR effect and beyond: Strategies to improve tumor targeting and cancer nanomedicine treatment efficacy, Theranostics, 2020, 10, 7921 CrossRef PubMed.
- J. Park, Y. Choi, H. Chang, W. Um, Ju H. Ryu and I. C. Kwon, Alliance with EPR effect: combined strategies to improve the EPR effect in the tumor microenvironment, Theranostics, 2019, 9, 8073 CrossRef CAS PubMed.
- B. Lahooti, R. G. Akwii, F. T. Zahra, M. S. Sajib, M. Lamprou and A. Alobaida,
et al., Targeting endothelial permeability in the EPR effect, J. Controlled Release, 2023, 361, 212–235 CrossRef CAS PubMed.
- J. Zhang, S. Wang, D. Zhang, X. He, X. Wang and H. Han,
et al., Nanoparticle-based drug delivery systems to enhance cancer immunotherapy in solid tumors, Front. Immunol., 2023, 14, 1230893 CrossRef CAS PubMed.
- G. H. Petersen, S. K. Alzghari, W. Chee, S. S. Sankari and N. M. La-Beck, Meta-analysis of clinical and preclinical studies comparing the anticancer efficacy of liposomal versus conventional non-liposomal doxorubicin, J. Controlled Release, 2016, 232, 255–264 CrossRef CAS PubMed.
- V. Vijayan, A. Sundaram, A. Vasukutty, R. Bardhan, S. Uthaman and I. K. Park, Tumor-targeting cell membrane-coated nanorings for magnetic-hyperthermia-induced tumor ablation, Biomater. Sci., 2023, 11, 7188–7202 RSC.
-
C. D. Arvanitis, V. Askoxylakis, Y. Guo, M. Datta, J. Kloepper, G. B. Ferraro, et al., Mechanisms of Enhanced Drug Delivery in Brain Metastases with Focused Ultrasound-Induced Blood–Tumor Barrier Disruption, Proceedings of the National Academy of Sciences, 2018, vol. 115, pp. E8717–E8726 Search PubMed.
- G. Gong, J. Pan, Y. He, J. Shang, X. Wang and Y. Zhang,
et al., Self-assembly of nanomicelles with rationally designed multifunctional building blocks for synergistic chemo-photodynamic therapy, Theranostics, 2022, 12, 2028–2040 CrossRef CAS PubMed.
- D. Wang, J. Fu, Y. Shi, P. Dong, L. Yuan and B. He,
et al., The modulation of tumor vessel permeability by thalidomide and its impacts on different types of targeted drug delivery systems in a sarcoma mouse model, J. Controlled Release, 2016, 238, 186–196 CrossRef CAS PubMed.
- M. Yin, S. Tan, Y. Bao and Z. Zhang, Enhanced tumor therapy via drug co-delivery and in situ vascular-promoting strategy, J. Controlled Release, 2017, 258, 108–120 CrossRef CAS PubMed.
- J. Fang, W. Islam and H. Maeda, Exploiting the dynamics of the EPR effect and strategies to improve the therapeutic effects of nanomedicines by using EPR effect enhancers, Adv. Drug Delivery Rev., 2020, 157, 142–160 CrossRef CAS PubMed.
- Y. He, Z. Xu, Y. He, G. Cao, S. Ni and Y. Tang,
et al., MoS2 nanoflower-mediated enhanced intratumoral penetration and piezoelectric catalytic therapy, Biomaterials, 2022, 290, 121816 CrossRef CAS PubMed.
- Y. He, Z. Li, C. Cong, F. Ye, J. Yang and X. Zhang,
et al., Pyroelectric catalysis-based “nano-lymphatic” reduces tumor interstitial pressure for enhanced penetration and hydrodynamic therapy, ACS Nano, 2021, 15, 10488–10501 CrossRef CAS PubMed.
- S. Son, V. G. Deepagan, S. Shin, H. Ko, J. Min and W. Um,
et al., Ultrasmall gold nanosatellite-bearing transformable hybrid nanoparticles for deep tumor penetration, Acta Biomater., 2018, 79, 294–305 CrossRef CAS PubMed.
- S. Jafari-Matanagh, S. Esmail Razavi, M. B. Ehghaghi Bonab, H. Omidian and Y. Omidi, Multi-dimensional modeling of nanoparticles transportation from capillary bed into the tumor microenvironment, Comput. Biol. Med., 2023, 152, 106477 CrossRef CAS PubMed.
- W. Fang, Y. Su, Y. Quan, X. Li, Z. Qi and L. Zhang,
et al., Anticoagulants Enhance Molecular and Cellular Immunotherapy of Cancer by Improving Tumor Microcirculation Structure and Function and Redistributing Tumor Infiltrates, Clin. Cancer Res., 2023, OF1–OF15 Search PubMed.
- X. Dong, H.-J. Liu, H.-Y. Feng, S.-C. Yang, X.-L. Liu and L. Xing,
et al., Enhanced drug delivery by nanoscale integration of a nitric oxide donor to induce tumor collagen depletion, Nano Lett., 2019, 19, 997–1008 CrossRef CAS PubMed.
- Y. Fu, F. Ye, X. Zhang, Y. He, X. Li and Y. Tang,
et al., Decrease in Tumor Interstitial Pressure for Enhanced Drug Intratumoral Delivery and Synergistic Tumor Therapy, ACS Nano, 2022, 16, 18376–18389 CrossRef CAS PubMed.
- Y. Wang, R. Wang, L. Chen, L. Chen, Z. Yi and Y. Xin,
et al., Enhanced tumor penetration for efficient chemotherapy by a magnetothermally sensitive micelle combined with magnetic targeting and magnetic hyperthermia, Front. Pharmacol, 2022, 13, 4771 Search PubMed.
- M. E. Wechsler, J. E. V. Ramirez and N. A. Peppas, 110(th) Anniversary: Nanoparticle mediated drug delivery for the treatment of Alzheimer's disease: Crossing the blood–brain barrier, Ind. Eng. Chem. Res., 2019, 58, 15079–15087 CrossRef CAS PubMed.
- L. Chen, F. Zang, H. Wu, J. Li, J. Xie and M. Ma,
et al., Using PEGylated magnetic nanoparticles to describe the EPR effect in tumor for predicting therapeutic efficacy of micelle drugs, Nanoscale, 2018, 10, 1788–1797 RSC.
- Q. Xia, J. Huang, Q. Feng, X. Chen, X. Liu and X. Li,
et al., Size- and cell type-dependent cellular uptake, cytotoxicity and in vivo distribution of gold nanoparticles, Int. J. Nanomed., 2019, 14, 6957–6970 CrossRef CAS PubMed.
- D. M. Smith, J. K. Simon and J. R. Baker Jr, Applications of nanotechnology for immunology, Nat. Rev. Immunol., 2013, 13, 592–605 CrossRef CAS PubMed.
Footnote |
† These authors contributed to the work equally. |
|
This journal is © The Royal Society of Chemistry 2024 |
Click here to see how this site uses Cookies. View our privacy policy here.