DOI:
10.1039/D3NA00903C
(Review Article)
Nanoscale Adv., 2024,
6, 1611-1642
Magnetic iron oxide-based nanozymes: from synthesis to application
Received
19th October 2023
, Accepted 24th January 2024
First published on 19th February 2024
Abstract
Iron oxide nanozymes (IONzymes) are a class of magnetic nanoparticles that mimic the enzymatic activity of natural enzymes. These particles have received significant attention in recent years due to their unique properties, such as high stability, tunable magnetic responsiveness, and ability to act as biocatalysts for various chemical reactions. In this review, we aim to provide an overview of the production methods of magnetic nanozymes, including chemical, physical, and biological synthesis. The structure and design of magnetic nanozymes are also discussed in detail, as well as their applications in various fields such as biomedicine and environmental science. The results of various studies and the latest advances in the field of magnetic nanozymes are also discussed. This review provides valuable insights into the current state of magnetic nanozymes and highlights their potential for further development and application in various fields.
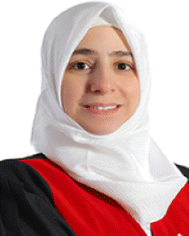 Asma Ghazzy | Dr Asma Ghazzy, a Jordanian national, obtained her PhD in Inorganic Chemistry from the University of Jordan in 2018. She has undertaken several research visits to the Technical University of Chemnitz. Currently, she is working as an Assistant Professor in the Faculty of Pharmacy at Al-Ahliyya Amman University. Dr Ghazzy previously earned her Master's Degree in Applied Chemistry. Her primary research focus is in the areas of photocatalysis, molecular sensors, and synthesis methodologies. Dr Ghazzy has made notable contributions, publishing her work in prestigious journals. She possesses expertise in instrumental analysis techniques and using chemical software. |
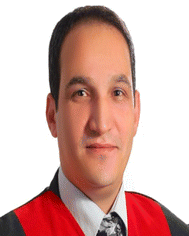 Hamdi Nsairat | Dr Hamdi Nsairat, a Jordanian national, earned his PhD in Biophysical and Pharmaceutical Chemistry from the University of Jordan in 2020. Currently, he is an Assistant Professor at Al Ahliyya Amman University's Faculty of Pharmacy, where he has over ten years of teaching experience in Biochemistry and related courses. He obtained his Master's Degree in 2005. His research focuses on liposomal drug delivery nano systems, aptamers, and targeting ligands. Hamdi has published innovative works in reputable journals and possesses excellent skills in instrumental analysis and chemical software applications. |
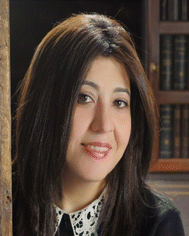 Rana Said | Dr Rana Said is an Associate Professor at Al-Ahliyya Amman University. She is specialist in sample preparation methods for the measurement of drugs and metabolites in biological samples to provide information that plays an important role in toxicokinetic and pharmacokinetic studies, and in therapeutic drug monitoring (TDM). She was always curious about drugs and how they work in the human body. She loved her chemistry and biological classes at school given that they were related to understanding more about drugs. She worked at a pharmaceutical company for a short time, and she travelled to the UK to attend a Master's Pharmaceutical Program, and at the end, she did her research project at one of the biggest pharmaceutical companies in the UK, AstraZeneca. Subsequently, she left for Sweden and joined a pharmacology department to get a PhD in developing new drugs analysis methods for pharmacokinetic studies. It was not easy to do this considering that this university is ranked number 4 in the world and a lot of work was required. |
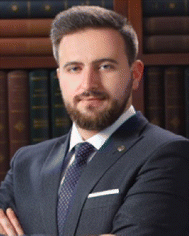 Obada A. Sibai | Obada Sibai, a Syrian national, earned his BSc in Pharmacy from Al-Ahliyya Amman University, 2022. He is a Research Assistant in the Faculty of Pharmacy/Al Ahliyya Amman University. He is currently a Master's student in Pharmaceutical Sciences in the Faculty of Pharmacy/Al-Zaytoonah University. His current work is in drug discovery, especially in computational drug design. He has published five articles in prestigious journals. He has excellent experience in many chemical synthesis and modelling software. |
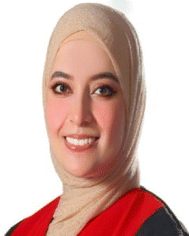 Aseel AbuRuman | Aseel Aburumman, a dedicated educator and pharmaceutical scientist, earned her Master's in Pharmaceutical Sciences in 2020 from Al-Ahliyya Amman University, Jordan. As a Lecturer at the same university, she imparts knowledge in different pharmaceutical aspects. Adept in research, her Master's project, “Development and In Vitro Evaluation of Soluplus® and/or Carbopol® 971 Buccoadhesive Patches Releasing Atorvastatin”, showcased her expertise in drug delivery. Aseel explored innovative approaches, including developing antipsychotic drugs with cancer treatment properties, demonstrating her commitment to advancing pharmaceutical science. |
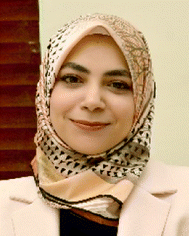 Alaa S. Shraim | Dr Ala'a S. Shraim holds the position of Assistant Professor at the Faculty of Allied Medical Sciences, Al-Ahliyya Amman University in Jordan. She has experience in the field of aptamer technology, namely, in the development and characterization of aptamers targeting kinases. She has developed her knowledge via extensive involvement in research, assessment, and teaching in academic institutions. |
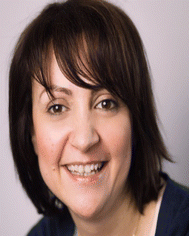 Afnan Al hunaiti | Dr Afnan Al-Hunaiti, an Associate Professor at the University of Jordan, is a seasoned academic with a comprehensive background in chemistry and catalysis. She earned her PhD from the University of Helsinki in 2015, focusing on the oxidation of fine chemicals through iron-based and metal-free catalysis. With over a decade of experience, Dr Afnan has held positions at the University of Petra and conducted research at the University of Helsinki. Dr Afnan's dedication extends to impactful publications in peer-reviewed journals, showcasing her commitment to advancing scientific knowledge in fields such as environmental inorganic chemistry and atmospheric catalysis. As an Associate Professor, Dr Afnan's multifaceted contributions underscore her significant role in advancing the understanding in the fields of catalysis and environmental science, making her a respected figure in the academic community. |
1. Introduction
The advancement of nanotechnology over the past two decades has highlighted new prospects in a wide range of industries due to the extraordinary qualities and distinct structure of nanomaterials.1,2 The structure of nanomaterials is divided into three layers (surface, shell, and core) where functional groups, including metal ions, tiny compounds, surfactants, and polymers, distinguish one layer from another.3,4 In general, the core is the nanoparticles (NPs), which can bond with various structures and macromolecules such as composites, metal organic frameworks (MOFs), polymers, and carbon nanotubes. This diversity enhances their unique properties in terms of size, shape, composition, and structural framework, which require optimization through synthesis procedures.5–8
Metallic nanoparticles represent a corner stone in the preparation of nanomaterials.9,10 Various metal oxides such as FeO, NiO, ZnO, CuO, AgO, TiO, SnO, and WO have unlimited applications in the medical sector (drug delivery, cancer treatment, and tissue repair), environment (qualitative and quantitative analysis of pollutants and toxins, water purification, and photodegradation), energy nanogenerators, electronics, catalysis, and mechanical and textile industries.11–17
Iron oxide is one of the best biocompatible inorganic nanoparticles, and it has remarkable microscopic physical properties including superparamagnetism, low susceptibility to oxidation, firmness in liquid solution, extended blood half-life, and flexible surface chemistry.18–22 Also, from an application point view, iron oxide NPs have high sustainability and superior properties in comparison to natural substances such as enzymes, which have drawbacks including high cost of isolation and purification, limited thermostability, and small pH window, which disrupts the enzyme activity upon handling, storage, and transportation.23,24
Artificial enzymes have replaced real enzymes in many applications for decades due to their stability and low cost. Metal complexes, cyclodextrins, polymers, dendrimers, and biomolecules have been studied to replicate enzyme activities and structures. Due to the rapid advancement of nano-studies and the exceptional properties of nanomaterials, several nanomaterials have shown enzyme-like functions. Moreover, nanozymes are popular because of their ease of manufacture, storage, isolation, and exceptional outcomes. In this respect, IONzymes can be used effectively to mimic natural enzymes and applied in several environmental applications, such as degradation of antibiotics and adsorption of dyes, in the food industry and biomedical, biosensing, cosmetics, and bioengineering.25–32
In this review, we highlight the methods for the synthesis of IONzymes and the current advances in the development of their applications. We discuss several nanomaterials that have been studied to imitate various types of enzymes in order to highlight the advancement in the area of nanomaterial-based artificial enzymes. We discuss their synthetic methods, processes, and applications in several domains, such as biosensing and immunoassays, as well as pollution elimination. We also outline techniques, such as several green, chemical, and physical methods, to produce iron oxide nanozymes.
2. Synthesis approaches of IONzymes
The methods commonly used to produce metal oxide nanoparticles are often applied in the creation of IONzymes, especially when they consist primarily of two magnetic nanoparticles, namely, magnetite (Fe3O4) and maghemite (γ-Fe2O3).33
The synthesis of IONzymes is accomplished using different chemical, physical, and biological techniques. Co-precipitation, evaporative decomposition of solution (EDS), aerosol, ultrasonic, sol–gel synthesis, micro-emulsion methods, reverse micelles, flow injection, solid-state reaction, spraying, and hydrothermal/solvothermal processes are typically used in chemical synthesis.34 The physical methods include milling, grinding, pyrolysis, and thermal ablation, as illustrated in Fig. 1. Also, the “Green Approach” has recently attracted significant consideration due to its eco-friendly nature and sustainability, which can be conducted using algae, bacteria, fungi, and plants.
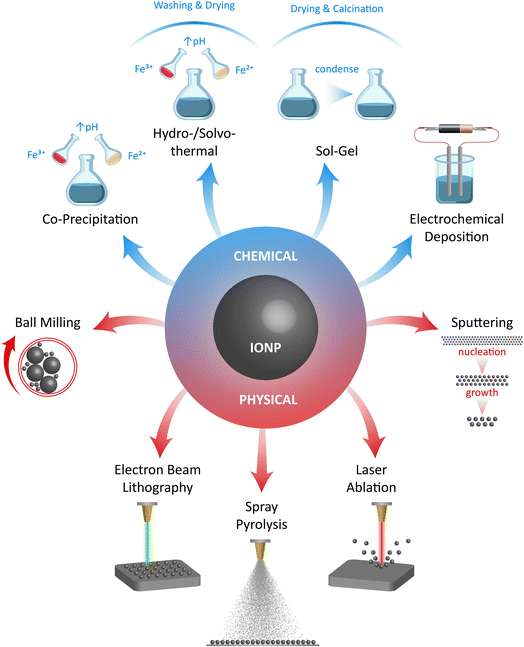 |
| Fig. 1 Chemical and physical techniques for the synthesis of IONzymes. | |
It is critical to distinguish between the general synthesis of iron oxide nanoparticles and specific processes that provide the characteristics of an enzyme in the production of IONzymes. Several conventional methods are effective in generating iron oxide nanoparticles, including co-precipitation, thermal breakdown, and hydrothermal synthesis. These steps must be performed to expose the properties of nanozymes. For instance, surface functionalization is essential to provide the ability to use enzymes.
Gao et al. (2007) demonstrated that the peroxidase-like activity of iron oxide nanoparticles can be significantly increased by adding specific functional groups to their surface. Both the size and structure of nanoparticles play a key role in determining their enzymatic activity.35 Another study demonstrated that an increase in the surface area to volume ratio of smaller iron oxide nanoparticles leads to higher catalytic efficacy. This characteristic resembles the active regions of natural enzymes.36 In addition, the crystalline structure of IONzymes influences their catalytic activity. Wei and co-workers showed that the intrinsic catalase-like, oxidase, and peroxidase activities of magnetite (Fe3O4) nanoparticles are attributed to their spinel structure.37
The synthesis method influences the stability and specificity of IONzymes prior to catalytic activity. Research in a related study indicated that the value of adding stabilizing chemicals during the synthesis of IONzymes can improve the lifespan of their catalytic activity and their thermal stability.38 This study emphasized that the functional characteristics of nanozymes are stable with time, stressing the importance of stabilization in the synthesis process.
Additionally, the effectiveness and selectivity of nanozymes can be altered by doping them with different metals towards particular substrates, hence expanding their applicative potential, as demonstrated by Zhang et al.39 These features demonstrate the significance of carefully choosing the methods for the synthesis of IONzymes to induce enzymatic activity and particle formation. This approach is consistent with the principles of biomimetic design given that it features both the functional and physical attributes of the nanoparticles. This illustrates the intricate nature of enzymes found in biological systems.
2.1. Chemical synthesis
The chemical production of nanoparticles is the most typical technique. However, the key challenges in this type of procedure include particle dispersion, clumping, and size uniformity. Additionally, chemical-based procedures involve the use of solvents such potassium bitartrate, sodium dodecyl sulfate, sodium borohydride, and hydrazine, all of which are detrimental to the environment given that they produce unpleasant waste flows. Herein, we focus on the four most popular methods, as listed in Table 1.
Table 1 The most common chemical techniques employed for the synthesis of IONzymes
Synthetic technique |
Advantage |
Disadvantage |
Ref. |
Co-precipitation |
Water-dispersed |
Multi-variable dependence |
40
|
Environmentally friendly |
Toxic liquid waste |
Efficient and economical |
Requires trained person for maintenance and regeneration |
Simple procedure |
Toxic liquid waste |
Rapid particle formation |
|
Hydrothermal/solvothermal |
Producing highly crystalline nanocrystals |
High temperature and pressure range |
41
|
Well-controlled dimensions |
Anti-corrosion autoclave material |
Combined with microwaves and magnetic fields improves reproducibility and quality |
Relatively costly reactors |
|
Mass production is not possible |
Electrochemical deposition |
Short formation time |
Stable solvent media |
42
|
Simple apparatus |
High electrophoretic mobility |
Uniformly coated on complicated geometries |
|
Control of film thickness and morphology |
|
Sol–gel synthesis |
Simplicity of the process |
Wear resistance reduced |
43
|
Uniform composition and high purity |
Weak bonding strength |
High production efficiency |
Hard to regulate porosity and permeability |
Production of intricately shaped optical components |
|
Controlling homogeneous products |
|
Capacity to use the product with unique structures such as fibers and aerogels |
|
2.1.1. Co-precipitation.
Massart developed a chemical co-precipitation approach for the large-scale synthesis of hydrophilic IONzymes.44 This reaction is performed in aqueous solution; therefore, the product is water-dispersed and may be directly employed for diverse applications without complicated ligand exchange procedures. The co-precipitation procedure to manufacture Fe3O4 involves the hydrolysis and condensation of ferrous and ferric ions in aqueous solution in the pH range of 8–14, as shown in eqn (1).18 | Fe2+ + 2Fe3+ + 8OH− → Fe3O4 + 4H2O | (1) |
Co-precipitation relies on the pH, reaction temperature, ion concentration, ionic strength, salt type, and alkali used. However, the application of co-precipitation to create magnetite NPs is a challenging process, and the reaction conditions must be tightly regulated.45,46
Another factor is the molar ratio of ferrous/ferric, which affects the physical and magnetic properties of NPs. When 1 ferrous
:
1 ferric is used, there is larger magnetization saturation than other ratios.47,48 Similar research has been conducted to create various synthesis techniques employing the chemical co-precipitation process to produce stable, homogeneous, smaller-sized, crystalline particles.49
A method involving co-precipitation in flow chemistry, combined with an in situ synchrotron X-ray diffraction (XRD) technique, was devised to “freeze” the transient reaction states through steady-state operation. This technique showed appealing findings, as follows:
(i) Five seconds after mixing, the only crystalline phase was the inverse spinel framework of magnetite/maghemite.
(ii) The particle size increased slightly, and solid phase development (owing to particle growth) was completed within 2 min.
(iii) The mixing conditions did not affect the XRD pattern.
(iv) During co-precipitation, the diffraction peaks widened, indicating the presence of smaller coherently scattered regions (Fig. 2).50,51
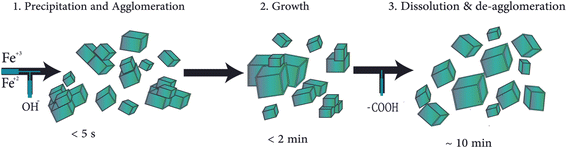 |
| Fig. 2 Suggested particle mechanism during the co-precipitation procedure: (1) particles are precipitated and agglomerated within 5 s, (2) agglomerated pieces grow over the next 2 or 3 min and (3) addition of neutralization solution causes particles to de-agglomerate within 10 min. | |
The co-precipitation chemical technique can be used to produce functional materials, as shown in Table 2. Chen et al. discovered a new co-precipitation approach to generate ferumoxytol, a therapeutically relevant magnetic nanoparticle with γ-Fe2O3 as the core. The magnetization of ferumoxytol is the greatest recorded to date, reaching 104–105 emu g−1, and its crystal structure has been substantially improved.52 Superparamagnetic IONzymes were produced with a limited size distribution, and their magnetic susceptibility, coercivity, remanence, and saturation magnetization at 5–300 K were analyzed.53
Table 2 Representative iron oxides obtained through co-precipitation procedures
Compound/property |
Particle size (nm) |
Morphology |
Magnetization (emu g−1) |
Ref. |
Ferumoxytol |
7.1 |
Spherical |
104–105 |
52
|
Temperature-dependent particles |
11.22 |
Spherical |
64–72 |
53
|
(Zn–Mn)-co-doped |
10–13 |
Spherical |
81 |
54
|
2.1.3. Electrochemical deposition.
The electrophoretic deposition (EPD) technique involves the use of charged particles that move and are deposited on the surface of a conductive electrode to create thin or thick coatings and films. A broad range of fine powder, composite particles, colloidal metals, and ceramics can be produced via EPD.64 EPD is one of several solution methods employing colloidal NPs, which has recently emerged as a successful method for producing dense and durable NP films. The relationship in EPD systems between colloidal NPs and the organic solvent has been studied using hexane, toluene, and chloroform in various solvent ratios to examine the charge formation function of the solvent in EPD systems (10
:
0, 7
:
3, 5
:
5, 3
:
7, and 0
:
10). The NP layer gets thicker and rougher as the toluene to hexane ratio increases. Alternatively, the film thickness is dramatically reduced when the chloroform to hexane ratio increases.65
The electrophoretic deposition approach was used to produce a bioactive coating, such as hydroxyapatite-iron oxide-chitosan (HA-CS) with varying amounts of Fe3O4 (1, 3, and 5 wt%) and porous morphology.13 Another methodology demonstrated potential for generating thick magnetic nanocomposites for on-chip power components by incorporating iron oxide nanoparticles into a mold, and subsequently performing electro-infiltration of nickel through the porous film. The resulting magnetic saturation of the nanocomposites was measured at 473 kA m−1, which is intermediate between the magnetic saturation values of iron oxide nanoparticles and nickel.66 Also, the formulated and cost-effective coating method can enhance the surface characteristics and hemocompatibility for biomedical applications, resulting in decreased contact angle values and hydrophilic nature. In one study, the Ti–13Nb–13Zr alloy was electrophoretically coated with Bioglass (BG), hydroxyapatite (HA), and iron oxide particles (FeO), which improved the stability of the suspension.67
2.1.4. Sol–gel synthesis.
Using the sol–gel method, a gel-like network is generated, incorporating both liquid and solid phases. Also, by selecting the appropriate complexing agent, concentration, type of chemical additives, and temperature settings, it is possible to control the crystallinity, shape, and magnetic characteristics of IONzymes.68,69 Apparently, the annealing temperature plays a central role in this method, and the outcome shows that the crystalline Fe2O3 nanoparticles70 and the dielectric properties are enhanced.71 Additionally, this technique can be used to create products with efficient physical characteristics, such as low UV absorption and thermal expansion coefficient and high optical transparency.72
The crystalline structure, composition, purity, magnetism, and morphology of iron oxide nanomaterials can be enhanced by optimizing some variables or combining techniques (Table 4). One technique is optimizing the precursor-to-solvent (P/S) ratio for three iron oxide phases (α-Fe2O3, Fe3O4, and γ-Fe2O3) to tune the structural and magnetic properties via sol–gel synthesis.73 Another method combines microwave radiation and aluminum doping in iron oxide thin films, which controls the structural transitions of the iron oxide thin film. A study demonstrated a γ-Fe2O3 to Fe3O4 transition at 6–10 wt% Al with increasing saturation magnetization of the films from 251.3 emu cm−3 to 405.6 emu cm−3.74
Table 4 Representative iron oxide nanozymes obtained through sol–gel procedures
Variable/technique |
Starting materials |
Solvent |
Particle size (nm) |
Morphology |
Additional property |
Ref. |
Agitation time |
Fe (NO3)3·9H2O |
Absolute ethanol |
10 nm |
Spherically |
Purity > 75% |
75
|
Ba (NO3)2 |
Carbonization method |
FeCl3·6H2O/rosin |
Deoxygenated H2O/ethanol |
<50 nm |
Varies with different rosins: FeCl3 |
Enhances interfacial reactivity |
76
|
Non-hydrolytic |
Anhydrous FeCl3 |
Anhydrous ethanol |
202–373 Å |
Rod-shaped |
Homogeneous dispersion |
77
|
2.2. Physical genesis
Physical techniques such as mechanical milling, grinding, and thermal ablation are all expensive given that they consume a significant amount of energy. Furthermore, another significant drawback of this strategy is its exceedingly low output yield.
2.2.1. Ball milling.
Ball milling is a shear-force-dominated method, which is also known as mechanical alloying, ultrafine grinding, and nanosizing in the literature. It is one of the most widely used industrial processes, in which the particle size is continuously reduced by impact and attrition. Metal balls, often made of zirconia (ZrO2) or steel, serve as the grinding medium, while a spinning shell generates centrifugal force. By regulating the milling variables, such as ball-to-powder ratio (B/P), milling time, milling rpm, starting weight, and ball diameter, the excessive compression force that may harm the crystalline characteristics of nanomaterials can be reduced. Table 5 demonstrates examples of two states of milling that can be initiated, i.e., dry ball milling (DBM) and wet ball milling (WBM). The solid-state mechanical size reduction process known as ball milling transforms iron precursors into MNPs (magnetic nanoparticles). To speed up milling and prevent the agglomeration of the created nanoparticles, solvents or excess salt can be added.78
Table 5 Iron oxide-based nanomaterials prepared via ball milling process
Milling process |
Equipment/ball properties |
Milling agent/solvents and conditions |
Characteristics |
Ref. |
WBM |
Planetary ball mill |
DI water, 4 h, 500 rpm |
High adsorption capacities of Cr(IV) qe = 48.1 mg g−1 |
34
|
DBM |
Iron balls with 1.5 cm diameter |
30 h and 90 h |
31.48 emu g−1 and 37.80 emu g−1 |
81
|
DBM |
Steel balls with 8 mm diameter |
25 rpm, 60 min |
Particle size = 45 nm |
82
|
DBM |
Planetary ball mill |
30 min, 320 rpm |
M
s = 20.45 emu g−1 |
83
|
Also, different mechanical ball milling techniques can be applied, such as conventional ball milling. Specifically, larger particles collide with steel balls or the interior wall of the tank to produce ultrafine particles, while high-energy ball milling uses a specialized grinding machine to synthesize a nano-spinel-type ferrite by mechanically alloying the initial materials.79 However, the significant drawbacks of ball milling are pollution of the steel ball, the potential chemical and mechanical amorphization of the crystals, the high power used, and the prolonged milling period.79,80
2.2.2. Electron beam lithography.
The use of electron beam lithography or electron beam deposition to apply either an exposure-sensitive resist material or high-purity iron material to a substrate can be employed of the synthesis of MNPs. This process produces MNPs by evaporating the first iron precursors onto the resist pattern, and then removing the resist through a lift-off procedure. Alternatively, the nanopatterns can be etched onto a functional substrate to produce MNPs.78,84
2.2.3. Laser ablation.
Laser ablation is a method that involves irradiating a solid material placed under a thin layer with a laser beam.80,85 When the solid material is placed at the bottom of a cell containing a liquid,86 the technique is referred to as “laser ablation synthesis in solution (LASiS)”. In this case, various lasers can be employed, including Nd:YAG, Ti:sapphire, and copper vapor lasers,80,87 allowing precise control of the phase composition, size, and shape of the particles, thus producing nanoparticles with an average diameter of approximately 15 nm.87 Although laser ablation can quickly generate MNPs when exposed to a laser for short periods, this method has a low production rate.88 Also, prolonged laser ablation leads to the formation of an excessive number of nanoparticles, which remain suspended in the colloidal solution and obstruct the laser beam. This causes the laser energy to be absorbed by the previously formed nanoparticles, rather than the target surface, resulting in a decreased ablation rate.80
2.2.4. Sputtering.
Sputtering is a process that entails bombarding the surface of a bulk material with high-energy particles, such as noble gas ion beams, to remove atoms from the surface. This method produces nanoparticles with the same composition as the target material and is more cost-effective than electron beam lithography.80 However, the choice of sputtering gas, such as helium, neon, argon, krypton, and xenon, can impact the surface morphology, texture, and optical properties of the resulting nanoparticles.89,90
2.2.5. Aerosol spray pyrolysis.
Aerosol spray pyrolysis is a scalable and cost-effective physical synthesis process.91 In this method, nanoparticle precursors are transmitted into a heated reactor in the form of small droplets suspended in a vapor, obtaining MNPs with a spherical morphology, narrow particle size distribution, and no agglomeration.80 However, there are still challenges to be addressed, such as difficulty in controlling the homogeneous pore sizes and inner structure of the particles.91,92
Spray pyrolysis is a chemical vapor deposition (CVD) process used to prepare nanomaterials, which have a consistent particle diameter compared to the traditional nanomaterials.93,94 A precursor solution of metallic salts is used to create an aerosol in the spray pyrolysis process. The produced solution droplets (aerosol) undergo several stages, as follows: (1) solvent evaporation from the droplet surfaces, (2) drying, (3) annealing, (4) production of microporous particles with a defined phase structure, (5) creation of solid particles, and (6) sintering of solid parts. Fig. 4 shows these steps starting from precursors to nanozyme formation.95
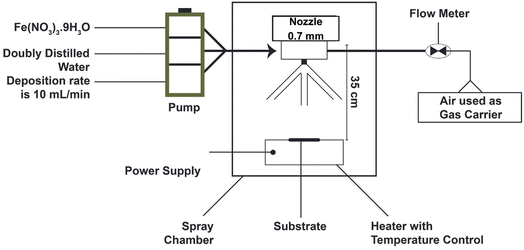 |
| Fig. 4 Setup of the spray pyrolysis technique used for synthesis IONzymes thin film at various temperatures. | |
Several studies were conducted to investigate the influence of different substrate temperatures,96,97 sampling techniques,98 presence of chloride ion,99 and other dispersion parameters100 on the pyrolysis process. Interestingly, highly porous ternary NiCoFe oxide nanomesh with a two-dimensional shape and quasi-single-crystalline (QSC) property was created using a practical molten-salt-protected pyrolysis method.
The NiCoFe oxide nanomesh possessed high stability, low over-potential, high current density, and excellent oxygen evolution reaction performance with increased intrinsic activity. A quick pyrolysis technique shielded by molten salt (MS, 53% KNO3, 7% NaNO3, and 40% NaNO2) was carried out at 300 °C to produce mild dehydration to form mixed metal oxides with retained morphology and minimum particle sintering.101 Another study employed the phase-selective laser-induced breakdown spectroscopy technique to investigate the production of FeO particles along the axial centerline of the spray in an external mixing spray flame pyrolysis reactor, under different precursor solutions. The addition of 2-ethylhexanoic acid to the precursors was examined and significant changes in the evolution of the atomic emission spectra were observed. These changes enabled the differentiation between the gas-to-particle and droplet-to-particle routes in situ.102
2.3. Biosynthesis
Green synthesis, which involves the use of plants, microbes, and other biological materials, has gained significant attention as a safe, sustainable, and biologically acceptable method for the synthesis of metal oxide nanoparticles, such as iron oxide nanoparticles (IONPs) (Fig. 5). IONPs have attracted particular interest due to their magnetic properties, which allow them to be easily separated from the reaction mixture using an external magnetic field. Biomaterials such as plants, fungi, bacteria, and algae can be used in green synthesis to produce IONPs with a size in the range of 1 to 100 nm and a variety of shapes, including cubic, tetragonal crystalline, spherical, cylindrical, elliptical, octahedral, orthorhombic, hexagonal rods, nanospheres, and quasi spherical. In addition to synthesizing IONPs, these biomaterials can also act as reducing agents, capping agents, stabilizing agents, and fabricating agents in the green synthesis of nanoparticles.103
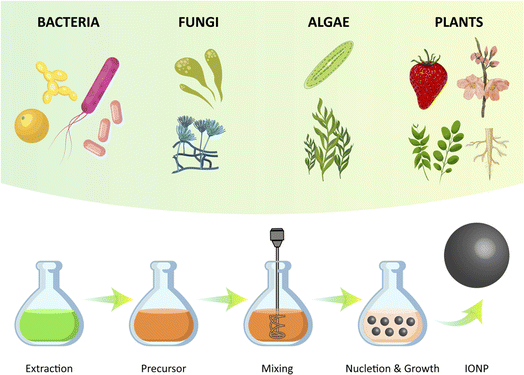 |
| Fig. 5 Biosynthesis of IONzymes using different green sources. | |
It is important to highlight the diverse biological pathways employed by various organisms. For instance, Plumeria obtusa leaves were employed for the biofabrication of well-defined, crystalline INPs via an eco-friendly, cost-effective, and surfactant-free technique. These nanoscale particles displayed potent antimicrobial and antioxidant activity, while remaining non-toxic to red blood cells. This green synthesis presented a potential strategy for the synthesis of sustainable nanomedicines against microbial infections.104 A study revealed the eco-friendly biosynthesis of IONPs from Penicillium spp. fungal filtrate. The extracellular strategy starts by the reduction of FeCl3, with the protein from Penicillium spp. playing a pivotal role in capping and stabilizing the IONPs. The characterization of the IONPs showed that they were spherical with high stability. The IONPs exhibited powerful antibacterial and antioxidant activities, making them potential alternatives to antimicrobial and anticancer agents in biomedical applications.105
A notable example is the use of bacterial extracellular polymeric substances (EPS) as reducing and stabilizing compounds during the bio-mediated production of metal nanoparticles for multifunctional applications, such as a new bacterium, E. faecalis_RMSN6. The EPS was extracted from E. faecalis and used for producing highly stable IONPs. This study aimed to assess the effectiveness of the synthesized Fe3O4 NPs as adsorbents for removing Cr(VI) metal ions from aqueous solutions. Furthermore, an in vitro toxicity analysis using bacterial EPS was conducted to evaluate the potential adverse effects of the synthesized Fe3O4 NPs.106
Shalaby et al. presented a green synthesis method for the preparation of recyclable IONPs utilizing Spirulina platensis microalgae. This study highlighted the efficient adsorptive removal of cationic and anionic dyes for water treatment applications. The environmentally friendly synthesis method not only contributes to the sustainable formation of nanomaterials but also exhibits the recyclability of the synthesized IONPs.107
2.3.1 Biosynthesis of IONPs using plants.
Plants are widely available, easy to handle, and relatively inexpensive materials that can be used for the synthesis of various types of nanoparticles.108 Different parts of plants, such as their roots, leaves, seeds, flowers, fruits, peels, petals, and whole plants, can be utilized in the biosynthesis process because they contain various biomolecules, such as amino acids, carbohydrates, terpenoids, flavonoids, saponins, proteins, and nitrogenous compounds, which can act as reducing agents, stabilizers, redox mediators, and capping agents in the synthesis of nanoparticles109–116 (Table 6).
Table 6 Biosynthesis of iron oxide nanoparticles using plants
Name of the plant |
Biomaterial used |
Iron precursor used |
Size |
Shape |
Application |
Ref. |
Hibiscus rosa-sinensis
|
Dried petals |
Ferric chloride (25 mM) and ferrous chloride (25 mM) (2 : 1) |
65 nm |
Spinel |
Biscuit fortification |
117
|
Carica papaya
|
Dried leaves |
FeCl3·6H2O (0.1 M), NaOH (1 M) |
2.159 nm |
Not uniform (agglomerated particles) |
Antibacterial |
118
|
Psidium guajava
|
Leaves |
FeCl3·6H2O |
1–5 nm |
Spherical |
Antibacterial |
119
|
Citrus |
Fresh leaves |
Iron chloride (0.1 mM) |
15–80 nm |
Spherical |
Antibacterial |
120
|
Malus pumila (apple) |
Peels |
FeCl2·4H2O (20 mM), FeCl3.6 H2O (40 mM), NaOH (1 M) |
50–100 nm |
Elliptical and spherical |
Decolorization of dye |
121
|
Citrus paradisi
|
Peels |
FeCl3·6H2O (6 mM) |
28–32 nm |
Spherical |
Antioxidant |
122
|
Syzygium cumini
|
Leaves |
FeCl3 (0.010 mol L−1) |
40–52 nm |
Spherical |
Antibacterial, antifungal, aflatoxin B1 adsorption |
123
|
Juglans regia
|
Dried green husk |
FeCl3·6H2O (97%), FeCl2·4H2O (99%), NaOH (2 M) |
12.6 nm |
Cubic |
Cytotoxic assay |
124
|
Pyrus sinkiangensis Yu
|
Peels |
FeSO4·7H2O (0.1 M) |
10–90 nm |
Irregularly shaped |
Cr(IV) removal |
125
|
Cymbopogon citratus
|
Leaves |
FeCl3·6H2O (0.26 M), FeCl3·6H2O (0.52 M), Na2CO3 (0.75 M) |
9 ± 4 nm |
Irregular cubic |
Nanotoxicological |
126
|
Laurus nobilis
|
Leaves |
FeCl3·6H2O (0.1 M) |
8.03 ± 8.99 nm |
Spherical |
Antimicrobial |
127
|
Hyphaene thebaica
|
Fruits |
FeH12N2O12 (5 g) |
5–10 nm |
Quasi-spherical and cuboidal |
Antimicrobial, antioxidant, and antiviral |
128
|
Solanum lycopersicum
|
Leaves |
FeSO4·5H2O (0.1 M) |
200–800 nm |
Flower |
Antibacterial and anticancer |
129
|
Lawsonia inermis
|
Leaves |
FeSO4 (0.01 M) |
2 μm |
Hexagonal |
Antimicrobial |
130
|
Ficus carica
|
Fruit |
(F2Cl3·6H2O) (100 mL) |
11–29 nm |
Spherical |
Antimicrobial |
131
|
Rhamnus Triquetra
|
Leaves |
Ferric acetate (3 g) |
∼21 nm |
Spherical |
Antimicrobial, antioxidant, anticancer, antileishmanial, brine shrimp cytotoxicity |
132
|
Trigonella foenumgraecum
|
Leaves |
FeCl3 (1 M) |
27.91–40.94 nm |
Grain |
Antibacterial |
133
|
Tomato |
Fruits |
FeCl3 (1 M) |
48.18–77.54 nm |
Semispherical |
Antibacterial |
133
|
Grapes |
Fruits |
FeCl3 (16.2 g) |
49–50 nm |
Cubic |
Antibacterial |
134
|
Moringa oleifera
|
Leaves |
FeCl3 (0.5 M) |
15.01 ± 6.03 nm |
Rod-like |
Antibacterial |
135
|
Withania coagulans
|
Berries |
FeCl3·6H2O, FeCl2·4H2O (1 : 2 M) |
16 ± 2–18 ± 2 nm |
Rods |
Photocatalytic degradation and antimicrobial |
136
|
Citrullus colocynth
|
Pulp |
FeCl3 (0.5 M) |
12–45 nm |
Spherical |
Antimicrobial |
137
|
Seed |
6–15 nm |
Durian rind
|
Peels |
Ferrous sulfate (0.05 M) |
10 nm |
Spherical |
Antibacterial |
138
|
Borassus flabellifer
|
Seed coat |
Ferric chloride (0.2 M), ferrous sulphate (0.1 M) (2 : 1) |
30–200 nm |
Hexagonal |
Antimicrobial, antioxidant |
139
|
Citrus sinensis
|
Peels |
Ferric chloride (1 mM) |
97.5 nm |
— |
Antibacterial |
140
|
Thymbra spicata
|
Leaves |
FeSO4·7H2O (0.1 M) |
120.3–17 nm |
Spherical |
Antibacterial, antibiofilm, and antioxidant |
141
|
93.9 nm |
Cocos nucifera L. |
Pulps |
0.502 g of FeCl3 |
90–95 nm |
Husked rice shape |
Antibacterial and anticancer |
142
|
Euphorbia herita
|
Leaves |
Ferrous sulfate (0.1 M), ferric chloride (0.1 M) |
25–80 nm |
Cavity like |
Antimicrobial |
143
|
Camellia sinensis L |
Grinded waste of pruned teas |
FeSO4·5H2O (0.1 M), NaOH (0.5 M) |
28.5 nm |
Regular spherical |
Antioxidant |
144
|
Gundelia tournefortii L |
Leaves |
FeCl3·6H2O (2 M), FeSO4·7H2O (1 M), NaOH (1 M) |
29.9 nm |
Spherical |
Remove crystal violet, malachite green, and safranin dyes from prepared aqueous solutions |
145
|
Aegle marmelos
|
Leaves |
Ferric nitrate (90 mL) |
— |
Agglomerated |
Antimicrobial and antifungal |
146
|
Alstonia scholaris
|
Leaves |
FeCl2·4H2O (0.5 M) MgCl2·6H2O (0.5 M) |
8.14–13.4 nm |
Cubic |
Antimicrobial, antioxidant and larvicidal |
147
|
Polyalthia longifolia
|
Leaves |
FeCl2·4H2O (0.5 M) MgCl2·6H2O (0.5 M) |
8.14–13.4 nm |
Cubic |
Antimicrobial, antioxidant and larvicidal |
147
|
Coffee |
Seed |
Fe2+ and Fe3+ (1 : 1) |
23.2–37.5 nm |
Cubic |
Antibacterial |
148
|
Brassica oleracea var. Capitata sub.var. rubra |
Peels |
Iron(III) chloride (10 mM) |
675 ± 25 nm |
Agglomerated |
Anticancer |
149
|
Zingiber officinale
|
Root |
Ferric chloride (0.1 M) |
5.10 nm |
Nanocube |
Antimicrobial |
150
|
Artemisia
|
Leaves |
FeCl3 (0.01, 0.04, 0.07, and 0.1 M) |
24.67–34.28 nm |
Cubical |
Antioxidant |
151
|
Garcinia mangostana
|
Peels |
FeCl3·6H2O and FeCl2·4H2O at a molar ratio of 2 : 1 |
13.42 ± 1.58 nm |
Spherical |
Anticancer |
152
|
Chlorophytum comosum
|
Leaves |
FeCl3·6H2O (0.1 M) |
<100 nm |
Spherical |
Methyl orange dye degradation and antimicrobial |
153
|
Mikania mikrantha
|
Leaves |
FeSO4·7H2O (5 mmol) and FeCl3 (10 mmol) |
20.27 nm |
Rhomboidal |
Antimicrobial |
154
|
Garlic |
Peels |
FeCl3 (1 M) |
24–44 nm |
Irregular |
Degrade methylene blue dye |
155
|
Onion |
Peels |
FeCl3 (1 M) |
29–32 nm |
Nanofiber |
Degrade methylene blue dye |
155
|
Ficus carica |
Leaves |
FeCl3·6H2O (0.01 M), NaOH (0.1 M) |
43–57 nm |
Agglomerated and are multiform |
Antioxidant |
156
|
Celosia argentea
|
Leaves |
Ferric nitrate (0.1 M) |
5–10 nm |
Spherical |
Antibiofilm, antioxidant, anti-inflammatory, antidiabetic, and larvicidal activities |
157
|
Plumeria obtusaobtuse
|
Leaves |
Fe(C2H3O2)2 (3 mM) |
50 nm |
Spheroidal |
Antimicrobial, antioxidant |
104
|
Camellia sinensis
|
Leaves |
FeCl3 (10 mM) |
13 nm |
Cubical |
Antioxidant, antimicrobial |
158
|
Persimmon
|
Fruits |
FeCl3 (0.04 M), NaOH (1 M) |
30–60 nm |
Spherical |
Antibacterial and anticancer |
159
|
Punica granatum
|
Peels |
FeCl3 (0.1 M) |
17.8 ± 6.5 nm |
Cubical |
Enzyme mimicking peroxidase, catalase, and superoxide dismutase |
160
|
Buddleja lindleyana
|
Leaves |
Fe (SO4)3·6H2O (1 g), AgNO3 (0.1 g) |
25 and 174 nm |
Triangular and spheroidal |
Antimicrobial |
161
|
Hibiscus rosa sinensis
|
Flowers |
FeCl2·4H2O (1 mM) |
51 nm |
Tetragonal |
Antibacterial |
162
|
Allium cepa
|
Peel |
Ferric chloride (250 mL) |
42.78 1 nm |
— |
Memory-enhancing agent |
163
|
Centaurea alba
|
Leaves |
FeCl3·H2O (0.001 M) |
10–52 nm |
Spherical |
Anti-atherosclerotic and antioxidant |
164
|
Peltophorum pterocarpum
|
Leaves |
FeSO4·7H2O (0.1 M) |
0.085 to 0.2 μm |
Irregular |
Photocatalytic and catalytic removal of organic pollutants |
165
|
Psidium guajava Linn
|
Leaves |
FeCl3 (1 M), NaOH (1 N) |
80.3–99.1 nm |
Spherical |
Antimicrobial, antioxidant |
166
|
Hylocereus undantus
|
Fruits |
Ferric sulphate and ferrous sulphate (2 : 1) |
10–15 nm |
Spherical |
— |
167
|
Nigella sativa
|
Seeds |
FeCl3 (1 M) and FeCl2 (2 M) |
31.45 nm |
Spherical |
Antimicrobial |
168
|
Mentha spicata
|
Leaves |
FeCl3 (0.4 M) |
21–82 nm |
Circular or rod |
Antimicrobial |
169
|
Cassia auriculata
|
Flowers |
FeCl3·6H2O (0.1 M) |
15–35 nm |
Spherical |
Photocatalytic degradation and larvicidal effect |
170
|
Melia azedarach
|
Flowers |
Ferrous sulphate (20 mM), NaOH (0.1 M) |
231.43 ± 5.21 nm |
Spherical |
Antimicrobial, antioxidant |
171
|
Echinochloa frumentacea
|
Grains |
Fe(NO3) (0.1 M) |
20–40 nm |
Rectangular and triangular |
Pharmaceutical, agricultural, targeted drug delivery and biomedical applications |
172
|
Pimenta dioica
|
Leaves |
FeSO4 (0.1 M) |
5–15 nm |
Spherical |
Anticancer |
173
|
Banana
|
Peels |
FeCl3·6H2O (2.16 g) |
44–58 nm |
Cubic and agglomerated |
Nondestructive technique (NDT) applications |
174
|
CH3COONa (6.56 g) |
Amla
|
Seeds |
FeCl3 (0.01 M) |
4–5 nm |
Spherical |
Removal of toxic dyes |
175
|
Centaurea solstitialis
|
Leaves |
FeCl3 (0.1 M) |
— |
Spherical |
Antimicrobial activity and dye decolorization |
176
|
Eucalyptus globulus
|
Leaves |
Fe(NO3)3·9H2O (0.1 M) |
2.34 ± 0.53 nm |
Spherical |
Removal of heavy metals from agricultural soil |
177
|
Galega officinalis
|
Leaves |
FeCl3·6H2O (40 mM), FeCl2·4H2O (20 mM) (2 : 1 M ratio) |
41.9 ± 1.00 nm |
Spherical |
Toxicity assessment in plants and aquatic model organisms |
178
|
Coriandrum sativum L. |
Leaves |
FeSO4 (0.01 mM) |
163.5 nm |
Spherical |
— |
179
|
2.3.2 Biosynthesis of IONPs using fungi.
The synthesis of iron oxide nanoparticles using fungal species has several advantages, including ease of scaling up the process, use of economical raw materials for growth, high biomass-forming capacity of fungi, simplicity of the downstream processing steps, low toxicity of the residue, and economic feasibility of the process.180–182 Fungal species also have superior tolerance and bioaccumulation properties, which can aid in the synthesis of metal nanoparticles.93 The relationship between microorganisms and metals has been thoroughly researched and applied in various biological processes, including bioleaching, heavy metal removal, and bioremediation.183 In these processes, microorganisms can accumulate and extract metals through the release of enzymes or other mechanisms. These interactions have practical applications in fields such as biotechnology, environmental science, and metallurgy (Table 7).116,184
Table 7 Biosynthesis of iron oxide nanoparticles using fungi
Fungal strain |
Biomaterial used |
Iron precursor used |
Size (nm) |
Shape |
Applications |
Ref. |
Aspergillus niger BSC-1 |
Cell-free filtrate |
(FeCl3·6H2O) and ferrous sulfate (FeSO4·7H2O) in 2 mM:1 mM |
20–40 nm |
Orthorhombic |
Cr(VI) removal |
182
|
Penicillium spp. |
Cell-free filtrate |
FeCl3 (3 mM) |
3.31–10.69 nm |
Spherical |
Antimicrobial, antioxidant |
105
|
Chaetomium cupreum
|
Fungal biomass |
FeSO4 (2 g) and NaOH (1.20 g) |
25 nm |
Spherical |
Anticancer |
185
|
Chitosan
|
— |
(FeCl3·6H2O), (FeSO4·4H2O) |
200–600 nm |
Spherical |
Postharvest disease inhibition in fruit |
186
|
Penicillium roqueforti
|
Fungal biomass |
Ferric chloride hexahydrate (10−3 M) and ferrous chloride tetrahydrate (10−3 M) |
5–16 nm |
Spherical |
Antimicrobial |
187
|
Lichen Ramalina sinensis
|
— |
Fe2+/Fe3+ (100 mL) |
31.74–53.91 nm |
Spherical |
Antimicrobial |
188
|
Pleurotus florida
|
— |
Ferric chloride (1 M) |
100 nm |
Spherical |
Antimicrobial |
189
|
Penicillium commune
|
— |
FeCl3 (1 mM), FeSO4 (1 mM) |
30–50 nm |
Spherical |
Cleaning gel |
190
|
Bacillus megaterium
|
— |
FeCl3 (1 mM), FeSO4 (1 mM) |
40–60 nm |
Cubic |
Cleaning gel |
190
|
Fusarium oxysporum
|
— |
FeCl3 (1 mM), FeSO4 (1 mM) |
20–50 nm |
Quasi-spherical |
Cleaning gel |
190
|
2.3.3 Biosynthesis of IONPs using bacteria.
Prokaryotes, which are simple organisms without a defined nucleus or membrane-bound organelles, have been extensively studied as a model system in the field of nanotechnology due to their widespread presence, fast doubling time, ability to grow under challenging conditions, and the fact that they can be cultivated using inexpensive and straightforward media.191,192 The application of this system is considered an effective method for synthesizing nanoparticles with a range of shapes, sizes, structural frameworks, and physical and chemical properties through the reduction of metal ions using reductase enzymes, which allow microorganisms to accumulate and detoxify metals.193 This process involves the use of metal salts as precursors in the reaction and has been used to synthesize metallic nanoparticles.116,194,195Table 8 presents some types of bacteria used to produce IONzymes.
Table 8 Biosynthesis of iron oxide nanoparticles using bacteria
Bacteria |
Salt |
Size |
Shape |
Applications |
Ref. |
Bacillus subtilis
|
FeCl3 (2 mM) |
12–32 nm |
Spherical |
Cytotoxicity assay |
196
|
Bacillus subtilis
|
FeCl3 (2 mM) |
3.6 nm |
Spherical |
— |
196
|
Proteus vulgaris
|
FeCl3 (3 mM) |
20–30 nm |
Spherical |
Antibacterial, antioxidant |
197
|
Enterococcus faecalis
|
FeCl2·4H2O (0.1 M), FeCl3·6H2O (0.2 M) |
15.4 nm |
Cubical, hexagonal, brick, and irregular |
Heavy metal removal and cytotoxic |
106
|
Enterococcus faecalis
|
FeCl-.6H–O (1 M) |
48.77–55.55 nm |
Cubic |
Antibiofilm |
198
|
Bacillus coagulans
|
FeCl3·6H2O, FeCl2·4H2O (2 : 1 M) |
15.18 nm |
Cubic |
Antibacterial |
199
|
Aeromonas hydrophila
|
FeCl2 (5 mmol), FeCl3 hexahydrate (10 mmol) |
8–12 nm |
Spherical |
Antibacterial |
200
|
2.3.4 Biosynthesis of IONPs using algae.
Algae, which include both microalgae (single-celled organisms) and macroalgae or seaweeds (multi-celled organisms), are used in the field of nanotechnology for the synthesis of various types of metallic nanoparticles, such as gold, silver, palladium, iron, and copper (Table 9).201,202 Similar to plants and bacteria, algae also produce a range of biomolecules, including proteins, fats, carbohydrates, peptides, alkaloids, terpenes, macrolides, cell wall polysaccharides, glycoproteins (containing functional groups such as carbonyl, hydroxyl, carboxyl, and sulfonate), and enzymes, which play a key role in the reduction, capping, fabrication, and stabilization of nanoparticles.201–203 The use of algae in the production of nanoparticles is considered a safe, simple, cost-effective, and environmentally friendly approach.116
Table 9 Biosynthesis of iron oxide nanoparticles using algae
Algae |
Biomaterial used |
Iron precursor salt |
Size |
Shape |
Applications |
Ref. |
Spirulina platensis
|
Powder |
FeCl3·6H2O (from 0.1 to 0.6 M) |
<10 nm |
Slightly irregular and rounded |
Adsorptive removal of cationic and anionic dyes |
107
|
Sargassum vulgare (Phaeophyceae) |
Powder |
FeCl3 (0.1 M) |
22.73 nm |
Nanospheres |
Antibiofilm |
204
|
Ulva fasciata (Chlorophyceae) |
Powder |
FeCl3 (0.1 M) |
28.41 nm |
Nanospheres |
Antibiofilm |
204
|
Jania rubens (Rhodophceae) |
Powder |
FeCl3 (0.1 M) |
27.78 nm |
Nanospheres |
Antibiofilm |
204
|
Sargassum crassifolium
|
Dried powder |
(FeCl3 : FeCl2) (0.1 : 0.05 and 0.02 : 0.01) |
40–215 nm |
Quasi-spherical |
— |
205
|
Chlorella vulgaris
|
Powder |
FeSO4·7H2O and Fe(NO3)3·9H2O |
4.855, 5.702 and 3.614 nm |
Amorphous biochar |
Adsorbent for dye removal |
206
|
Aegagropila linnaei
|
Powder |
FeSO4·7H2O (0.01 mol) |
100–150 nm |
Geomorphic |
Adsorption and Fenton-like reaction |
207
|
Ulva lactuca
|
Powder |
FeCl3·6H2O (28 mM), FeSO4·7H2O (14 mM) |
50–80 nm |
Spherical |
Adsorptive removal of Pb(II) from heavy metal bearing water |
208
|
Ulva prolifera
|
Dried-refrigerated powder |
FeSO4·7H2O (0.1 M) |
41.23 nm |
Spherical |
As(III) removal |
209
|
3. Structure and design
Functionalized polymeric MNPs exhibit certain distinguished features for drug delivery in terms of effectiveness and efficiency compared to traditional oral and intravenous techniques. This is because they can control the particle size, morphology, and surface charge,210–212 which enhance the drug delivery and release by joining with other molecules such as antibodies, proteins, and ligands. This can help reduce the side effects associated with chemotherapy, radiotherapy, and surgery.213 MNPs used for biomedical purposes are often composed of metals such as iron and iron oxide, which can possess a variety of morphologies.
Iron oxide MNPs with nanocrystalline magnetite (Fe3O4) cores are preferred for biomedical applications because of their biocompatibility (they swiftly decompose into non-toxic iron and oxygen elements in vivo), biodegradability,214–216 and ease of manufacturing.135,217 Iron oxide nanoparticles (FeO-NPs) are classified into two categories, i.e., superparamagnetic iron oxide nanoparticles (SPIONs) and ultra-small superparamagnetic iron oxide nanoparticles (USPIONs). These two classes have different relaxometry properties and mean hydrodynamic sizes.214 SPIONs are made of iron oxide cores with average diameters in the range of 3–20 nm and composed of agglomerates with a hydrodynamic diameter of more than 50 nm.214 Therefore, any spherical FeO-NPs with a diameter equal to or less than 20 nm will exhibit SPION behavior, which can be used to facilitate targeted drug delivery in the treatment of oncological diseases (Fig. 6).
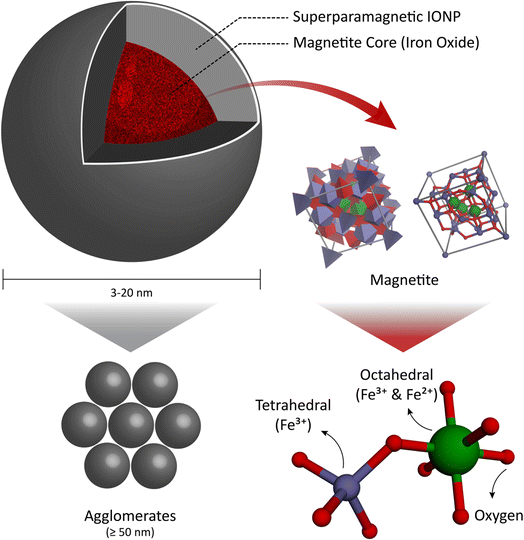 |
| Fig. 6 (a) Superparamagnetic iron oxide nanoparticles (SPIONs). (b) Face-centered cubic (FCC) closed packing, with Fe3+ in the tetrahedral sites and Fe2+ occupying the octahedral sites. | |
Magnetite has a face-centered cubic (FCC), closed packing cubic, and inverse spinel structure with the ferric (Fe3+) ion occupying all the tetrahedral (Th) sites and both ferric (Fe3+) and ferrous (Fe2+) ions occupying the octahedral (Oh) sites. It has attracted significant attention due to the hopping of electrons between Fe2+ and Fe3+ ions in its octahedral lattice at ambient temperature, as well as its low toxicity (Fig. 6). This is because the iron oxide core of magnetite degrades to low molecular weight iron, making it a useful material in biomedical applications and an effective carrier for drug delivery to target locations, avoiding the negative effects of oral and intravenous drug delivery.218–227 This is due to the unique properties of magnetite, such as its biocompatibility, lack of toxicity, targeting ability, biodegradability, chemical stability, stable dispersion, and magnetic stability.228–232
Due to the anisotropic dipolar attraction and high surface energies of FeO-NPs, large surface-to-volume ratio unmodified FeO-NPs tend to form clusters of large aggregates, which can reduce their surface area to volume ratio and decrease their effectiveness. Additionally, FeO-NPs are prone to oxidation in air, which can lead to the loss of magnetization because of their chemical activity from the oxidation of ferrous (Fe2+) to ferric (Fe3+) ions. Thus, to avoid this oxidation, experiments with FeO-NPs are often conducted under dry conditions.232–234
4. Characteristics and applications of IONzymes
Nanomaterials have established numerous novel applications to improve human health, ranging from diagnosis to therapeutic effects, control, and monitoring environment pollution, together with improving the chemical industry.235–237 Iron oxide nanomaterials have versatile applications that are not limited to their magnetic properties. IONzymes are considered one of the most representative nanozymes being explored for their kinetics and catalytic properties.238 IONzymes have several benefits in real applications, particularly in biomedicine. In this section (Fig. 7), we focus on the biomedical and environmental applications of IONzymes.
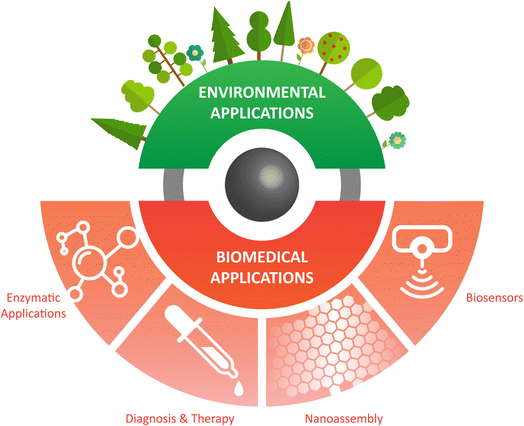 |
| Fig. 7 Applications of IONzymes. | |
4.1. Characteristics of IONzymes
Recently, diverse nanomaterials with enzyme-like actions were discovered with catalytic properties such as the natural oxidoreductase enzyme family as artificial enzymes or enzyme mimics.239–243 Recently, researchers have utilized IONzymes in numerous innovative biomedical applications due to their enzyme-like activities.244–246 In addition, the features of IONzymes are not limited to catalytic activity, where they are also widely applied as biosensors, biomarkers, and in immunoassay approaches.247–249 Here, we intend to highlight the importance of IONzymes in biomedical applications.
4.1.1. Enzymatic-like characteristics.
In 2007, it was reported for the first time that iron oxide nanomaterials display enzymatic-like characteristics. Gao et al. stated that FeO-NPs showed basic peroxidase (POD)-like activity, with catalytic behavior similar to horseradish POD (HRP).250 Since then, IONzymes and their typical POD and catalase (CAT)-like activities have attracted attention because they have been proven to work under physiological conditions like natural enzymes, including the same substrate, pH, and temperature. Moreover, they follow similar kinetics and pathways as conventional enzymes.251–255 IONzymes are stated to mimic the peroxidase and catalase enzymes. Both enzymes have a porphyrin heme as a cofactor in their active site and utilize hydrogen peroxide as the substrate. Also, both enzymes play a crucial role in avoiding cellular oxidative damage in aerobically respiring creatures by forming free radicals and oxygen.240,256,257
4.1.1.1. IONzymes mimic POD activity.
POD-like activity was verified for both Fe2O3 and Fe3O4 IONzymes, which catalyzed a colorimetric reaction including hydrogen peroxide (H2O2) utilizing the same optimal conditions as HRP at the physiological temperature and in acidic media.258 In addition, IONzymes can function over several substrates, including 3,3′,5,5′-tetramethylbenzidine (TMB), o-phenylenediamine (OPD), 3,3′-diaminobenzidine (DAB), 2,2′-azino-bis(3-ethylbenzthiazoline-6-sulfonic acid) (ABTS),250,259,260 polydopamine,261 terephthalic acid (TA),262 luminol, and benzoic acid.263 Moreover, IONzymes could peroxidize biomolecules such as proteins, nucleic acids, sugars,264 and lipids.265
Furthermore, the enzymatic activity of IONzymes similar to natural PODs can be affected by several natural effectors. ATP, ADP, AMP,265–267 and DNA are the main activators to improve the POD-like activity of IONzymes by involving them in the electron transfer mechanism.268,269 Free radical quenchers as sodium azide, ascorbic acid, hypotaurine, and catecholamines were found to decrease the POD activity of IONzymes270,271 by delaying the affinity of the substrate to IONzymes more than quenching the free radicals.238
4.1.1.2. IONzymes mimic catalase activity.
IONzymes have been reported to exhibit catalase-like activity via H2O2 decomposition under neutral and high pH conditions. As previously described for POD and proven by Chen et al., pH plays a significant role in the effectiveness of the H2O2 decomposition rate.259
4.1.1.3. Kinetics of IONzymes.
IONzymes, as POD and CAT enzymes, follow Michaelis–Menten behavior.272 The apparent affinity of a substrate to the enzyme (KM) value for H2O2 was higher for IONzymes compared to the native HRP, indicating that IONzymes have a lower affinity to H2O2 than HRP by nearly 41-fold.250 Alternatively, the KM value for TMB against IONzymes was lower than that of HRP, indicating that the IONzymes have a higher affinity to TMB than the natural enzyme.273 Given that IONzymes have an abundance of iron ions, this increases their POD activity by around 40-times compared to HRP.250
The rate of the CAT activity depends on the O2 production rate in the solution. IONzymes also adopt Michaelis–Menten kinetics for the CAT reaction.259 The volumetric measurement of oxygen gas is influenced by many other parameters, such as temperature and O2 diffusion and can be attained by a volumetric bar-chart chip.274
4.1.1.4. Mechanism of action of IONzymes.
IONzymes show a catalytic mechanism similar to HRP, where they react with H2O2 to form hydroxyl free radicals (˙OH) as an intermediate state like the POD enzyme state. ˙OH captures H+ from the hydrogen donor such as TMB.265 Interestingly, the produced ˙OH does not have reaction specificity and can bind to any hydrogen donors, leading to a wide range of applications.275
During the activity of IONzymes, two types of free radicals are produced, i.e., ˙OH and hydroperoxyl (
) radicals. The ferryl ion (FeO2+) that is typically formed in POD catalysis is not detected in the POD-like IONzyme activity but produced in the CAT-like IONzyme activity.276,277 Moreover, IONzymes have two iron types, i.e., Fe2+ and Fe3+, where Fe2+ ions may play a major role in their catalytic POD-like activity.250
The POD-like activity arises also from the integral nanoparticles rather than free iron ions, and thus the IONzyme mechanism performance includes kinetic procedures involving substrate binding, surface reaction, and product release, displaying similar enzymatic kinetics.278,279 Furthermore, IONzymes can be utilized as an exceptional carrier to load other enzymatic functionalities on their surface. For example, glucose oxidase (GOx) can form a new nano-complex by GOx catalyzing glucose to produce hydrogen peroxide, which in turn can be catalyzed by IONzymes.280
4.2. Biomedical applications of IONzymes
4.2.1. Immunoassay, diagnosis, therapy, and biomarker detection.
Based on the superiority of IONzymes over HRP, they can be used as an alternative to HRP in the enzyme-linked immunosorbent assay (ELISA) and other associated molecular detection procedures through the conjugation of antibodies.281,282 Based on the superparamagnetism of IONzymes, they can be used to enhance antigen detection at low concentrations.283 Gao et al. developed chitosan-modified magnetic nanoparticles (CS-MNPs) as additional enzymes in conventional ELISA configurations, with 1 ng mL−1 detection limit for a carcinoembryonic antigen (CEA).281 Similar approaches were adapted to detect other antigens or pathogens, containing immunoglobulin G (IgG), hepatocellular carcinoma biomarker Golgi protein 73 (GP73),284 human chorionic gonadotropin (HCG),285 mycoplasma pneumonia,286Vibrio cholerae, rotavirus,287 cancer cells with human epidermal growth factor receptor 2 (HER2),287,288 and epidermal growth factor receptor (EGFR).289 Daun et al. developed an iron oxide nanozyme-strip to sense Ebola virus (EBOV) with a detection limit as low as 1 ng mL−1 for EBOV glycoprotein.290 Moreover, the surface of IONzymes was covered with streptavidin to attain signal amplification via IONzyme catalysis by Thiramanas et al. to sense Vibrio cholerae with a sensitivity of 103 CFU mL−1 in drinking and tap water.291 Moreover, Zhang et al. established a colorimetric aptasensor for the determination of thrombin by employing chitosan-modified Fe3O4 (MNPs). The results exhibited that the thrombin absorption values improved in a concentration-dependent manner with a linear range from 1 to 100 nM.292 Based on the aptamer conjugated to the IONzymes, Fe3O4 NPs with an aptamer-based immunosorbent assay (NAISA) were developed for aflatoxin B1 (AFB1) recognition with better operation and separation. The aptamer was implemented to diagnose AFB1, and this method showed a limit of detection of 5 pg mL−1 (Fig. 8).293
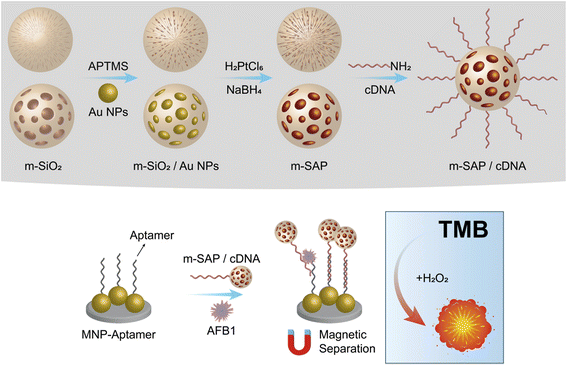 |
| Fig. 8 Schematic presentation of nanozyme and aptamer-based immunosorbent assay (NAISA): (A) preparation of m-SAP/cDNA and (B) construction of NAISA for AFB1 detection. | |
Magnetoferritin NPs (M-HFn) are a certain type of IONzymes that are linked to the recombinant human heavy-chain ferritin (HFn) protein shell, which binds to transferrin receptor 1 (TfR1) overexpressed in most tumor cells.294 This approach enables tumor diagnosis by utilizing the POD/CAT functionality of the Fe3O4 core to yield a color reaction, which can be utilized to visualize cancer tissues. This strategy can differentiate cancerous cells from normal cells with a sensitivity of 98% and specificity of 95%.238,295 IONzymes and their POD activity can be used in bio-distribution studies. Based on this technique, Zhuang reported that dextran-coated Fe3O4 NPs were confined in the liver, spleen, and lungs more than the kidney, lymph nodes, and thymus (Fig. 9A).295
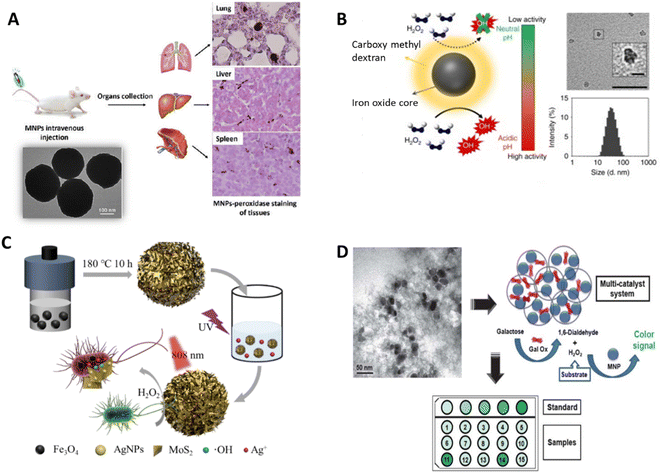 |
| Fig. 9 Schematic illustration of (A) investigation of dextran-coated Fe3O4 NPs in the liver, spleen, and lungs295 and (B) pH-dependent catalytic activity of ferumoxytol. (Insets) negative stain TEM of ferumoxytol (Scale bar: 50 nm and 10 nm for close image) and hydrodynamic diameter measurements.298 (C) Recoverable peroxidase-like Fe3O4@MoS2-Ag nanozyme with enhanced antibacterial ability.303 (D) Multi-catalyst system for the quantification of galactose, entrapping both MNPs and Gal Ox in mesocellular silica.316 | |
In addition, IONzymes showed a therapeutic effect on tumor cells and against bacterial growth by catalyzing H2O2 to produce toxic radicals.296,297 To increase the intracellular H2O2 concentration, H2O2 was directly injected, or an enzyme was merged to generate H2O2. The former showed a significant inhibition efficacy against a mouse model bearing subcutaneous HeLa tumors.296 Ferumoxytol was utilized with a low concentration of H2O2 to fight oral biofilms and avoid dental decay. Ferumoxytol, carboxymethyl dextran-coated IONzymes could catalyze the decomposition of H2O2 to hydroxyl radicals (Fig. 9B).298 However, due to the increased toxicity of the H2O2 injection option, incorporating an H2O2-producing enzyme is considered an efficient and safer choice. For example, Huo et al. reported that Fe3O4 NPs and GOx co-entrapped in mesoporous silica NPs could be used for tumor catalytic therapy.299
Moreover, iron-based NPs can cause generate sufficient reactive oxygen species (ROS) to induce apoptosis in tumor cells into (ferroptosis).300,301 For example, Fe3+ is reduced to Fe2+ by the overexpressed glutathione (GSH) in tumor tissues, leading to the promotion of ROS production and resultant tumor ferroptosis.257,302 In addition, Wei et al. developed Fe3O4@MoS2-Ag IONzymes that showed a good antibacterial effect against E. coli (∼69.4%) by the generated ROS through POD-like activity and released Ag+ (Fig. 9C).303 Furthermore, Wang et al. conveyed that a new cobalt-doped Fe3O4 (Co@Fe3O4) IONzyme exhibited better POD activity and a 100-fold higher affinity to H2O2 than Fe3O4 nanozymes to generate ROS for kidney tumor catalytic therapy in vitro and in vivo, presenting a potential novel avenue for tumor nanozyme catalytic treatment.304 Similarly, Sun et al. improved highly toxic ROS levels from iron oxide core–shell mesoporous silica nanocarrier-mediated Fenton reactions for cancer therapy.305 Furthermore, Li et al. prepared an H2O2-responsive POD and CAT-mimic PtFe@Fe3O4 IONzyme, which displaced a 99.8% anti-tumor rate for deep pancreatic cancer in collaboration with photothermal treatment.306 In a recent study, the application of adenosine triphosphate disodium salt (ATP) as a synergistic agent increased the generation of OH radicals and restored the antibacterial activity of Fe3O4 IONzymes over the full pH range against both Gram-positive (B. subtilis) and Gram-negative (E. coli) bacterial strains in the presence of H2O2 at a pH of around 7.0.307
4.2.2. Enzyme-IONzyme nanoassembly.
This approach utilizes IONzymes loaded with oxidase enzymes to enable the fast colorimetric detection of biomolecules. The natural enzyme usually produces H2O2 as an intermediate, which is catalyzed by the activity of the POD like-IONzymes.308
Glucose oxidase is the main enzyme assembled with IONzymes for the detection of glucose.309,310 Firstly, GOx catalyzes glucose to produce H2O2, which is then catalyzed by IONzymes, and a color signal can be formed related to the amount of glucose with a detection limit of 0.5 to 3 μM.311–314 Other oxidases and esterases can also be utilized in this approach such as cholesterol oxidase for cholesterol detection,315,316 galactose oxidase (Gal Ox) for galactose (Fig. 9D),316 alcohol oxidase (AlOx) for alcohol,317 and acetylcholine esterase (AChE) for acetylcholine (ACh).265
4.2.3. Biosensors.
Several IONzymes have been used by researchers for the development of IONzyme-based biosensors for biomedical applications. IONzyme-based biosensors are based on the mimicking activity of IONzymes and can be categorized into three main groups: POD, oxidase, and CAT mimics.318Table 10 summarizes the reported IONzyme-based biosensors together with their enzyme-simulating activities and sensing mechanism.
Table 10 IONzyme-based biosensors and their type of enzyme-mimicking activities and sensing procedures
IONzymes |
Enzyme-mimicking activities |
Biotarget |
Biosensor type |
Ref. |
Fe3O4 |
POD |
Ebola virus |
Colorimetric |
319
|
Fe3O4-Pt/core–shell |
POD |
Human chorionic gonadotropin (hCG) |
Colorimetric (paper-based strip) |
320
|
Fe-MOF-Au NPs |
POD |
Salmonella enteritidis
|
Colorimetric immunosensor |
321
|
Fe3O4 |
POD |
Glucose |
Colorimetric |
322
|
Fe3O4 |
POD |
Listeria monocytogenes
|
Colorimetric |
323
|
Fe3O4 |
POD |
Prostate-specific antigen |
Photoelectrochemical (PEC) immunoassay |
324
|
Fe3O4 |
POD |
Micro RNA |
Electrochemical |
325
|
Fe3O4 |
POD |
Hepatitis B virus surface antigen (preS1) |
Colorimetric, immunoassay |
250
|
Fe@PCN-224 NPs |
POD |
Glucose |
Colorimetric |
326
|
Fe3O4@C |
POD |
Platelet-derived growth factor BB (PDGF-BB) |
Colorimetric |
327
|
Fe3O4/CoFe-LDH hybrid |
POD |
Ascorbic acid |
Colorimetric |
328
|
4.3. Environmental applications of IONzymes
Due to their high catalytic activity, stability, and multifunctionality, IONzymes have shown an increasingly wide range of applications in the biomedical, agricultural, and environmental fields.329–331 Given that IONzymes possess intrinsic POD and CAT properties and follow a Fenton and/or Haber–Weiss reaction mechanism (including ˙OH/
), they can be utilized for the degradation of organic pollutants by combining free radical production with the magnetic characteristics of iron oxide.332 Moreover, the catalytic activity IONzymes can be used for environmental monitoring, for example, detecting H2O2 in rainwater and measuring heavy metals in environmental samples. The environmental applications of IONzymes are considered suitable for numerous environmental conditions, relatively easy and cheap, and can be simply applied to the screening of pesticides, organophosphorus compounds, and other ingredients. IONzymes can determine pollutants indirectly when they enable a target to undergo an alteration in chemical properties and react with the colorimetric sensor to be detected.333
A histidine-modified Fe3O4 IONzyme offered an easy, inexpensive approach to detect Ag+ with a detection limit of 18 fg mL−1.334 4-Chloro-1-naphthol was utilized as a substrate, in which the Fe3O4 IONzyme POD enzyme activity was activated in the presence of Ag+, which produced the insulating precipitation of benzo-4-chlorohexadienone. The insulating products attenuated the photocurrent signal, reflecting the presence of Ag+. Guo et al. developed an excellent colorimetric selective method for the detection of Hg2+ based on the stimulus of the intrinsic oxidase-like catalytic activity of Ag–CoFe2O4/rGO NPs via a one-pot microwave-assisted reaction, which can oxidize 3,3′,5,5′-tetramethylbenzidine (TMB) to yield a light-blue product.335
Recently, IONzymes have been established as anti-microbial for environmental treatments. IONzymes effectively inactivate viruses (IAVs) via envelope lipid peroxidation and destruction of the integrity of neighboring proteins, including hemagglutinin, neuraminidase, and matrix protein. Furthermore, IONzymes possess a broad-spectrum antiviral activity against 12 subtypes of IAVs 244 (H1–H12).336
In the treatment of organic pollutants in water, ferromagnetic chitosan IONzymes (MNP@CTS), which have superior catalytic activity and exceptional POD activity, were produced for the degradation of phenol. MNP@CTS removed over 95% of phenol from an aqueous solution within 5 h under the optimum conditions (pH range of 2–10).337
Huo et al. showed that IONzymes can enhance the performance of plants under unfavorable conditions such as abiotic stresses. They studied the effect of a 25 ppm IONzyme dose on Eucalyptus tereticornis against a high salinity concentration of 300 mM NaCl. The IONzymes showed a separate biochemical change in superoxide dismutase, malondialdehyde concentration, and total soluble sugar and proline content, which are biomarkers that circumvent the stress response and synergistically improve the activity of CAT and POD enzymes.338
Recently, Fe3O4-TiO2/reduced graphene oxide (Fe3O4-TiO2/rGO) NPs with hydrogen peroxide activity and photocatalytic efficiency were designed for the colorimetric detection of atrazine pesticides, which can cause long-term negative effects because of their persistence. TMB was used as the substrate compound with a detection limit of 2.98 μg L−1.339 Moreover, the POD-like activity of IONzymes was utilized in water purification in another study.340–343 This designates the promising application potential of Fe3O4 IONzymes in water treatment and quality analysis.
4. Future scope and drawbacks of IONzymes
The future potential as and present limitations of the applications of IONzymes must be considered for their development. IONzymes have enormous potential for use in the environmental and biomedical fields. Employing IONzymes in targeted drug delivery systems and improved diagnostics is one field of research and development that has great potential.344 Thorek et al. suggested that IONzymes may revolutionize magnetic resonance imaging (MRI) by improving the imaging contrast and specificity. However, there are a few issues and disadvantages that need to be resolved. One of the main challenges is still their long-term biocompatibility and toxicity, particularly for in vivo applications.345
Although iron oxides are considered to be biocompatible in general, Szalay et al. proposed that further research is needed to determine their long-term impacts in biological systems.346 Another serious obstacle is the synthesis of IONzymes on a large scale for industrial use. Researchers emphasized that the shift from laboratory-scale production to large-scale manufacturing frequently leads to instabilities in particle size and enzyme activity, which can hinder their practical implementation.346
One more crucial element is the stability of IONzymes in physiological settings. Thus, enhancing their stability through surface modifications, while maintaining their enzymatic activity under various pH and temperature conditions, is crucial for their effective use in biological systems.38 In addition, achieving high specificity in the catalytic action of IONzymes remains a major research goal. As noted by Zhang and co-workers, tailoring nanozymes to exhibit enzyme-like specificity is a complex but vital aspect for their application in both the medical and environmental fields. Enabling the actual deployment of IONzymes requires addressing these obstacles via inventive research and technological developments.39 To fully utilize the promise of IONzymes in a variety of applications, future research should focus on improving their biocompatibility, scalability, stability, and specificity.346
5. Conclusion
In conclusion, the study of IONzymes has seen significant advances in recent years. IONzymes can be synthesized using chemical, physical, and biological techniques and offer unique advantages for various applications, including biomedical and environmental purposes. IONzymes have been explored for their enzymatic properties and used in enzyme mimicry, immunoassays, diagnosis, therapy, and biomarker detection. Thus, the versatile nature of IONzymes, combined with their biocompatibility and biodegradability, make them a promising area for continued research and development.
Author contributions
Ghazzy A, conceptualization, writing – original draft, writing, review & editing, supervision; Nsairat H, writing – original draft; Said R, writing – original draft, writing, review & editing; Sibai1 O, writing – original draft, resources; AbuRuman A, writing – original draft; Shraim A, visualization & editing; Al hunaiti A, conceptualization.
Conflicts of interest
There are no conflicts to declare.
Acknowledgements
A. G. would like to acknowledge support by Deanship of Scientific Research at Al-Ahliyya Amman University. A. A.-H. Acknowledges support by the Deanship of Scientific Research at the University of Jordan.
References
- G. A. Silva, Introduction to nanotechnology and its applications to medicine, Surg. Neurol., 2004, 61, 216–220 CrossRef PubMed.
- V. Amendola and M. Meneghetti, What controls the composition and the structure of nanomaterials generated by laser ablation in liquid solution?, Phys. Chem. Chem. Phys., 2013, 15, 3027–3046 RSC.
- B. T. Sone, A. Diallo, X. G. Fuku, A. Gurib-Fakim and M. Maaza, Biosynthesized CuO nano-platelets: Physical properties & enhanced thermal conductivity nanofluidics, Arabian J. Chem., 2020, 13, 160–170 CrossRef CAS.
- A. U. Khan, M. Khan, N. Malik, M. H. Cho and M. M. Khan, Recent progress of algae and blue–green algae-assisted synthesis of gold nanoparticles for various applications, Bioprocess Biosyst. Eng., 2019, 42, 1–15 CrossRef CAS PubMed.
- A. Ash, K. Revati and B. D. Pandey, Microbial synthesis of iron-based nanomaterials – A review, Bull. Mater. Sci., 2011, 34, 191–198 CrossRef.
- H. Geng, Y. Peng, L. Qu, H. Zhang and M. Wu, Structure Design and Composition Engineering of Carbon-Based Nanomaterials for Lithium Energy Storage, Adv. Energy Mater., 2020, 10, 1–34 Search PubMed.
- X. Zhang, W. Li, H. Kou, J. Shao, Y. Deng, X. Zhang, J. Ma, Y. Li and X. Zhang, Temperature and size dependent surface energy of metallic nano-materials, J. Appl. Phys., 2019, 125, 185105 CrossRef.
- S. Dang, Q. L. Zhu and Q. Xu, Nanomaterials derived from metal-organic frameworks, Nat. Rev. Mater., 2017, 3, 1–14 Search PubMed.
- J. T. Lue, A review of characterization and physical property studies of metallic nanoparticles, J. Phys. Chem. Solids, 2001, 62, 1599–1612 CrossRef CAS.
- V. V. Mody, R. Siwale, A. Singh and H. R. Mody, Introduction to metallic nanoparticles, J. Pharm. BioAllied Sci., 2010, 2, 282–289 CrossRef CAS.
- K. I. Bogutska, Y. P. Sklyarov and Y. I. Prylutskyy, Zinc and zinc nanoparticles : biological role and application in biomedicine, Ukrainica Bioorganica Acta, 2013, 1, 9–16 Search PubMed.
- S. G. Kim, Y. Terashi, A. Purwanto and K. Okuyama, Synthesis and film deposition of Ni nanoparticles for base metal electrode applications, Colloids Surf., 2009, 337, 96–101 CrossRef CAS.
- M. Parashar, V. K. Shukla and R. Singh, Metal oxides nanoparticles via sol–gel method: a review on synthesis, characterization and applications, J. Mater., 2020, 31, 3729–3749 CAS.
- S. U. Son, I. K. Park, P. Jongnam and T. Hyeon, Synthesis of Cu2O coated Cu nanoparticles and their successful applications to Ullmann-type amination coupling reactions of aryl chlorides, Chem. Commun., 2004, 4, 778–779 RSC.
- A. Rita, A. Sivakumar, S. S. J. Dhas and S. A. M. B. Dhas, Structural, optical and magnetic properties of silver oxide (AgO) nanoparticles at shocked conditions, J. Nanostruct. Chem., 2020, 10, 309–316 CrossRef.
- B. P. Vinayan and S. Ramaprabhu, Facile synthesis of SnO2 nanoparticles dispersed nitrogen doped graphene anode material for ultrahigh capacity lithium ion battery applications, J. Mater. Chem. A, 2013, 1, 3865–3871 RSC.
- B. Mughal, S. Z. J. Zaidi, X. Zhang and S. U. Hassan, Biogenic nanoparticles: Synthesis, characterisation and applications, Appl. Sci., 2021, 11, 2598 CrossRef CAS.
- M. Mahdavi, M. B. Ahmad, M. J. Haron, F. Namvar, B. Nadi, M. Z. Ab Rahman and J. Amin, Synthesis, surface modification and characterisation of biocompatible magnetic iron oxide nanoparticles for biomedical applications, Molecules, 2013, 18, 7533–7548 CrossRef CAS PubMed.
- D. Ling and T. Hyeon, Iron Oxide Nanoparticles Chemical Design of Biocompatible Iron Oxide Nanoparticles for Medical Applications, Small, 2013, 9, 1449 CrossRef CAS.
- M. A. Wahab, S. M. A. Hossain, M. K. Masud, H. Park, A. Ashok, M. Mustapić, M. Kim, D. Patel, M. Shahbazi and M. S. A. Hossain, Nanoarchitectured superparamagnetic iron oxide-doped mesoporous carbon nanozymes for glucose sensing, Sens. Actuators, B, 2022, 366, 131980 CrossRef CAS.
- Q. Chen, X. Zhang, S. Li, J. Tan, C. Xu and Y. Huang, MOF-derived Co3O4@Co-Fe oxide double-shelled nanocages as multi-functional specific peroxidase-like nanozyme catalysts for chemo/biosensing and dye degradation, Chem. Eng. J., 2020, 395, 125130 CrossRef CAS.
- Y. Wang, W. Wang, Z. Gu, X. Miao, Q. Huang and B. Chang, Temperature-responsive iron nanozymes based on poly(N-vinylcaprolactam) with multi-enzyme activity, RSC Adv., 2020, 10, 39954–39966 RSC.
- Y. Lin, J. Ren and X. Qu, Catalytically active nanomaterials: A promising candidate for artificial enzymes, Acc. Chem. Res., 2014, 47, 1097–1105 CrossRef CAS PubMed.
- T. Kang, Y. G. Kim, D. Kim and T. Hyeon, Inorganic nanoparticles with enzyme-mimetic activities for biomedical applications, Coord. Chem. Rev., 2020, 403, 213092 CrossRef CAS.
- K. Boriachek, M. K. Masud, C. Palma, H. P. Phan, Y. Yamauchi, M. S. A. Hossain, N. T. Nguyen, C. Salomon and M. J. A. Shiddiky, Avoiding pre-isolation step in exosome analysis: Direct isolation and sensitive detection of exosomes using gold-loaded nanoporous ferric oxide nanozymes, Anal. Chem., 2019, 91, 3827–3834 CrossRef CAS PubMed.
- P. Wang, T. Wang, J. Hong, X. Yan and M. Liang, Nanozymes: A New Disease Imaging Strategy, Front. Bioeng. Biotechnol., 2020, 8, 1–10 Search PubMed.
- B. Wang, A. Moyano, J. M. Duque, L. Sánchez, G. García-Santos, L. J. G. Flórez, E. Serrano-Pertierra and M. D. C. Blanco-López, Nanozyme-Based Lateral Flow Immunoassay (LFIA) for Extracellular Vesicle Detection, Biosensors, 2022, 12, 1–13 Search PubMed.
- Z. Nie, Y. Vahdani, W. C. Cho, S. H. Bloukh, Z. Edis, S. Haghighat, M. Falahati, R. Kheradmandi, L. A. Jaragh-Alhadad and M. Sharifi, 5-Fluorouracil-containing inorganic iron oxide/platinum nanozymes with dual drug delivery and enzyme-like activity for the treatment of breast cancer, Arabian J. Chem., 2022, 15, 103966 CrossRef CAS.
- Y. He, X. Chen, Y. Zhang, Y. Wang, M. Cui, G. Li, X. Liu and H. Fan, Magnetoresponsive nanozyme: magnetic stimulation on the nanozyme activity of iron oxide nanoparticles, Sci. China Life Sci., 2021, 65, 184–192 CrossRef PubMed.
- G. Tang, J. He, J. Liu, X. Yan, K. Fan and C. Xiyun Yan, Nanozyme for tumor therapy: Surface modification matters, Exploration, 2021, 1, 75–89 CrossRef.
- K. Fan, H. Wang, J. Xi, Q. Liu, X. Meng, D. Duan, L. Gao and X. Yan, Optimization of Fe3O4 nanozyme activity via single amino acid modification mimicking an enzyme active site, Chem. Commun., 2016, 53(2), 424–427 RSC.
-
R. Zhang, H. Zhao, and K. Fan, in Nanozymes: Design, Synthesis, and Applications, ed. X. Wang, American Chemical Society, Washington, DC, first edition, 2022, ch. 1, pp. 1–35 Search PubMed.
- Y. Chen, J. Zhang, Z. Wang and Z. Zhou, Solvothermal Synthesis of Size-Controlled Monodispersed Superparamagnetic Iron Oxide Nanoparticles, Appl. Sci., 2019, 9, 5157 CrossRef CAS.
- H. Zou, J. Zhao, F. He, Z. Zhong, J. Huang, Y. Zheng, Y. Zhang, Y. Yang, F. Yu, M. A. Bashir and B. Gao, Ball milling biochar iron oxide composites for the removal of chromium (Cr(VI)) from water: Performance and mechanisms, J. Hazard. Mater., 2021, 413, 125252 CrossRef CAS PubMed.
- L. Gao, J. Zhuang, L. Nie, J. Zhang, Y. Zhang, N. Gu, T. Wang, J. Feng, D. Yang, S. Perrett and X. Yan, Intrinsic peroxidase-like activity of ferromagnetic nanoparticles, Nat. Nanotechnol., 2007, 2, 577–583 CrossRef CAS PubMed.
- M. Wu, H. Guo, L. Liu, Y. Liu and L. Xie, Size-dependent cellular uptake and localization profiles of silver nanoparticles, Int. J. Nanomed., 2019, 14, 4247–4259 CrossRef CAS PubMed.
- H. Wei and E. Wang, Fe3O4 magnetic nanoparticles as peroxidase mimetics and their applications in H2O2 and glucose detection, Anal. Chem., 2008, 80, 2250–2254 CrossRef CAS PubMed.
- S. Laurent, D. Forge, M. Port, A. Roch, C. Robic, L. Vander Elst and R. N. Muller, Magnetic iron oxide nanoparticles: synthesis, stabilization, vectorization, physicochemical characterizations, and biological applications, Chem. Rev., 2008, 108, 2064–2110 CrossRef CAS PubMed.
- S. Zhang, X. Zhang, G. Jiang, H. Zhu, S. Guo, D. Su, G. Lu and S. Sun, Tuning nanoparticle structure and surface strain for catalysis optimization, J. Am. Chem. Soc., 2014, 136, 7734–7739 CrossRef CAS PubMed.
-
T. Athar, in Emerging Nanotechnologies for Manufacturing, ed. W. Ahmed and M. J. Jackson, William Andrew Publishing, Elsevier Inc, second edition, 2015, ch. 17, pp. 444–538 Search PubMed.
-
N. S. Satpute and S. J. Dhoble, in Energy Materials: Fundamentals to Applications, ed. S. J. Dhoble, N. T. Kalyani,B. Vengadaesvaran and A. K. Arof, Elsevier, first edition, 2021, ch. 14, pp. 407–444 Search PubMed.
-
J. Chang, Y. L. Zhou and Y. Zhou, in Bioactive Glasses: Materials, Properties and Applications, ed. H. O.Ylänen, Woodhead Publishing, first edition, 2011, ch. 2, pp. 29–52 Search PubMed.
-
A. Sarkar, N. Saha and G. Majumdar, in Encyclopedia of Materials: Plastics and Polymers, ed. M. S. J. Hashmi, Elsevier Inc, first edition, 2022, vol. 2, pp. 646–655 Search PubMed.
- R. Massart, Preparation of Aqueous Magnetic Liquids in Alkaline and Acidic Media, IEEE Trans. Magn., 1981, 17, 1247–1248 CrossRef.
- J. C. Aphesteguy, G. V. Kurlyandskaya, J. P. De Celis, A. P. Safronov and N. N. Schegoleva, Magnetite nanoparticles prepared by co-precipitation method in different conditions, Mater. Chem. Phys., 2015, 161, 243–249 CrossRef CAS.
- S. Laurent, D. Forge, M. Port, A. Roch, C. Robic, L. Vander Elst and R. N. Muller, Magnetic iron oxide nanoparticles: synthesis, stabilization, vectorization, physicochemical characterizations, and biological applications, Chem. Rev., 2008, 108, 2064–2110 CrossRef CAS PubMed.
- A. M. Mazrouaa, M. G. Mohamed and M. Fekry, Physical and magnetic properties of iron oxide nanoparticles with a different molar ratio of ferrous and ferric, Egypt. J. Pet., 2019, 28, 165–171 CrossRef.
- S. P. Schwaminger, C. Syhr and S. Berensmeier, Controlled Synthesis of Magnetic Iron Oxide Nanoparticles: Magnetite or Maghemite?, Crystals, 2020, 10, 214 CrossRef CAS.
- A. V. Samrot, C. S. Sahithya, A. J. Selvarani, S. K. Purayil and P. Ponnaiah, A review on synthesis, characterization and potential biological applications of superparamagnetic iron oxide nanoparticles, Curr. Res. Green Sustainable Chem., 2021, 4, 100042 CrossRef CAS.
- M. O. Besenhard, A. P. LaGrow, A. Hodzic, M. Kriechbaum, L. Panariello, G. Bais, K. Loizou, S. Damilos, M. M. Cruz, N. T. K. Thanh and A. Gavriilidis, Co-precipitation synthesis of stable iron oxide nanoparticles with NaOH: New insights and continuous production via flow chemistry, Chem. Eng. J., 2020, 399, 125740 CrossRef CAS.
- A. P. Lagrow, M. O. Besenhard, A. Hodzic, A. Sergides, L. K. Bogart, A. Gavriilidis and N. T. K. Thanh, Unravelling the growth mechanism of the co-precipitation of iron oxide nanoparticles with the aid of synchrotron X-Ray diffraction in solution, Nanoscale, 2019, 11, 6620–6628 RSC.
- B. Chen, J. Sun, F. Fan, X. Zhang, Z. Qin, P. Wang, Y. Li, X. Zhang, F. Liu, Y. Liu, M. Ji and N. Gu, Ferumoxytol of ultrahigh magnetization produced by hydrocooling and magnetically internal heating co-precipitation, Nanoscale, 2018, 10, 7369–7376 RSC.
- I. Nkurikiyimfura, Y. Wang, B. Safari and E. Nshingabigwi, Temperature-dependent magnetic properties of magnetite nanoparticles synthesized via coprecipitation method, J. Alloys Compd., 2020, 846, 156344 CrossRef CAS.
- L. B. de Mello, L. C. Varanda, F. A. Sigoli and I. O. Mazali, Co-precipitation synthesis of (Zn-Mn)-co-doped magnetite nanoparticles and their application in magnetic hyperthermia, J. Alloys Compd., 2019, 779, 698–705 CrossRef CAS.
-
M. Aliofkhazraei, Handbook of Nanoparticles, Springer Cham, Switzerland, 2015 Search PubMed.
- H. Hayashi and Y. Hakuta, Hydrothermal Synthesis of Metal Oxide Nanoparticles in Supercritical Water, Materials, 2010, 3, 3794–3817 CrossRef CAS PubMed.
- S. Takami, T. Sato, T. Mousavand, S. Ohara, M. Umetsu and T. Adschiri, Hydrothermal synthesis of surface-modified iron oxide nanoparticles, Mater. Lett., 2007, 61, 4769–4772 CrossRef CAS.
-
M. O'Donoghue, A Guide to Man-Made Gemstones, Van Nostrand Reinhold, New York, 1983 Search PubMed.
- D. A. Brewster, D. J. Sarappa and K. E. Knowles, Role of aliphatic ligands and solvent composition in the solvothermal synthesis of iron oxide nanocrystals, Polyhedron, 2019, 157, 54–62 CrossRef CAS.
- O. S. Hammond, R. S. Atri, D. T. Bowron, L. De Campo, S. Diaz-Moreno, L. L. Keenan, J. Doutch, S. Eslava and K. J. Edler, Structural evolution of iron forming iron oxide in a deep eutectic-solvothermal reaction, Nanoscale, 2021, 13, 1723 RSC.
- J. González-Rivera, A. Spepi, C. Ferrari, J. Tovar-Rodriguez, E. Fantechi, F. Pineider, M. A. Vera-Ramírez, M. R. Tiné and C. Duce, Magnetothermally-responsive nanocarriers using confined phosphorylated halloysite nanoreactor for in situ iron oxide nanoparticle synthesis: A MW-assisted solvothermal approach, Colloids Surf., A, 2022, 635, 128116 CrossRef.
- Y.-Y. Zheng, Q. Sun, Y.-H. Duan, J. Zhai, L.-L. Zhang and J.-X. Wang, Controllable synthesis of monodispersed iron oxide nanoparticles by an oxidation-precipitation combined with solvothermal process, Mater. Chem. Phys., 2020, 252, 123431 CrossRef CAS.
- D. Gusain, O. O. Awolusi and F. Bux, Synthesis and characterization of iron oxide/MIL-101 composite via microwave solvothermal treatment, Surf. Sci., 2022, 716, 121952 CrossRef CAS.
- L. Besra and M. Liu, A review on fundamentals and applications of electrophoretic deposition (EPD), Prog. Mater. Sci., 2007, 52, 1–6 CrossRef CAS.
- H. Kang, Y. Park, Y. K. Hong, S. Yoon, M. H. Lee and D. H. Ha, Solvent-induced charge formation and electrophoretic deposition of colloidal iron oxide nanoparticles, Surf. Interfaces, 2021, 22, 100815 CrossRef CAS.
- S. C. Mills, C. S. Smith, D. P. Arnold and J. S. Andrew, Electrophoretic deposition of iron oxide nanoparticles to achieve thick nickel/iron oxide magnetic nanocomposite films, AIP Adv., 2020, 10, 15308 CrossRef CAS.
- S. Singh, G. Singh and N. Bala, Characterization, electrochemical behavior and in vitro hemocompatibility of hydroxyapatite-bioglass-iron oxide-chitosan composite coating by electrophoretic deposition, Surf. Coat. Technol., 2021, 405, 126564 CrossRef CAS.
-
N. Ugemuge, Y. R. Parauha and S. J. Dhoble, in Energy Materials: Fundamentals to Applications, ed. S. J. Dhoble, N. T. Kalyani, B. Vengadaesvaran and A. K. Arof, Elsevier, first edition, 2021, ch. 15, pp. 445–480 Search PubMed.
- Q. Wu, L. He, Z. W. Jiang, Y. Li, Z. M. Cao, C. Z. Huang and Y. F. Li, CuO nanoparticles derived from metal-organic gel with excellent electrocatalytic and peroxidase-mimicking activities for glucose and cholesterol detection, Biosens. Bioelectron., 2019, 145, 111704 CrossRef CAS PubMed.
- R. Rasheed, H. Kareem and H. Mansoor, Preparation and Characterization of Hematite Iron Oxide (α-Fe2O3) by Sol-Gel Method, Chem. Sci. J., 2018, 9, 1000197 Search PubMed.
- S. Yasnur, P. Maji, A. Ray, H. Mullick and S. Das, Effect of annealing temperature on dielectric properties of iron oxide prepared by sol–gel auto combustion method, Ferroelectrics, 2021, 577, 38–51 CrossRef CAS.
- D. Bokov, A. T. Jalil, S. Chupradit, W. Suksatan, M. J. Ansari, I. H. Shewael, G. H. Valiev and E. Kianfar, Nanomaterial by Sol-Gel Method: Synthesis and Application, Adv. Mater. Sci. Eng., 2021, 2021, 1–21 CrossRef.
- A. Akbar, H. Yousaf, S. Riaz and S. Naseem, Role of precursor to solvent ratio in tuning the magnetization of iron oxide thin films – A sol-gel approach, J. Magn. Magn. Mater., 2019, 471, 14–24 CrossRef CAS.
- S. Khalid, S. Riaz, S. Naeem, A. Akbar, S. S. Hussain, Y. B. Xu and S. Naseem, Spin polarization and magneto-dielectric coupling in Al-modified thin iron oxide films-microwave mediated sol-gel approach, J. Ind. Eng. Chem., 2021, 103, 49–66 CrossRef CAS.
- J. López-Sánchez, A. Serrano, A. Del Campo, M. Abuín, E. Salas-Colera, A. Muñoz-Noval, G. R. Castro, J. De La Figuera, J. F. Marco, P. Marín, N. Carmonaag and O. Rodríguez de la Fuente, Self-assembly of iron oxide precursor micelles driven by magnetic stirring time in sol–gel coatings, RSC Adv., 2019, 9, 17571–17580 RSC.
- Y. L. Liu, Y. T. Li, J. F. Huang, Y. L. Zhang, Z. H. Ruan, T. Hu, J. J. Wang, W. Y. Li, H. J. Hu and G. B. Jiang, An advanced sol–gel strategy for enhancing interfacial reactivity of iron oxide nanoparticles on rosin biochar substrate to remove Cr(VI), Sci. Total Environ., 2019, 690, 438–446 CrossRef CAS PubMed.
- G. P. Ricci, L. O. Garcia, E. J. Nassar, S. Nakagaki, J. F. Stival, Z. Novaes da Rocha, M. A. Vicente, R. Trujillano, A. Jiménez, V. Rives, L. Marçal, E. Henrique de Faria and K. J. Ciuffi, Non-hydrolytic sol-gel synthesis of mesoporous iron-aluminum oxide and their properties in the oxidation of hydrocarbons by hydrogen peroxide, Microporous Mesoporous Mater., 2021, 325, 111317 CrossRef CAS.
- G. A. Marcelo, C. Lodeiro, J. L. Capelo, J. Lorenzo and E. Oliveira, Magnetic, fluorescent and hybrid nanoparticles: From synthesis to application in biosystems, Mater. Sci. Eng., C, 2020, 106, 110104 CrossRef CAS PubMed.
- A. Erwin, S. Salomo, P. Adhy, N. Utari, W. Ayu, Y. Wita and S. Nani, Magnetic iron oxide particles (Fe3O4) fabricated by ball milling for improving the environmental quality, IOP Conf. Ser.: Mater. Sci. Eng., 2020, 845, 012051 CAS.
- H. S. Woon, L. S. Ewe, K. P. Lim and I. Ismail, Synthesis and Characterization of Iron Oxide Waste-Derived Red Colour Pigment via Cryo-Milling Process, Asian J. Appl. Sci., 2020, 1, 22–28 Search PubMed.
- A. Younes, N. Dilmi and A. Bouamer, Effect of zinc oxide and alumina nanoparticles on Structural, magnetic and mechanical properties of the iron matrix synthesized by mechanical milling and thermal spraying, Mater. Today: Proc., 2021, 42, 2990–2995 CAS.
- S. Liu, B. Yu, S. Wang, Y. Shen and H. Cong, Preparation, surface functionalization and application of Fe3O4 magnetic nanoparticles, Adv. Colloid Interface Sci., 2020, 281, 102165 CrossRef CAS PubMed.
- P. G. Jamkhande, N. W. Ghule, A. H. Bamer and M. G. Kalaskar, Metal nanoparticles synthesis: An overview on methods of preparation, advantages and disadvantages, and applications, J. Drug Delivery Sci. Technol., 2019, 53, 101174 CrossRef CAS.
- X. Fu, J. Cai, X. Zhang, W.-D. Li, H. Ge and Y. Hu, Top-down fabrication of shape-controlled, monodisperse nanoparticles for biomedical applications, Adv. Drug Delivery Rev., 2018, 132, 169–187 CrossRef CAS PubMed.
-
T. Cele, in Engineered Nanomaterials-Health and Safety,ed. S. M. Avramescu, K. Akhtar, I. Fierascu, S. B. Khan, F. Ali and A. M. Asiri, IntchOpen, London, 2020, ch. 2, pp. 15–28 Search PubMed.
- V. Amendola, P. Riello and M. Meneghetti, Magnetic Nanoparticles of Iron Carbide, Iron Oxide, Iron@Iron Oxide, and Metal Iron Synthesized by Laser Ablation in Organic Solvents, J. Phys. Chem. C, 2011, 115, 5140–5146 CrossRef CAS.
- X. Luo, A. H. M. Al-Antaki, T. M. D. Alharbi, W. D. Hutchison, Y.-C. Zou, J. Zou, A. Sheehan, W. Zhang and C. L. Raston, Laser-Ablated Vortex Fluidic-Mediated Synthesis of Superparamagnetic Magnetite Nanoparticles in Water Under Flow, ACS Omega, 2018, 3, 11172–11178 CrossRef CAS PubMed.
- M. James, R. A. Revia, Z. Stephen and M. Zhang, Microfluidic Synthesis of Iron Oxide Nanoparticles, Nanomaterials, 2020, 10, 2113 CrossRef CAS PubMed.
- R. Chandra, A. K. Chawla and P. Ayyub, Optical and structural properties of sputter-deposited nanocrystalline Cu2O films: effect of sputtering gas, J. Nanosci. Nanotechnol., 2006, 6, 1119–1123 CrossRef CAS PubMed.
- Q. Chen, H. Wang, S. Perero, Q. Wang, Q. Chen and Q. Structural, optical and magnetic properties of Fe3O4 sputtered TeO2–PbO–B2O3 and PbO–Bi2O3–B2O3 glasses for sensing applications, J. Non-Cryst. Solids, 2015, 408, 43–50 CrossRef CAS.
- P. Nie, G. Xu, J. Jiang, H. Dou, Y. Wu, Y. Zhang, J.-F. Wang, M. Shi, R. Fu and X. Zhang, Aerosol-Spray Pyrolysis toward Preparation of Nanostructured Materials for Batteries and Supercapacitors, Small Methods, 2018, 2, 1700272 CrossRef.
- A.-G. Niculescu, C. Chircov and A. M. Grumezescu, Magnetite nanoparticles: Synthesis methods – A comparative review, Methods, 2022, 199, 16–27 CrossRef CAS PubMed.
-
T. V. Gavrilović, D. J. Jovanović and M. D. Dramićanin, in Nanomaterials for Green Energy: A Volume of Micro and Nano Technologies, ed. B. A. Bhanvase, V. B. Pawade, S. J. Dhoble, S. H. Sonawane and M. Ashokkumar, Elsevier, 2018, ch. 2, pp. 55–81 Search PubMed.
-
L. T. Govindaraman, A. Arjunan, A. Baroutaji, J. Robinson, M. Ramadan and A. G. Olabi, Nanomaterials Theory and Applications, Encyclopedia of Smart Materials, 2022, vol. 3, pp. 302–314 Search PubMed.
-
S. K. Das, S. Chakraborty, S. Naskar and R. Rajabalaya, in Bionanocomposites in Tissue Engineering and Regenerative Medicine: A Volume in Woodhead Publishing Series in Biomaterials, ed. S. Ahmed and Annu, Woodhead Publishing, 2021, ch. 3, pp. 17–43 Search PubMed.
- Z. Ferdous, M. S. Ullah and S. Hussain, Temperature and Substrate Effects on the Structural, Morphological, and Optical Properties of Iron Oxide Thin Films Prepared by Spray Pyrolysis Technique, Dhaka Univ. J. Sci., 2020, 68, 79–85 CrossRef.
- A. S. Hassanien and A. A. Akl, Optical characteristics of iron oxide thin films prepared by spray pyrolysis technique at different substrate temperatures, Appl. Phys. A, 2018, 124, 752 CrossRef CAS.
- R. Tischendorf, M. Simmler, C. Weinberger, M. Bieber, M. Reddemann, F. Fröde, J. Lindner, H. Pitsch, R. Kneer, M. Tiemann, H. Nirschl and H.-J. Schmid, Examination of the evolution of iron oxide nanoparticles in flame spray pyrolysis by tailored in situ particle sampling techniques, J. Aerosol Sci., 2021, 154, 105722 CrossRef CAS.
- J. Zheng, K. Liu, W. Cai, L. Qiao, Y. Ying, W. Li, J. Yu, M. Lin and S. Che, Effect of chloride ion on crystalline phase transition of iron oxide produced by ultrasonic spray pyrolysis, Adv. Powder Technol., 2018, 29, 1953–1959 CrossRef CAS.
- A. T. Hassan and E. S. Hassan, Effect of Thickness on Crystal Structure and Dispersion Parameters of Haematite Iron Oxide by Spray Pyrolysis Technique, Acta Phys. Pol., A, 2021, 140, 295–298 CrossRef CAS.
- J. Xie, Y. Guo, S. Lou, Z. Wei, P. Hao, F. Lei and B. Tang, A molten-salt protected pyrolysis approach for fabricating a ternary nickel-cobalt-iron oxide nanomesh catalyst with promoted oxygen-evolving performance, Chem. Commun., 2020, 56, 4579–4582 RSC.
- M. F. B. Stodt, C. Liu, S. Li, L. Mädler, U. Fritsching and J. Kiefer, Phase-selective laser-induced breakdown spectroscopy in flame spray pyrolysis for iron oxide nanoparticle synthesis, Proc. Combust. Inst., 2021, 38, 1711–1718 CrossRef CAS.
- N. Priya, K. Kaur and A. K. Sidhu, Green Synthesis: An Eco-friendly Route for the Synthesis of Iron Oxide Nanoparticles, Frontal Nanotechnol., 2021, 3, 655062 CrossRef.
- S. Perveen, R. Nadeem, S. Rehman, N. Afzal, S. Anjum, S. Noreen, R. Saeed, M. Amami, M. S. H. Al-Mijalli and M. Iqbal, Green synthesis of iron (Fe) nanoparticles using Plumeria obtusa extract as a reducing and stabilizing agent: Antimicrobial, antioxidant and biocompatibility studies, Arabian J. Chem., 2022, 15, 103764 CrossRef CAS.
- N. A. Zakariya, S. Majeed and W. H. W. Jusof, Investigation of antioxidant and antibacterial activity of iron oxide nanoparticles (IONPS) synthesized from the aqueous extract of Penicillium spp, Sens. Int., 2022, 3, 100164 CrossRef.
- M. S. Samuel, S. Datta, N. Chandrasekar, R. Balaji, E. Selvarajan and S. Vuppala, Biogenic Synthesis of Iron Oxide Nanoparticles Using Enterococcus faecalis: Adsorption of Hexavalent Chromium from Aqueous Solution and In Vitro Cytotoxicity Analysis, Nanomaterials, 2021, 11, 3290 CrossRef CAS PubMed.
- S. M. Shalaby, F. F. Madkour, H. Y. El-Kassas, A. A. Mohamed and A. M. Elgarahy, Green synthesis of recyclable iron oxide nanoparticles using Spirulina platensis microalgae for adsorptive removal of cationic and anionic dyes, Environ. Sci. Pollut. Res., 2021, 28, 65549–65572 CrossRef CAS PubMed.
- M. Noruzi, Biosynthesis of gold nanoparticles using plant extracts, Bioprocess Biosyst. Eng., 2015, 38, 1–14 CrossRef CAS PubMed.
- A. K. Mittal, Y. Chisti and U. C. Banerjee, Synthesis of metallic nanoparticles using plant extracts, Biotechnol. Adv., 2013, 31, 346–356 CrossRef CAS PubMed.
- S. Roy and T. K. Das, Plant Mediated Green Synthesis of Silver Nanoparticles-A Review, Int. J. Plant Biol., 2015, 3, 1044–1055 Search PubMed.
- H. Agarwal, S. V. Kumar and S. Rajeshkumar, A review on green synthesis of zinc oxide nanoparticles-An eco-friendly approach, Resour.-Effic. Technol., 2017, 3, 406–413 Search PubMed.
- A. U. Mirza, A. Kareem, S. A. A. Nami, M. S. Khan, S. Rehman, S. A. Bhat, A. Mohammad and N. Nishat, Biogenic synthesis of iron oxide nanoparticles using Agrewia optiva and Prunus persica phyto species: Characterization, antibacterial and antioxidant activity, J. Photochem. Photobiol. B, Biol., 2018, 185, 262–274 CrossRef CAS PubMed.
- M. Nadeem, D. Tungmunnithum, C. Hano, B. H. Abbasi, S. S. Hashmi, W. Ahmad and A. Zahir, The current trends in the green syntheses of titanium oxide nanoparticles and their applications, Green Chem. Lett. Rev., 2018, 11, 492–502 CrossRef CAS.
- S. Vasantharaj, S. Sathiyavimal, P. Senthilkumar, F. LewisOscar and A. Pugazhendhi, Biosynthesis of iron oxide nanoparticles using leaf extract of Ruellia tuberosa: Antimicrobial properties and their applications in photocatalytic degradation, J. Photochem. Photobiol. B, Biol., 2019, 192, 74–82 CrossRef CAS PubMed.
- P. Mondal, A. Anweshan and M. K. Purkait, Green synthesis and environmental application of iron-based nanomaterials and nanocomposite: A review, Chemosphere, 2020, 259, 127509 CrossRef CAS PubMed.
- K. Kaur and A. K. Sidhu, Green synthesis: An eco-friendly route for the synthesis of iron oxide nanoparticles, Frontal Nanotechnol., 2021, 3, 655062 CrossRef.
- S. A. Razack, A. Suresh, S. Sriram, G. Ramakrishnan, S. Sadanandham, M. Veerasamy, R. B. Nagalamadaka and R. Sahadevan, Green synthesis of iron oxide nanoparticles using Hibiscus rosa-sinensis for fortifying wheat biscuits, SN Appl. Sci., 2020, 2, 898 CrossRef.
- M. S. H. Bhuiyan, M. Y. Miah, S. C. Paul, T. D. Aka, O. Saha, M. M. Rahaman, M. J. I. Sharif, O. Habiba and M. Ashaduzzaman, Green synthesis of iron oxide nanoparticle using Carica papaya leaf extract: application for photocatalytic degradation of remazol yellow RR dye and antibacterial activity, Heliyon, 2020, 6, e04603 CrossRef PubMed.
- N. Madubuonu, S. O. Aisida, I. Ahmad, S. Botha, T.-K. Zhao, M. Maaza and F. I. Ezema, Bio-inspired iron oxide nanoparticles using Psidium guajava aqueous extract for antibacterial activity, Appl. Phys. A, 2020, 126, 1–8 CrossRef.
- R. Hooda and M. Sharma, Green synthesis, characterization and antibacterial activity of iron oxide nanoparticles, Plant Arch., 2020, 20, 1196–1200 Search PubMed.
- A. S. Y. Ting and J. E. Chin, Biogenic Synthesis of Iron Nanoparticles from Apple Peel Extracts for Decolorization of Malachite Green Dye, Water, Air, Soil Pollut., 2020, 231, 278 CrossRef CAS.
- B. Kumar, K. Smita, S. Galeas, V. Sharma, V. H. Guerrero, A. Debut and L. Cumbal, Characterization and application of biosynthesized iron oxide nanoparticles using Citrus paradisi peel: A sustainable approach, Inorg. Chem. Commun., 2020, 119, 108116 CrossRef CAS.
- M. A. Asghar, E. Zahir, M. A. Asghar, J. Iqbal and A. A. Rehman, Facile, one-pot biosynthesis and characterization of iron, copper and silver nanoparticles using Syzygium cumini leaf extract: as an effective antimicrobial and aflatoxin B1 adsorption agents, PLoS One, 2020, 15, e0234964 CrossRef CAS PubMed.
- Z. Izadiyan, K. Shameli, M. Miyake, H. Hara, S. E. B. Mohamad, K. Kalantari, S. H. M. Taib and E. Rasouli, Cytotoxicity assay of plant-mediated synthesized iron oxide nanoparticles using Juglans regia green husk extract, Arabian J. Chem., 2020, 13, 2011–2023 CrossRef CAS.
- K. Rong, J. Wang, Z. Zhang and J. Zhang, Green synthesis of iron nanoparticles using Korla fragrant pear peel extracts for the removal of aqueous Cr(VI), Ecol. Eng., 2020, 149, 105793 CrossRef.
- D. Patiño-Ruiz, L. Sánchez-Botero, L. Tejeda-Benitez, J. Hinestroza and A. Herrera, Green synthesis of iron oxide nanoparticles using Cymbopogon citratus extract and sodium carbonate salt: Nanotoxicological considerations for potential environmental applications, Environ. Nanotechnol., Monit. Manage., 2020, 14, 100377 Search PubMed.
- M. Jamzad and M. Kamari Bidkorpeh, Green synthesis of iron oxide nanoparticles by the aqueous extract of Laurus nobilis L. leaves and evaluation of the antimicrobial activity, J. Nanostructure Chem., 2020, 10, 193–201 CrossRef CAS.
- H. E. A. Mohamed, S. Afridi, A. T. Khalil, M. Ali, T. Zohra, M. Salman, A. Ikram, Z. K. Shinwari and M. Maaza, Bio-redox potential of Hyphaene thebaica in bio-fabrication of ultrafine maghemite phase iron oxide nanoparticles (Fe2O3 NPs) for therapeutic applications, Mater. Sci. Eng., C, 2020, 112, 110890 CrossRef CAS PubMed.
- D. Bharathi, S. Preethi, K. Abarna, M. Nithyasri, P. Kishore and K. Deepika, Bio-inspired synthesis of flower shaped iron oxide nanoparticles (FeONPs) using phytochemicals of Solanum lycopersicum leaf extract for biomedical applications, Biocatal. Agric. Biotechnol., 2020, 27, 101698 CrossRef.
- C. Noorjahan, Photosynthesis, Characterization and Antimicrobial Activity of Iron Oxide Nanoparticle using Henna (Lawsonia Inermis), Int. J. Eng. Sci., 2020, 7, 38–45 Search PubMed.
- N. K. Abbas and A. A. Al-Attraqchi, Antimicrobial Activities of Green Biosynthesized Iron Oxide Nanoparticles Using F. Carica Fruit Extract, Indian J. Forensic Med. Toxicol., 2020, 14, 2181–2187 Search PubMed.
- B. A. Abbasi, J. Iqbal, S. A. Zahra, A. Shahbaz, S. Kanwal, A. Rabbani and T. Mahmood, Bioinspired synthesis and activity characterization of iron oxide nanoparticles made using Rhamnus Triquetra leaf extract, Mater. Res. Express, 2020, 6, 1250e1257 Search PubMed.
- M. A. Abid, D. A. Kadhim and W. J. Aziz, Iron oxide nanoparticle synthesis using trigonella and tomato extracts and their antibacterial activity, Mater. Technol., 2022, 37, 547–554 CrossRef CAS.
- W. Aziz, M. Abid, D. Kadhim and M. Mejbel, Synthesis of iron oxide (β-Fe2O3) nanoparticles from Iraqi grapes extract and its biomedical application, IOP Conf. Ser.: Mater. Sci. Eng., 2020, 881, 1–9 Search PubMed.
- S. O. Aisida, N. Madubuonu, M. H. Alnasir, I. Ahmad, S. Botha, M. Maaza and F. I. Ezema, Biogenic synthesis of iron oxide nanorods using Moringa oleifera leaf extract for antibacterial applications, Appl. Nanosci., 2020, 10, 305–315 CrossRef CAS.
- S. Qasim, A. Zafar, M. S. Saif, Z. Ali, M. Nazar, M. Waqas, A. U. Haq, T. Tariq, S. G. Hassan, F. Iqbal, X.-G. Shu and M. Hasan, Green synthesis of iron oxide nanorods using Withania coagulans extract improved photocatalytic degradation and antimicrobial activity, J. Photochem. Photobiol. B, Biol., 2020, 204, 111784 CrossRef CAS PubMed.
- F. Farouk, M. Abdelmageed, M. Azam Ansari and H. M. E. Azzazy, Synthesis of magnetic iron oxide nanoparticles using pulp and seed aqueous extract of Citrullus colocynth and evaluation of their antimicrobial activity, Biotechnol. Lett., 2020, 42, 231–240 CrossRef CAS PubMed.
- L. P. Yan, S. C. B. Gopinath, P. Anbu, F. H. Kasim, H. I. Zulhaimi and A. R. W. Yaakub, Characterization and anti-bacterial potential of iron oxide nanoparticle processed eco-friendly by plant extract, Prep. Biochem. Biotechnol., 2020, 50, 1053–1062 CrossRef CAS PubMed.
- J. Sandhya and S. Kalaiselvam, Biogenic synthesis of magnetic iron oxide nanoparticles using inedible borassus flabellifer seed coat: characterization, antimicrobial, antioxidant activity and in vitro cytotoxicity analysis, Mater. Res. Express, 2020, 7, 015045 CrossRef CAS.
- M. Bashir, S. Ali and M. A. Farrukh, Green Synthesis of Fe2O3 Nanoparticles from Orange Peel Extract and a Study of Its Antibacterial Activity, J. Korean Phys. Soc., 2020, 76, 848–854 CrossRef CAS.
- F. Erci and R. Cakir-Koc, Rapid green synthesis of noncytotoxic iron oxide nanoparticles using aqueous leaf extract of Thymbra spicata and evaluation of their antibacterial, antibiofilm, and antioxidant activity, Inorg. Nano-Met. Chem., 2021, 51, 683–692 CrossRef CAS.
- A. Rajendran, M. Alsawalha and T. Alomayri, Biogenic synthesis of husked rice-shaped iron oxide nanoparticles using coconut pulp (Cocos nucifera L.) extract for photocatalytic degradation of Rhodamine B dye and their in vitro antibacterial and anticancer activity, J. Saudi Chem. Soc., 2021, 25, 101307 CrossRef CAS.
- W. Ahmad, K. Kumar Jaiswal and M. Amjad, Euphorbia herita leaf extract as a reducing agent in a facile green synthesis of iron oxide nanoparticles and antimicrobial activity evaluation, Inorg. Nano-Met. Chem., 2021, 51, 1147–1154 CrossRef CAS PubMed.
- R. Periakaruppan, X. Chen, K. Thangaraj, A. Jeyaraj, H. H. Nguyen, Y. Yu, S. Hu, L. Lu and X. Li, Utilization of tea resources with the production of superparamagnetic biogenic iron oxide nanoparticles and an assessment of their antioxidant activities, J. Cleaner Prod., 2021, 278, 123962 CrossRef CAS.
-
A. Jalal and N. Fakhre, Preparation and Characterization of Green Fe3O4 Nanoparticle Using the Aqueous Plant Extract of Gundelia Tournefortii L, Aro-The Scientific Journal of Koya University, 2021, vol. 9, pp. 58–63 Search PubMed.
- M. Sriramulu, Balaji and S. Sumathi, Photo Catalytic, Antimicrobial and Antifungal Activity of Biogenic Iron Oxide Nanoparticles Synthesised Using Aegle marmelos Extracts, J. Inorg. Organomet. Polym., 2021, 31, 1738–1744 CrossRef CAS.
- M. W. Yaseen, M. Sufyan, R. Nazir, A. Naseem, R. Shah, A. A. Sheikh and M. Iqbal, Simple and cost-effective approach to synthesis of iron magnesium oxide nanoparticles using Alstonia scholaris and Polyalthia longifolia leaves extracts and their antimicrobial, antioxidant and larvicidal activities, Appl. Nanosci., 2021, 11, 2479–2488 CrossRef CAS.
- Y. P. Teoh, Z. X. Ooi, S. S. Leong, P. T. Ng and W. W. Liu, Green Synthesis of Iron Oxide Nanoparticle Using Coffee Seed Extract and Its Antibacterial Activity, J. Eng. Sci., 2021, 17, 19–29 CrossRef.
- Ö. Erdoğan, S. Paşa, G. M. Demirbolat and O. Çevik, Green biosynthesis, characterization, and cytotoxic effect of magnetic iron nanoparticles using Brassica Oleracea var capitata sub var rubra (red cabbage) aqueous peel extract, Turk. J. Chem., 2021, 45, 1086–1096 CrossRef PubMed.
- P. N. Kirdat, P. B. Dandge, R. M. Hagwane, A. S. Nikam, S. P. Mahadik and S. T. Jirange, Synthesis and characterization of ginger (Z. officinale) extract mediated iron oxide nanoparticles and its antibacterial activity, Mater. Today: Proc., 2021, 43, 2826–2831 CAS.
- A. Bouafia, S. E. Laouini, A. Khelef, M. L. Tedjani and F. Guemari, Effect of Ferric Chloride Concentration on the Type of Magnetite (Fe3O4) Nanoparticles Biosynthesized by Aqueous Leaves Extract of Artemisia and Assessment of Their Antioxidant Activities, J. Cluster Sci., 2021, 32, 1033–1041 CrossRef CAS.
- M. Yusefi, K. Shameli, O. S. Yee, S.-Y. Teow, Z. Hedayatnasab, H. Jahangirian, T. J. Webster and K. Kuča, Green synthesis of Fe3O4 nanoparticles stabilized by a Garcinia mangostana fruit peel extract for hyperthermia and anticancer activities, Int. J. Nanomed., 2021, 16, 2515 CrossRef PubMed.
- L. Shaker Ardakani, V. Alimardani, A. M. Tamaddon, A. M. Amani and S. Taghizadeh, Green synthesis of iron-based nanoparticles using Chlorophytum comosum leaf extract: methyl orange dye degradation and antimicrobial properties, Heliyon, 2021, 7, e06159 CrossRef CAS PubMed.
- A. Biswas, C. Vanlalveni, R. Lalfakzuala, S. Nath and L. Rokhum, Mikania mikrantha leaf extract mediated biogenic synthesis of magnetic iron oxide nanoparticles: Characterization and its antimicrobial activity study, Mater. Today: Proc., 2021, 42, 1366–1373 CAS.
- M. A. Abid, D. A. Abid, W. J. Aziz and T. M. Rashid, Iron oxide nanoparticles synthesized using garlic and onion peel extracts rapidly degrade methylene blue dye, Physica B Condens. Matter., 2021, 622, 413277 CrossRef CAS.
- E. Üstün, S. C. Önbaş, S. K. Çelik, M. C. Ayvaz and N. Şahin, Green synthesis of iron oxide nanoparticles by using Ficus carica leaf extract and its antioxidant activity, Biointerface Res. Appl. Chem., 2022, 2021, 2108–2116 Search PubMed.
- K. Velsankar, G. Parvathy, S. Mohandoss, M. Krishna Kumar and S. Sudhahar, Celosia argentea leaf extract-mediated green synthesized iron oxide nanoparticles for bio-applications, J. Nanostructure Chem., 2022, 12, 625–640 CrossRef CAS.
- M. S. Haydar, D. Das, S. Ghosh and P. Mandal, Implementation of mature tea leaves extract in bioinspired synthesis of iron oxide nanoparticles: preparation, process optimization, characterization, and assessment of therapeutic potential, Chem. Pap., 2022, 76, 491–514 CrossRef CAS.
- S. Abd Al-hameed and A. M. Mohammed, Novel green synthesis of Fe2O3 nanoparticles using persimmon extract and study their anti-cancer and anti-bacterial activity, J. Pharm. Negat. Results, 2022, 13, 958–967 Search PubMed.
- D. Mundekkad and A. V. Alex, Analysis of structural and biomimetic characteristics of the green-synthesized Fe3O4 nanozyme from the fruit peel extract of Punica granatum, Chem. Pap., 2022, 76, 3863–3878 CrossRef CAS.
- F. A. Al-Zahrani, S. S. Salem, H. A. Al-Ghamdi, L. M. Nhari, L. Lin and R. M. El-Shishtawy, Green synthesis and antibacterial activity of Ag/Fe2O3 nanocomposite using Buddleja lindleyana extract, Bioengineering, 2022, 9, 452 CrossRef CAS PubMed.
- F. Buarki, H. AbuHassan, F. Al Hannan and F. Z. Henari, Green Synthesis of Iron Oxide Nanoparticles Using Hibiscus rosa sinensis Flowers and Their Antibacterial Activity, J. Nanotechnol., 2022, 2022, 5474645 Search PubMed.
- S. Kumar, S. Ashique, H. Kumar, S. Verma, K. Dutt, A. Goswami, S. Pal, D. S. Chopra, Sonali, A. Iqbal and I. Kayes, Green Synthesis and Evaluation of Quercetin Conjugated Superparamagnetic Iron Oxide Nanoparticles, J. Harbin Inst. Technol., 2022, 54, 53–61 Search PubMed.
- J. Xiu, Y. Zhang, B. A. Paray, A. Gulnaz and M. War, Facile preparation of Fe2O3 nanoparticles mediated by Centaurea alba extract and assessment of the anti-atherosclerotic properties, Arabian J. Chem., 2022, 15, 103493 CrossRef CAS.
- Y. Shah, M. Maharana and S. Sen, Peltophorum pterocarpum leaf extract mediated green synthesis of novel iron oxide particles for application in photocatalytic and catalytic removal of organic pollutants, Biomass Convers. Biorefin., 2022, 1–14 Search PubMed.
- N. Dildar, S. N. Ali, T. Sohail, M. Lateef, S. T. Khan, S. F. Bukhari and P. Fazil, Biosynthesis, Characterization, Radical Scavenging and Antimicrobial Properties of Psidium guajava Linn Coated Silver and Iron Oxide Nanoparticles, Egypt. J. Chem., 2022, 65, 145–151 Search PubMed.
- M. Rizvi, T. Bhatia and R. Gupta, Green & sustainable synthetic route of obtaining iron oxide nanoparticles using Hylocereus undantus (pitaya or dragon fruit), Mater. Today: Proc., 2022, 50, 1100–1106 CAS.
- H. Al-Karagoly, A. Rhyaf, H. Naji, S. Albukhaty, F. A. AlMalki, A. A. Alyamani, J. Albaqami and S. Aloufi, Green synthesis, characterization, cytotoxicity, and antimicrobial activity of iron oxide nanoparticles using Nigella sativa seed extract, Green Process. Synth., 2022, 11, 254–265 CrossRef CAS.
- S. Khan, G. Bibi, S. Dilbar, A. Iqbal, M. Ahmad, A. Ali, Z. Ullah, M. Jaremko, J. Iqbal and M. Ali, Biosynthesis and characterization of iron oxide nanoparticles from Mentha spicata and screening its combating potential against Phytophthora infestans, Front. Plant Sci., 2022, 13, 1001499 CrossRef PubMed.
- C. Sudhakar, M. Poonkothai, T. Selvankumar and K. Selvam, Facile synthesis of iron oxide nanoparticles using Cassia auriculata flower extract and accessing their photocatalytic degradation and larvicidal effect, J. Mater. Sci. Mater. Electron., 2022, 33, 11434–11445 CrossRef CAS.
- W. Muzafar, T. Kanwal, K. Rehman, S. Perveen, T. Jabri, F. Qamar, S. Faizi and M. R. Shah, Green synthesis of iron oxide nanoparticles using Melia azedarach flowers extract and evaluation of their antimicrobial and antioxidant activities, J. Mol. Struct., 2022, 1269, 133824 CrossRef CAS.
- K. Velsankar, G. Parvathy, S. Mohandoss, G. Ravi and S. Sudhahar, Echinochloa frumentacea grains extract mediated synthesis and characterization of iron oxide nanoparticles: A greener nano drug for potential biomedical applications, J. Drug Delivery Sci. Technol., 2022, 76, 103799 CrossRef.
- R. R. Pillai, P. B. Sreelekshmi, A. P. Meera and S. Thomas, Biosynthesized iron oxide nanoparticles: Cytotoxic evaluation against human colorectal cancer cell lines, Mater. Today: Proc., 2022, 50, 187–195 CAS.
- C. Harmansah, M. Karatay Kutman and F. Z. Biber Muftuler, Preparation of iron oxide nanoparticles by banana peels extract and its usage in NDT, Measurement, 2022, 204, 112081 CrossRef.
- I. Ashraf, N. B. Singh and A. Agarwal, Green synthesis of iron oxide nanoparticles using Amla seed for methylene blue dye removal from water, Mater. Today: Proc., 2023, 72, 311–316 CAS.
- Z. Isik, R. Bouchareb, H. Arslan, S. Özdemir, S. Gonca, N. Dizge, D. Balakrishnan and S. V. S. Prasad, Green synthesis of iron oxide nanoparticles derived from water and methanol extract of Centaurea solstitialis leaves and tested for antimicrobial activity and dye decolorization capability, Environ. Res., 2023, 219, 115072 CrossRef CAS PubMed.
- K. Andrade-Zavaleta, Y. Chacon-Laiza, D. Asmat-Campos and N. Raquel-Checca, Green Synthesis of Superparamagnetic Iron Oxide Nanoparticles with Eucalyptus globulus Extract and Their Application in the Removal of Heavy Metals from Agricultural Soil, Molecules, 2022, 27, 1367 CrossRef CAS PubMed.
- N. Hoffmann, G. Tortella, E. Hermosilla, P. Fincheira, M. C. Diez, I. M. Lourenço, A. B. Seabra and O. Rubilar, Comparative Toxicity Assessment of Eco-Friendly Synthesized Superparamagnetic Iron Oxide Nanoparticles (SPIONs) in Plants and Aquatic Model Organisms, Minerals, 2022, 12, 451 CrossRef CAS.
- K. Singh, D. Sethi Chopra, D. Singh and N. Singh, Green synthesis and characterization of iron oxide nanoparticles using Coriandrum sativum L. leaf extract, Indian J. Biochem. Biophys., 2022, 59, 450–454 CAS.
- J. C. Tarafdar and R. Raliya, Rapid, Low-Cost, and Ecofriendly Approach for Iron Nanoparticle Synthesis Using Aspergillus oryzae TFR9, J. Nanopart., 2013, 2013, 141274 Search PubMed.
- M. Guilger-Casagrande and R. D. Lima, Synthesis of Silver Nanoparticles Mediated by Fungi: A Review, Front. Bioeng. Biotechnol., 2019, 7, 287 CrossRef PubMed.
- S. Chatterjee, S. Mahanty, P. Das, P. Chaudhuri and S. Das, Biofabrication of iron oxide nanoparticles using manglicolous fungus Aspergillus niger BSC-1 and removal of Cr(VI) from aqueous solution, Chem. Eng. J., 2020, 385, 123790 CrossRef CAS.
- T. J. Beveridge, M. N. Hughes, H. Lee, K. T. Leung, R. K. Poole, I. Savvaidis, S. Silver and J. T. Trevors, Metal-Microbe Interactions: Contemporary Approaches, Adv. Microb. Physiol., 1997, 38, 177–243 CrossRef CAS PubMed.
- M. Gericke and A. Pinches, Biological synthesis of metal nanoparticles, Hydrometallurgy, 2006, 83, 132–140 CrossRef CAS.
- N. A. Wani, W. I. Khanday and S. Tirumale, Biosynthesis of iron oxide nanoparticles using ethyl acetate extract of Chaetomium cupreum and their anticancer activity, Matrix Sci. Pharma, 2021, 40, 23–30 Search PubMed.
- S. Saqib, W. Zaman, A. Ayaz, S. Habib, S. Bahadur, S. Hussain, S. Muhammad and F. Ullah, Postharvest disease inhibition in fruit by synthesis and characterization of chitosan iron oxide nanoparticles, Biocatal. Agric. Biotechnol., 2020, 28, 101729 CrossRef.
- A. Ali, N. Elewa, N. Elbostany, Y. Shetaia and M. Swilm, Antimicrobial potentiality of green synthesized iron oxide nanoparticles by Penicillium roqueforti, Egypt J. Pure Appl. Sci., 2021, 59, 29–43 CrossRef.
- R. Safarkar, G. Ebrahimzadeh Rajaei and S. Khalili-Arjagi, The study of antibacterial properties of iron oxide nanoparticles synthesized using the extract of lichen Ramalina sinensis, Asian J. Nanosci. Mater., 2020, 3, 157–166 CAS.
- G. Manik and R. Ramasubbu, Biosynthesis of Iron Nanoparticles from Pleurotus florida and its Antimicrobial Activity against Selected Human Pathogens, Indian J. Pharm. Sci., 2021, 83, 45–51 Search PubMed.
- H. Sayed, H. Sadek, M. Abdel-Aziz, N. Mahmoud, W. Sabry, G. Genidy and M. Maher, Biosynthesis of iron oxide nanoparticles from fungi isolated from deteriorated historical gilded cartonnage and its application in cleaning, Egypt J. Archeol. Restor. Stud., 2021, 11, 129–145 Search PubMed.
- N. Pantidos and L. E. Horsfall, Biological synthesis of metallic nanoparticles by bacteria, fungi and plants, J. Nanomed. Nanotechnol., 2014, 5, 1 Search PubMed.
- A. Fariq, T. Khan and A. Yasmin, Microbial synthesis of nanoparticles and their potential applications in biomedicine, J. Appl. Biomed., 2017, 15, 241–248 CrossRef.
- G. Gahlawat and A. R. Choudhury, A review on the biosynthesis of metal and metal salt nanoparticles by microbes, RSC Adv., 2019, 9, 12944–12967 RSC.
- P. Singh, Y.-J. Kim, D. Zhang and D.-C. Yang, Biological synthesis of nanoparticles from plants and microorganisms, Trends Biotechnol., 2016, 34, 588–599 CrossRef CAS PubMed.
- H. Alam, N. Khatoon, M. A. Khan, S. A. Husain, M. Saravanan and M. Sardar, Synthesis of selenium nanoparticles using probiotic bacteria Lactobacillus acidophilus and their enhanced antimicrobial activity against resistant bacteria, J. Cluster Sci., 2020, 31, 1003–1011 CrossRef CAS.
- A. S. Jubran, O. M. Al-Zamely and M. H. Al-Ammar, A study of iron oxide nanoparticles synthesis by using bacteria, Int. J. Pharm. Qual. Assur., 2020, 11, 1–8 CrossRef.
- S. Majeed, M. Danish, M. N. Mohamad Ibrahim, S. H. Sekeri, M. T. Ansari, A. Nanda and G. Ahmad, Bacteria Mediated Synthesis of Iron Oxide Nanoparticles and Their Antibacterial, Antioxidant, Cytocompatibility Properties, J. Cluster Sci., 2021, 32, 1083–1094 CrossRef CAS.
- M. A. Ali and M. H. Alkhafaji, Antibiofilm Activity of Biosynthesized Enterococcus-Iron Oxide Nanoparticles against uropathogenic Bacteria, Egypt. J. Bot., 2023, 63, 1201–1214 Search PubMed.
- Q. A. Al-Maliki and W. R. Taj-Aldeen, Antibacterial Activity Of Bacterial Mediated Synthesized Iron Oxide Nanoparticles Using Bacillus Coagulans, Nat. Volatiles Essent. Oils, 2022, 9, 1312–1320 CAS.
- M. Kumar, G. Gupta, T. Varghese, A. M. Shankregowda, P. P. Srivastava, S. Bhushan, P. P. Shukla, G. Krishna and S. Gupta, Synthesis and characterisation of super-paramagnetic iron oxide nanoparticles (SPIONs) for minimising Aeromonas hydrophila load from freshwater, Curr. Nanosci., 2022, 18, 224–236 CrossRef CAS.
- V. Subramaniyam, S. R. Subashchandrabose, V. Ganeshkumar, P. Thavamani, Z. Chen, R. Naidu and M. Megharaj, Cultivation of Chlorella on brewery wastewater and nano-particle biosynthesis by its biomass, Bioresour. Technol., 2016, 211, 698–703 CrossRef CAS PubMed.
- H. Agarwal, S. V. Kumar and S. Rajeshkumar, A review on green synthesis of zinc oxide nanoparticles–An eco-friendly approach, Resour.-Effic. Technol., 2017, 3, 406–413 Search PubMed.
- K. S. Siddiqi and A. Husen, Fabrication of metal nanoparticles from fungi and metal salts: scope and application, Nanoscale Res. Lett., 2016, 11, 1–15 CrossRef PubMed.
- D. M. Salem, M. M. Ismail and H. R. Tadros, Evaluation of the antibiofilm activity of three seaweed species and their biosynthesized iron oxide nanoparticles (Fe3O4-NPs), Egypt. J. Aquat. Res., 2020, 46, 333–339 CrossRef.
- M. L. Budlayan, J. N. Patricio, S. D. Arco, R. Y. Capangpangan and A. C. Alguno, Effects of precursor concentration on the properties of magnetic iron oxide nanoparticles synthesized using brown seaweed (Sargassum crassifolium) extract, Mater. Today: Proc., 2021, 46, 1608–1612 CAS.
- Z. Peng, Z. Fan, X. Chen, X. Zhou, Z. F. Gao, S. Deng, S. Wan, X. Lv, Y. Shi and W. Han, Fabrication of Nano Iron Oxide–Modified Biochar from Co-Hydrothermal Carbonization of Microalgae and Fe (II) Salt for Efficient Removal of Rhodamine B, Nanomater, 2022, 12, 2271 CrossRef CAS PubMed.
- C. Yu, J. Tang, H. Su, J. Huang, F. Liu, L. Wang and H. Sun, Development of a novel biochar/iron oxide composite from green algae for bisphenol-A removal: Adsorption
and Fenton-like reaction, Environ. Technol. Innov., 2022, 102647 CrossRef CAS.
- G. Sarojini, S. Venkateshbabu and M. Rajasimman, Facile synthesis and characterization of polypyrrole-iron oxide–seaweed (PPy-Fe3O4-SW) nanocomposite and its exploration for adsorptive removal of Pb (II) from heavy metal bearing water, Chemosphere, 2021, 278, 130400 CrossRef CAS PubMed.
- R. Selvaraj, G. Murugesan, G. Rangasamy, R. Bhole, N. Dave, S. Pai, K. Balakrishna, R. Vinayagam and T. Varadavenkatesan, As (III) removal using superparamagnetic magnetite nanoparticles synthesized using Ulva prolifera− optimization, isotherm, kinetic and equilibrium studies, Chemosphere, 2022, 308, 136271 CrossRef CAS PubMed.
- S. O. Aisida, P. A. Akpa, I. Ahmad, M. Maaza and F. I. Ezema, Influence of PVA, PVP and PEG doping on the optical, structural, morphological and magnetic properties of zinc ferrite nanoparticles produced by thermal method, Physica B Condens. Matter, 2019, 571, 130–136 CrossRef CAS.
- D. O. Okoroh, J. Ozuomba, S. O. Aisida and P. U. Asogwa, Thermal treated synthesis and characterization of polyethylene glycol (PEG) mediated zinc ferrite nanoparticles, Surf. Interfaces, 2019, 16, 127–131 CrossRef CAS.
- D. O. Okoroh, J. O. Ozuomba, S. O. Aisida and P. U. Asogwa, Properties of zinc ferrite nanoparticles due to PVP mediation and annealing at 500 °C, Adv. Nanopart., 2019, 8, 36–45 CrossRef CAS.
- M. Chastellain, A. Petri, A. Gupta, K. V. Rao and H. Hofmann, Superparamagnetic silica-iron oxide nanocomposites for application in hyperthermia, Adv. Eng. Mater., 2004, 6, 235–241 CrossRef CAS.
- R. Weissleder, D. D. Stark, B. L. Engelstad, B. R. Bacon, C. C. Compton, D. L. White, P. Jacobs and J. Lewis, Superparamagnetic iron oxide: pharmacokinetics and toxicity, Am. J. Roentgenol., 1989, 152, 167–173 CrossRef CAS PubMed.
- S. M. Dadfar, K. Roemhild, N. I. Drude, S. Von Stillfried, R. Knüchel, F. Kiessling and T. Lammers, Iron oxide nanoparticles: Diagnostic, therapeutic and theranostic applications, Adv. Drug Delivery Rev., 2019, 138, 302–325 CrossRef CAS PubMed.
- R. P. Dhavale, S. B. Parit, S. C. Sahoo, P. Kollu, P. S. Patil, P. B. Patil and A. D. Chougale, α-amylase immobilized on magnetic nanoparticles: reusable robust nano-biocatalyst for starch hydrolysis, Mater. Res. Express, 2018, 5, 075403 CrossRef.
- N. Madubuonu, S. O. Aisida, A. Ali, I. Ahmad, T.-K. Zhao, S. Botha, M. Maaza and F. I. Ezema, Biosynthesis of iron oxide nanoparticles via a composite of Psidium guavaja-Moringa oleifera and their antibacterial and photocatalytic study, J. Photochem. Photobiol. B, Biol., 2019, 199, 111601 CrossRef CAS PubMed.
- Y. Zhang, M. Liu, Y. Zhang, X. Chen, W. Ren and Z.-G. Ye, Atomic layer deposition of superparamagnetic and ferrimagnetic magnetite thin films, J. Appl. Phys., 2015, 117, 17C743 CrossRef.
- M. G. Naseri, E. B. Saion, M. Hashim, A. H. Shaari and H. A. Ahangar, Synthesis and characterization of zinc ferrite nanoparticles by a thermal treatment method, Solid State Commun., 2011, 151, 1031–1035 CrossRef CAS.
- S. Arora, Superparamagnetic iron oxide nanoparticles: magnetic nanoplatforms as drug carriers, Int. J. Nanomed., 2012, 7, 3445 Search PubMed.
- H. Mok and M. Zhang, Superparamagnetic iron oxide nanoparticle-based delivery systems for biotherapeutics, Expert Opin. Drug Delivery, 2013, 10, 73–87 CrossRef CAS PubMed.
-
C. S. Hurlbut and C. Klein, Manual of Mineralogy, ed. J. D. Dana, Wiley, New York, 1993 Search PubMed.
- G. H. Kwei, R. B. Von Dreele, A. Williams, J. A. Goldstone, A. C. Lawson and W. K. Warburton, Structure and valence from complementary anomalous X-ray and neutron powder diffraction, J. Mol. Struct., 1990, 223, 383–406 CrossRef CAS.
- S. Naqvi, M. Samim, A. Abdin, F. J. Ahmed, A. Maitra, C. Prashant and A. K. Dinda, Concentration-dependent toxicity of iron oxide nanoparticles mediated by increased oxidative stress, Int. J. Nanomed., 2010, 5, 983 CrossRef CAS PubMed.
- Z. Sun, V. Yathindranath, M. Worden, J. A. Thliveris, S. Chu, F. E. Parkinson, T. Hegmann and D. W. Miller, Characterization of cellular uptake and toxicity of aminosilane-coated iron oxide nanoparticles with different charges in central nervous system-relevant cell culture models, Int. J. Nanomed., 2013, 8, 961–970 CrossRef PubMed.
- Z. M. Saiyed, N. H. Gandhi and M. P. Nair, Magnetic nanoformulation of azidothymidine 5'-triphosphate for targeted delivery across the blood–brain barrier, Int. J. Nanomed., 2010, 5, 157–166 CAS.
- M. Arruebo, R. Fernández-Pacheco, M. R. Ibarra and J. Santamaría, Magnetic nanoparticles for drug delivery, Nano Today, 2007, 2, 22–32 CrossRef.
- J. Gao, H. Gu and B. Xu, Multifunctional magnetic nanoparticles: design, synthesis, and biomedical applications, Acc. Chem. Res., 2009, 42, 1097–1107 CrossRef CAS PubMed.
- G. Prabha and V. Raj, Preparation and characterization of polymer nanocomposites coated magnetic nanoparticles for drug delivery applications, J. Magn. Magn. Mater., 2016, 408, 26–34 CrossRef CAS.
- J. Xu, J. Sun, Y. Wang, J. Sheng, F. Wang and M. Sun, Application of iron magnetic nanoparticles in protein immobilization, Molecules, 2014, 19, 11465–11486 CrossRef PubMed.
- T. K. Jain, M. K. Reddy, M. A. Morales, D. L. Leslie-Pelecky and V. Labhasetwar, Biodistribution, Clearance, and Biocompatibility of Iron Oxide Magnetic Nanoparticles in Rats, Mol. Pharm., 2008, 5, 316–327 CrossRef CAS PubMed.
- W. Wu, Q. He and C. Jiang, Magnetic Iron Oxide Nanoparticles: Synthesis and Surface Functionalization Strategies, Nanoscale Res. Lett., 2008, 3, 397 CrossRef CAS PubMed.
- A. Andrade, D. Souza, M. Pereira, J. Fabris and R. Domingues, Synthesis and characterization of magnetic nanoparticles coated with silica through a sol-gel approach, Ceramica, 2009, 55, 420–424 CrossRef CAS.
- S. O. Aisida, P. A. Akpa, I. Ahmad, T.-K. Zhao, M. Maaza and F. I. Ezema, Bio-inspired encapsulation and functionalization of iron oxide nanoparticles for biomedical applications, Eur. Polym. J., 2020, 122, 109371 CrossRef CAS.
- Z. Chen, H. Ji, C. Liu, W. Bing, Z. Wang and X. Qu, A Multinuclear Metal Complex Based DNase-Mimetic Artificial Enzyme: Matrix Cleavage for Combating Bacterial Biofilms, Angew Chem. Int. Ed. Engl., 2016, 55, 10732–10736 CrossRef CAS PubMed.
- K. Korschelt, R. Ragg, C. S. Metzger, M. Kluenker, M. Oster, B. Barton, M. Panthöfer, D. Strand, U. Kolb, M. Mondeshki, S. Strand, J. Brieger, M. N. Tahir and W. Tremel, Glycine-functionalized copper(ii) hydroxide nanoparticles with high intrinsic superoxide dismutase activity, Nanoscale, 2017, 9, 3952–3960 RSC.
- A. Sun, L. Mu and X. Hu, Graphene Oxide Quantum Dots as Novel Nanozymes for Alcohol Intoxication, ACS Appl. Mater. Interfaces, 2017, 9, 12241–12252 CrossRef CAS PubMed.
- L. Gao, K. Fan and X. Yan, Iron Oxide Nanozyme: A Multifunctional Enzyme Mimetic for Biomedical Applications, Theranostics, 2017, 7, 3207–3227 CrossRef CAS PubMed.
- Y. Song, K. Qu, C. Zhao, J. Ren and X. Qu, Graphene Oxide: Intrinsic Peroxidase Catalytic Activity and Its Application to Glucose Detection, Adv. Mater., 2010, 22, 2206–2210 CrossRef CAS PubMed.
- R. Ragg, M. N. Tahir and W. Tremel, Solids Go Bio: Inorganic Nanoparticles as Enzyme Mimics, Eur. J. Inorg. Chem., 2016, 2016, 1906–1915 CrossRef CAS.
- H. Cheng, S. Lin, F. Muhammad, Y.-W. Lin and H. Wei, Rationally Modulate the Oxidase-like Activity of Nanoceria for Self-Regulated Bioassays, ACS Sens., 2016, 1, 1336–1343 CrossRef CAS.
- S. Singh, Cerium oxide based nanozymes: Redox phenomenon at biointerfaces, Biointerphases, 2016, 11, 04b202 CrossRef PubMed.
- Z. Dong, Q. Luo and J. Liu, Artificial enzymes based on supramolecular scaffolds, Chem. Soc. Rev., 2012, 41, 7890–7908 RSC.
- Z. Shen, A. Wu and X. Chen, Iron Oxide Nanoparticle Based Contrast Agents for Magnetic Resonance Imaging, Mol. Pharm., 2017, 14, 1352–1364 CrossRef CAS PubMed.
- N. Lee, D. Yoo, D. Ling, M. H. Cho, T. Hyeon and J. Cheon, Iron Oxide Based Nanoparticles for Multimodal Imaging and Magnetoresponsive Therapy, Chem. Rev., 2015, 115, 10637–10689 CrossRef CAS PubMed.
- G. Liu, J. Gao, H. Ai and X. Chen, Applications and potential toxicity of magnetic iron oxide nanoparticles, Small, 2013, 9, 1533–1545 CrossRef CAS PubMed.
- V. Naresh and N. Lee, A Review on Biosensors and Recent Development of Nanostructured Materials-Enabled Biosensors, Sensors, 2021, 21, 1109 CrossRef CAS PubMed.
- R. G. Mahmudunnabi, F. Farhana, N. Kashaninejad, S. Firoz, Y.-B. Shim and M. Shiddiky, Nanozyme-based electrochemical biosensors for disease biomarker detection, Analyst, 2020, 145, 4398–4420 RSC.
- L. Gao, K. Fan and X. Yan, Iron Oxide Nanozyme: A Multifunctional Enzyme Mimetic for Biomedical Applications, Theranostics, 2017, 7, 3207–3227 CrossRef CAS PubMed.
- L. Gao, J. Zhuang, L. Nie, J. Zhang, Y. Zhang, N. Gu, T. Wang, J. Feng, D. Yang, S. Perrett and X. Yan, Intrinsic peroxidase-like activity of ferromagnetic nanoparticles, Nat. Nanotechnol., 2007, 2, 577–583 CrossRef CAS PubMed.
- D. P. Cormode, L. Gao and H. Koo, Emerging Biomedical Applications of Enzyme-Like Catalytic Nanomaterials, Trends Biotechnol., 2018, 36, 15–29 CrossRef CAS PubMed.
- J. Hernández-Ruiz, M. Arnao, A. Hiner, G. Canovas and M. Acosta, Catalase-like activity of horseradish peroxidase: Relationship to enzyme inactivation by H2O2, J. Biol. Chem., 2001, 354, 107–114 Search PubMed.
- P. Campomanes, U. Rothlisberger, M. Alfonso-Prieto and C. Rovira, The Molecular Mechanism of the Catalase-like Activity in Horseradish Peroxidase, J. Am. Chem. Soc., 2015, 137, 11170–11178 CrossRef CAS PubMed.
- G. F. Goya, A. Mayoral, E. Winkler, R. D. Zysler, C. Bagnato, M. Raineri, J. A. Fuentes-García and E. Lima, Next generation of nanozymes: A perspective of the challenges to match biological performance, J. Appl. Phys., 2021, 130, 190903 CrossRef CAS.
- R. Zhang, L. Chen, Q. Liang, J. Xi, H. Zhao, Y. Jin, X. Gao, X. Yan, L. Gao and K. Fan, Unveiling the active sites on ferrihydrite with apparent catalase-like activity for potentiating radiotherapy, Nano Today, 2021, 41, 101317 CrossRef CAS.
- B. Das, J. L. Franco, N. Logan, P. Balasubramanian, M. I. Kim and C. Cao, Nanozymes in Point-of-Care Diagnosis: An Emerging Futuristic Approach for Biosensing, Nano-Micro Lett., 2021, 13, 1–51 CrossRef PubMed.
- X. Ren, D. Chen, Y. Wang, H. Li, Y. Zhang, H. Chen, X. Li and M. Huo, Nanozymes-recent development and biomedical applications, J. Nanobiotechnol., 2022, 20, 92 CrossRef CAS PubMed.
- A. Dutta, S. Maji, D. Srivastava, A. Mondal, P. Biswas, P. Paul and B. Adhikary, Peroxidase-like activity and amperometric sensing of hydrogen peroxide by Fe2O3 and Prussian Blue-modified Fe2O3 nanoparticles, J. Mol. Catal. A: Chem., 2012, 360, 71–77 CrossRef CAS.
- Z. Chen, J.-J. Yin, Y.-T. Zhou, Y. Zhang, L. Song, M. Song, S. Hu and N. Gu, Dual Enzyme-like Activities of Iron Oxide Nanoparticles and Their Implication for Diminishing Cytotoxicity, ACS Nano, 2012, 6, 4001–4012 CrossRef CAS PubMed.
- M. Kurz and R. R. Breaker, In vitro selection of nucleic acid enzymes, Curr. Top. Microbiol. Immunol., 1999, 243, 137–158 CAS.
- B. Liu, X. Han and J. Liu, Iron oxide nanozyme catalyzed synthesis of fluorescent polydopamine for light-up Zn2+ detection, Nanoscale, 2016, 8, 13620–13626 RSC.
- R. Zhang, S. He, C. Zhang and W. Chen, Three-dimensional Fe- and N-incorporated carbon structures as peroxidase mimics for fluorescence detection of hydrogen peroxide and glucose, J. Mater. Chem. B, 2015, 3, 4146–4154 RSC.
- Y. Shi, P. Su, Y. Wang and Y. Yang, Fe3O4 peroxidase mimetics as a general strategy for the fluorescent detection of H2O2-involved systems, Talanta, 2014, 130, 259–264 CrossRef CAS PubMed.
- L. Gao, K. M. Giglio, J. L. Nelson, H. Sondermann and A. J. Travis, Ferromagnetic nanoparticles with peroxidase-like activity enhance the cleavage of biological macromolecules for biofilm elimination, Nanoscale, 2014, 6, 2588–2593 RSC.
- J. Qian, X. Yang, L. Jiang, C. Zhu, H. Mao and K. Wang, Facile preparation of Fe3O4 nanospheres/reduced graphene oxide nanocomposites with high peroxidase-like activity for sensitive and selective colorimetric detection of acetylcholine, Sens. Actuators, B, 2014, 201, 160–166 CrossRef CAS.
- Y. Lin, Y. Huang, J. Ren and X. Qu, Incorporating ATP into biomimetic catalysts for realizing exceptional enzymatic performance over a broad temperature range, NPG Asia Mater., 2014, 6, e114 CrossRef CAS.
- Y.-C. Yang, Y.-T. Wang and W.-L. Tseng, Amplified Peroxidase-Like Activity in Iron Oxide Nanoparticles Using Adenosine Monophosphate: Application to Urinary Protein Sensing, ACS Appl. Mater. Interfaces, 2017, 9, 10069–10077 CrossRef CAS PubMed.
- N. V. S. Vallabani, A. S. Karakoti and S. Singh, ATP-mediated intrinsic peroxidase-like activity of Fe3O4-based nanozyme: One step detection of blood glucose at physiological pH, Colloids Surf., B, 2017, 153, 52–60 CrossRef CAS PubMed.
- B. Liu and J. Liu, Accelerating peroxidase mimicking nanozymes using DNA, Nanoscale, 2015, 7, 13831–13835 RSC.
- C.-H. Liu, C.-J. Yu and W.-L. Tseng, Fluorescence assay of catecholamines based on the inhibition of peroxidase-like activity of magnetite nanoparticles, Anal. Chim. Acta, 2012, 745, 143–148 CrossRef CAS PubMed.
- Y. S. Kim and J. Jurng, A simple colorimetric assay for the detection of metal ions based on the peroxidase-like activity of magnetic nanoparticles, Sens. Actuators, B, 2013, 176, 253–257 CrossRef CAS.
- F. Yu, Y. Huang, A. J. Cole and V. C. Yang, The artificial peroxidase activity of magnetic iron oxide nanoparticles and its application to glucose detection, Biomaterials, 2009, 30, 4716–4722 CrossRef CAS PubMed.
- N. V. S. Vallabani, A. S. Karakoti and S. Singh, ATP-mediated intrinsic peroxidase-like activity of Fe(3)O(4)-based nanozyme: One step detection of blood glucose at physiological Ph, Colloids Surf., B, 2017, 153, 52–60 CrossRef CAS PubMed.
- Y. Song, X. Xia, X. Wu, P. Wang and L. Qin, Integration of Platinum Nanoparticles with a Volumetric Bar-Chart Chip for Biomarker Assays, Angew. Chem., Int. Ed., 2014, 53, 12451–12455 CrossRef CAS PubMed.
- K. Fan, H. Wang, J. Xi, Q. Liu, X. Meng, D. Duan, L. Gao and X. Yan, Optimization of Fe3O4 nanozyme activity via single amino acid modification mimicking an enzyme active site, Chem. Commun., 2017, 53, 424–427 RSC.
- N. Wang, L. Zhu, D. Wang, M. Wang, Z. Lin and H. Tang, Sono-assisted preparation of highly-efficient peroxidase-like Fe3O4 magnetic nanoparticles for catalytic removal of organic pollutants with H2O2, Ultrason. Sonochem., 2010, 17, 526–533 CrossRef CAS PubMed.
- N. C. Veitch, Horseradish peroxidase: a modern view of a classic enzyme, Phytochemistry, 2004, 65, 249–259 CrossRef CAS PubMed.
- X. Li and J. Paier, Adsorption of Water on the Fe3O4 (111) Surface: Structures, Stabilities, and Vibrational Properties Studied by Density Functional Theory, J. Phys. Chem. C, 2016, 120, 1056–1065 CrossRef CAS.
- S. Liu, F. Lu, R. Xing and J.-J. Zhu, Structural Effects of Fe3O4 Nanocrystals on Peroxidase-Like Activity, Chem.– Eur. J., 2011, 17, 620–625 CrossRef CAS PubMed.
- H. Wei and E. Wang, Fe3O4 Magnetic Nanoparticles as Peroxidase Mimetics and Their Applications in H2O2 and Glucose Detection, Anal. Chem., 2008, 80, 2250–2254 CrossRef CAS PubMed.
- L. Gao, J. Wu, S. Lyle, K. Zehr, L. Cao and D. Gao, Magnetite Nanoparticle-Linked Immunosorbent Assay, J. Phys. Chem. C, 2008, 112, 17357–17361 CrossRef CAS.
- X. Wang, R. Niessner, D. Tang and D. Knopp, Nanoparticle-based immunosensors and immunoassays for aflatoxins, Anal. Chim. Acta, 2016, 912, 10–23 CrossRef CAS PubMed.
- D. Bhattacharya, A. Baksi, I. Banerjee, R. Ananthakrishnan, T. K. Maiti and P. Pramanik, Development of phosphonate modified Fe(1−x)MnxFe2O4 mixed ferrite nanoparticles: Novel peroxidase mimetics in enzyme linked immunosorbent assay, Talanta, 2011, 86, 337–348 CrossRef CAS PubMed.
- M. Yang, Y. Guan, Y. Yang, L. Xie, T. Xia, W. Xiong and C. Guo, Immunological detection of hepatocellular carcinoma biomarker GP73 based on dissolved magnetic nanoparticles, Colloids Surf., A, 2014, 443, 280–285 CrossRef CAS.
- M. Yang, Y. Guan, Y. Yang, T. Xia, W. Xiong, N. Wang and C. Guo, Peroxidase-like activity of amino-functionalized magnetic nanoparticles and their applications in immunoassay, J. Colloid Interface Sci., 2013, 405, 291–295 CrossRef CAS PubMed.
- M. Yang, Y. Guan, Y. Yang, T. Xia, W. Xiong and C. Guo, A sensitive and rapid immunoassay for mycoplasma pneumonia based on Fe3O4 nanoparticles, Mater. Lett., 2014, 137, 113–116 CrossRef CAS.
- M. A. Woo, M. I. Kim, J. H. Jung, K. S. Park, T. S. Seo and H. G. Park, A novel colorimetric immunoassay utilizing the peroxidase mimicking activity of magnetic nanoparticles, Int. J. Mol. Sci., 2013, 14, 9999–10014 CrossRef PubMed.
- M. I. Kim, M. S. Kim, M. A. Woo, Y. Ye, K. S. Kang, J. Lee and H. G. Park, Highly efficient colorimetric detection of target cancer cells utilizing superior catalytic activity of graphene oxide-magnetic-platinum nanohybrids, Nanoscale, 2014, 6, 1529–1536 RSC.
- Y. Wu, M. Song, Z. Xin, X. Zhang, Y. Zhang, C. Wang, S. Li and N. Gu, Ultra-small particles of iron oxide as peroxidase for immunohistochemical detection, Nanotechnol, 2011, 22, 225703 CrossRef PubMed.
- D. Duan, K. Fan, D. Zhang, S. Tan, M. Liang, Y. Liu, J. Zhang, P. Zhang, W. Liu, X. Qiu, G. P. Kobinger, G. F. Gao and X. Yan, Nanozyme-strip for rapid local diagnosis of Ebola, Biosens. Bioelectron., 2015, 74, 134–141 CrossRef CAS PubMed.
- R. Thiramanas, K. Jangpatarapongsa, P. Tangboriboonrat and D. Polpanich, Detection of Vibrio cholerae using the intrinsic catalytic activity of a magnetic polymeric nanoparticle, Anal. Chem., 2013, 85, 5996–6002 CrossRef CAS PubMed.
- Z. Zhang, Z. Wang, X. Wang and X. Yang, Magnetic nanoparticle-linked colorimetric aptasensor for the detection of thrombin, Sens. Actuators, B, 2010, 147, 428–433 CrossRef CAS.
- L. Wu, M. Zhou, Y. Wang and J. Liu, Nanozyme and aptamer- based immunosorbent assay for aflatoxin B1, J. Hazard. Mater., 2020, 399, 123154 CrossRef CAS PubMed.
- K. Fan, C. Cao, Y. Pan, D. Lu, D. Yang, J. Feng, L. Song, M. Liang and X. Yan, Magnetoferritin nanoparticles for targeting and visualizing tumour tissues, Nat. Nanotechnol., 2012, 7, 459–464 CrossRef CAS PubMed.
- J. Zhuang, K. Fan, L. Gao, D. Lu, J. Feng, D. Yang, N. Gu, Y. Zhang, M. Liang and X. Yan, Ex Vivo Detection of Iron Oxide Magnetic Nanoparticles in Mice Using Their Intrinsic Peroxidase-Mimicking Activity, Mol. Pharm., 2012, 9, 1983–1989 CrossRef CAS PubMed.
- D. Zhang, Y.-X. Zhao, Y.-J. Gao, F.-P. Gao, Y.-S. Fan, X.-J. Li, Z.-Y. Duan and H. Wang, Anti-bacterial and in vivo tumor treatment by reactive oxygen species generated by magnetic nanoparticles, J. Mater. Chem. B, 2013, 1, 5100–5107 RSC.
- K. Fan, C. Cao, Y. Pan, D. Lu, D. Yang, J. Feng, L. Song, M. Liang and X. Yan, Magnetoferritin nanoparticles for targeting and visualizing tumour tissues, Nat. Nanotechnol., 2012, 7, 459–464 CrossRef CAS PubMed.
- Y. Liu, P. Naha, G. Hwang, D. Kim, Y. Huang, A. Simon-Soro, H.-I. Jung, Z. Ren, Y. Li, S. Gubara, F. Alawi, D. Zero, A. T. Hara, D. P. Cormode and H. Koo, Topical ferumoxytol nanoparticles disrupt biofilms and prevent severe tooth decay in vivo via intrinsic catalytic activity, Nat. Commun., 2018, 9, 2920 CrossRef PubMed.
- M. Huo, L. Wang, Y. Chen and J. Shi, Tumor-selective catalytic nanomedicine by nanocatalyst delivery, Nat. Commun., 2017, 8, 357 CrossRef PubMed.
- S. E. Kim, L. Zhang, K. Ma, M. Riegman, F. Chen, I. Ingold, M. Conrad, M. Z. Turker, M. Gao, X. Jiang, S. Monette, M. Pauliah, M. Gonen, P. Zanzonico, T. Quinn, U. Wiesner, M. S. Bradbury and M. Overholtzer, Ultrasmall nanoparticles induce ferroptosis in nutrient-deprived cancer cells and suppress tumour growth, Nat. Nanotechnol., 2016, 11, 977–985 CrossRef CAS PubMed.
- S. Zanganeh, G. Hutter, R. Spitler, O. Lenkov, M. Mahmoudi, A. Shaw, J. S. Pajarinen, H. Nejadnik, S. Goodman, S. Moseley, L. M. Coussens and H. E. Daldrup-Link, Iron oxide nanoparticles inhibit tumour growth by inducing pro-inflammatory macrophage polarization in tumour tissues, Nat. Nanotechnol., 2016, 11, 986–994 CrossRef CAS PubMed.
- G. Tang, J. He, J. Liu, X. Yan and K. Fan, Nanozyme for tumor therapy: Surface modification matters, Exploration, 2021, 1, 75–89 CrossRef PubMed.
- F. Wei, X. Cui, Z. Wang, C. Dong, J. Li and X. Han, Recoverable peroxidase-like Fe3O4@MoS2-Ag nanozyme with enhanced antibacterial ability, Chem. Eng. J., 2021, 408, 127240 CrossRef CAS PubMed.
- Y. Wang, H. Li, L. Guo, Q. Jiang and F. Liu, A cobalt-doped iron oxide nanozyme as a highly active peroxidase for renal tumor catalytic therapy, RSC Adv., 2019, 9, 18815–18822 RSC.
- K. Sun, Z. Gao, Y. Zhang, H. Wu, C. You, S. Wang, P. An, C. Sun and B. Sun, Enhanced highly toxic reactive oxygen species levels from iron oxide core-shell mesoporous silica nanocarrier-mediated Fenton reactions for cancer therapy, J. Mater. Chem. B, 2018, 6, 5876–5887 RSC.
- S. Li, L. Shang, B. Xu, S. Wang, K. Gu, Q. Wu, Y. Sun, Q. Zhang, H. Yang, F. Zhang, L. Gu, T. Zhang and H. Liu, A Nanozyme with Photo-Enhanced Dual Enzyme-Like Activities for Deep Pancreatic Cancer Therapy, Angew. Chem., Int. Ed., 2019, 58, 12624–12631 CrossRef CAS PubMed.
- N. V. S. Vallabani, A. Vinu, S. Singh and A. Karakoti, Tuning the ATP-triggered pro-oxidant activity of iron oxide-based nanozyme towards an efficient antibacterial strategy, J. Colloid Interface Sci., 2020, 567, 154–164 CrossRef CAS PubMed.
- H. Cheng, L. Zhang, J. He, W. Guo, Z. Zhou, X. Zhang, S. Nie and H. Wei, Integrated Nanozymes with Nanoscale Proximity for in Vivo Neurochemical Monitoring in Living Brains, Anal. Chem., 2016, 88, 5489–5497 CrossRef CAS PubMed.
- H. Wei and E. Wang, Fe3O4 magnetic nanoparticles as peroxidase mimetics and their applications in H2O2 and glucose detection, Anal. Chem., 2008, 80, 2250–2254 CrossRef CAS PubMed.
- Y. Gao, G. Wang, H. Huang, J. Hu, S. M. Shah and X. Su, Fluorometric method for the determination of hydrogen peroxide and glucose with Fe3O4 as catalyst, Talanta, 2011, 85, 1075–1080 CrossRef CAS PubMed.
- Y.-I. Dong, H.-G. Zhang, Z. U. Rahman, I. Su, X.-J. Chen, J. Hu and X.-G. Chen, Graphene oxide-Fe3O4 magnetic nanocomposites with peroxidase-like activity for colorimetric detection of glucose, Nanoscale, 2012, 4, 3969–3976 RSC.
- Q. Liu, L. Zhang, H. Li, Q. Jia, Y. Jiang, Y. Yang and R. Zhu, One-pot synthesis of porphyrin functionalized γ-Fe2O3 nanocomposites as peroxidase mimics for H2O2 and glucose detection, Mater. Sci. Eng., C, 2015, 55, 193–200 CrossRef CAS PubMed.
- Y. Wang, B. Zhou, S. Wu, K. Wang and X. He, Colorimetric detection of hydrogen peroxide and glucose using the magnetic mesoporous silica nanoparticles, Talanta, 2015, 134, 712–717 CrossRef CAS PubMed.
- Q. Chang and H. Tang, Optical determination of glucose and hydrogen peroxide using a nanocomposite prepared from glucose oxidase and magnetite nanoparticles immobilized on graphene oxide, Microchim. Acta, 2014, 181, 527–534 CrossRef CAS.
- M. I. Kim, J. Shim, T. Li, J. Lee and H. G. Park, Fabrication of Nanoporous Nanocomposites Entrapping Fe3O4 Magnetic Nanoparticles and Oxidases for Colorimetric Biosensing, Chem.–Eur. J., 2011, 17, 10700–10707 CrossRef CAS PubMed.
- M. I. Kim, J. Shim, T. Li, M.-A. Woo, D. Cho, J. Lee and H. G. Park, Colorimetric quantification of galactose using a nanostructured multi-catalyst system entrapping galactose oxidase and magnetic nanoparticles as peroxidase mimetics, Analyst, 2012, 137, 1137–1143 RSC.
- M. I. Kim, J. Shim, H. J. Parab, S. C. Shin, J. Lee and H. G. Park, A convenient alcohol sensor using one-pot nanocomposite entrapping alcohol oxidase and magnetic nanoparticles as peroxidase mimetics, J. Nanosci. Nanotechnol., 2012, 12, 5914–5919 CrossRef CAS PubMed.
- S. Singh, Nanomaterials Exhibiting Enzyme-Like Properties (Nanozymes): Current Advances and Future Perspectives, Front. Chem., 2019, 7, 46 CrossRef CAS PubMed.
- D. Duan, K. Fan, D. Zhang, S. Tan, M. Liang, Y. Liu, J. Zhang, P. Zhang, W. Liu and X. Qiu, Nanozyme-strip for rapid local diagnosis of Ebola, Biosens. Bioelectron., 2015, 74, 134–141 CrossRef CAS PubMed.
- M. S. Kim, S. H. Kweon, S. Cho, S. S. A. An, M. I. Kim, J. Doh and J. Lee, Pt-Decorated Magnetic Nanozymes for Facile and Sensitive Point-of-Care Bioassay, ACS Appl. Mater. Interfaces, 2017, 9, 35133–35140 CrossRef CAS PubMed.
- N. Cheng, C. Zhu, Y. Wang, D. Du, M.-J. Zhu, Y. Luo, W. Xu and Y. Lin, Nanozyme Enhanced Colorimetric Immunoassay for Naked-Eye Detection of Salmonella Enteritidis, J. Anal. Test., 2019, 3, 99–106 CrossRef.
- H. Y. Shin, B.-G. Kim, S. Cho, J. Lee, H. B. Na and M. I. Kim, Visual determination of hydrogen peroxide and glucose by exploiting the peroxidase-like activity of magnetic nanoparticles functionalized with a poly(ethylene glycol) derivative, Microchim. Acta, 2017, 184, 2115–2122 CrossRef CAS.
- L. Zhang, R. Huang, W. Liu, H. Liu, X. Zhou and D. Xing, Rapid and visual detection of Listeria monocytogenes based on nanoparticle cluster catalyzed signal amplification, Biosens. Bioelectron., 2016, 86, 1–7 CrossRef CAS PubMed.
- W. Li, G.-C. Fan, F. Gao, Y. Cui, W. Wang and X. Luo, High-activity Fe3O4 nanozyme as signal amplifier: A simple, low-cost but efficient strategy for ultrasensitive photoelectrochemical immunoassay, Biosens. Bioelectron., 2019, 127, 64–71 CrossRef CAS PubMed.
- L. Tian, J. Qi, O. Oderinde, C. Yao, W. Song and Y. Wang, Planar intercalated copper (II) complex molecule as small molecule enzyme mimic combined with Fe3O4 nanozyme for bienzyme synergistic catalysis applied to the microRNA biosensor, Biosens. Bioelectron., 2018, 110, 110–117 CrossRef CAS PubMed.
- T. Li, P. Hu, J. Li, P. Huang, W. Tong and C. Gao, Enhanced peroxidase-like activity of Fe@PCN-224 nanoparticles and their applications for detection of H2O2 and glucose, Colloids Surf., A, 2019, 577, 456–463 CrossRef CAS.
- R. Zhang, N. Lu, J. Zhang, R. Yan, J. Li, L. Wang, N. Wang, M. Lv and M. Zhang, Ultrasensitive aptamer-based protein assays based on one-dimensional core-shell nanozymes, Biosens. Bioelectron., 2020, 150, 111881 CrossRef CAS PubMed.
- W. Yang, J. Li, M. Wang, X. Sun, Y. Liu, J. Yang and D. H. Ng, A colorimetric strategy for ascorbic acid sensing based on the peroxidase-like activity of core-shell Fe3O4/CoFe-LDH hybrid, Colloids Surf., B, 2020, 188, 110742 CrossRef CAS PubMed.
- X. Wang, Y. Hu and H. Wei, Nanozymes in bionanotechnology: from sensing to therapeutics and beyond, Inorg. Chem. Front., 2016, 3, 41–60 RSC.
- L. Gao, X. Yan and X. Nanozymes, an emerging field bridging nanotechnology and biology, Sci. China: Life Sci., 2016, 59, 400–402 CrossRef PubMed.
- D. Jiang, D. Ni, Z. T. Rosenkrans, P. Huang, X. Yan and W. Cai, Nanozyme: new horizons for responsive biomedical applications, Chem. Soc. Rev., 2019, 48, 3683–3704 RSC.
- J. Wu, X. Wang, Q. Wang, Z. Lou, S. Li, Y. Zhu, L. Qin and H. Wei, Nanomaterials with enzyme-like characteristics (nanozymes): next-generation artificial enzymes (II), Chem. Soc. Rev., 2019, 48, 1004–1076 RSC.
- Y. Meng, W. Li, X. Pan and G. M. Gadd, Applications of nanozymes in the environment, Environ. Sci.: Nano, 2020, 7, 1305–1318 RSC.
- W. Li, G. C. Fan, F. Gao, Y. Cui, W. Wang and X. Luo, High-activity Fe(3)O(4) nanozyme as signal amplifier: A simple, low-cost but efficient strategy for ultrasensitive photoelectrochemical immunoassay, Biosens. Bioelectron., 2019, 127, 64–71 CrossRef CAS PubMed.
- Y. Guo, Y. Tao, X. Ma, J. Jin, S. Wen, W. Ji, W. Song, B. Zhao and Y. Ozaki, A dual colorimetric and SERS detection of Hg2+ based on the stimulus of intrinsic oxidase-like catalytic activity of Ag-CoFe2O4/reduced graphene oxide nanocomposites, Chem. Eng. J., 2018, 350, 120–130 CrossRef CAS.
- T. Qin, R. Ma, Y. Yin, X. Miao, S. Chen, K. Fan, J. Xi, Q. Liu, Y. Gu, Y. Yin, J. Hu, X. Liu, D. Peng and L. Gao, Catalytic inactivation of influenza virus by iron oxide nanozyme, Theranostics, 2019, 9, 6920–6935 CrossRef CAS PubMed.
- J. Jiang, C. He, S. Wang, H. Jiang, J. Li and L. Li, Recyclable ferromagnetic chitosan nanozyme for decomposing phenol, Carbohydr. Polym., 2018, 198, 348–353 CrossRef CAS PubMed.
- M. Huo, L. Wang, Y. Chen and J. Shi, Tumor-selective catalytic nanomedicine by nanocatalyst delivery, Nat. Commun., 2017, 8, 357 CrossRef PubMed.
- P. Boruah and M. Das, Dual responsive magnetic Fe3O4-TiO2/graphene nanocomposite as an artificial nanozyme for the colorimetric detection and photodegradation of pesticide in an aqueous medium, J. Hazard. Mater., 2019, 385, 121516 CrossRef PubMed.
- A. Nsabimana, S. A. Kitte, F. Wu, L. Qi, Z. Liu, M. N. Zafar, R. Luque and G. Xu, Multifunctional magnetic Fe3O4/nitrogen-doped porous carbon nanocomposites for removal of dyes and sensing applications, Appl. Surf. Sci., 2019, 467, 89–97 CrossRef.
- M. Wang, N. Wang, H. Tang, M. Cao, Y. She and L. Zhu, Surface modification of nano- Fe3O4 with EDTA and its use in H2O2 activation for removing organic pollutants, Catal. Sci. Technol., 2012, 2, 187–194 RSC.
- F. Chen, S. Xie, X. Huang and X. Qiu, Ionothermal synthesis of Fe3O4 magnetic nanoparticles as efficient heterogeneous Fenton-like catalysts for degradation of organic pollutants with H2O2, J. Hazard. Mater., 2017, 322, 152–162 CrossRef CAS PubMed.
- C. Xiao, J. Li and G. Zhang, Synthesis of stable burger-like α-Fe2O3 catalysts: Formation mechanism and excellent photo-Fenton catalytic performance, J. Cleaner Prod., 2018, 180, 550–559 CrossRef CAS.
- M. Mahmoudi, A. Simchi, M. Imani and U. O. Häfeli, Superparamagnetic iron oxide nanoparticles with rigid cross-linked polyethylene glycol fumarate coating for application in imaging and drug delivery, J. Phys. Chem. C, 2009, 115, 9325–9335 Search PubMed.
- D. L. Thorek, A. K. Chen, J. Czupryna and A. Tsourkas, Superparamagnetic iron oxide nanoparticle probes for molecular imaging, Ann. Biomed. Eng., 2006, 34, 23–38 CrossRef PubMed.
- B. Szalay, E. Tátrai, G. Nyírő, T. Vezér and G. Dura, Potential toxic effects of iron oxide nanoparticles in in vivo and in vitro experiments, J. Appl. Toxicol., 2012, 32, 446–453 CrossRef CAS PubMed.
|
This journal is © The Royal Society of Chemistry 2024 |
Click here to see how this site uses Cookies. View our privacy policy here.