DOI:
10.1039/D4MH00500G
(Communication)
Mater. Horiz., 2024,
11, 3561-3572
Femtosecond laser composite manufactured double-bionic micro–nano structure for efficient photothermal anti-icing/deicing†
Received
27th April 2024
, Accepted 13th June 2024
First published on 14th June 2024
Abstract
The solar anti-icing/deicing (SADI) strategy represents an environmentally friendly approach for removing ice efficiently. However, the extensive use of photothermal materials could negatively impact financial performance. Therefore, enhancing light utilization efficiency, especially by optimizing the design of a structure with a low content of photothermal materials, has rapidly become a focal point of research. Drawing inspiration from the antireflective micro–nano structure of compound eyes and the thermal insulating hollow structure of polar bear hair, we proposed a new strategy to design a bionic micro–nano hollow film (MNHF). The MNHF was created using a composite manufacturing process that combines femtosecond laser ablation with template transfer techniques. Both theoretical simulations and empirical tests have confirmed that this structure significantly improves photothermal conversion efficiency and thermal radiation capability. Compared to plane film, the photothermal conversion efficiency of MNHF is increased by 45.85%. Under 1.5 sun, the equilibrium temperature of MNHF can reach 73.8 °C. Moreover, even after 10 icing–deicing cycles, MNHF maintains an ultra-low ice adhesion strength of 1.8 ± 0.3 kPa. Additionally, the exceptional mechanical stability, chemical resistance, and self-cleaning capabilities of the MNHF make its practical application feasible. This innovative structure paves the way for designing cost-effective and robust surfaces for efficient photothermal anti-icing/deicing on airplane wings.
New concepts
The extensive use of expensive photothermal materials limits the practical application of photothermal superhydrophobic surfaces. Therefore, improving the photothermal efficiency with a low content of photothermal materials through structural optimization has become both a research focus and a challenge. In this work, drawing inspiration from the micro–nano structure found in the compound eyes of a mosquito and the hollow structure present in the hair of polar bears, we designed a novel micro–nano hollow film (MNHF) to achieve an excellent anti-icing/deicing performance. As expected, the hollow microcolumn structure inside the MNHF can not only facilitate the absorption of light due to multiple reflections, but also block the conduction of heat to the metal substrate. Besides, the micro–nano structure on the upper surface of the MNHF can reduce the reflection of light and extend the light path, finally contributing to the considerable promotion of light absorption. The double-bionic MNHF, which simultaneously increases the photothermal conversion efficiency by about 45.85% more than plane film and enhances the thermal radiation property, even at a low MWCNT content of only 0.05 wt%, can contribute a lot to the increase in surface temperature and the reduction in ice formation. Furthermore, the MNHF shows an extremely low ice adhesion strength of 1.8 ± 0.3 kPa after 10 icing–deicing cycles. In addition, MNHF, exhibiting attractive mechanical stability, chemical stability, and excellent self-cleaning ability, is believed to meet the requirements for long-term usage. This work offers a new idea for low-cost, high-efficiency and mild anti-icing/deicing.
|
Introduction
Airplane wings constantly experience the impact of supercooled water drops which are easier to freeze, leading to undesirable ice accumulation and even causing severe safety issues.1–3 Hence, efficient anti-icing and deicing are important to the global aviation industry. Currently, common active deicing tactics include electro-thermal methods,4 photothermal methods,5–7 mechanical removal,8 and chemical methods.9 Among these techniques, photothermal deicing shows great prospects due to the inexhaustible supply of solar energy, and it is highly consistent with the sustainable development concept.10,11 It converts the absorbed solar energy into heat using photothermal conversion materials, thus keeping the temperature above freezing point to activate anti-icing/deicing. Unfortunately, photothermal deicing surfaces show poor anti-icing/deicing efficiency at night. A passive anti-icing/deicing method is a good strategy to address this issue. It relies mainly on Nepenthes-inspired slippery liquid-infused porous surfaces (SLIPS)12–14 and lotus-leaf-inspired superhydrophobic surfaces (SHS).15–18 However, SLIPS cannot avoid the loss of lubricant caused by the removal of ice.19,20 Furthermore, under harsh environmental conditions such as low temperature, a droplet on SHS will experience the transition from the Cassie–Baxter state to the Wenzel state, resulting in an undesirable increase in ice adhesion strength. In addition, the poor mechanical durability of the SHS surface limits its further use.21 Therefore, a promising approach is to endow surfaces with both passive anti-icing and active deicing properties simultaneously.
Recently, researchers have engineered surfaces that exhibit both photothermal deicing and superhydrophobic anti-icing properties. To enhance the photothermal conversion efficiency, the introduction of multiscale micro–nanostructures as photothermal traps has been extensively reported. For instance, Xie et al. utilized a template spraying method to create a multi-scale micro–nano hierarchical structure, achieving a photothermal conversion efficiency of 60.76%.22 Li et al. combined laser surface direct writing and thermal evaporation techniques to produce micro/nano structured surfaces with high-efficiency photothermal trap capacity and superhydrophobicity, where solar absorbance on the surface can exceed 94.5%.23 However, most photothermal conversion materials, such as graphene24 and MXenes25 are too expensive to benefit the financial performance. Thus, it is crucial to devise a rational structure to improve photothermal conversion efficiency even at low concentrations of photothermal materials. The polar bear and mosquito have unique properties. A polar bear can endure extremely cold conditions due to the distinctive photothermal conversion characteristics of its hollow hair.26,27 Light penetrates through the hair's hollow structure and is refracted to the dark skin of the polar bear, providing energy for survival. The porous matrix within the hollow structure also creates “light traps”, ensuring that incident light is fully captured and converted into thermal energy. Bio-inspired hollow carbon microtubes (HCMTs) fabricated by Li et al. confirmed that the hollow structure can reduce heat loss and possesses the ability to trap heat, thereby improving light utilization.28 Besides, the nano cone array (300 nm) on the compound eye structure of a mosquito mitigates variation in the refractive index, significantly enhancing the Fresnel reflection.29 The micro–nano structure of a compound eye also endows it with excellent superhydrophobicity, enabling it to achieve anti-fog properties.30,31 Zhao et al. designed moth-eye-inspired textured surfaces that were proven to reduce light reflection.32 However, a single biomimetic structure can hardly meet the simultaneous needs of both improving photothermal utilization efficiency and reducing the use of photothermal materials, leading to an unsatisfactory anti-icing/deicing effect. Combining the hollow structure of polar bear hair with the compound eye structure of a mosquito may represent an effective approach to further improve photothermal efficiency and anti-icing/deicing performance.
In this study, inspired by the thermal insulation capabilities of polar bear hollow hair and anti-reflective effects endowed by the micro–nano structure of mosquito compound eyes, we propose a new strategy to enhance photothermal conversion efficiency by optimizing the design of a structure with a typically low concentration of photothermal materials. A micro–nano hollow film (MNHF), characterized by highly efficient anti-icing/deicing properties, was prepared using femtosecond laser ablation and template transfer methods. First, a hollow polystyrene membrane (PSM) template with a high aspect ratio was prepared through femtosecond laser ablation, taking advantage of high processing accuracy and uniformity.33–36 Subsequently, a hollow film (HF) of PDMS prepolymer, loaded with multi-walled carbon nanotubes (PDMS-MWCNTs), was obtained using a double template transfer method. Specific micro–nano structures were then imparted to the HF surface by femtosecond laser ablation, resulting in the final MNHF. Theoretical simulations and experiments confirmed that the MNHF exhibits excellent photothermal conversion efficiency and thermal radiation capability, even with a mere 0.05 wt% of MWCNTs. The rapid photothermal response effect of the superhydrophobic surface accelerates the ice melting process, allowing the ice–melt water mixture to quickly roll off the surface, thus reducing mechanical wear during deicing. Even after 10 bending–torsion cycles as well as 50 h of processing in acid or alkali solutions, the MNHF maintains an unexpectedly good photothermal effect and superhydrophobicity. Furthermore, the MNHF demonstrates a remarkable ice-phobic property at night, keeping an ice adhesion strength of 1.8 ± 0.3 kPa after 10 icing–deicing cycles. Due to its superior photothermal effect and surprising ice-phobic properties, the MNHF is expected to achieve promising anti-icing/deicing performance.
Results and discussion
Fabrication and optimization of MNHF
As reported earlier, the hollow hair of a polar bear can effectively inhibit heat loss.37 The micro–nano structure of mosquito compound eyes can increase the absorption of light and delay the freezing of droplets29 (Fig. 1(a)). Inspired by these special characteristics of polar bear hair and mosquito eyes, the MNHF can achieve efficient anti-icing/deicing performance through promoted photothermal conversion efficiency during the daytime and decreased ice adhesion at night (Fig. 1(b)). To replicate the hollow structure of polar bear hair, HF, with high-aspect-ratio hollow microcolumns, was prepared (Fig. 1(c)-I–VI). PSM was selected to be template material because of its great thermal shrinkage performance. Holes were first made on the surface of PSM by femtosecond laser ablation. Then, the processed porous PSM was heated by a heat gun to shrink it (Movie S1, ESI†). The thickness of the PSM increases from 200 μm to about 1200 μm, and the length of the PSM shrinks to 40% of the original size (Fig. S1, ESI†). Fig. S2, ESI† shows a microcolumn template transferred by a shrunken porous PSM. MWCNT was selected as a photothermal material because it is cheap and shows broadband absorption of natural light. Finally, HF was obtained by transferring a PDMS-MWCNTs mixture using a microcolumn template. To enhance the anti-icing/deicing ability of HF in a dark environment, a micro–nano structure was constructed by femtosecond laser ablation on the upper surface of HF (Fig. 1(c)-VII), and a double-bionic MNHF was finally obtained (Fig. 1(d)). The operations of femtosecond laser ablation and template transfer are relatively simple, which can meet the needs of practical applications for large-area preparation. There are micro wrinkles in the inner wall of the hollow microcolumn of the MNHF (Fig. 1(e)), which can increase the number of reflections and refractions of incident light and improve the light consumption efficiency. An MNHF with enhanced light utilization and superhydrophobicity is believed to be a potential candidate to attach on airplane wings for highly efficient anti-icing/deicing (Fig. 1(f)).
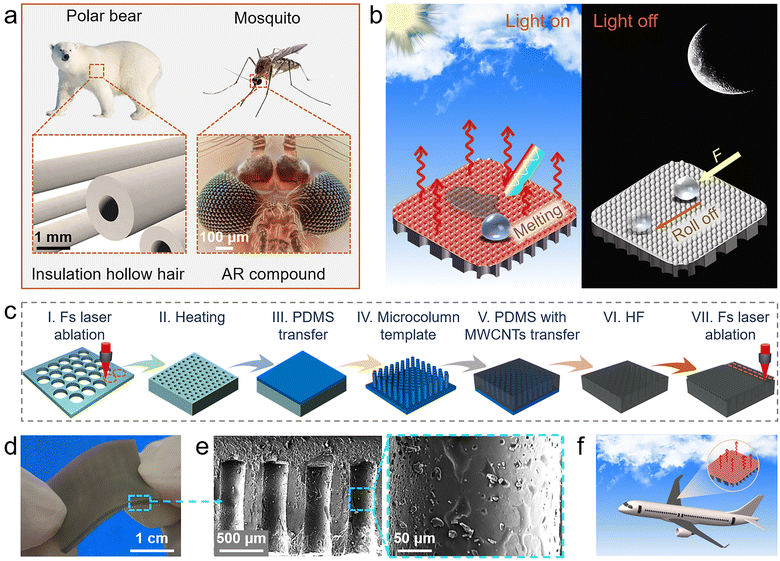 |
| Fig. 1 Design, fabrication, and characterization of MNHF. (a) The design of MNHF was inspired by polar bear hair and mosquito eyes. (b) Deicing and anti-icing performance of MNHF during the day and at night. (c) Illustration of the fabrication process of MNHF. (d) Optical image of MNHF. (e) SEM images of the hollow structure of MNHF. (f) Schematic illustration of an airplane with two wings covered with MNHF. | |
In order to adjust the micro–nano structure to an optimal parameter, the surface morphology of the samples was controlled by adjusting the scanning distance when the laser power was set to 30 mW. When the scanning distance is 16 μm, the surface is completely covered with PDMS nanoparticles but without a micron-scale structure (Fig. 2(a)), and it is recorded as inhomogeneous micro–nano hollow film (IMNHF). This is because the ablation areas overlap each other when the scanning distance (l) is less than twice the diameter of the laser spot (dl) (Fig. 2(b)). When the scanning distance is 20 μm, the surface of the MNHF is observed to possess a micro cone array covered with PDMS nanoparticles due to having the same values of l and 2dl (Fig. 2(c) and (d)). When the scanning distance is 24 μm, the top of this micro cone array turns out to have a truncated shape and it is only partially covered by PDMS nano-particles because l is larger than 2dl (Fig. 2(e) and 2(f)), defining it as a micro–nano truncated-shaped hollow film (MNTHF). The 3D surface topography and cross-sectional topography of IMNHF, MNHF, and MNTHF can be observed in Fig. S3, ESI.† These results are consistent with the test results of SEM. The roughness (Ra) of MNHF is between that of IMNHF and MNTHF because its variation trend is highly consistent with that of l (Fig. S4a, ESI†). Fig. S4b, ESI† illustrates the elemental contents of IMNHF, MNHF, and MNTHF. It can be determined from Fig. S4b, ESI† that the content of O is largest in MNTHF and smallest in IMNHF. The reason is that the ratio of femtosecond laser scanning area to total area decreases with increasing l, leading to a lower content of O. These three kinds of surfaces all exhibit superhydrophobicity. As shown in Fig. 2(g) and (h), the CAs and SAs of IMNHF, MNHF, and MNTHF are all higher than 150° and lower than 10°, which are much higher than the CA of plane (113.6°) and lower than the SA of plane (180°). It is noteworthy that IMNHF possesses no micro cone array, which is instrumental to the maintenance of an air cushion and superhydrophobicity. Besides, the top surface of the microstructure on MNTHF is not completely covered by PDMS nanoparticles, which is undesirable in promoting hydrophobic properties. This well explains the reason why MNHF presents the largest CA of about 163.8° and lowest SA of about 0.5° among these surfaces.
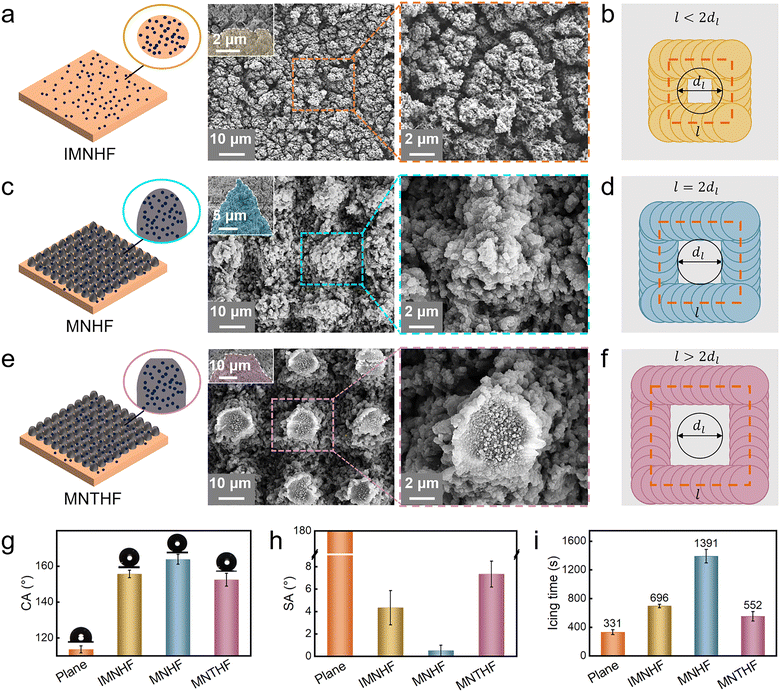 |
| Fig. 2 The morphology, wettability, and anti-icing performance of three kinds of micro–nano structures. (a) Structural schematic and SEM images of IMNHF. (b) Laser scanning mode and related parameters of IMNHF. (c) Structural schematic and SEM images of MNHF. (d) The laser scanning mode and related parameters of MNHF. (e) Structural schematic and SEM images of MNTHF. (f) Laser scanning mode and related parameters of MNTHF. (g) Water CAs of plane, IMNHF, MNHF, and MNTHF surface. (h) SAs of plane, IMNHF, MNHF, and MNTHF surfaces. (i) Icing time of plane, IMNHF, MNHF, and MNTHF surfaces. | |
In order to further verify the anti-icing property, anti-icing tests were carried out on IMNHF, MNHF, and MNTHF. The results are shown in Fig. 2(i), where MNHF has the longest icing delay time, because the micro-/nano-scale structure of MNHF can trap a thicker air cushion. In addition, the icing delay time of a superhydrophobic surface is believed to be affected mainly by surface morphology. The MNHF surface that is completely covered by PDMS nanoparticles has a higher icing nucleation barrier, which can be explained by the classical nucleation formula:38,39
| 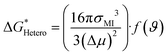 | (1) |
| 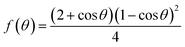 | (2) |
where

is the energy barrier that needs to be broken in the heterogeneous nucleation process of droplets;
σMI is the surface tension between liquid and ice crystals; and Δ
μ is the degree of supersaturation caused by temperature and determines the higher

than for the other two according to the proportional relation of the formulas. In addition, the micro cone array further inhibits heat transfer by trapping more air cushions, which endows MNHF with a longer icing delay time than that of IMNHF or MNTHF. Therefore, MNHF was selected for subsequent experiments.
Photothermal performance
A double-bionic composite structure, composed of a mosquito-eye-like upper surface and polar-bear-hair-like hollow structure (Fig. 3(a)), can spontaneously form a double-layer light trap, endowing a carbon-based material with higher utilization efficiency of light. Light will be reflected multiple times between adjacent micro cones when the micro–nano structure is irradiated. On one hand, the micro–nano structure can reduce the reflection of light. On the other hand, the extended light path will promote absorption of light by the surface. The internal hollow microcolumn structure can not only facilitate the absorption of light due to multiple reflections, but also block conduction of heat to the metal substrate, which greatly increases the temperature of the surface and inhibits the formation of ice. To determine the necessity for such a hollow structure and micro–nano morphology, HF and micro–nano film (MNF) were designed for further comparison. In addition, theoretical simulations using a finite-element method were selected to hypothetically verify that a double-bionic composite structure promotes the absorption of light. The light distributions of different models were calculated with finite-element analysis software. A planar optical source with an intensity of 1-sun (1 kW m−2) was selected as incident light. The results are shown in Fig. 3(b) and (c) and Fig. S5, ESI.† For plane, light will be first reflected and refracted, when it reaches the upper surface. None of the reflected light is absorbed by plane. While the light refracted inside plane will be reflected a second time and refracted on the lower surface. The second refracted light eventually penetrates plane and becomes transmitted light. When the second reflected light reaches the upper surface, it will be reflected and refracted until it is completely absorbed inside plane. Overall, the intensity of reflected light and transmitted light, which are not absorbed, is obviously large on plane, meaning that only a small part of the light is converted into heat. Similarly, it will be first reflected and refracted when reaching the upper surface of MNF. However, light after first reflection will be reflected and refracted many times due to the presence of micro–nano structures on the surface of MNF, which is quite different from plane. Ultimately, the intensities of both reflected and transmitted light of MNF are reduced, indicating that the micro–nano structure on its upper surface can significantly improve light absorption efficiency. For HF, light refracted then will be reflected and refracted many times in the interior walls until the light is completely absorbed because of the existence of an internal hollow structure. Eventually, the intensity of transmitted light of HF is obviously reduced compared with plane, which means that the hollow structure inside the sample dominates the effect of reducing the intensity of transmission light. In addition, the intensities of both reflected light and transmitted light on MNHF are significantly lower than that of plane owing to the combination of a micro–nano structure on the surface and a hollow structure inside it. This result indicates that a double-bionic composite structure can facilitate the absorption of light and thus improve the photothermal conversion effect. Moreover, the hollow structure inside MNHF significantly reduces heat conduction because the coefficient of the thermal conductivity of air is much smaller than that of solid film, which inhibits heat transfer to the substrate and further enhances the utilization of heat.
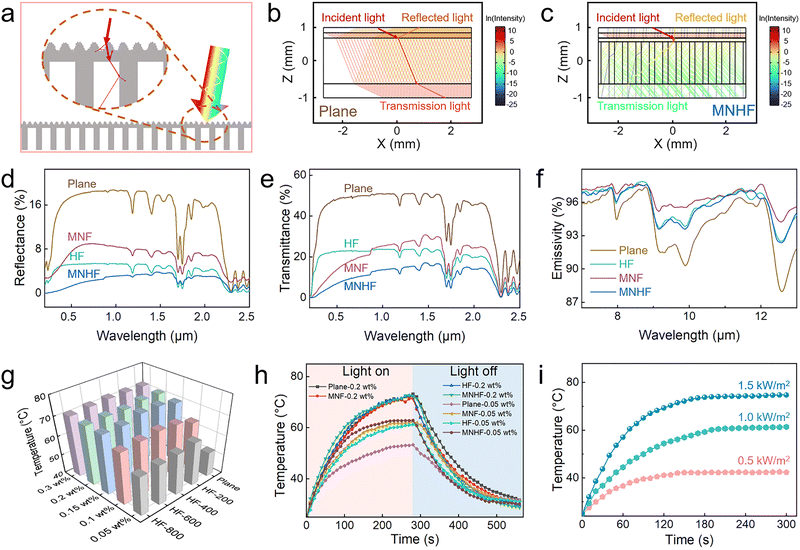 |
| Fig. 3 Photothermal properties of MNHF. (a) Schematic diagram of ray trace in MNHF. (b) Ray-tracing simulation results of plane. (c) Ray-tracing simulation results of MNHF. (d) The reflectance of plane, HF, MNF, and MNHF at wavelengths of 0.2–2.5 μm. (e) Transmittance of plane, HF, MNF, and MNHF at a wavelength of 0.2–2.5 μm. (f) Emissivity of plane, HF, MNF, and MNHF at a wavelength of 7–13 μm. (g) Temperature of HF with different MWCNT contents and hollow diameters under ten minutes’ illumination of 1 sun. (h) Surface temperature of different samples with 0.05 wt% and 0.2 wt% MWCNTs under conditions of light ON and light OFF successively. (i) Surface temperature of MNHF with 0.05 wt% MWCNTs under 0.5, 1, and 1.5 sun illumination. | |
In order to justify the above conclusions, the reflectance and transmittance of the bare plane, MNF, HF, and MNHF at wavelengths of 0.2–2.5 μm were tested (Fig. 3(d) and (e)). Compared with the other three samples, MNHF is proved to possess the minimum reflectance and transmittance. In the wavelength range 0.2–2.5 μm, the average reflectance and transmittance of MNHF decrease from 14.26% and 41.95% for plane to 2.35% and 10.25% of that in plane film, respectively. The lower reflectance and transmittance confirm the higher absorption of light by MNHF. This is very consistent with the simulation results.
Radiation is a significant method to transfer heat. Any object with a temperature above 0 K radiates and transfers heat to its surroundings at any time according to Kirchhoff's law.40 To analyze the heat radiation ability of different structures, especially to surrounding ice, the emissivity of plane, MNF, HF, and MNHF were tested at wavelengths of 7–13 μm. As shown in Fig. 3(f), the average emissivity of MNHF is 96.01%, which is higher than that of plane (94.68%), proving that the composite structure can radiate heat to surrounding ice better than plane. The synergistic effect of reduced heat conduction and enhanced thermal radiation properties allows rapid melting of frozen ice, resulting in the rapid deicing ability of the MNHF surface. Although the average emissivity of MNF is slightly higher than that of MNHF, the much higher light absorption capacity makes MNHF more desirable and outstanding. Looking at these two factors comprehensively, MNHF is considered to be the most promising candidate for photothermal deicing.
To further analyze the influence of photothermal material contents and hollow diameter sizes on the photothermal effect, the equilibrium temperature on the surfaces of different samples under 1 sun was recorded with an infrared thermal imager at an initial temperature of 25 °C (Fig. 3(g)). The x in HF-x indicates the diameter of the hollow microcolumn. The temperature difference between HF of different diameters is small, indicating that the size of the hollow diameter exerts no obvious influence on the equilibrium temperature. Moreover, the temperature of HF increases with an increase in the content of MWCNTs when the content of MWCNTs is less than 0.2 wt%. Part of the light is reflected and refracted many times in the hollow microcolumn until it is completely absorbed, contributing to a higher temperature than plane. Notably, when the content of MWCNTs exceeds 0.2 wt%, the temperature of HF tends to stabilize. This is because light cannot penetrate the planar layer to reach the inner walls of the hollow microcolumn. To put it simply, light is trapped only by a planar structure.
In order to compare the photothermal conversion ability of samples with different structures and MWCNT contents, the heating curves of MNHF under 1 sun and cooling curves after turning off the light source were obtained and are shown in Fig. 3(h). When the content of MWCNTs is set to 0.2 wt%, the temperature of all samples is almost the same due to the inability of light to penetrate the micro–nano structured layer. However, the hollow structure in MNHF can inhibit the heat conduction process between MNHF and the substrate material due to the low thermal conductivity of air, leading to less heat loss. Therefore, MNHF has a higher warming rate since more heat generated by photothermal conversion is stored. When the content of MWCNT is adjusted to 0.05 wt%, light is fully captured and utilized by the double-layer photothermal traps, which causes the temperature of MNHF to rise higher. The results show that MNHF with a low content of photothermal material has a more obvious effect on promoting the photothermal property. Moreover, the concentration of MWCNTs does not significantly affect CA or ice adhesion strength (Fig. S6, ESI†).
The photothermal conversion efficiency of a material, η, is defined as the ratio of heat generated by light-to-heat conversion of the material to input solar energy, and the calculation formula is as follows:22
|  | (3) |
where
Q is the heat generated by photothermal conversion of the material;
q is the input of solar power density, 100 mW cm
−2;
A is the surface area of MNHF, 4 cm
2. The detailed calculation process for
Q is described in supporting materials, and the values of the corresponding parameters are recorded in detail in Table S1 (ESI
†). By calculation, a low concentration of MNHF (0.05 wt%) increases
η from 22.42% of plane to 32.70% in 280 s, which increases the
η of plane by about 45.85%. Although the calculated
η of MNHF is not as high as that reported in the literature, the low cost of this design is obviously competitive and desirable in practical requirements, and we believe that the double-bionic structure proposed in this paper will achieve a higher temperature rise and
η when other photothermal materials with a higher
η are selected.
Considering that solar power density varies with time in practical application, the photothermal performance of MNHF at different power densities was further studied (Fig. 3(i)). As the solar power density increases from 0.5 kW m−2 to 1.5 kW m−2, the surface temperature of MNHF increases from 42.2 °C to 73.8 °C after 180 s of illumination, showing great photothermal properties under different sunshine conditions. To further confirm the anti-icing effect of MNHF, all samples were placed on a cooling table to simulate actual low-temperature conditions when thin films are attached onto metal surfaces (Fig. S7, ESI†). The initial temperature of the cooling table was 20 °C, and it was reduced at a rate of 0.1 °C s−1 until it reached −15 °C. The surface temperatures of plane and MNF are less than 0 °C after 10 min, which will directly cause freezing of droplets. However, the surface temperatures of HF and MNHF are higher than 0 °C due to weak thermal conductivity by the hollow microcolumn. Interestingly, the surface temperature of MNHF even goes up to 15.5 ± 0.8 °C, which means that MNHF can remain ice-free under the synergistic action of the micro–nano structure and hollow microcolumn structure. This indicates that MNHF has an admirable anti-icing effect under light illumination.
Photothermal deicing performance
Although MWCNTs can generate some heat to delay icing under sunlight irradiation, icing on photothermal superhydrophobic surfaces is inevitable even with sunlight in extremely cold environments.21 It is necessary to study the ice melting ability of different surfaces. Hence, photothermal deicing experiments were carried out on MNHF, MNF, and HF under 1 sun. Samples were first placed on a piece of thermal insulation foam. Ice cubes were frozen in a mold (25 mm × 25 mm × 1.5 mm). As shown in Fig. 4(a) and Movie S2, ESI,† ice on the surfaces of all three samples started to melt from the edge under 1 sun. However, only part of the melt water is removed from the HF surface after 600 s, and the surface temperature is 48.6 °C. Besides, melt water on the MNF surface is firmly trapped in the micro–nano structure, and the surface temperature is only 28.0 °C. However, the result on MNHF is obviously different. Benefiting from the combination of high photothermal conversion efficiency, low heat conduction, and increased thermal radiation, ice on the upper surface of MNHF is completely melted and removed after 519 s, and the surface temperature is up to 60.4 °C.
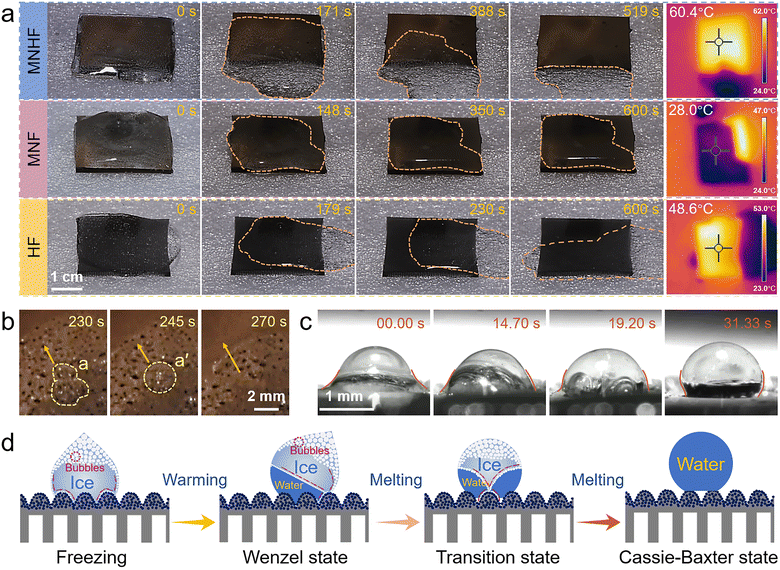 |
| Fig. 4 Deicing performance of MNHF. (a) Temperature distribution and optical photographs of a photothermal deicing experiment of a square ice plate under 1 sun. (b) Optical photographs of the movement of small air bubbles in a photothermal deicing experiment on an MNHF surface. (c) Optical pictures of the spontaneous recovery of a frozen droplet from the Wenzel state to the Cassie–Baxter state during the ice melting process. (d) Schematic diagram of the spontaneous recovery of a frozen droplet with photothermal effect from the Wenzel state to the Cassie–Baxter state. | |
During the ice melting process on the MNHF surface, the bottom part of the ice will quickly melt into water as the surface temperature rises, and the underlayer of melt water has a higher temperature, ascribed to the heat conductive principle. So, melt water will constantly move up via the Marangoni effect, where bubbles wrapped by surrounding water will also constantly move. These adjacent bubbles quickly merge into bigger ones and excess surface energy can be released within this process. Among bubble motions, some move around and continuously migrate to the melting area (Fig. 4(b)), while others have a downward impact on the micro–nano structure so that melt water can spontaneously recover from the Wenzel state to the Cassie–Baxter state (Fig. 4(c) and Movie S3, ESI†). A schematic diagram for the spontaneous recovery of a droplet to the Cassie–Baxter state during the ice melting process is shown in Fig. 4(d). Bubbles need to overcome the buoyancy Fb and fluid resistance Fd when they have a downwards impact on the micro–nano structure. The critical condition can be expressed as:38
|  | (4) |
where d
γ/d
T = 0.1 mN m
−1 K
−1; Δ
T is the temperature difference between the substrate material and the top of the non-melting area of the ice;
ρ is the density of water, 960 kg m
−3;
g is the gravity constant, 9.8 N kg
−1;
rbmax is the maximum bubble radius in downward movement;
vb is the bubble impact velocity; and
C is the drag coefficient of the fluid. It is not difficult to see that Δ
T is a prerequisite for the droplet to spontaneously recovery to the Cassie–Baxter state after the bubbles’ rapid impact on the micro–nano structure. All ice cubes on the three samples can be melted under the photothermal effect, but Δ
T on MNHF is the largest in the same short time. Therefore, a melt water droplet on MNHF can spontaneously recover from the Wenzel state to the Cassie–Baxter state, and can be removed under a given inclination angle in time (Fig. S8 and Movie S4, ESI
†).
Application and durability tests
A lighting environment does not always exist in practice. The anti-icing/deicing effect of photothermal superhydrophobic surfaces in non-light environments should also be considered. The superhydrophobic surface can only delay icing to some extent, but cannot completely inhibit icing.41 It is necessary to consider the ice adhesion strength (τice) after droplet freezing. A device for measuring ice adhesion strength and a schematic diagram of the measuring process are shown in Fig. S9, ESI.† When τice < 100 kPa, ice can be removed under the action of natural forces, such as gravity or wind.42 The adhesion strength of ice can be expressed as:16 |  | (5) |
where E* is the apparent elastic modulus, G is the surface energy, a is the crack length, and Λ is a dimensionless constant determined by the crack geometry.
To analyze the deicing effect and reusability of MNHF, variations in τice, CA, and SA in 10 icing–deicing cycles are shown in Fig. 5(a) and (b), respectively. The τice and SA both increased slowly, and CA decreased slightly as the number of icing–deicing cycles increased. Remarkably, τice remains as low as 1.84 ± 0.3 kPa even after 10 icing–deicing cycles, which is an ultra-low value in the reported literature.43 This is because the apparent elastic modulus of MNHF is low. Microcracks are produced due to inadaptability between elastomer and ice, according to eqn (5) (Fig. 5(c)), which further reduces the ice adhesion strength. Moreover, MNHF remains superhydrophobic with CA of 152.1° and SA of 5.5° even after 10 icing–deicing cycles, which contribute to a highly satisfactory photothermal deicing performance during the day. The ultra-low ice adhesion strength and great superhydrophobic robustness can meet the practical needs of anti-icing/deicing in a lightless environment.
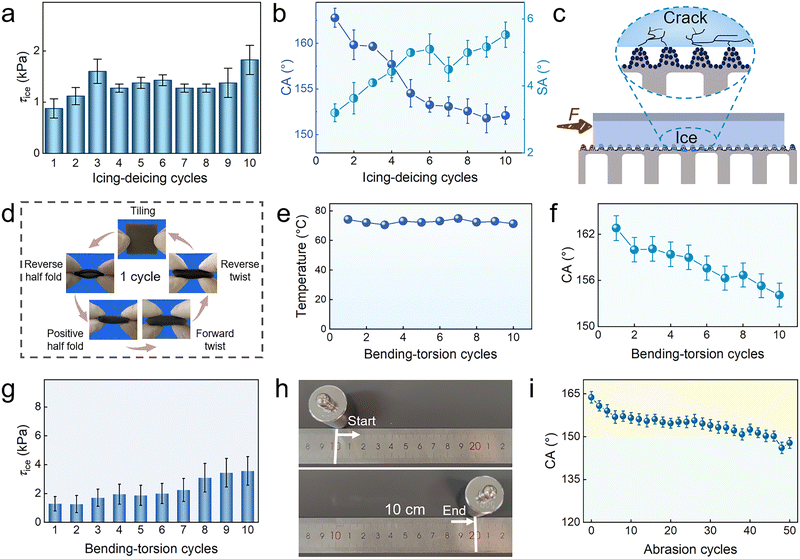 |
| Fig. 5 Super-robustness against mechanical abrasion. (a) Effect of icing–deicing cycles on ice adhesion strength. (b) Effect of icing–deicing cycles on CAs and SAs. (c) Schematic diagram of ice on the MNHF surface forming multiscale cracks under the action of an external force. (d) Illustration of one bending–torsion cycle. (e) The effect of bending–torsion cycles on equilibrium temperature. (f) The effect of bending–torsion cycles on CAs. (g) The effect of bending–torsion cycles on ice adhesion strength. (h) Illustration of one abrasion cycle. (i) The effect of abrasion cycles on CAs. | |
To investigate the flexibility of MNHF, 10 bending–torsion cycle experiments were carried out. One bending–torsion cycle is defined as bending MNHF forward and backward, then twisting it to left and right, and finally unfolding it (Fig. 5(d)). The equilibrium temperature, CA and ice adhesion strength of MNHF were tested after each cycle. As shown in Fig. 5(e)–(g), the equilibrium temperature, CA and ice adhesion strength of MNHF are only slightly decreased after 10 bending–torsion cycles. This indicates that the toughness of MNHF means it is capable of keeping its excellent photothermal effect, superhydrophobicity and ice-phobic properties even after many bending and folding cycles, which well proves the remarkable flexibility of MNHF.
Mechanical durability is an important factor limiting the practical application of superhydrophobic surfaces.44,45 To evaluate the mechanical durability of MNHF, sandpaper abrasion and tape peeling tests were carried out. MNHF was pasted on the bottom of a 100 g weight, and it was moved at a constant speed on 2000 mesh sandpaper, where a displacement of 10 cm was defined as one abrasion cycle (Fig. 5(h)). As shown in Fig. 5(i), although the CA of MNHF gradually decreased with an increase in abrasion cycles, it can still exceed 150° up to 46 abrasion cycles, indicating the excellent mechanical durability of MNHF. Moreover, in tape peeling experiments which consisted of tape adhesion and tape peeling (Fig. 6(a)), the CAs of MNHF after each 100 peeling cycles were recorded within 500 peeling cycles (Fig. 6(b)). The results show that the CA of MNHF can still reach 153.5° even after 500 peeling cycles. What is more, water jet impinging tests (the volume velocity of the water jet was 36 mL min−1) were subsequently conducted on MNHF samples before and after 500 peeling cycles to further analyze the extent of wear (Fig. 6(c)). The incidence angle and reflex angle on the initial MNHF surface are 17° and 24°, respectively. Meanwhile, the incidence angle and reflex angle on MNHF after 500 peeling cycles are 16° and 29°, respectively. The difference between incidence angle and reflex angle Δα increased slightly from 7° to 13° as the peeling cycles increased to 500 (Fig. 6(d)), which further indicates the excellent mechanical durability of MNHF.
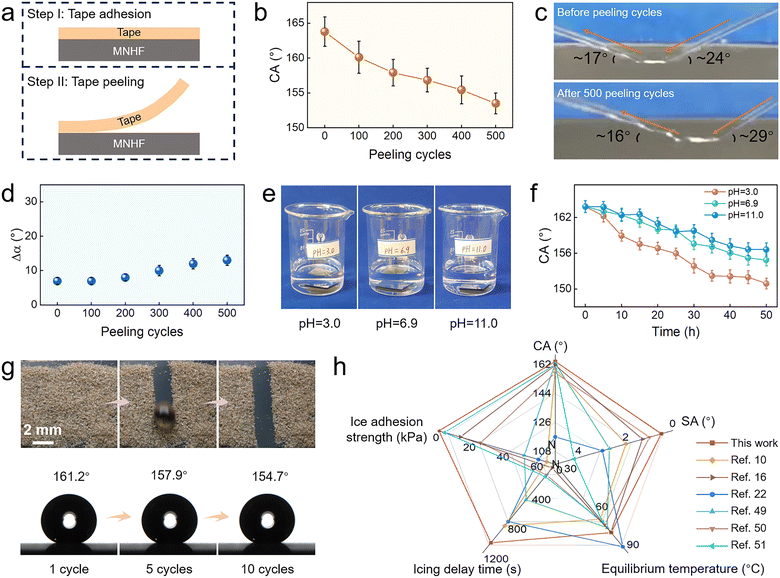 |
| Fig. 6 The stability of MNHF. (a) Illustration of one tape peeling cycle. (b) The effect of tape peeling cycles on CAs. (c) The deflection of a water jet from an MNHF surface before and after 500 peeling cycles. (d) The effect of tape peeling cycles on Δα. (e) Optical images of an MNHF surface immersed in solutions with different pH. (f) Variation of CAs of MNHF after being immersed in different solutions for different times. (g) Optical images of self-cleaning and water contact angle images after 1, 5, and 10 self-cleaning cycles. (h) Radar map of photothermal anti-icing and deicing performance of MNHF compared with other similar work. | |
Airplanes often encounter impacts of droplets with different pH values during flight.46 In order to test the hydrophobic properties of the MNHF surface when encountering droplets with different pH values, droplets with pH values of 3.0, 6.9, and 11.0 were placed on the surface of MNHF (Fig. S10, ESI†). All these three droplets are in an idea superhydrophobic state, which confirms the excellent superhydrophobicity of MNHF regardless of pH value. To further analyze the chemical stability of MNHF, three MNHF samples were soaked in solutions at pH values of 3.0, 6.9, and 11.0 (Fig. 6(e), ESI†). The three MNHF samples were all taken out after every 5 h and the CAs were tested after they were dried. As shown in Fig. 5(f), the CAs of the three surfaces are 150.9°, 156.6°, and 154.9° even after 50 h of soaking, which are all higher than 150°. This result strongly proves the excellent chemical stability of MNHF.
In nature, surface contamination will not only weaken the photothermal property of functional film surfaces, but also further trigger the heterogeneous nucleation of droplets.47,48 Therefore, self-cleaning performance is of great importance to a photothermal superhydrophobic anti-icing/deicing strategy. Thus, 10 repeats of sand covering and self-cleaning experiments on MNHF were carried out to verify its self-cleaning performance. The results are shown in Fig. 5(g) and Movie S5, ESI.† The sand was removed from the surface after dropping 10 μL droplets under the combination of superhydrophobicity and low water adhesion. The surface of MNHF became completely clean. In addition, the CA of MNHF suffered only a tiny decline to 154.7° after 10 self-cleaning cycles, which further confirms its highly satisfactory stability and reusability.
In order to comprehensively evaluate the MNHF, the CA, SA, equilibrium temperature, icing delay time, and ice adhesion strength were selected for comparison with other similar work.10,16,22,49–51 As shown in Fig. 5(h), although the equilibrium temperature of MNHF is slightly lower than that of ref. 22, its wettability and de-icing performance are much better than those in other literature. In particular, the ultra-low ice adhesion strength of MNHF is at a greatly desirable level among all these pieces of literature. Conclusively, the excellent superhydrophobicity, good photothermal conversion performance, long icing delay time and ultra-low ice adhesion strength enable MNHF to have great development potential in the field of photothermal anti-icing/deicing of airplane wings.
Conclusions
In this attempt, inspired by the compound eyes of mosquito and insulation hollow hairs of polar bear, we have developed an MNHF with excellent photothermal anti-icing/deicing properties. The synergistic effect of micro–nano structures and a hollow microcolumn structure has been confirmed to greatly enhance light absorption and thermal radiation, as demonstrated by both theoretical simulations and experimental results. Notably, the photothermal conversion efficiency of MNHF with a photothermal material concentration of 0.05 wt% is 45.85% higher than that of planar photothermal films. Under a solar power density of 1.5 kW m−2, the equilibrium temperature of MNHF can reach 73.8 °C. In addition, the combination of photothermal effects and superhydrophobicity greatly reduces the damage to the micro–nano structure during the deicing process, allowing for rapid melting of ice at the bottom and removal of the ice–water mixture. A low elastic modulus and the presence of microcracks decrease ice adhesion strength. Moreover, the ice adhesion strength remains 1.8 ± 0.3 kPa even after 10 icing–deicing cycles, confirming the admirable reusability of MNHF. The film's soft characteristic enables it to preserve its excellent photothermal and superhydrophobic properties after bending and twisting. Additionally, the exceptional chemical stability and self-cleaning characteristics of MNHF ensure its functionality under harsh applied conditions. With its superior superhydrophobic properties, outstanding photothermal conversion efficiency, remarkable anti-icing performance, and exceptional deicing ability, MNHF shows tremendous application potential in the anti-icing/deicing of airplanes.
Experimental section
Materials
The thickness of PSM was 200 μm. PDMS containing prepolymer and curing agent was provided by Dow Corning. MWCNTs within 3–5 nm in inner diameter, 8–15 nm in outer diameter, and 3–12 μm in length were purchased from SHENGHUI SHIYE, Beijing, China. FENGCHUANJIU release agent 184 was provided by Wuxi Fites Electronic Technology Co., Ltd, Jiangsu, China. Ultrapure water was made using a UPRO-500L/H ultrapure water system. All chemicals were used directly without further treatment.
Fabrication of microcolumn templates with high aspect ratio
Hexagonally distributed through holes with diameters of 200, 400, 600, and 800 μm were fabricated on PSMs by a femtosecond laser processing system (SolsticeAce100F-1KHP). Then, films were cut into 60 mm × 60 mm and washed in an ultrasonic cleaner (GS-010A) for 10 min. Microporous templates were obtained after heating for 10 s by a hot air gun (F3) at 400 °C. The PDMS prepolymer and curing agent mixture (10
:
1) were poured into microporous templates then the air between the mixture and microporous templates was removed by vacuuming. Finally, they were dried at 80 °C for 2 h to obtain microcolumn templates with a high aspect ratio.
Fabrication of MNHF
The microcolumn template was sprayed with a release agent to facilitate demolding. Different contents of MWCNTs were added to PDMS prepolymer and dispersed evenly by an ultrasonic oscillator for 2 h, and a curing agent (10 wt% of the prepolymer) was added subsequently. Next, the mixture was poured into a microcolumn template to prepare an HF. Finally, an MNHF with a size of 25 mm × 25 mm was fabricated by femtosecond laser ablation on the upper surface of HF.
Characterization
The microscopic morphology of the sample surfaces was observed by a field emission scanning electron microscope system (ultra55). Element content was analyzed with an energy dispersive spectrometer (Oxford Xplore 30). The contact angle (CA) and sliding angle (SA) were measured with a contact angle measurement system (SDC-350). CAs and SAs were randomly measured at three different positions to obtain average values. The 3D surface topography was analyzed with an optical profiler (Bruker Counter GT K 3D). The reflectance and transmittance were measured with a UV-Vis-NIR spectrophotometer (UV-3700). Emissivity was analyzed with an FTIR spectrometer with a gold integrating sphere (iS50). A solar simulator (71S603A) was used to simulate sunlight. Samples were placed vertically at 45 cm below the light outlet of the solar simulator, and tests were carried out under a light spot of 160 cm × 160 cm. Surface temperatures were recorded by an infrared detector (i7).
Anti-icing and deicing experiments
A cooling table was made from a power module and a cryostat. In anti-icing experiments, the relative humidity of the surrounding air was 65% and the temperature of the cooling table could rapidly decrease from 21 °C to −40 °C within 240 s after turning on the power (Fig. S11, ESI†). A droplet with a volume of 10 μL was placed on the surface of a sample which was placed on the cooling table. The icing process was recorded with a digital camera (RX100III). The icing time, which was defined as the time from when the icing table was powered on to when droplet froze completely, was recorded. In deicing experiments, the self-made ice adhesion testing system consisted of a thrust meter (SH-III-10N) and a refrigerator with an environmental temperature of −20 °C and relative humidity of 55%. A hollow tube mold (5 mm × 5 mm × 50 mm) was placed above the sample. Then, water was added to the mold and frozen in a refrigerator for 12 h. Finally, the icicle in the mold was pushed down at a constant speed by the thrust meter, and the ice adhesion strength was calculated.
Author contributions
S. X., H. Y., and G. L. conceived the idea and designed the project. S. X., H. Y., Y. Y., Y. W., and J. L. fabricated the samples. S. X., H. Y., and T. W. performed the characterization. S. X., H. Y., G. L., S. L., X. L., Y. S., and K. Y. completed the data analysis and figure depiction. S. X. and H. Y. wrote the manuscript and all authors contributed to its final contents.
Data availability statements
The data supporting this article have been included as part of the ESI.†
Conflicts of interest
There are no conflicts to declare.
Acknowledgements
This research was supported by the National Key R&D Program of China (2023YFB4604200), National Natural Science Foundation of China (grant no. 52222513, 52075557, 22075202), Open Fund of Key Laboratory of Icing and Anti/Deicing (IADL20210408, IADL20220405), Sichuan Science and Technology Program (2022JDRC0028, 2023NSFSC0853, 2024NSFSC0898), Research Programs Funded by Frontier Project, Natural Science Foundation of Southwest University of Science and Technology (21zx7136), Central South University Innovation-Driven Research Programme (grant no. 2023CXQD019), State Key Laboratory of Precision Manufacturing for Extreme Service Performance, Central South University (ZZYJKT2023-12). The authors would like to thank you Shiyanjia Lab (https://www.shiyanjia.com) for theoretical simulations.
Notes and references
- Z. Tian, L. Wang, D. Zhu, C. Chen, H. Zhao, R. Peng, H. Zhang, P. Fan and M. Zhong, ACS Appl. Mater. Interfaces, 2023, 15, 6013–6024 CrossRef CAS PubMed.
- D. Zeng, Y. Li, H. Liu, Y. Yang, L. Peng, C. Zhu and N. Zhao, Colloids Surf., A, 2023, 660, 130824 CrossRef CAS.
- S. Wang, S. Chang, H. Qi and H. Zhao, Appl. Therm. Eng., 2024, 238, 122045 CrossRef CAS.
- X. Gong, H. Yu, X. Chen, P. Xu and H. Wang, Mater. Today Phys., 2023, 34, 101076 CrossRef CAS.
- S. Cheng, P. Guo, X. Wang, P. Che, X. Han, R. Jin, L. Heng and L. Jiang, Chem. Eng. J., 2022, 431, 133411 CrossRef CAS.
- T. Hao, D. Wang, X. Chen, A. Jazzar, P. Shi, C. Li, H. Wang, X. He and Z. He, Appl. Phys. Rev., 2023, 10, 021317 CAS.
- Z. Xie, H. Wang, Y. Geng, M. Li, Q. Deng, Y. Tian, R. Chen, X. Zhu and Q. Liao, ACS Appl. Mater. Interfaces, 2021, 13, 48308–48321 CrossRef CAS PubMed.
- J. L. Laforte, M. A. Allaire and J. Laflamme, Atmos. Res., 1998, 46, 143–158 CrossRef.
- M. H. Nazari, S. Z. Mousavi, A. Potapova, J. McIntyre and X. Shi, Water Environ. Res., 2021, 93, 1855–1881 CrossRef PubMed.
- B. Wang, P. Yu, Q. Yang, Z. Jing, W. Wang, P. Li, X. Tong, F. Lin, D. Wang, G. E. Lio, R. Caputo, O. Ávalos-Ovando, A. O. Govorov, H. Xu and Z. M. Wang, Mater. Today Phys., 2022, 24, 100683 CrossRef CAS.
- L. Zhu, M. Gao, C. K. N. Peh and G. W. Ho, Mater. Horiz., 2018, 5, 323–343 RSC.
- H. Xiang, Y. Yuan, T. Zhu, X. Dai, C. Zhang, Y. Gai and R. Liao, Mater. Today Phys., 2023, 35, 101137 CrossRef CAS.
- Y. Sun, X. Han, P. Guo, Z. Chai, J. Yue, Y. Su, S. Tan, X. Sun, L. Jiang and L. Heng, ACS Nano, 2023, 17, 12616–12628 CrossRef CAS PubMed.
- P. Guo, Z. Teng, X. Han, Y. Sun, R. Jin, L. Jiang and L. Heng, Chem. Eng. J., 2023, 471, 144518 CrossRef CAS.
- F. Wang, Y. Zhuo, Z. He, S. Xiao, J. He and Z. Zhang, Adv. Sci., 2021, 8, 2101163 CrossRef PubMed.
- C. Chen, Z. Tian, X. Luo, G. Jiang, X. Hu, L. Wang, R. Peng, H. Zhang and M. Zhong, Chem. Eng. J., 2022, 450, 137936 CrossRef CAS.
- W. Li, Y. Zhan and S. Yu, Prog. Org. Coat., 2021, 152, 106117 CrossRef CAS.
- W. Huang, J. Huang, Z. Guo and W. Liu, Adv. Colloid Interface Sci., 2022, 304, 102658 CrossRef CAS PubMed.
- W. S. Y. Wong, K. I. Hegner, V. Donadei, L. Hauer, A. Naga and D. Vollmer, Nano Lett., 2020, 20, 8508–8515 CrossRef CAS PubMed.
- C. Liu, Y. Li, C. Lu, Y. Liu, S. Feng and Y. Liu, ACS Appl. Mater. Interfaces, 2020, 12, 25471–25477, DOI:10.1021/acsami.0c05954.
- H. Y. Erbil, Langmuir, 2020, 36, 2493–2509 CrossRef CAS PubMed.
- Z. Xie, H. Wang, M. Li, Y. Tian, Q. Deng, R. Chen, X. Zhu and Q. Liao, Chem. Eng. J., 2022, 435, 135025 CrossRef CAS.
- N. Li, Y. Zhang, H. Zhi, J. Tang, Y. Shao, L. Yang, T. Sun, H. Liu and G. Xue, Chem. Eng. J., 2022, 429, 132183, DOI:10.1016/j.cej.2021.132183.
- X. Lai, J. Hu and J. Qu, Carbon, 2023, 212, 118155 CrossRef CAS.
- R. Li, L. Zhang, L. Shi and P. Wang, ACS Nano, 2017, 11, 3752–3759 CrossRef CAS PubMed.
- R. E. Grojean, J. A. Sousa and M. C. Henry, Appl. Opt., 1980, 19, 339–346 CrossRef CAS PubMed.
- Q. Wang, J. H. He and Z. Liu, Front. Bioeng. Biotechnol., 2022, 10, 926253, DOI:10.3389/fbioe.2022.926253.
- H. Li, Y. Li, J. Wu, X. Jia, J. Yang, D. Shao, L. Feng, S. Wang and H. Song, ACS Appl. Mater. Interfaces, 2022, 14, 29302–29314 CrossRef CAS PubMed.
- S. Dou, H. Xu, J. Zhao, K. Zhang, N. Li, Y. Lin, L. Pan and Y. Li, Adv. Mater., 2021, 33, 2000697 CrossRef CAS PubMed.
- X. He, G. Li, Y. Zhang, X. Lai, M. Zhou, L. Xiao, X. Tang, Y. Hu, H. Liu, Y. Yang, Y. Cai, L. Guo, S. Liu and W. Zhao, Chem. Eng. J., 2021, 416, 129113 CrossRef CAS.
- Y. Wang, D. Zhang, J. Deng, F. Zhou, Z. Duan, Q. Su and S. Pang, ACS Sustainable Chem. Eng., 2019, 7, 3883–3894 CrossRef CAS.
- W. Zhao, L. Xiao, X. He, Z. Cui, J. Fang, C. Zhang, X. Li, G. Li, L. Zhong and Y. Zhang, Opt. Laser Technol., 2021, 141, 107115 CrossRef CAS.
- L. Wang, K. Yin, Q. Deng, Q. Huang, J. He and J. A. Duan, Adv. Sci., 2022, 9, 2204891 CrossRef CAS PubMed.
- Q. Huang, K. Yin, L. Wang, Q. Deng and C. J. Arnusch, Nanoscale, 2023, 15, 11247–11254 RSC.
- J. Pei, K. Yin, T. Wu, L. Wang, Q. Deng, Y. Huang, K. Wang and C. J. Arnusch, Nanoscale, 2023, 15, 15708–15716 RSC.
- Q. Deng, K. Yin, L. Wang, H. Zhang, Q. Huang, Z. Luo, J. He and J. A. Duan, Small Methods, 2023, 7, 2300290 CrossRef CAS PubMed.
- Y. Cui, H. Gong, Y. Wang, D. Li and H. Bai, Adv. Mater., 2018, 30, 1706807 CrossRef PubMed.
- L. Wang, Z. Tian, G. Jiang, X. Luo, C. Chen, X. Hu, H. Zhang and M. Zhong, Nat. Commun., 2022, 13, 378 CrossRef CAS PubMed.
- G. S. Watson, D. W. Green, L. Schwarzkopf, X. Li, B. W. Cribb, S. Myhra and J. A. Watson, Acta Biomater., 2015, 21, 109–122 CrossRef CAS PubMed.
- M. He, B. Zhao, X. Yue, Y. Chen, F. Qiu and T. Zhang, Nano Energy, 2023, 116, 108821 CrossRef CAS.
- Y. Cheng, Y. Wang, X. Zhang, J. Zhang, Z. He, J. Wang and J. Zhang, Nano Res., 2023, 16, 7171–7179 CrossRef CAS.
- W. Niu, G. Y. Chen, H. Xu, X. Liu and J. Sun, Adv. Mater., 2022, 34, 2108232 CrossRef CAS PubMed.
- S. Xuan, H. Yin, G. Li, Z. Zhang, Y. Jiao, Z. Liao, J. Li, S. Liu, Y. Wang, C. Tang, W. Wu, G. Li and K. Yin, ACS Nano, 2023, 17, 21749–21760 CrossRef PubMed.
- W. Zhang, D. Wang, Z. Sun, J. Songa and X. Deng, Chem. Soc. Rev., 2021, 50, 4031–4061 RSC.
- A. Allahdini, R. Jafari and G. Momen, Prog. Org. Coat., 2022, 165, 106758 CrossRef CAS.
- L. Wang, Z. Tian, X. Luo, C. Chen, G. Jiang, X. Hu, R. Peng, H. Zhang and M. Zhong, Nano Res., 2023, 16, 3267–3277 CrossRef CAS.
- L. Wang, J. Li, Z. Chen, Z. Song, X. Meng and X. Chen, Adv. Mater. Interfaces, 2022, 9, 2201758 CrossRef CAS.
- X. Xiao, X. Wei, J. Wei and J. Wang, Sol. Energy Mater. Sol. Cells, 2023, 256, 112313 CrossRef CAS.
- H. Xie, J. Wei, S. Duan, Q. Zhu, Y. Yang, K. Chen, J. Zhang, L. Li and J. Zhang, Chem. Eng. J., 2022, 428, 132585 CrossRef CAS.
- C. H. Xue, H. G. Li, X. J. Guo, Y. R. Ding, B. Y. Liu, Q. F. An and Y. Zhou, Chem. Eng. J., 2021, 424, 130553 CrossRef CAS.
- D. Li, L. Ma, B. Zhang and S. Chen, Chem. Eng. J., 2022, 450, 138429 CrossRef CAS.
|
This journal is © The Royal Society of Chemistry 2024 |