DOI:
10.1039/D3MD00580A
(Research Article)
RSC Med. Chem., 2024,
15, 234-253
The development of thymol–isatin hybrids as broad-spectrum antibacterial agents with potent anti-MRSA activity†
Received
16th October 2023
, Accepted 17th November 2023
First published on 20th November 2023
Abstract
Bacterial resistance toward available therapeutic agents has become a nightmare for the healthcare system, causing significant mortality as well as prolonged hospitalization, thereby needing the urgent attention of research groups working on antimicrobial drug development worldwide. Molecular hybridization is a well-established tool for developing multifunctional compounds to tackle drug resistance. Inspired by the antibacterial profiles of isatin and thymol, along with the efficiency of a triazole linker in molecular hybridization, herein, we report the design, synthesis and antibacterial activity of a novel series of triazole tethered thymol–isatin hybrids. Most of the hybrids exhibited a broad-spectrum antibacterial efficacy against standard human pathogenic as well as clinically isolated multidrug-resistant bacterial strains listed in the WHO's ‘priority pathogen’ list and also in the ESKAPE group. Among them, hybrid compound AS8 was the most effective against methicillin-resistant Staphylococcus aureus (MIC = 1.9 μM and MBC = 3.9 μM), exhibiting biofilm inhibitory potential. AS8 exhibited dehydrosqualene synthase (CrtM) inhibitory potential in MRSA and decreased the production of virulence factor staphyloxanthin, which is one of the key mechanisms of its anti-MRSA efficacy, which was further supported by molecular docking and simulation studies. Moreover, AS8 was found to be non-toxic and showed a potent in vivo antibacterial efficacy (90% survival at 10 mg kg−1) as well as a modulated immune response in the larva-based (Galleria mellonella) model of systemic infections. Overall findings confirmed that AS8 can be a promising candidate or take the lead in the treatment and further drug development against drug-resistant infectious diseases, especially against MRSA infections.
1. Introduction
Antimicrobial resistance is an emerging threat to human health and poses a significant burden on the healthcare system across the globe owing to a high mortality, prolonged hospitalization, and elevated medical cost.1–3 Approximately 10 million people are expected to die annually by 2050 due to microbial resistance toward available antimicrobial agents if the situation is not dealt with utmost importance.4 In 2017, the World Health Organization (WHO) published a list of ‘priority pathogens’ that are resistant to currently available antibacterial agents and need new antibiotics and immediate attention from drug development authorities around the globe.5 Methicillin-resistant Staphylococcus aureus (MRSA) is one among the list and is identified as a high priority pathogen and a member of antimicrobial-resistant ESKAPE (Enterococcus faecium, Staphylococcus aureus, Klebsiella pneumoniae, Acinetobacter baumannii, Pseudomonas aeruginosa, and Enterobacter species) pathogens. In 1961, MRSA was reported as a prevalent pathogen in healthcare settings, while in 2019, the Centre for Disease Control and Prevention (CDC) listed MRSA as a serious threat.6,7 It is one of the prime community-acquired and hospital-acquired pathogens responsible for mild (boils, sores, impetigo, lesions and abscesses) to life-threatening systemic infections (bacteremia, meningo-encephalitis, pneumonia, pyomyositis, pericarditis, endocarditis, osteomyelitis, toxic shock syndrome and sepsis).8,9 Thus, there is a global emergency to develop a new class of anti-MRSA agents to tackle the situation.
MRSA is a well-known biofilm producer that makes it more persistent in nature and its treatment more difficult in systemic infections.10 Besides, MRSA synthesizes numerous virulence factors that defend it from unfavourable environmental conditions and promote its survival. Among them, staphyloxanthin (a golden coloured membrane-bound carotenoid pigment) is a hallmark virulence factor whose production is confined to the strains of Staphylococcus aureus, which is responsible for the golden colour as well as the nomenclature of the bacterium as the meaning of ‘aureus’ is golden in Latin.11,12 Staphyloxanthin is located in the cell membrane of MRSA.13 It acts as an internal antioxidant that protects MRSA from host-mediated immune response.14,15 In the membrane, it provides structural integrity to MRSA that promotes survival in harsh environmental conditions.13 Staphyloxanthin is synthesized by the functioning of a series of enzymes encoded by the crtOPQMN operon. In the biosynthesis pathway of staphyloxanthin, dehydrosqualene synthase (CrtM) catalyses the first step of condensation of two farnesyl diphosphate (FPP) molecules to dehydrosqualene that followed subsequent steps catalysed by CrtN, CrtP, and CrtQ, which sequentially leads to the formation of 4,4-diapophytoene, 4,4-diaponeutosporene, 4,4-diaponeurosporenic acid, glucosyl-4,4-diaponeurosporenic acid, and subsequently staphyloxanthin at the end. Thus, CrtM inhibition is one of the key strategies to develop anti-Staphylococcus agents.13,16–18 Oldfield's group initially identified important CrtM inhibitors (Fig. 1) that established CrtM as an important target for tacking Staphylococcus resistance.19 However, various research groups are working on the development of CrtM inhibitors and have reported several important molecules based on different pharmacophores, but they did not explore their potential against biofilms or systemic infections.20–22 Therefore, rigorous efforts are needed to develop CrtM inhibitors with anti-bacterial as well as antibiofilm capabilities.
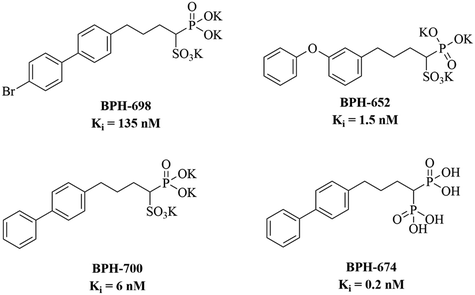 |
| Fig. 1 CrtM inhibitors initially identified by Oldfield's group. | |
Thymol (2-isopropyl-5-methylphenol) is a biologically important monoterpene alcohol, first isolated by Neumann from Thymus vulgaris in 1719.23 It is a major bioactive constituent in essential oils of various plants of genera Thymus, Origanum, and Carum belong to the Lamiaceae family.24,25 Thymol has been observed to have CrtM inhibition properties in MRSA but is devoid of antibacterial efficacy at the same dose level (100 mg mL−1).23 However, at the same time, thymol is also reported to possess antibiofilm efficacy against MRSA at the dose level of 512 μg mL−1.26 Thus, it can act as a key pharmacophore in designing potential anti-MRSA agents. Similarly, isatin (2,3-indolinedione) is an indole derivative with a wide range of biological activities and widely distributed among natural products, especially in fungal metabolites, plants and marine natural products, as well as in human urine and brain.27–29 Numerous reports are available in the literature describing the antibacterial (1, 2) as well as antibiofilm efficacy (3) of its derivatives against MRSA (Fig. 2).30–32
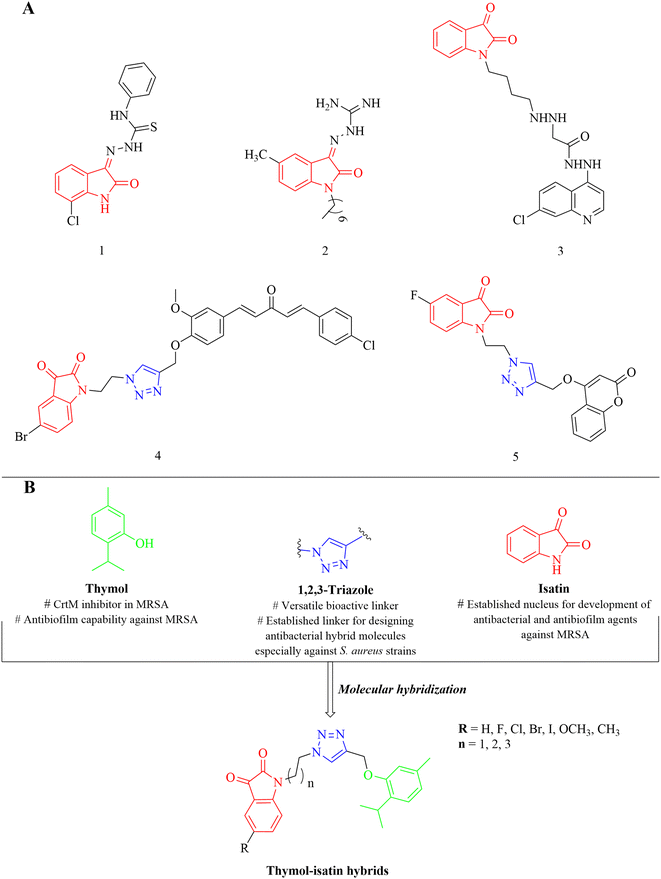 |
| Fig. 2 A. Previously reported isatin-based derivatives possessing antibacterial (1 and 2) and antibiofilm efficacy (3) against MRSA and triazole-linked isatin efficacy against Staphylococcus aureus (4 and 5); B. the design strategy for the proposed thymol–isatin based hybrids. | |
Molecular hybridization is a well-established stratagem in which two or more pharmacophores are clubbed together for generating desired hybrid molecule that possess the biological efficacy of its parent pharmacophores with enhanced potency and reduced toxicity along with lower drug resistance and eliminated drug–drug interactions.33 1,2,3-Triazole is a widely popular and versatile nucleus in medicinal chemistry, which is efficiently used as a linker for the development of hybrid molecules with a wide range of biological activities.27 Previously, we have reported isatin-containing triazole-linked hybrid molecules (4, 5) that were effective against Staphylococcus aureus (Fig. 2).27,28 CrtM inhibitory potential of thymol along with anti-MRSA and antibiofilm efficacy of isatin-containing derivatives as well as applicability of triazole ring in the development of antibacterial hybrids makes a perfect blend of these three moieties to be utilized in anti-MRSA drug design. Keeping in mind the pharmacological efficacy of thymol, isatin, and triazole moieties as well as urgent need of new anti-MRSA agents, we have rationally designed triazole-tethered thymol–isatin hybrid molecules (Fig. 2) as potential antibacterial agents, especially against MRSA. The designed hybrid molecules were synthesized and evaluated for their antibacterial efficacy against a panel of standard as well as clinically isolated human pathogenic bacterial strains that include bacterial strains from the ESKAPE pathogens group as well as WHO priority pathogens list and MRSA, respectively. The most active hybrid molecule against MRSA was further subjected to a series of biological tests for the evaluation of its anti-biofilm efficacy and to gain mechanistic insights. Anti-infective efficacy was also evaluated via the larva (Galleria mellonella)-based in vivo model.
2. Results and discussion
2.1. Chemistry
The synthesis of designed hybrid molecules was performed via a series of chemical reactions (Scheme 1), initialized from isatins. Various substituted isatins (6) were treated with dibromoalkanes in the presence of K2CO3 at 25 °C using DMF as the solvent to get various N-bromoalkyl isatins (7), which were further treated with NaN3 at 25 °C in DMF to get the desired N-azidoalkyl isatins (8) characterized from the triplets of alkyl chain hydrogens around 3.91–3.63 ppm with J values of 5 Hz. Simultaneously, thymol (9) was reacted with propargyl bromide in the presence of K2CO3 at 25 °C in DMF to get 1-isopropyl-4-methyl-2-(prop-2-yn-1-yloxy)benzene (propargylated thymol) (10) characterized by the appearance of two doublets at 7.11–7.10 ppm and 6.80–6.78 ppm for two individual flagpole protons present on the 3rd and 4th position of thymol nucleus. Finally, N-azidoalkyl isatins were treated with propargylated thymol in the presence of a catalytic amount of copper sulfate and sodium ascorbate as the reducing agent at 25 °C in DMF to yield the desired isatin–thymol hybrids (AS1–AS18) via copper-catalysed alkyne–azide cycloaddition (click chemistry approach), characterized from the singlet of triazole hydrogen at about 8.30–7.76 ppm. All reactions proceeded smoothly, and NMR and mass spectroscopy (MS) data along with elemental analysis were corresponding to the assumed structures of the hybrids.
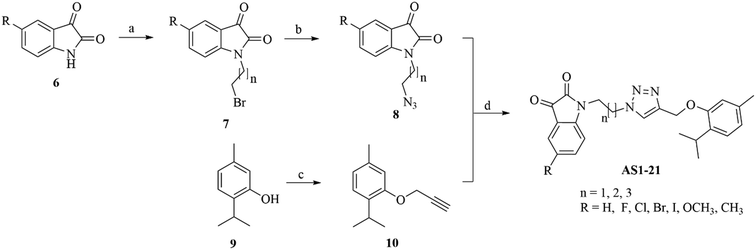 |
| Scheme 1 The synthesis of triazole-linked thymol–isatin hybrids. Reagents and conditions: (a) dibromoalkane, K2CO3, DMF, 0.5 to 2 h, stir, rt; (b) NaN3, DMF, 1 to 2 h, stir, rt; (c) propargyl bromide, K2CO3, DMF, 0.5 to 4 h, stir, rt; (d) sodium ascorbate, CuSO4, DMF, 0.5 to 24 h, rt. | |
2.2. Antibacterial activity
All synthetics were preliminary evaluated for their antibacterial activity against seven pathogenic standard antibacterial strains as well as nine clinically isolated multidrug resistant bacterial isolates using the agar well diffusion method at a concentration level of 50 μM. Overall results revealed that hybrid molecules are broad spectrum in nature and were active against both Gram-positive as well as Gram-negative standards as well as multidrug resistant bacterial strains (Table 1). It is also worth to note that hybrid molecules are slightly more selective toward Staphylococcus over other bacterial strains. Against MRSA, the hybrid with unsubstituted isatin nucleus (AS8) showed the highest ZOI value of 28.84 ± 1.3 mm, followed by methyl (AS14: ZOI = 26.69 ± 1.9 mm) and methoxy (AS13: ZOI = 25.12 ± 1.7 mm) substitutions at the 5th position of the isatin moiety, respectively. Electronegative halogen substitutions on the 5th position of the isatin moiety including fluorine (AS9: ZOI = 22.18 ± 1.8 mm), chlorine (AS10: ZOI = 21.32 ± 1.2 mm), bromine (AS11: ZOI = 21.23 ± 1.3 mm), and iodine (AS12: ZOI = 20.21 ± 1.2 mm) seem to be unfavourable for the antibacterial efficacy of the structure. It is also worth to note that with the increase or decrease in chain length between isatin and triazole nucleus from three carbons, a decrease in the antibacterial efficacy of the structure was observed (AS8: ZOI = 28.84 ± 1.3 mm, AS1: ZOI = 20.23 ± 1.6 mm, AS15: ZOI = 19.64 ± 1.1 mm). In the case of electropositive substitutions (AS14: ZOI = 26.69 ± 1.9 mm > AS13: ZOI = 25.12 ± 1.7 mm), a slight increase in the electronegativity resulted in a decrease in the antibacterial efficacy while in the case of electronegative halogen substitutions (AS9: ZOI = 22.18 ± 1.8 mm > AS10: ZOI = 21.32 ± 1.2 > AS11: ZOI = 21.23 ± 1.3 mm > AS12: ZOI = 20.21 ± 1.2 mm), the antibacterial activity surprisingly increased with an increase in the electronegativity but by not much difference. The overall biological data established an exclusive structure activity relationship between the substitution pattern on the 5th position of isatin nucleus and chain length between the isatin and triazole moiety, and the preference order for R becomes H > CH3 > OCH3 > F > Cl > Br > I, and for the carbon chain length between isatin and triazole, it is n = 3 > 2 > 4. Hybrid molecules with ZOI values greater than 20 mm were considered as susceptible and subjected to the evaluation of MIC and MBC values. Among them, hybrid molecule with highest ZOI value (AS8) also showed the lowest MIC and MBC values of 1.9 μM and 3.9 μM against MRSA, respectively (Table 2). The time-kill assay suggested the dose-dependent effect of AS8 against MRSA. At a concentration level of 1/2 × MIC, it acts as a bacteriostatic, while at 1 × MIC and 2 × MIC concentration levels, it showed bactericidal characteristics33 (Fig. 3). The cytotoxic evaluation of AS8 against normal murine fibroblast cells (L929) suggested its IC50 value of 125 μM, which makes it highly selective for MRSA with a selectivity index of 65.78, thus making it safer for normal cells34 (Table 2).
Table 1 The preliminary antibacterial screening of thymol–isatin hybrids against standard human pathogenic and clinically isolated multidrug resistant bacterial strains showing the zone of inhibitions (ZOIs)
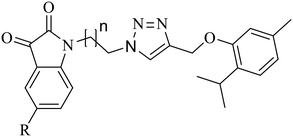
|
Compound |
R |
n
|
S.a.
|
cS.a.
|
S.e.
|
cMRSA |
MRSA |
E.f.
|
cE.f.
|
K.p.
|
cK.p.1
|
cK.p.2
|
E.c.
|
cE.c.1
|
cE.c.2
|
cE.co.
|
cA.b.
|
P.a.
|
Bacterial strains: S.a. = Staphylococcus aureus (MTCC 740), cS.a. = clinically isolated Staphylococcus aureus (RBRJ010), S.e. = Staphylococcus epidermidis (MTCC 435), cMRSA = clinically isolated methicillin resistant Staphylococcus aureus, MRSA = methicillin resistant Staphylococcus aureus (ATCC 33591), E.f. = Enterococcus faecalis (MTCC 439), cE.f. = clinically isolated Enterococcus faecalis (RBRJ015), K.p. = Klebsiella pneumoniae (MTCC 109), cK.p.1 = clinically isolated Klebsiella pneumoniae (RBRJ019), cK.p.2 = clinically isolated Klebsiella pneumoniae (RBRJ024), E.c. = Escherichia coli (MTCC 119), cE.c.1 = clinically isolated Escherichia coli (RBRJ005), cE.c.2 = clinically isolated Escherichia coli (RBRJ013), cE.co. = clinically isolated Enterobacter cloacae (RBRJ017), cA.b. = clinically isolated Acinetobacter baumannii (RBRJ027) and P.a. = Pseudomonas aeruginosa (MTCC 424). No zone of inhibition observed. |
AS1
|
H |
1 |
20.22 ± 1.6 |
22.23 ± 1.4 |
22.69 ± 1.2 |
20.23 ± 1.6 |
18.22 ± 1.4 |
15.36 ± 1.2 |
18.32 ± 1.4 |
18.32 ± 1.5 |
16.36 ± 1.4 |
16.49 ± 1.2 |
18.63 ± 1.4 |
15.21 ± 1.5 |
14.36 ± 1.6 |
16.54 ± 1.2 |
13.26 ± 1.4 |
18.24 ± 1.6 |
AS2
|
F |
1 |
20.27 ± 1.2 |
19.17 ± 1.8 |
17.32 ± 1.4 |
16.56 ± 1.5 |
15.27 ± 1.6 |
15.11 ± 1.5 |
19.27 ± 1.2 |
15.23 ± 1.8 |
14.23 ± 1.5 |
13.22 ± 1.9 |
13.29 ± 1.2 |
14.27 ± 1.4 |
14.21 ± 1.9 |
14.22 ± 1.9 |
11.94 ± 1.2 |
14.23 ± 1.5 |
AS3
|
Cl |
1 |
19.32 ± 1.9 |
19.56 ± 1.9 |
16.23 ± 1.2 |
14.26 ± 1.3 |
14.59 ± 1.2 |
11.23 ± 1.3 |
15.23 ± 1.3 |
15.08 ± 1.2 |
15.24 ± 1.3 |
13.59 ± 1.2 |
13.56 ± 1.3 |
13.21 ± 1.3 |
13.11 ± 1.2 |
14.24 ± 1.2 |
12.02 ± 1.3 |
14.07 ± 1.2 |
AS4
|
Br |
1 |
16.22 ± 1.7 |
16.36 ± 1.7 |
15.26 ± 1.7 |
14.22 ± 1.7 |
13.29 ± 1.8 |
13.56 ± 1.1 |
12.36 ± 1.1 |
13.22 ± 1.9 |
11.23 ± 1.2 |
12.26 ± 1.1 |
12.54 ± 1.2 |
12.53 ± 1.2 |
13.24 ± 1.1 |
13.27 ± 1.1 |
12.48 ± 1.2 |
13.97 ± 1.1 |
AS5
|
I |
1 |
15.23 ± 1.1 |
14.12 ± 1.5 |
13.45 ± 1.4 |
13.21 ± 1.2 |
13.11 ± 1.7 |
12.17 ± 1.2 |
12.89 ± 1.2 |
12.65 ± 1.1 |
11.59 ± 1.1 |
12.17 ± 1.3 |
11.27 ± 1.4 |
10.29 ± 1.4 |
11.26 ± 1.4 |
13.07 ± 1.3 |
12.11 ± 1.4 |
10.36 ± 1.1 |
AS6
|
OCH3 |
1 |
16.24 ± 1.4 |
20.26 ± 1.2 |
16.36 ± 1.8 |
15.29 ± 1.9 |
15.49 ± 1.3 |
15.48 ± 1.4 |
11.34 ± 1.9 |
15.27 ± 1.2 |
14.56 ± 1.2 |
14.87 ± 1.2 |
16.23 ± 1.2 |
11.54 ± 1.9 |
11.09 ± 1.6 |
13.25 ± 1.2 |
11.36 ± 1.5 |
11.49 ± 1.2 |
AS7
|
CH3 |
1 |
18.23 ± 1.3 |
21.25 ± 1.6 |
19.56 ± 1.3 |
16.23 ± 1.2 |
15.97 ± 1.8 |
14.27 ± 1.7 |
16.34 ± 1.7 |
16.56 ± 1.4 |
15.43 ± 1.5 |
15.69 ± 1.8 |
17.11 ± 1.9 |
12.65 ± 1.2 |
11.36 ± 1.2 |
14.25 ± 1.7 |
12.69 ± 1.8 |
11.26 ± 1.4 |
AS8
|
H |
2 |
28.57 ± 1.3 |
27.12 ± 1.7 |
25.49 ± 1.3 |
28.84 ± 1.3 |
27.48 ± 1.6 |
18.49 ± 1.3 |
20.24 ± 1.4 |
21.41 ± 1.1 |
20.22 ± 1.3 |
21.25 ± 1.1 |
22.11 ± 1.4 |
19.32 ± 1.3 |
20.27 ± 1.2 |
19.24 ± 1.8 |
18.33 ± 1.2 |
22.29 ± 1.4 |
AS9
|
F |
2 |
24.11 ± 1.7 |
23.21 ± 1.2 |
16.23 ± 1.2 |
22.18 ± 1.8 |
20.27 ± 1.7 |
16.41 ± 1.7 |
15.33 ± 1.2 |
15.22 ± 1.2 |
15.36 ± 1.2 |
14.26 ± 1.4 |
15.23 ± 1.2 |
16.24 ± 1.2 |
16.22 ± 1.3 |
15.27 ± 1.7 |
14.69 ± 1.3 |
15.96 ± 1.2 |
AS10
|
Cl |
2 |
22.14 ± 1.6 |
21.22 ± 1.3 |
18.23 ± 1.5 |
21.32 ± 1.2 |
20.52 ± 1.4 |
14.22 ± 1.2 |
14.11 ± 1.6 |
14.34 ± 1.6 |
15.13 ± 1.7 |
14.84 ± 1.5 |
14.22 ± 1.3 |
15.21 ± 1.7 |
15.48 ± 1.7 |
15.69 ± 1.6 |
13.21 ± 1.2 |
13.27 ± 1.7 |
AS11
|
Br |
2 |
21.23 ± 1.3 |
19.27 ± 1.1 |
17.22 ± 1.7 |
21.23 ± 1.3 |
21.48 ± 1.7 |
12.11 ± 1.6 |
12.23 ± 1.2 |
14.27 ± 1.7 |
13.25 ± 1.9 |
13.28 ± 1.1 |
14.12 ± 1.7 |
15.39 ± 1.9 |
15.47 ± 1.9 |
14.22 ± 1.9 |
11.24 ± 1.4 |
11.42 ± 1.9 |
AS12
|
I |
2 |
20.24 ± 1.1 |
19.02 ± 1.4 |
14.23 ± 1.2 |
20.21 ± 1.2 |
20.46 ± 1.4 |
11.65 ± 1.2 |
11.06 ± 1.9 |
12.26 ± 1.9 |
11.26 ± 1.2 |
12.69 ± 1.2 |
13.21 ± 1.2 |
12.32 ± 1.2 |
11.24 ± 1.2 |
13.69 ± 1.2 |
11.49 ± 1.5 |
11.64 ± 1.2 |
AS13
|
OCH3 |
2 |
25.32 ± 1.3 |
23.32 ± 1.3 |
15.69 ± 1.6 |
25.12 ± 1.7 |
25.29 ± 1.4 |
12.63 ± 1.8 |
11.27 ± 1.8 |
17.26 ± 1.2 |
18.09 ± 1.7 |
17.63 ± 1.6 |
16.54 ± 1.9 |
17.22 ± 1.4 |
15.41 ± 1.1 |
13.54 ± 1.1 |
12.26 ± 1.9 |
16.41 ± 1.8 |
AS14
|
CH3 |
2 |
26.11 ± 1.5 |
24.63 ± 1.2 |
22.21 ± 1.9 |
26.69 ± 1.9 |
24.13 ± 1.3 |
13.28 ± 1.9 |
13.36 ± 1.2 |
19.24 ± 1.7 |
18.46 ± 1.2 |
17.23 ± 1.7 |
16.54 ± 1.8 |
18.22 ± 1.2 |
17.63 ± 1.2 |
15.41 ± 1.5 |
13.09 ± 1.2 |
19.54 ± 1.2 |
AS15
|
H |
3 |
22.12 ± 1.5 |
16.23 ± 1.2 |
16.23 ± 1.9 |
19.64 ± 1.1 |
18.98 ± 1.5 |
13.21 ± 1.9 |
17.63 ± 1.2 |
15.21 ± 1.2 |
14.36 ± 1.2 |
15.63 ± 1.6 |
16.54 ± 1.6 |
13.56 ± 1.7 |
11.48 ± 1.1 |
12.58 ± 1.5 |
11.24 ± 1.2 |
13.74 ± 1.2 |
AS16
|
F |
3 |
19.21 ± 1.6 |
12.22 ± 1.7 |
15.11 ± 1.2 |
16.23 ± 1.2 |
16.35 ± 1.6 |
10.01 ± 1.7 |
14.64 ± 1.2 |
14.36 ± 1.6 |
13.21 ± 1.8 |
11.29 ± 1.3 |
11.23 ± 1.7 |
12.21 ± 1.2 |
11.06 ± 1.2 |
12.49 ± 1.4 |
11.84 ± 1.9 |
12.23 ± 1.8 |
AS17
|
Cl |
3 |
18.36 ± 1.4 |
12.59 ± 1.8 |
14.26 ± 1.1 |
14.17 ± 1.3 |
13.59 ± 1.2 |
11.22 ± 1.5 |
13.41 ± 1.4 |
13.23 ± 1.2 |
12.26 ± 1.9 |
10.26 ± 1.2 |
10.26 ± 1.2 |
12.29 ± 1.1 |
11.42 ± 1.5 |
11.54 ± 1.2 |
11.08 ± 1.2 |
12.43 ± 1.7 |
AS18
|
Br |
3 |
18.11 ± 1.8 |
11.25 ± 1.2 |
14.19 ± 1.2 |
13.54 ± 1.4 |
13.12 ± 1.3 |
10.27 ± 1.6 |
13.84 ± 1.3 |
13.54 ± 1.7 |
11.45 ± 1.4 |
10.87 ± 1.1 |
11.09 ± 1.8 |
11.84 ± 1.7 |
11.03 ± 1.7 |
11.12 ± 1.6 |
10.69 ± 1.1 |
11.32 ± 1.2 |
AS19
|
I |
3 |
16.89 ± 1.1 |
11.89 ± 1.5 |
13.54 ± 1.7 |
13.48 ± 1.2 |
13.98 ± 1.6 |
10.09 ± 1.1 |
11.23 ± 1.8 |
12.24 ± 1.9 |
10.69 ± 1.2 |
10.09 ± 1.2 |
10.24 ± 1.1 |
10.43 ± 1.9 |
10.87 ± 1.2 |
10.23 ± 1.2 |
10.11 ± 1.1 |
10.63 ± 1.5 |
AS20
|
OCH3 |
3 |
18.97 ± 1.4 |
13.25 ± 1.3 |
12.58 ± 1.5 |
15.21 ± 1.5 |
13.22 ± 1.7 |
11.23 ± 1.2 |
13.25 ± 1.7 |
14.69 ± 1.3 |
12.23 ± 1.3 |
11.27 ± 1.1 |
14.36 ± 1.6 |
14.22 ± 1.2 |
11.26 ± 1.1 |
10.89 ± 1.7 |
10.24 ± 1.2 |
11.27 ± 1.2 |
AS21
|
CH3 |
3 |
19.54 ± 1.6 |
14.59 ± 1.4 |
11.36 ± 1.2 |
15.78 ± 1.2 |
14.25 ± 1.4 |
12.39 ± 1.3 |
15.33 ± 1.2 |
15.36 ± 1.2 |
13.22 ± 1.2 |
12.23 ± 1.3 |
15.47 ± 1.2 |
13.09 ± 1.1 |
11.37 ± 1.2 |
11.59 ± 1.1 |
10.36 ± 1.3 |
12.89 ± 1.1 |
Ciprofloxacin
|
|
19.52 ± 1.6 |
20.12 ± 1.5 |
17.41 ± 1.4 |
21.27 ± 14 |
24.51 ± 1.4 |
15.95 ± 1.7 |
19.11 ± 1.35 |
14.47 ± 1.3 |
—a |
—a |
11.22 ± 1.2 |
13.27 ± 1.1 |
—a |
11.09 ± 1.3 |
22.29 ± 2.11 |
24.23 ± 2.03 |
Table 2 The antibacterial activity of thymol–isatin hybrids against Staphylococcus species showing minimum inhibitory concentrations (MICs), minimum bactericidal concentrations (MBCs) and a selectivity toward L929 cells
Compound |
Staphylococcus aureus (MTCC 740) |
Staphylococcus aureus (RBRJ010) |
Staphylococcus epidermidis (MTCC 435) |
cMRSA |
MRSA (ATCC 33591) |
L929 |
SId |
MICa |
MBCb |
MICa |
MBCb |
MICa |
MBCb |
MICa |
MBCb |
MICa |
MBCb |
IC50c |
Bacterial strains: S.a. = Staphylococcus aureus (MTCC 740), cS.a. = clinically isolated Staphylococcus aureus (RBRJ010), S.e. = Staphylococcus epidermidis (MTCC 435), cMRSA = clinically isolated methicillin resistant Staphylococcus aureus, MRSA = methicillin resistant Staphylococcus aureus (ATCC 33591). Minimum inhibitory concentration in μM. Minimum bactericidal concentration in μM. Inhibitory concentration (50%) in μM for test compounds (AS1, AS8–AS10 and AS14) and in nM for paclitaxel. Selectivity index value of >3 is considered to be highly selective. Not tested. Not calculated. |
AS1
|
62.5 |
125 |
15.6 |
31.2 |
15.6 |
31.2 |
62.5 |
125 |
—e |
—e |
250 |
4 |
AS2
|
62.5 |
125 |
—e |
—e |
—e |
—e |
—e |
—e |
—e |
—e |
—e |
—f |
AS3
|
—e |
—e |
—e |
—e |
—e |
—e |
—e |
—e |
—e |
—e |
—e |
—f |
AS4
|
—e |
—e |
—e |
—e |
—e |
—e |
—e |
—e |
—e |
—e |
—e |
—f |
AS5
|
—e |
—e |
—e |
—e |
—e |
—e |
—e |
—e |
—e |
—e |
—e |
—f |
AS6
|
—e |
—e |
62.5 |
125 |
—e |
—e |
—e |
—e |
—e |
—e |
—e |
—f |
AS7
|
—e |
—e |
31.2 |
62.5 |
—e |
—e |
—e |
—e |
—e |
—e |
—e |
—f |
AS8
|
1.9 |
3.9 |
1.9 |
3.9 |
3.9 |
7.8 |
1.9 |
3.9 |
3.9 |
7.8 |
125 |
65 |
AS9
|
7.8 |
15.6 |
7.8 |
15.6 |
—e |
—e |
15.6 |
31.2 |
62.5 |
125 |
31.2 |
2 |
AS10
|
15.6 |
31.2 |
15.6 |
31.2 |
—e |
—e |
31.2 |
62.5 |
62.5 |
125 |
125 |
4 |
AS11
|
31.2 |
62.5 |
—e |
—e |
—e |
—e |
31.2 |
62.5 |
62.5 |
125 |
—e |
—f |
AS12
|
31.2 |
125 |
—e |
—e |
—e |
—e |
62.5 |
125 |
62.5 |
125 |
—e |
—f |
AS13
|
62.5 |
125 |
15.6 |
31.2 |
—e |
—e |
3.9 |
7.8 |
7.8 |
15.6 |
—e |
—f |
AS14
|
3.9 |
7.8 |
7.8 |
15.6 |
15.6 |
31.2 |
3.9 |
7.8 |
3.9 |
7.8 |
125 |
32 |
AS15
|
15.6 |
31.2 |
—e |
—e |
—e |
—e |
—e |
—e |
—e |
—e |
—e |
—f |
AS16
|
—e |
—e |
—e |
—e |
—e |
—e |
—e |
—e |
—e |
—e |
—e |
—f |
AS17
|
—e |
—e |
—e |
—e |
—e |
—e |
—e |
—e |
—e |
—e |
—e |
—f |
AS18
|
—e |
—e |
—e |
—e |
—e |
—e |
—e |
—e |
—e |
—e |
—e |
—f |
AS19
|
—e |
—e |
—e |
—e |
—e |
—e |
—e |
—e |
—e |
—e |
—e |
—f |
AS20
|
—e |
—e |
—e |
—e |
—e |
—e |
—e |
—e |
—e |
—e |
—e |
—f |
AS21
|
—e |
—e |
—e |
—e |
—e |
—e |
—e |
—e |
—e |
—e |
—e |
—f |
Ciprofloxacin
|
—e |
—e |
—e |
—e |
—e |
—e |
3.9 |
7.8 |
1.9 |
3.9 |
—e |
—f |
Paclitaxel
|
—e |
—e |
—e |
—e |
—e |
—e |
—e |
—e |
—e |
—e |
62.5 |
—f |
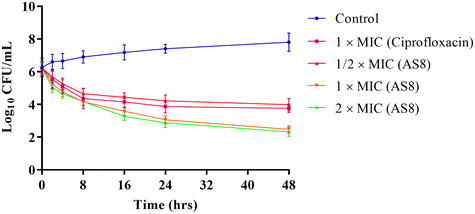 |
| Fig. 3 The time-kill assay depicting the bacteriostatic potential of AS8 at 1/2 × MIC and the bactericidal efficacy at 1 × MIC and 2 × MIC levels against MRSA. | |
2.3. Anti-biofilm activity
Static biofilm formation assay was performed to evaluate the biofilm inhibitory potential of AS8 against MRSA.33 Biofilm mass was significantly reduced on treatment with AS8 dose dependent reduction in biofilm formation was observed on treatment with 1/2 × MIC [percentage reduction in biofilm formation (PR): 78.43 ± 3.47], 1 × MIC (PR: 89.63 ± 3.75%), and 2 × MIC (PR: 96.21 ± 3.59%), respectively. In addition, AS8 showed biofilm eradication capability in a dose-dependent manner with minimum biofilm eradication concentration (MBEC) value of 7.8 μM. Similar outcomes were observed in both microscopy assays (crystal violet and Syto-9) (Table 3). In fluorescence assay (Syto-9), a decrease in the integrated density was recorded on treatment with 1/2 × MIC (1609.248), 1 × MIC (63.224), and 2 × MIC (31.28) as compared to the control (3126.145), respectively (Fig. 4E–H). A similar pattern was observed in the case of crystal violet microscopic assay (Fig. 4A–D), in which a significant decrease in biofilm, mean thickness, and substratum coverage was observed as compared to the control on treatment with AS8. The anti-biofilm efficacy of AS8 was further validated by analysing the morphology of the treated cells via scanning electron microscopy, which depicted the morphological deformities in the MRSA cells. Well organized and aggregated biofilm were observed in the control, which were heavily interrupted on the treatment of AS8. On treatment with 1 × MIC and 2 × MIC, AS8 cell leakage and irregular surface-like characters were observed. All outcomes ensured the anti-biofilm efficacy of AS8 (Fig. 4I–L).
Table 3 The anti-biofilm capability of AS8 against MRSA
Sr. no. |
Treatment |
Crystal violet assaya (96-well plates) |
Crystal violet assay (microscopy) |
Fluorescence assay (microscopy-Syto9) |
Volume/area (μm3 μm−2) |
Mean thickness (μm) |
Substratum coverage (%) |
Integrated density |
Percentage reduction of biofilm formation as compared to the control.
|
1. |
Control |
— |
18.37 ± 1.14 |
15.03 ± 1.63 |
81.43 ± 3.57 |
3126.145 ± 133.756 |
2. |
1/2 × MIC |
78.43 ± 3.47 |
3.48 ± 0.53 |
2.97 ± 0.34 |
19.12 ± 2.01 |
1609.248 ± 69.47 |
3. |
1 × MIC |
89.63 ± 3.75 |
1.57 ± 0.26 |
1.11 ± 0.19 |
5.11 ± 0.63 |
63.224 ± 4.12 |
4. |
2 × MIC |
96.21 ± 3.59 |
0.69 ± 0.15 |
0.16 ± 0.03 |
1.83 ± 0.31 |
31.28 ± 2.156 |
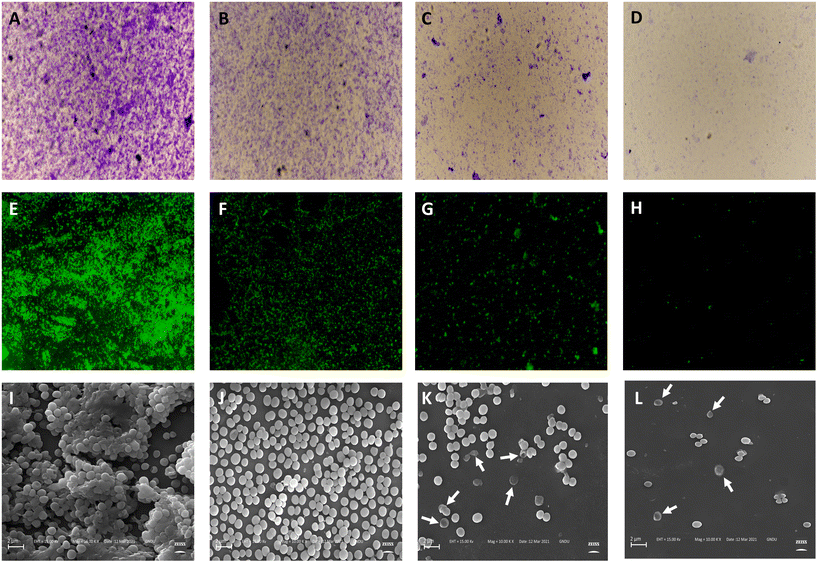 |
| Fig. 4 The microscopic assessment of the anti-biofilm capability of AS8 against MRSA at three concentration levels [1/2 × MIC (B, F and J), 1 × MIC (C, G and K) and 2 × MIC (D, H and L)] as compared to the control (A, E and I) by crystal violet (A–D), fluorescence (E–H, Styto-9) and scanning electron microscopy (I–L) assays. The scanning electron microscopy assay revealed cell leakage (K) and irregular surface (L) like characteristics on treatment with 1 × MIC and 2 × MIC levels as highlighted by white arrows. | |
2.4. Effect on staphyloxanthin and metabolic intermediates in its biosynthesis
The effect of AS8 on the production of staphyloxanthin in MRSA was qualitatively analysed, and results revealed the dose-dependent disappearance of golden-yellow pigment production (reduction in golden-yellow pigment production: 1/4 × MIC > 1/8 × MIC > 1/16 × MIC), as compared to the control (Fig. 5A–D). Staphyloxanthin and its metabolic intermediates were further quantitatively analysed. Spectroscopic quantification confirmed the significant reduction of all intermediates such as 4,4′-diapophytoene (reduced by 86 ± 2.31%), 4,4′-diaponeurosporene (reduced by 85%), and 4,4′-diaponeurosporenic acid (reduced by 86 ± 1.49%) and ultimately staphyloxanthin (reduced by 86 ± 1.94%) at the 1/4 × MIC level, as compared to the control (Fig. 5E–H). Overall analysis suggests the interruption of initial condensation of farnesyl diphosphate to form 4,4′-diapophytoene by CrtM inhibition that further hampers the synthesis of subsequent intermediates that ultimately reduce the production of staphyloxanthin pigment.35
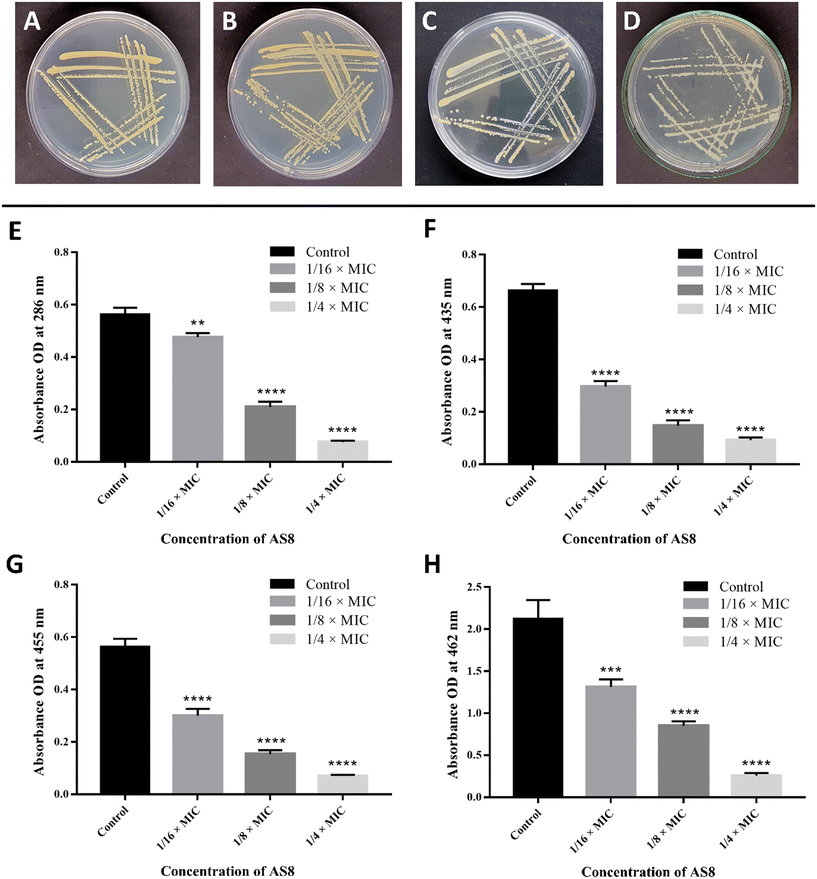 |
| Fig. 5 The qualitative assessment of the decreased production of golden-yellow pigment staphyloxanthine after the treatment of AS8 (1/16 ×, 1/8 ×, and 1/4 × MIC) showing a dose-dependent effect as compared to the control (A–D) and at the same dose levels; quantitative spectrometric analysis also showed reduced levels of staphyloxanthin (H) as well as its biosynthesis pathway intermediates, i.e., 4,4′-diapophytoene (E), 4,4′-diaponeurosporene (F) and 4,4′-diaponeurosporenic acid (G), respectively, confirming CrtM inhibition. | |
2.5. Molecular docking studies
Molecular docking studies were performed to get insight into the various molecular interactions of the most potent compound (AS8) with the dehydrosqualene synthase (CrtM) enzyme responsible for the MRSA virulence.33 For that purpose, the X-ray crystallographic structure of dehydrosqualene synthase (CrtM) complexed with BPH-830 (PDB entry: 2ZY1; resolution: 1.78 Å) was employed.36 The accuracy of the docking protocol was validated by docking co-crystallized ligand BPH-830 into its binding site. The program was capable of reproducing the best fit confirmation of BPH-830 with root mean square deviation (RMSD) value of 0.5005, indicating the reliability of the docking protocol.37 After that, AS8 was docked into the BPH-830 binding site, and the best pose with a dock score of −18.8031 and ΔG value of −25 kJ mol−1 were selected for discussion (Fig. 6).
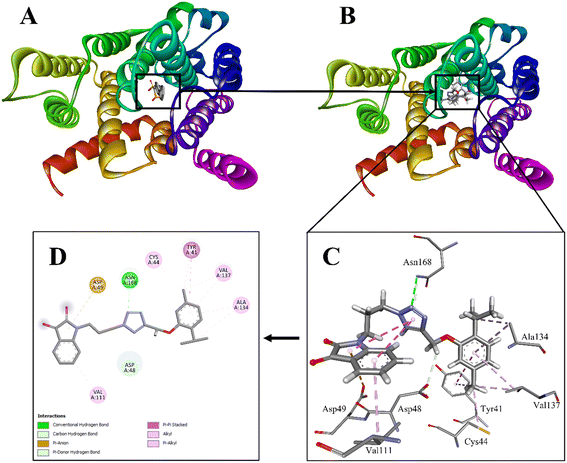 |
| Fig. 6 A. Dehydrosqualene synthase (CrtM) from Staphylococcus aureus in complex with BPH-830 (PDB entry: 2ZY1; resolution: 1.78 Å). B. Dehydrosqualene synthase (CrtM) from Staphylococcus aureus in complex with AS8. C. A 3D view of interactions of AS8 with the residues of the binding site. D. A 2D view of the interactions of A8 with the residues of the binding site. | |
Overall binding pattern of AS8 with the residues of BPH-830 binding site suggest that the hybrid is properly positioned in the cavity and well stabilized by various favourable electrostatic interactions. Major interactions with enzyme include π–cation, π–alkyl, carbon–hydrogen bond, alkyl interactions, and conventional hydrogen bond interactions. The isatin moiety is well positioned in the cavity formed by one hydrophobic (Val111) and one hydrophilic (Asp49) residue. Val111 is making π–alkyl interactions while Asp49 is forming π–anion interaction with the isatin moiety. The triazole between isatin and thymol nucleus is making π-donor hydrogen bond and conventional hydrogen bond interaction with hydrophilic residue Asn168 (H-bond acceptor; with amine group: d = 3.0132), suggesting the strong hold of the triazole ring in the cavity. Next to the triazole ring, the methyl group is making carbon–hydrogen bond interaction with the hydrophilic residue Asp48. The thymol nucleus is further well positioned in the cavity formed by two hydrophobic (Ala134 and Val137) and two hydrophilic (Cys44 and Tyr41) residues. The isopropyl group on the thymol nucleus is making alkyl interaction with Ala134. Another π–alkyl interaction is also observed with Ala134 from the central aromatic core. The methyl group opposite to the isopropyl substitution is making π–alkyl interaction with Tyr41 and alkyl interactions with Cys44 and Val137, respectively. The central core aromatic ring is also observed to form π–π stacked interaction with Tyr41 and π–alkyl interaction with Val137. Both Ala134 and Val137 are the key residues of the CrtM active site. A significant number of interactions with the active site is making thymol a key pharmacophore for binding in the active site of CrtM. Thus, overall study suggests that AS8 is well decorated with small, rigid, and planar groups, making the finest scaffold, which is able to satisfy the necessary pharmacophoric requirements for the inhibition of dehydrosqualene synthase (CrtM) of MRSA.
2.6. Molecular dynamic simulations
The molecular dynamics (MD) simulation of the complex between dehydrosqualene synthase (CrtM) from Staphylococcus aureus and hybrid AS8 was thoroughly evaluated. Fig. 7A, which displays the RMSD trajectories and confirms the conformational stability of both the protein and the ligand throughout the simulation. The RMSD of the protein's backbone was found to average at 2.34 ± 0.42 Å. In comparison, the ligand's RMSD relative to the protein was 5.97 ± 0.92 Å. The RMSF of the protein's backbone further indicates the stability, averaging at 1.10 ± 0.54 Å. Among the residues, Met1 exhibited the highest fluctuation of 4.66 Å, suggesting its involvement in dynamic interactions or conformational changes. In contrast, Tyr284 showed minimal fluctuation with an RMSF of 1.527 Å, as depicted in Fig. 7B.
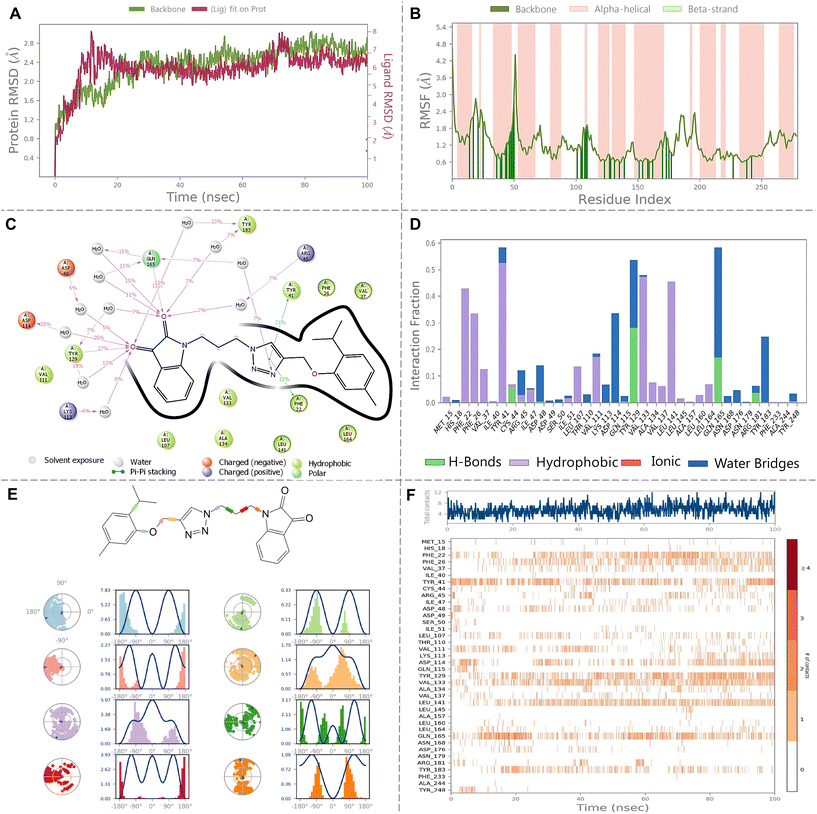 |
| Fig. 7 A. The RMSD plot of CrtM (green) and AS8 (dark red); B. the RMSF plot of the amino acid residues of CrtM. C. The 2D interactions of AS8 with amino acid residues showing major interactions during the simulation time. D. A histogram of amino acid residues versus the fraction of interactions, where 0.1 interaction fraction means interaction persisted for 1 ns of the simulation time. E. The torsion profile of AS8, where the centre of the circle is the starting point of the simulation, i.e., 0 ns. F. The interaction of amino acids with AS8 throughout the simulation time (100 ns). | |
A detailed investigation into the interactions between the ligand and the binding site amino acid residues revealed several critical interactions. Tyr129 and Gln165 formed direct hydrogen bonds with the ligand. Water bridge interactions, facilitating binding, were observed with residues such as Lys113, Asp114, Asp48, Tyr18, and Arg45. A π–π stacking interaction between Tyr41 and the ligand also emerged as significant for maintaining the ligand's position in the binding site. These interaction patterns are thoroughly visualized in Fig. 7C (percentage interaction diagram) and Fig. 7D (interaction fraction plot). Timeline interaction plot in Fig. 7F provides insight into the interactions' dynamics over the 100 ns simulation period. Moreover, Fig. 7E represents the ligand's torsional behaviour during the simulation. The 2D ligand diagram, complemented with individual dial and bar charts, tracks the ligand's conformational changes. The dial charts, starting from the trajectory's beginning, represent the current torsion configurations, while the bar charts offer an overview of torsion probabilities over time. If torsional potential data is available, it is represented to showcase the energy potential of the rotatable bonds, expressed in kcal mol−1 on the primary Y-axis. Analysing this data provides a comprehensive view of the ligand's structural adaptability within the protein complex.
2.7.
In vivo toxicity and protection from MRSA infection in Galleria mellonella larva
Galleria mellonella is a popular alternative and most suitable nonmammalian animal model for the evaluation of the in vivo toxicity and efficacy of anti-infective agents and was employed for the in vivo assessment of AS8 (Fig. 8).38AS8, when administered at a high dose level of 20 mg kg−1 to the larva hemocoel, showed no visible injury and normal metabolic activity of the larva when compared to the control larva. The survival rate of the larva was 90% upto 120 h, which indicates the non-toxicity of AS8 toward the larva (Fig. 8A). Furthermore, the survival plot suggests the protective effects of AS8 and effectively rescued the larva from MRSA infection at all tested low, medium, and higher concentration levels, i.e., 2.5, 5, and 10 mg kg−1, which was further validated through larval bacterial burden count that was evaluated 24 and 48 h post inoculation. At the dose level of 2.5 mg kg−1, 80% survival was observed, while at 5 and 10 mg kg−1, survival was 90%, respectively. This reflects the capability of AS8 to tackle MRSA infections in biological medium (Fig. 8B and C). Circulating haemocyte population in the larvae has been previously observed to be modulated by immune response toward microorganism in insects. Haemocytes in circulation lymph were also found to be elevated in the treated group when compared to the infection control group (Fig. 8D and E). This reflects the capability of AS8 not only to rescue the larvae from MRSA infection but also to modulate its immune response.35
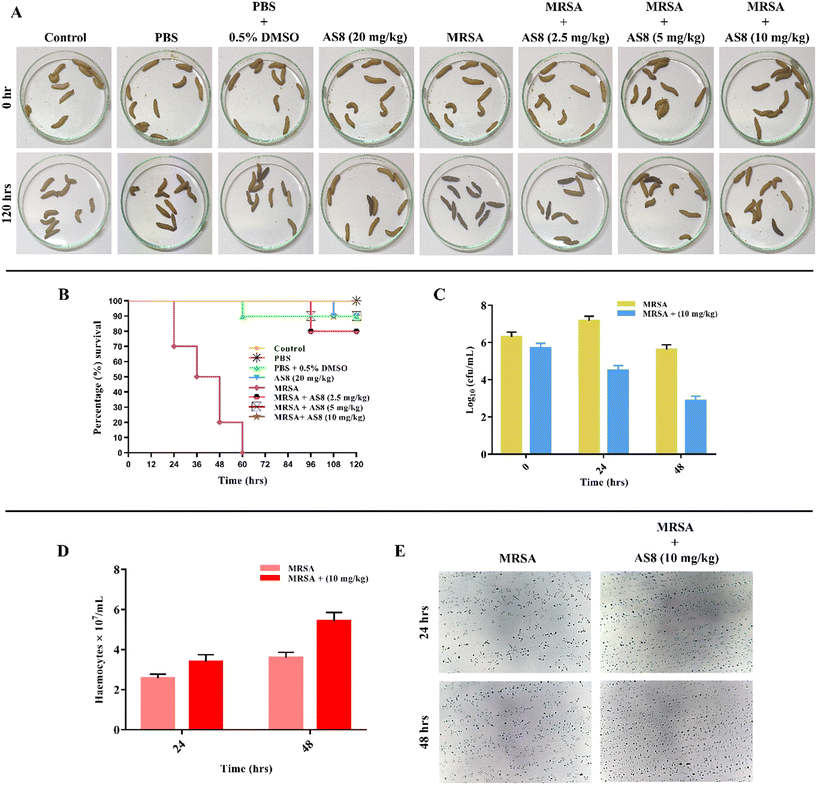 |
| Fig. 8
In vivo toxicity and anti-MRSA efficacy assessment of AS8 by Galleria mellonella-based larva model. A. Representative images displaying the survival status of Galleria mellonella at the beginning (0 h) and at end (120 h) of the survival experiment; B. Kaplan–Meier survival plot displaying the survival of Galleria mellonella challenged with different treatments; C. MRSA burden in Galleria mellonella with or without AS8 treatment, at different time intervals (0, 24, and 48 h) of experimentation; D. haemocytes density in Galleria mellonella at different time intervals (24 and 48 h) of experimentation, with or without AS8 treatment; E. representative microscopic images of haemocytes at different time intervals (24 and 48 h) of experimentation, with or without AS8 treatment. | |
3. Conclusion
Keeping in mind the emerging threat of bacterial resistance all over the globe and CrtM inhibitory potential of thymol in MRSA along with known anti-MRSA and antibiofilm capacity of isatin-based derivatives, a new series of thymol–isatin hybrids were designed, synthesized, and evaluated for their antibacterial efficacy against a panel of standard and clinically isolated multidrug-resistant bacterial strains. Most compounds were found sensitive against all tested strains while more selective toward Staphylococcus strains. Compound AS8 exhibited potent antibacterial activity against methicillin-resistant Staphylococcus aureus (MRSA: MIC = 1.9 μM and MBC = 3.9 μM) and was also capable to inhibit biofilm formation. AS8 was found to exhibit CrtM inhibitory efficacy in MRSA and decreased virulence factor staphyloxanthin production, which was further supported by positive interactions in molecular docking studies. In the larva-based (Galleria mellonella) model, AS8 was found to be non-toxic and capable to rescue larva from MRSA-based systemic infection (90% survival at 10 mg kg−1) and also modulated the immune response. Overall findings established AS8 as a promising candidate for the management and further drug development against drug-resistant infectious diseases, especially against MRSA infections.
4. Experimental
4.1. Materials and measurements
All chemicals were procured from Sigma Aldrich, CDH, and Loba, India and utilized without any further purification. The progress of the reaction was monitored using analytical thin-layer chromatography (TLC) performed on pre-coated silica gel GF254 plates. All yields refer to isolated products after purification by column chromatography performed on silica gel (60–120 mm). Characterization of the products was done by spectroscopic techniques such as 1H and 13C NMR, mass spectroscopy, and elemental analysis. NMR spectra were recorded using an ADVANCE III HD 500 MHz Bruker Biospin spectrometer. The spectra were measured in DMSO-d6 and CDCl3. In 1H NMR, chemical shifts were reported in δ values with a number of protons, multiplicities (s-singlet, d-doublet, t-triplet, m-multiplet), and coupling constants (J) in Hertz (Hz). Mass spectra were recorded on a Bruker micrOTOF QII mass spectrometer. Elemental analysis was performed on a Thermo Scientific FLASH 2000 Analyzer. Melting points were determined using a digital melting point apparatus (SMP20, STUART, Cole-Parmer Ltd. UK) and were uncorrected.
4.1.1. Synthesis of 1-(2-bromoethyl)indoline-2,3-dione.
To a solution of isatin (1 equiv.) in DMF, K2CO3 (1.5 equiv.) was added and allowed to stir for 15 min. After 15 min, dibromoethane (1 equiv.) was added and the reaction mixture was stirred at room temperature till it was completed (examined by TLC). After completion, the reaction mixture was poured in crushed ice and set aside for some time (until ice converted into the water completely), filtered, and dried. The obtained crude product was purified via column chromatography using hexane
:
ethylacetate (9.5
:
0.5) to get N-bromoethyl isatin. The same procedure mentioned above was used to synthesize remaining N-bromoalkyl isatins by taking different types of substituted isatins and dibromoalkanes.27
4.1.2. Synthesis of 1-(2-azidoethyl)indoline-2,3-dione.
To a solution of 1-(2-bromoethyl)indoline-2,3-dione (1 equiv.) in DMF, sodium azide (1 equiv.) was added, and the obtained reaction mixture was stirred at room temperature till it was completed (examined by TLC). After completion, the reaction mixture was poured in crushed ice and set aside for a while (until ice converted into water completely), filtered, and dried to get pure 1-(2-azidoethyl)indoline-2,3-dione.27
1-(2-Azidoethyl)indoline-2,3-dione (8).
Yield 94%, red powder. 1H NMR (500 MHz, DMSO-d6) δ 7.71–7.68 (m, 1H), 7.59–7.58 (d, J = 5 Hz, 1H), 7.31–7.29 (d, J = 10 Hz, 1H), 7.18–7.15 (m, 1H), 3.91–3.89 (t, J = 5 Hz, 1H), 3.65–3.63 (t, J = 5 Hz, 1H). 13C NMR (125 MHz, DMSO-d6) δ 183.52, 158.79, 150.91, 138.75, 125.02, 123.83, 117.91, 111.46, 48.56. Anal. calcd. for C10H8N4O2: C, 55.55; H, 3.73; N, 25.91; found: C, 55.54; H, 3.72; N, 25.89.
The same procedure mentioned above was used to synthesize the remaining N-azidoalkyl isatins.
4.1.3. Synthesis of 1-isopropyl-4-methyl-2-(prop-2-yn-1-yloxy)benzene.
Thymol (1 equiv.) was suspended in DMF, and K2CO3 (1.5 equiv.) was added to it and allowed to be stirred for 10 min. After 10 min, propargyl bromide (1 equiv.) was added and stirring was continued at room temperature till it was completed (monitored by analytical TLC). As the reaction was completed, the reaction mixture was poured on crushed ice and set aside for a while (until the ice was completely converted into water). The compound suspended in water was separated through fractionation using ethyl acetate. The obtained crude product was purified using column chromatography using hexane
:
ethyl acetate (9.5
:
0.5) to get 1-isopropyl-4-methyl-2-(prop-2-yn-1-yloxy)benzene.39
1-Isopropyl-4-methyl-2-(prop-2-yn-1-yloxy)benzene (10).
Yield 61%, light-brown semisolid. 1H NMR (500 MHz, CDCl3) δ 7.11–7.10 (d, J = 5 Hz, 1H), 6.80–6.78 (d, J = 10 Hz, 1H), 6.77 (s, 1H), 4.70–7.69 (m, 2H), 3.32–3.27 (m, 1H), 2.51–2.50 (m, 1H), 2.33 (s, 3H), 1.20 (s, 3H), 1.19 (s, 3H). 13C NMR (125 MHz, CDCl3) δ 154.69, 136.23, 134.66, 126.07, 122.17, 113.00, 79.09, 75.03, 56.05, 26.39, 22.83, 21.03. Anal. calcd. for C13H16O: C, 82.94; H, 8.57; found: C, 82.92; H, 8.56. HRMS (ESI) m/z for C13H16O [M]+ calcd. 188.2700, found 188.2698.
4.1.4. General procedure for the synthesis of isatin–thymol hybrids (AS1–AS21).
To a solution of 1-isopropyl-4-methyl-2-(prop-2-yn-1-yloxy)benzene (1 equiv.) in DMF, 1-(2-azidoalkyl)indoline-2,3-dione (1 equiv.) was added along with a catalytic amount of copper sulphate and sodium ascorbate. The reaction mixture so obtained was stirred at room temperature till it was completed (examined by TLC). After completion, the reaction mixture was poured into crushed ice with gentle stirring (100 rpm) to eliminate extra copper sulfate and sodium ascorbate, kept aside for a while (until ice converted into water completely), filtered, and dried to get the desired isatin–thymol hybrids.
1-(2-(4-((2-Isopropyl-5-methylphenoxy)methyl)-1H-1,2,3-triazol-1-yl)ethyl)indoline-2,3-dione (AS1).
Yield 85%, mp 105–107 °C, reddish-brown powder. 1H NMR (500 MHz, DMSO-d6) δ 8.29 (s, 1H), 7.55–7.51 (m, 2H), 7.11–7.08 (m, 1H), 7.06–7.04 (d, J = 10 Hz, 1H), 6.91–6.88 (m, 2H), 6.73–6.72 (d, J = 5 Hz, 1H), 5.04 (s, 2H), 4.71–4.68 (t, J = 5 Hz, 2H), 4.17–4.15 (t, J = 5 Hz, 2H), 3.17–3.07 (m, 1H), 2.26 (s, 3H), 1.09 (s, 3H), 1.08 (s, 3H). 13C NMR (125 MHz, DMSO-d6) δ 183.41, 158.60, 155.51, 150.71, 138.54, 136.33, 133.70, 126.04, 125.42, 124.97, 123.74, 121.81, 117.81, 113.49, 113.34, 110.67, 61.89, 47.46, 26.46, 26.39, 23.09, 21.44. Anal. calcd. for C23H24N4O3: C, 68.30; H, 5.98; N, 13.85; found: C, 68.29; H, 5.97; N, 13.83. HRMS (ESI) m/z for C23H24N4O3 [M + H]+ calcd. 405.1882, found 405.1885.
5-Fluoro-1-(2-(4-((2-isopropyl-5-methylphenoxy)methyl)-1H-1,2,3-triazol-1-yl)ethyl)indoline-2,3-dione (AS2).
Yield 91%, mp 123–125 °C, brown powder. 1H NMR (500 MHz, DMSO-d6) δ 8.30 (s, 1H), 7.47–7.45 (m, 1H), 7.39–7.35 (m, 1H), 7.05–7.04 (d, J = 5 Hz, 1H), 6.91 (s, 1H), 6.91–6.85 (m, 1H), 6.73–6.71 (d, J = 10 Hz, 1H), 5.05 (s, 2H), 4.69–4.67 (t, J = 5 Hz, 2H), 4.17–4.15 (t, J = 5 Hz, 2H), 3.20–3.06 (m, 1H), 2.26 (s, 3H), 1.08 (s, 3H), 1.07 (s, 3H). 13C NMR (125 MHz, DMSO-d6) δ 182.83, 158.65, 157.92, 155.50, 146.97, 136.32, 133.69, 126.08, 124.54, 124.35, 121.80, 120.08, 118.65, 115.97, 113.32, 111.83, 61.86, 47.48, 26.40, 23.12, 21.43, 21.14. Anal. calcd. for C23H23FN4O3: C, 65.39; H, 5.49; N, 13.26; found: C, 65.37; H, 5.51; N, 13.28. HRMS (ESI) m/z for C23H23FN4O3 [M + H]+ calcd. 423.1788, found 423.1791.
5-Chloro-1-(2-(4-((2-isopropyl-5-methylphenoxy)methyl)-1H-1,2,3-triazol-1-yl)ethyl)indoline-2,3-dione (AS3).
Yield 79%, mp 112–114 °C, dark-orange powder. 1H NMR (500 MHz, DMSO-d6) δ 8.30 (s, 1H), 7.60 (s, 1H), 7.56–7.54 (m, 1H), 7.08–7.04 (m, 2H), 6.91 (s, 1H), 6.73–6.71 (d, J = 10 Hz, 1H), 5.05 (s, 2H), 4.69–4.65 (m, 2H), 4.17–4.15 (t, J = 5 Hz, 2H), 3.20–3.07 (m, 1H), 2.26 (s, 3H), 1.09 (s, 3H), 1.08 (s, 3H). 13C NMR (125 MHz, DMSO-d6) δ 182.32, 158.39, 155.51, 149.26, 137.35, 136.32, 133.69, 131.61, 127.98, 126.08, 124.48, 121.80, 120.08, 119.17, 115.97, 113.33, 112.38, 66.29, 61.88, 47.47, 26.45, 23.17, 21.43. Anal. calcd. for C23H23ClN4O3: C, 62.94; H, 5.28; N, 12.77; found: C, 62.93; H, 5.26; N, 12.75. HRMS (ESI) m/z for C23H23ClN4O3 [M + H]+ calcd. 439.1537, found 439.1536.
5-Bromo-1-(2-(4-((2-isopropyl-5-methylphenoxy)methyl)-1H-1,2,3-triazol-1-yl)ethyl)indoline-2,3-dione (AS4).
Yield 85%, mp 116–118 °C, dark-orange powder. 1H NMR (500 MHz, DMSO-d6) δ 8.06 (s, 1H), 7.67 (s, 1H), 7.55–7.53 (d, J = 10 Hz, 1H), 7.11–7.10 (d, J = 5 Hz, 1H), 6.80–6.78 (d, J = 10 Hz, 1H), 6.76 (s, 1H), 6.51–6.49 (d, J = 10 Hz, 1H), 5.16 (s, 2H), 4.76 (s, 2H), 4.31–4.29 (t, J = 5 Hz, 2H), 3.21–3.15 (m, 1H), 2.32 (s, 3H), 1.17 (s, 3H), 1.15 (s, 3H). 13C NMR (125 MHz, DMSO-d6) δ 181.23, 162.62, 157.92, 155.06, 148.76, 140.88, 136.49, 134.09, 128.39, 126.12, 121.97, 118.53, 117.14, 112.54, 111.37, 62.00, 47.99, 40.91, 36.56, 31.49, 26.64, 22.73, 21.36. Anal. calcd. for C23H23BrN4O3: C, 57.15; H, 4.80; N, 11.59; found: C, 57.13; H, 4.79; N, 11.58. HRMS (ESI) m/z for C23H23BrN4O3 [M + H]+ calcd. 484.6438, found 484.6440.
5-Iodo-1-(2-(4-((2-isopropyl-5-methylphenoxy)methyl)-1H-1,2,3-triazol-1-yl)ethyl)indoline-2,3-dione (AS5).
Yield 86%, mp 119–121 °C, brown powder. 1H NMR (500 MHz, DMSO-d6) δ 8.29 (s, 1H), 7.80 (s, 1H), 7.06–7.04 (d, J = 10 Hz, 1H), 6.91 (s, 1H), 6.74–6.71 (m, 3H), 5.06 (s, 2H), 4.68–4.66 (t, J = 5 Hz, 2H), 4.15–4.13 (t, J = 5 Hz, 2H), 3.18–3.08 (m, 1H), 2.27 (s, 3H), 1.10 (s, 3H), 1.09 (s, 3H). 13C NMR (125 MHz, DMSO-d6) δ 182.08, 162.78, 157.95, 155.52, 150.06, 145.93, 136.32, 133.70, 132.68, 126.05, 121.87, 119.83, 115.98, 113.35, 113.12, 86.78, 61.89, 47.44, 36.26, 31.24, 26.48, 23.12, 21.45. Anal. calcd. for C23H23IN4O3: C, 52.09; H, 4.37; N, 10.56; found: C, 52.07; H, 4.35; N, 10.55. HRMS (ESI) m/z for C23H23IN4O3 [M + H]+ calcd. 531.3743, found 531.3741.
1-(2-(4-((2-Isopropyl-5-methylphenoxy)methyl)-1H-1,2,3-triazol-1-yl)ethyl)-5-methoxyindoline-2,3-dione (AS6).
Yield 81%, mp 113–115 °C, dark brown-powder. 1H NMR (500 MHz, CDCl3) δ 8.21 (s, 1H), 7.49–7.46 (m, 1H), 7.40 (s, 1H), 7.05–7.04 (m, 2H), 6.96 (s, 1H), 6.75–6.73 (d, J = 10 Hz, 1H), 5.11 (s, 2H), 4.52–4.49 (m, 2H), 3.74–3.71 (m, 2H), 3.16–3.12 (m, 1H), 2.30 (s, 3H), 2.28 (s, 3H), 1.09 (s, 3H), 1.08 (s, 3H). 13C NMR (125 MHz, CDCl3) δ 183.22, 158.78, 155.51, 148.73, 138.65, 136.36, 133.75, 132.96, 126.10, 125.11, 124.68, 121.98, 118.11, 115.78, 113.65, 110.97, 62.07, 47.54, 37.28, 28.15, 26.37, 23.15, 21.36. Anal. calcd. for C24H26N4O4: C, 66.34; H, 6.03; N, 12.89; found: C, 66.32; H, 6.01; N, 12.87. HRMS (ESI) m/z for C24H26N4O4 [M + H]+ calcd. 435.5038, found 435.5037.
1-(2-(4-((2-Isopropyl-5-methylphenoxy)methyl)-1H-1,2,3-triazol-1-yl)ethyl)-5-methylindoline-2,3-dione (AS7).
Yield 78%, mp 102–104 °C, dark brown-powder. 1H NMR (500 MHz, DMSO-d6) δ 8.28 (s, 1H), 7.37 (s, 1H), 7.35–7.33 (d, J = 10 Hz, 1H), 7.06–7.04 (d, J = 10 Hz, 1H), 6.91 (m, 1H), 6.79–6.78 (d, J = 5 Hz, 1H), 6.73–6.72 (d, J = 5 Hz, 1H), 5.05 (s, 2H), 4.69–4.67 (t, J = 5 Hz, 2H), 4.14–4.12 (t, J = 5 Hz, 2H), 3.16–3.09 (m, 1H), 2.26 (s, 3H), 2.25 (s, 3H), 1.09 (s, 3H), 1.08 (s, 3H). 13C NMR (125 MHz, DMSO-d6) δ 182.08, 162.78, 157.95, 155.52, 150.06, 145.93, 136.32, 133.70, 132.68, 126.05, 121.87, 119.83, 115.98, 113.35, 113.12, 86.78, 61.89, 47.44, 36.26, 31.24, 26.48, 23.12, 21.45. Anal. calcd. for C24H26IN4O3: C, 68.88; H, 6.26; N, 13.39; found: C, 68.87; H, 6.25; N, 13.37. HRMS (ESI) m/z for C24H26IN4O3 [M + H]+ calcd. 419.5048, found 419.5051.
1-(3-(4-((2-Isopropyl-5-methylphenoxy)methyl)-1H-1,2,3-triazol-1-yl)propyl)indoline-2,3-dione (AS8).
Yield 76%, mp 98–99 °C, reddish-brown powder. 1H NMR (500 MHz, DMSO-d6) δ 8.21 (s, 1H), 7.67–7.64 (m, 1H), 7.57–7.56 (d, J = 5 Hz, 1H), 7.18–7.12 (m, 2H), 7.06–7.05 (d, J = 5 Hz, 1H), 6.95 (s, 1H), 6.74–6.72 (d, J = 10 Hz, 1H), 5.12 (s, 2H), 4.52–4.49 (m, 2H), 3.76–3.74 (t, J = 5 Hz, 2H), 3.18–3.12 (m, 1H), 2.27 (s, 3H), 2.24–2.18 (m, 2H), 1.10 (s, 3H), 1.09 (s, 3H). 13C NMR (125 MHz, DMSO-d6) δ 183.76, 158.82, 155.54, 150.85, 138.43, 136.34, 133.81, 126.09, 124.89, 124.78, 123.60, 121.87, 120.09, 118.22, 113.54, 110.96, 62.04, 47.56, 37.36, 28.09, 26.45, 23.13, 21.45. Anal. calcd. for C24H26N4O3: C, 68.88; H, 6.26; N, 13.39; found: C, 68.85; H, 6.24; N, 13.38. HRMS (ESI) m/z for C24H26N4O3 [M + H]+ calcd. 419.2083, found 419.2085.
5-Fluoro-1-(3-(4-((2-isopropyl-5-methylphenoxy)methyl)-1H-1,2,3-triazol-1-yl)propyl)indoline-2,3-dione (AS9).
Yield 76%, mp 114–116 °C, brown powder. 1H NMR (500 MHz, CDCl3) δ 7.81 (s, 1H), 7.56 (s, 1H), 7.11–7.05 (m, 2H), 6.90–6.88 (d, J = 10 Hz, 1H), 6.71–6.69 (m, 1H), 6.58–6.57 (d, J = 5 Hz, 1H), 5.01 (s, 2H), 4.28–4.25 (m, 2H), 3.63–3.60 (m, 2H), 3.08–3.02 (m, 1H), 2.23–2.18 (m, 2H), 2.10 (s, 3H), 0.97 (s, 3H), 0.96 (s, 3H). 13C NMR (125 MHz, CDCl3) δ 182.39, 162.73, 160.46, 158.34, 155.20, 146.30, 136.53, 134.27, 126.10, 124.91, 121.97, 118.24, 112.87, 111.44, 109.81, 62.34, 47.79, 37.68, 36.55, 31.48, 27.58, 26.59, 22.82, 21.37. Anal. calcd. for C24H25FN4O3: C, 66.04; H, 5.77; N, 12.84; found: C, 66.02; H, 5.75; N, 12.83. HRMS (ESI) m/z for C24H25FN4O3 [M + H]+ calcd. 437.4952, found 437.4954.
5-Chloro-1-(3-(4-((2-isopropyl-5-methylphenoxy)methyl)-1H-1,2,3-triazol-1-yl)propyl)indoline-2,3-dione (AS10).
Yield 78%, mp 104–106 °C, dark-orange powder. 1H NMR (500 MHz, CDCl3) δ 7.76 (s, 1H), 7.61 (s, 1H), 7.57–7.55 (d, J = 10 Hz, 1H), 7.14–7.13 (d, J = 5 Hz, 1H), 6.92–6.90 (d, J = 10 Hz, 1H), 6.82–6.80 (m, 2H), 5.25 (s, 2H), 4.50–4.47 (m, 2H), 3.87–3.84 (m, 2H), 3.33–3.26 (m, 1H), 2.47–2.42 (m, 2H), 2.35 (s, 3H), 1.22 (s, 3H), 1.21 (s, 3H). 13C NMR (125 MHz, CDCl3) δ 182.66, 158.61, 155.51, 149.34, 137.26, 136.36, 133.82, 127.83, 126.10, 124.36, 121.90, 120.11, 119.61, 115.97, 113.59, 112.67, 62.01, 47.53, 37.46, 27.93, 26.44, 23.11, 21.42. Anal. calcd. for C24H25ClN4O3: C, 63.64; H, 5.56; N, 12.37; found: C, 63.62; H, 5.53; N, 12.35. HRMS (ESI) m/z for C24H25ClN4O3 [M + H]+ calcd. 453.9468, found 453.9471.
5-Bromo-1-(3-(4-((2-isopropyl-5-methylphenoxy)methyl)-1H-1,2,3-triazol-1-yl)propyl)indoline-2,3-dione (AS11).
Yield 83%, mp 110–112 °C, dark-orange powder. 1H NMR (500 MHz, CDCl3) δ 8.30 (s, 1H), 7.69–7.67 (d, J = 5 Hz, 1H), 7.06–7.04 (d, J = 10 Hz, 1H), 6.97–6.95 (d, J = 10 Hz, 1H), 6.91 (s, 1H), 6.84–6.82 (d, J = 10 Hz, 1H), 6.73–6.72 (d, J = 5 Hz, 1H), 5.05 (s, 2H), 4.69–4.67 (t, J = 5 Hz, 2H), 4.16–4.14 (t, J = 5 Hz, 2H), 3.18–3.07 (m, 1H), 2.29–2.28 (m, 2H), 2.26 (s, 3H), 1.09 (s, 3H), 1.08 (s, 3H). 13C NMR (125 MHz, CDCl3) δ 182.17, 158.23, 155.51, 154.60, 149.63, 140.16, 136.32, 133.70, 127.23, 126.05, 121.81, 120.08, 119.55, 115.97, 115.52, 113.35, 112.82, 61.89, 47.45, 31.24, 26.46, 23.13, 21.44, 21.15. Anal. calcd. for C24H25BrN4O3: C, 63.64; H, 5.56; N, 12.37; found: C, 63.62; H, 5.53; N, 12.35. HRMS (ESI) m/z for C24H25BrN4O3 [M + H]+ calcd. 498.4008, found 498.4006.
5-Iodo-1-(3-(4-((2-isopropyl-5-methylphenoxy)methyl)-1H-1,2,3-triazol-1-yl)propyl)indoline-2,3-dione (AS12).
Yield 71%, mp 113–115 °C, brown powder. 1H NMR (500 MHz, CDCl3) δ 8.19 (s, 1H), 7.71–7.69 (d, J = 10 Hz, 1H), 7.63 (s, 1H), 7.23–7.21 (d, J = 10 Hz, 1H), 7.07–7.05 (d, J = 10 Hz, 1H), 6.96 (s, 1H), 6.74–6.72 (d, J = 10 Hz, 1H), 5.12 (s, 2H), 4.51–4.48 (m, 2H), 3.77–3.74 (m, 2H), 3.15–3.14 (m, 1H), 2.28 (s, 3H), 2.22–2.19 (m, 2H), 1.10 (s, 3H), 1.09 (s, 3H). 13C NMR (125 MHz, CDCl3) δ 182.65, 155.54, 151.88, 149.38, 143.03, 137.23, 136.34, 136.32, 133.81, 130.12, 127.80, 126.09, 124.37, 121.87, 113.53, 112.68, 62.03, 49.07, 47.51, 26.45, 23.13, 21.45, 19.51. Anal. calcd. for C24H25IN4O3: C, 52.95; H, 4.63; N, 10.29; found: C, 52.93; H, 4.62; N, 10.28. HRMS (ESI) m/z for C24H25IN4O3 [M + H]+ calcd. 545.4013, found 545.4016.
1-(3-(4-((2-Isopropyl-5-methylphenoxy)methyl)-1H-1,2,3-triazol-1-yl)propyl)-5-methoxyindoline-2,3-dione (AS13).
Yield 88%, mp 103–105 °C, dark brown-powder. 1H NMR (500 MHz, CDCl3) δ 8.20 (s, 1H), 7.47–7.45 (d, J = 10 Hz, 1H), 7.39 (s, 1H), 7.06–7.05 (m, 2H), 6.95 (s, 1H), 6.74–6.72 (d, J = 10 Hz, 1H), 5.12 (s, 2H), 4.51–4.48 (m, 2H), 3.74–3.71 (m, 2H), 3.15–3.13 (m, 1H), 2.29 (s, 3H), 2.27 (s, 3H), 2.22–2.18 (m, 2H), 1.10 (s, 3H), 1.09 (s, 3H). 13C NMR (125 MHz, CDCl3) δ 182.66, 158.61, 155.51, 149.34, 137.26, 136.36, 133.82, 127.83, 126.10, 124.36, 121.90, 120.11, 119.61, 115.97, 113.59, 112.67, 62.01, 47.53, 37.46, 27.93, 26.44, 23.11, 21.42. Anal. calcd. for C25H28N4O4: C, 66.95; H, 6.29; N, 12.49; found: C, 66.93; H, 6.27; N, 12.48. HRMS (ESI) m/z for C25H28N4O4 [M + H]+ calcd. 449.5308, found 449.5311.
1-(3-(4-((2-Isopropyl-5-methylphenoxy)methyl)-1H-1,2,3-triazol-1-yl)propyl)-5-methylindoline-2,3-dione (AS14).
Yield 81%, mp 92–94 °C, dark brown-powder. 1H NMR (500 MHz, CDCl3) δ 8.27 (s, 1H), 7.14–7.12 (m, 2H), 7.05–7.03 (d, J = 10 Hz, 1H), 6.90 (s, 1H), 6.85–6.83 (d, J = 10 Hz, 1H), 6.72–6.71 (d, J = 10 Hz, 1H), 5.04 (s, 2H), 4.68–4.66 (t, J = 5 Hz, 1H), 4.13–4.11 (t, J = 5 Hz, 1H), 3.13–3.07 (m, 1H), 2.50 (s, 3H), 2.26–2.24 (m, 5H), 1.08 (s, 3H), 1.07 (s, 3H). 13C NMR (125 MHz, CDCl3) δ 183.50, 158.48, 155.22, 148.56, 148.31, 142.06, 138.96, 136.53, 134.27, 133.84, 125.97, 124.85, 121.90, 117.60, 112.79, 109.94, 50.85, 39.55, 39.17, 27.42, 26.62, 22.83, 21.35, 20.71. Anal. calcd. for C25H28N4O3: C, 69.42; H, 6.53; N, 12.95; found: C, 69.40; H, 6.52; N, 12.93. HRMS (ESI) m/z for C25H28N4O3 [M + H]+ calcd. 433.5318, found 433.5320.
1-(4-(4-((2-Isopropyl-5-methylphenoxy)methyl)-1H-1,2,3-triazol-1-yl)butyl)indoline-2,3-dione (AS15).
Yield 83%, mp 92–94 °C, reddish-brown powder. 1H NMR (500 MHz, DMSO-d6) δ 8.06 (s, 1H), 7.64–7.60 (m, 2H), 7.16–7.11 (m, 2H), 6.93–6.91 (d, J = 10 Hz, 1H), 6.80–6.79 (m, 2H), 5.24 (s, 2H), 4.52–4.49 (m, 2H), 3.80–3.77 (m, 2H), 3.31–3.26 (m, 1H), 2.23 (s, 3H), 2.08–2.03 (m, 2H), 1.81–1.75 (m, 2H), 1.21 (s, 3H), 1.19 (s, 3H). 13C NMR (125 MHz, DMSO-d6) δ 183.22, 158.41, 158.40, 155.20, 150.49, 138.61, 136.52, 134.25, 126.07, 125.66, 123.98, 121.94, 117.59, 112.82, 110.13, 49.64, 39.19, 36.54, 31.47, 27.41, 26.59, 24.15, 22.81, 21.36. Anal. calcd. for C25H28N4O3: C, 69.42; H, 6.53; N, 12.95; found: C, 69.40; H, 6.51; N, 12.94. HRMS (ESI) m/z for C25H28N4O3 [M + H]+ calcd. 433.5318, found 433.5321.
5-Fluoro-1-(4-(4-((2-isopropyl-5-methylphenoxy)methyl)-1H-1,2,3-triazol-1-yl)butyl)indoline-2,3-dione (AS16).
Yield 76%, mp 103–105 °C, brown powder. 1H NMR (500 MHz, DMSO-d6) δ 8.06 (s, 1H), 7.60–7.57 (m, 2H), 7.13–7.11 (d, J = 10 Hz, 1H), 6.92–6.89 (m, 1H), 6.81–6.78 (m, 2H), 5.23 (s, 2H), 4.52–4.50 (m, 2H), 3.80–3.73 (m, 2H), 3.31–3.25 (m, 1H), 2.33 (s, 3H), 2.08–2.02 (m, 2H), 1.79–1.72 (m, 2H), 1.21 (s, 3H), 1.19 (s, 3H). 13C NMR (125 MHz, CDCl3) δ 182.26, 157.84, 155.22, 148.68, 137.94, 136.48, 134.17, 129.75, 128.69, 126.04, 125.47, 121.92, 118.35, 112.74, 111.45, 111.31, 39.74, 39.35, 27.34, 26.58, 24.45, 24.01, 22.77, 21.32. Anal. calcd. for C25H27FN4O3: C, 66.65; H, 6.04; N, 12.44; found: C, 66.63; H, 6.02; N, 12.42. HRMS (ESI) m/z for C25H27FN4O3 [M + H]+ calcd. 451.5222, found 451.5219.
5-Chloro-1-(4-(4-((2-isopropyl-5-methylphenoxy)methyl)-1H-1,2,3-triazol-1-yl)butyl)indoline-2,3-dione (AS17).
Yield 75%, mp 96–98 °C, dark-orange powder. 1H NMR (500 MHz, DMSO-d6) δ 8.06 (s, 1H), 7.60–7.55 (m, 2H), 7.13–7.11 (d, J = 10 Hz, 1H), 6.92–6.89 (m, 1H), 6.81–6.79 (m, 2H), 5.24 (s, 2H), 4.52–4.50 (m, 2H), 3.79–3.76 (m, 2H), 3.31–3.25 (m, 1H), 2.33 (s, 3H), 2.08–2.02 (m, 2H), 1.79–1.70 (m, 2H), 1.21 (s, 3H), 1.19 (s, 3H). 13C NMR (125 MHz, CDCl3) δ 182.23, 157.83, 155.18, 148.73, 137.96, 136.53, 134.22, 129.82, 128.65, 126.08, 125.54, 121.95, 118.40, 112.81, 111.49, 111.34, 39.78, 39.37, 27.37, 26.60, 24.48, 24.03, 22.81, 21.36. Anal. calcd. for C25H27ClN4O3: C, 64.30; H, 5.83; N, 12.00; found: C, 64.28; H, 5.81; N, 12.01. HRMS (ESI) m/z for C25H27ClN4O3 [M + H]+ calcd. 467.9738, found 467.9741.
5-Bromo-1-(4-(4-((2-isopropyl-5-methylphenoxy)methyl)-1H-1,2,3-triazol-1-yl)butyl)indoline-2,3-dione (AS18).
Yield 77%, mp 102–104 °C, dark-orange powder. 1H NMR (500 MHz, DMSO-d6) δ 8.07 (s, 1H), 7.74–7.72 (m, 2H), 7.12–7.11 (d, J = 5 Hz, 1H), 6.86–6.84 (m, 1H), 6.81–6.79 (m, 2H), 5.24 (s, 2H), 4.52–4.50 (t, J = 5 Hz, 2H), 3.77–3.75 (t, J = 5 Hz, 2H), 3.28–3.25 (m, 1H), 2.33 (s, 3H), 2.06–2.03 (m, 2H), 1.78–1.73 (m, 2H), 1.20 (s, 3H), 1.19 (s, 3H). 13C NMR (125 MHz, CDCl3) δ 182.08, 162.64, 157.65, 155.18, 150.16, 149.18, 140.82, 136.53, 134.20, 128.37, 126.07, 121.95, 118.74, 116.80, 112.82, 111.92, 39.36, 36.56, 31.48, 27.37, 26.60, 24.01, 22.81, 21.37. Anal. calcd. for C25H27BrN4O3: C, 58.71; H, 5.32; N, 10.96; found: C, 58.69; H, 5.30; N, 10.95. HRMS (ESI) m/z for C25H27BrN4O3 [M]+ calcd. 512.4278, found 512.4281.
5-Iodo-1-(4-(4-((2-isopropyl-5-methylphenoxy)methyl)-1H-1,2,3-triazol-1-yl)butyl)indoline-2,3-dione (AS19).
Yield 73%, mp 108–110 °C, brown powder. 1H NMR (500 MHz, DMSO-d6) δ 8.07 (s, 1H), 7.74–7.72 (m, 2H), 7.12–7.11 (d, J = 5 Hz, 1H), 6.86–6.84 (m, 1H), 6.81–6.79 (m, 2H), 5.24 (s, 2H), 4.52–4.50 (t, J = 5 Hz, 2H), 3.77–3.75 (t, J = 5 Hz, 2H), 3.28–3.25 (m, 1H), 2.33 (s, 3H), 2.06–2.03 (m, 2H), 1.78–1.73 (m, 2H), 1.20 (s, 3H), 1.19 (s, 3H). 13C NMR (125 MHz, CDCl3) δ 182.07, 162.66, 157.63, 155.15, 150.18, 149.16, 140.80, 135.24, 134.59, 127.89, 126.45, 121.91, 118.05, 116.24, 112.86, 111.97, 39.35, 35.97, 31.56, 27.59, 26.04, 24.27, 22.69, 21.48. Anal. calcd. for C25H27N4O3: C, 53.77; H, 4.87; N, 10.03; found: C, 53.75; H, 4.85; N, 10.02. HRMS (ESI) m/z for C25H27IN4O3 [M]+ calcd. 559.4283, found 559.4286.
1-(4-(4-((2-Isopropyl-5-methylphenoxy)methyl)-1H-1,2,3-triazol-1-yl)butyl)-5-methoxyindoline-2,3-dione (AS20).
Yield 83%, mp 97–99 °C, dark brown-powder. 1H NMR (500 MHz, CDCl3) δ 8.20 (s, 1H), 7.47–7.45 (d, J = 10 Hz, 1H), 7.39 (s, 1H), 7.06–7.05 (d, J = 5 Hz, 1H), 6.95 (s, 1H), 6.74–6.72 (d, J = 10 Hz, 1H), 5.12 (s, 2H), 4.51–4.48 (m, 2H), 3.74–3.71 (m, 2H), 3.17–3.13 (m, 1H), 2.29 (s, 3H), 2.27 (s, 3H), 2.22–2.15 (m, 4H), 1.10 (s, 3H), 1.09 (s, 3H). 13C NMR (125 MHz, CDCl3) δ 13C NMR (126 MHz, DMSO) δ 183.98, 158.84, 155.54, 148.70, 138.68, 136.34, 133.81, 132.97, 126.09, 125.14, 121.87, 118.17, 113.53, 110.82, 62.04, 49.07, 47.56, 37.35, 28.10, 26.45, 23.13, 21.45, 20.51. Anal. calcd. for C26H30N4O4: C, 67.51; H, 6.54; N, 12.11; found: C, 67.50; H, 6.52; N, 12.10. HRMS (ESI) m/z for C26H30N4O4 [M]+ calcd. 463.5578, found 463.5577.
1-(4-(4-((2-Isopropyl-5-methylphenoxy)methyl)-1H-1,2,3-triazol-1-yl)butyl)-5-methylindoline-2,3-dione (AS21).
Yield 76%, mp 88–90 °C, dark brown-powder. 1H NMR (500 MHz, CDCl3) δ 8.05 (s, 1H), 7.65 (s, 1H), 7.45–7.41 (m, 2H), 7.13–7.12 (d, J = 5 Hz, 1H), 6.81–6.79 (m, 2H), 5.23 (s, 2H), 4.51–4.48 (m, 2H), 3.78–3.75 (m, 2H), 3.33–3.25 (m, 1H), 2.35 (s, 3H), 2.34 (s, 3H), 2.07–2.01 (m, 2H), 1.79–1.74 (s, 2H), 1.21 (s, 3H), 1.19 (s, 3H). 13C NMR (125 MHz, CDCl3) δ 183.49, 158.51, 155.20, 148.51, 148.29, 142.03, 138.94, 136.52, 134.26, 133.85, 125.99, 124.87, 121.93, 117.62, 112.81, 109.92, 50.83, 39.57, 39.16, 27.41, 26.59, 24.15, 22.81, 21.36, 20.68. Anal. calcd. for C26H30N4O3: C, 69.93; H, 6.77; N, 12.55; found: C, 69.92; H, 6.76; N, 12.53. HRMS (ESI) m/z for C26H30N4O3 [M]+ calcd. 447.5588, found 447.5591.
4.2. Antibacterial activity
4.2.1. Bacterial strains and culture media.
Pathogenic Gram-positive [Staphylococcus aureus (MTCC 740), Staphylococcus epidermidis (MTCC 435), and Enterococcus faecalis (MTCC 439)] and Gram-negative [Klebsiella pneumoniae (MTCC 109), Escherichia coli (MTCC 119) and Pseudomonas aeruginosa (MTCC 424)] bacterial strains were procured from Microbial Type Culture Collection (MTCC), Institute of Microbial Technology (IMTECH), Chandigarh, India. Methicillin resistant Staphylococcus aureus NCIM Accession No. 5718 equivalent to ATCC 33591 was procured from National Chemical Laboratory, Pune, India. Multidrug resistant bacterial clinical isolates [Escherichia coli (RBRJ005), Escherichia coli (RBRJ013), Klebsiella pneumoniae (RBRJ019), Klebsiella pneumoniae (RBRJ024), Enterobacter Cloacae (RBRJ017), Acinetobacter baumannii (RBRJ027), Enterococcus faecalis (RBRJ015), Staphylococcus aureus (RBRJ010) and methicillin resistant Staphylococcus aureus] were acquired from Guru Nanak Dev Hospital, Amritsar, Punjab, India and Post Graduate Institute of Medical Education and Research (PGIMER), Chandigarh, India.
4.2.2. Agar well diffusion method.
Mueller Hinton agar plates were employed for the initial screening of synthesized hybrids. 50 μL of each hybrid (50 μM), standard (ciprofloxacin), and negative control (DMSO) were introduced into the wells of agar plates, which were seeded with 100 μL of activated bacterial cultures (0.5 McFarland standard). Plates were incubated for 48 h at 37 °C under aerobic conditions. Clear zones of inhibition around the wells were measured and compared with the standard and the negative control. All experiments were performed in triplicate.27,35
4.2.3. Minimum inhibitory concentration (MIC).
Minimum inhibitory concentrations (MICs) of sensitive hybrid molecules were determined using the broth microdilution method, performed as per CLSI reference methods for bacteria (CLSI, 2012) with some modifications.23,35 Two-fold dilutions of hybrid molecules and ciprofloxacin (250 to 0.48 μM) were prepared in Mueller Hinton broth. 100 μL bacterial cell suspensions (1 × 105 CFU mL−1) and 100 μL prepared dilutions of hybrid molecules were added to wells that makes the final volume of 200 μL and get the desired final concentrations of hybrid molecules in 96-well plates. Control wells consisted of Mueller Hinton broth alone, sterile water alone, and bacterial cell suspensions alone while standard well contains bacterial cell suspensions + ciprofloxacin. Plates were incubated for 48 h at 37 °C. After incubation period was over, 40 μL (0.2 mg mL−1) p-iodonitrotetrazolium chloride (INT) was added to each well and incubated at 37 °C for another 30 min. All experiments were performed in triplicate. MIC was considered as the lowest concentration of the hybrid molecule with no colour change (yellow to pink), which is considered as the complete inhibition of bacterial growth.40,41
4.2.4. Minimum bactericidal concentration (MBC).
10 μL aliquots from each negative well (wells showed no colour change) and positive wells (wells with bacterial cell suspensions alone) were sub-cultured on drug-free Mueller Hinton agar plates and incubated for 48 h at 37 °C under aerobic conditions. All experiments were performed in triplicate. Concentrations with no visible growth were considered as MBC values of hybrid molecules.40,42
4.2.5. Time-kill assay.
Cell suspension of MRSA (1 × 105 CFU mL−1) was incubated with various concentration of AS8 (1/2 ×, 1 ×, and 2 × MIC) at 37 °C. Aliquots were obtained at different time intervals (1, 2, 4, 8, 12, 24, and 48 h), diluted with 0.9% saline, inoculated on Mueller Hinton agar plates, and incubated at 37 °C for 48 h; then, the colony forming units were counted. The experiment was performed in triplicate. Graph was plotted between log10 CFU mL−1 and time function.42,43
4.3. Cytotoxicity screening
Cell culturing.
Dulbecco's modified Eagle's medium (DMEM) supplemented with 10% fetal bovine serum (FBS), 100 units per mL penicillin, and 100 μg mL−1 streptomycin mixture were used to maintain the cell lines (L929: acquired from American Type Culture Collection, ATCC, USA) at 37 °C in a humidified (containing 5% CO2) atmosphere.34
MTT assay.
96-Well plates seeded with cells (5 × 103 cells per well) were treated with the serial dilutions of test compounds and the positive control paclitaxel (100 μL) for 72 h under same conditions used for maintaining cells. After 24 h of incubation, MTT reagent (5 mg mL−1) was added to each well of the plate. DMSO (100 μL) was added to solubilize formazan crystals. Absorption changes were noted down using a microplate reader at 490 nm.34 The cell viability was calculated using the following equation.
Cell viability = 100 × [Optical density of treated wells − Optical density of blank wells/Optical density of control wells − Optical density of blank wells] |
4.4. Biofilm formation/disruption assays
4.4.1. Static biofilm formation assay.
Static biofilm formation assay was performed according to the previously described crystal violet staining method in 96-well polystyrene plate with some modifications. Cell suspension of MRSA (1 × 105 CFU mL−1) from stationary phase was added to each well. The control well contained cell suspension + Mueller Hinton broth alone, sterile water alone, and MRSA cell suspensions alone while the test wells contained cell suspensions + concentrations of AS8 (1/2 ×, 1 ×, 2 × MIC). The plate was incubated for 48 h at 37 °C. After incubation, the cells were washed with distilled water thrice to remove non-adherent cells. Biofilms formed were stained with aqueous crystal violet (0.5%) and incubated for 30 min at 25 °C. Stained biofilms were washed with distilled water thrice. The bound dye was extracted using ethanol (95%), and the optical density was recorded at 545 nm using a microplate reader. Experiments were performed in triplicate.33
4.4.2. Biofilm disruption assay.
Biofilm disruption assay was performed according to the previously described 96-well polystyrene plate-based crystal violet staining method with some modifications. The cell suspension (100 μL) of MRSA (1 × 105 CFU mL−1), diluted (1
:
100) in tryptone soy broth containing glucose (3%), was added to each well and incubated for 48 h at 37 °C. After that, 100 μL prepared dilutions of AS8 were added to wells that made the final volume of 200 μL to get the desired final concentrations of AS8 and standard drug in 96-well plates and incubated for another 24 h. The control wells consisted of tryptone soy broth containing glucose (3%) alone, sterile water alone, and bacterial cell suspensions alone while the standard well contains bacterial cell suspensions + standard ciprofloxacin. After incubation, the plates were washed thrice with saline solution and fixed with ethanol (95%) for 30 min at 25 °C. Biofilms formed were stained with aqueous crystal violet (0.1%) and incubated for 30 min at 25 °C. Stained biofilms were washed with distilled water thrice. The bound dye was extracted using ethanol (95%), and the optical density was recorded at 545 nm using a microplate reader. Experiments were performed in triplicate.33,44,45
4.4.3. Antibiofilm assessment by microscopic assays.
Anti-biofilm activity assessment via microscopic assays was done according to previously described procedures with some modification. Cell suspension of MRSA (1 × 105 CFU mL−1) was added to a 6 well-plate having sterile coverslip in it. AS8 (1/2 ×, 1 ×, 2 × MIC) was added and incubated for another 48 h at 37 °C. Wells treated with ciprofloxacin were taken as the standard while untreated wells were considered as the control. After the incubation period, the coverslip was cleaned with PBS three times. The slides were stained with aqueous crystal violet (0.5%) and SYTO-9 (20 mM) and incubated for 15 minutes at 25 °C. Cells stained with crystal violet were analysed using phase contrast microscope while SYTO-9-stained cells were analysed with a fluorescence microscope at 480 nm (excitation). Biofilm quantification was done using the ImageJ software. For scanning electron microscopy (SEM) assay, the prepared biofilms with and without treatment were fixed with glutaraldehyde solution (2.0% with pH 7.0 prepared in 0.1 M phosphate buffer). Biofilms were examined by SEM (Carl Zeiss, EVO LS10, Jena, Germany) with an accelerating voltage of 14 kV.33
4.5. Qualitative analysis of staphyloxanthin production
The effect of AS8 on the production of staphyloxanthin in MRSA was qualitatively assessed by the previously described method with some modifications.23 Boiling tubes containing Mueller Hinton agar media were supplemented with different concentrations of AS8 (1/4 ×, 1/8 ×, 1/16 × MIC), which were poured on Petri dishes for solidification. MRSA was streaked on the prepared plates with or without AS8 and incubated at 37 °C for 48 h. After incubation, the plates were visualized for the production of pigmentation.
4.6. Quantitative analysis of staphyloxanthin and intermediates in staphyloxanthin biosynthesis
The estimation of staphyloxanthin content was done using previously described procedure with some modifications.23 Cell suspension of MRSA (1 × 105 CFU mL−1) was treated with AS8 (1/4 ×, 1/8 ×, 1/16 × MIC) and incubated for 24 h at 37 °C with continuous shaking at 100 rpm. Cells treated with ciprofloxacin were taken as the standard while untreated cells were considered as the control. After incubation, cells were harvested using 8000 rpm centrifugation at 4 °C for 10 min, washed with phosphate buffer saline (PBS) thrice, and suspended in 1 mL methanol. Cell suspension in methanol was shaken overnight at 37 °C. After that, the suspension was centrifuged, and the supernatant was collected, which contains pigments and was measured at 462 nm. Percentage staphyloxanthine inhibition was calculated using the following formula.
Percentage staphyloxanthine inhibition (%): [(Control OD462 nm − Treated OD462 nm)/Control OD462 nm] × 100 |
The same method was employed for the quantification of intermediates in staphyloxanthin biosynthesis at different wavelengths, i.e., 4,4′-diapophytoene, was quantified at 286 nm, 4,4′-diaponeurosporene was quantified at 435 nm, and 4,4′-diaponeurosporenic acid was quantified at 455 nm.
4.7. Molecular docking studies
The crystal structure of dehydrosqualene synthase (CrtM) from Staphylococcus aureus in the complex with BPH-830 (PDB entry: 2ZY1; resolution: 1.78 Å) was retrieved from Protein Data Bank (https://www.rcsb.org/structure/2zy1).46 The preparation of structures was performed using the drug design platform LeadIt. Co-crystalized ligand BPH-830 was used to define the binding site in CrtM with a radius of 6.50 Å.33 The structure of AS8 was drawn on ChemDraw Ultra (2013), and its energy was minimized using MM2 force field in Chem3D Ultra software. The prepared structure of AS8 was protonated in aqueous solution using ligand preparation module in LeadIt and docked into the defined binding site of sterol 14-alpha demethylase using the FlexX docking module in LeadIt. All FlexX solution yielded were scored using consensus scoring function (CScore) and ranked accordingly. The top best pose with the highest score was selected for the investigation of interactions, HYDE assessment, and calculation of free energy of binding (ΔG). The top best pose with the highest score was selected for further analysis of the interactions.27,33 2D and 3D enzyme–hybrid interactions were visualized using Discovery Studio Visualizer.29,33
4.8. Molecular dynamic simulations
Molecular dynamic simulations were performed using Desmond package in the Schrodinger suite.47 The ‘system builder’ module was employed to setup the simulation system. The protein–ligand complex was solvated in an orthorhombic box with TIP3P water molecules. The dimensions of the box were determined using a buffer distance of 10 Å from any atom of the protein–ligand complex to the box edge to ensure adequate space for system dynamics without interacting with its periodic images. Counter ions were added to the system to neutralize the overall charge and maintain physiological ion concentration. The simulation parameters were set to default, and the simulation was performed at a constant temperature and pressure (NPT ensemble). The system was initially minimized and then gradually heated to 300 K. The simulation was then equilibrated before the production run to allow the system to reach a stable state. The production run was conducted for a total of 100 ns to examine the dynamic behaviour of the protein–ligand complexes. The simulation trajectories were analysed using the ‘simulation interaction diagram’ module in Maestro. The root-mean-square deviation (RMSD) of the protein and ligand were tracked over the course of the simulation to evaluate the stability of the system. In addition, protein–ligand contacts were monitored throughout the simulation to provide insights into the dynamic interactions between the ligands and the binding site residues. Furthermore, a manual inspection of the MD trajectory was conducted by visualizing the simulation movie to gain more detailed insights into the temporal behaviour of the system.48,49
4.9.
In vivo assessment using Galleria mellonella-based model
4.9.1. Larva culture and inoculation.
Assays on greater wax moth were performed according to the previously described procedures with some modifications.35,38 Ten healthy larvae (0.2 to 0.4 g) were randomly chosen for each group. Each group was segregated and held in Petri plates with a layer of Whatman filter paper. MRSA culture (5 × 108 ± 0.2 cells per mL) was injected in the last proleg of the test larvae using insulin syringe, such that the final concentration in each group was 5 × 108 ± 0.2 cells per larva. The larvae were maintained at 37 °C and monitored every 12 h. Larvae that turned black and did not respond any physical stimulus were considered dead. The groups were allocated as follows.
Group I: Control, did not received any treatment.
Group II: Control PBS.
Group III: Vehicle control, received PBS + 2% dimethyl sulfoxide (DMSO).
Group IV: AS8 (20 mg kg−1 of AS8), to access survival rate and toxic effect, if any.
Group V: Infection group, inoculated with MRSA (5 × 108 ± 0.2 cells per larva).
Group VI: Treatment group I, inoculated with MRSA (5 × 108 ± 0.2 cells per larva) + 2.5 mg kg−1 of AS8.
Group VII: Treatment group II, inoculated with MRSA (5 × 108 ± 0.2 cells per larva) + 5 mg kg−1 of AS8.
Group VIII: Treatment group III, inoculated with MRSA (5 × 108 ± 0.2 cells per larva) + 10 mg kg−1 of AS8.
4.9.2. Acute toxicity study.
Each group after interventions was maintained at 37 °C and monitored every 12 h for 120 h. Dead larva, if any, were removed at each time point of survival, percentage survival was calculated, and the graph was plotted.35
4.9.3.
In vivo antibacterial efficacy.
Separate groups were made for in vivo assay and the given above-described interventions and were maintained at 37 °C. The in vivo survival of MRSA in larva was accessed at 0, 24, and 48 h post inoculation by the CFU assay. Three larva were randomly chosen, surface-sterilized using ethanol, and cut open with a scalpel. The material obtained was suspended in sterile PBS, serially diluted, and plated on Mueller Hinton agar plates.35
4.9.4. Estimation of hemocyte density in hemolymph.
Hemolymph collected (in microcentrifuge tube placed in ice to prevent melanisation) at 24 and 48 h post inoculation was diluted with PBS, and the density was determined using an automated cell counter. Hemolymph and trypan blue (0.1%) were mixed together and observed under a fluorescent microscope.35
4.10. Statistical analysis
All experiments were performed in triplicate, and the data were represented as mean ± standard deviation (SD). Statistical analysis was carried out through the one-way analysis of variance (ANOVA), followed by the Tukey's test. The p-value of < 0.05 was regarded as significant, and asterisks are used to indicate significance.
Author contributions
Atamjit Singh: conceptualization, methodology, software, investigation, data curation, formal analysis, writing – original draft; Kirandeep Kaur: data curation, formal analysis; Pallvi Mohana: data curation, formal analysis; Karanvir Singh: data curation, formal analysis; Aman Sharma: data curation, formal analysis; Neha Khosla: data curation, formal analysis; Uttam Kaur: statistical analysis; Rajanbir Kaur: procurement and maintenance of clinically isolated bacterial strains; Rajinder Kaur: bacterial culture, writing – review & editing; Sandeep Kour: larva culture and maintenance; Puja Ohri: larva culture, writing – review & editing; Saroj Arora: cell culture, writing – review & editing; Renu Chadha: visualization, writing – review & editing; Preet Mohinder Singh Bedi: supervision, writing – review & editing.
Conflicts of interest
The authors confirm that this article content has no conflicts of interest.
Acknowledgements
Authors are grateful to the University Grants Commission for providing funds under National Fellowship for Other Backward Classes (NFOBC: Candidate Id: NFO-2018-19-OBCPUN-74306) to Atamjit Singh. Authors are thankful to Prof. Daljit Singh Arora and Dr. Sukhraj Kaur from Microbial Technology Laboratory, Department of Microbiology, Guru Nanak Dev University, Amritsar, India for providing bacterial strains used in this project. The authors are also thankful to Guru Nanak Dev University, Amritsar for providing various facilities to carry out the research work.
References
- E. D. Brown and G. D. Wright, Nature, 2016, 529, 336–343 CrossRef CAS PubMed.
- U. Hofer, Nat. Rev. Microbiol., 2019, 17, 3–3 CrossRef CAS PubMed.
-
A. Singh, K. Kaur, P. Mohana, A. Kaur, K. Kaur, S. Heer, S. Arora, N. Bedi and P. M. S. Bedi, Insights Into Drug Resistance in Staphylococcus aureus, 2021, p. 61 Search PubMed.
- Y. Li, Y. Dong, J. Lu, J. Zhang, M. Feng and J. Feng, J. Antibiot., 2023, 1–10 Search PubMed.
-
WHO, 2017.
- S. S. Kadri, Crit. Care Med., 2020, 48(7), 939–945 CrossRef PubMed.
- K. Hiramatsu, Am. J. Med., 1998, 104, 7S–10S CrossRef CAS PubMed.
- N. A. Turner, B. K. Sharma-Kuinkel, S. A. Maskarinec, E. M. Eichenberger, P. P. Shah, M. Carugati, T. L. Holland and V. G. Fowler Jr, Nat. Rev. Microbiol., 2019, 17, 203–218 CrossRef CAS PubMed.
- C.-M. Wang, C.-H. Chuang and C.-H. Chiu, Ann. Trop. Paediatr., 2005, 25, 53–57 CrossRef PubMed.
- M. K. Luther, D. M. Parente, A. R. Caffrey, K. E. Daffinee, V. V. Lopes, E. T. Martin and K. L. LaPlante, Antimicrob. Agents Chemother., 2018, 62, e02252-02317 CrossRef PubMed.
- W. Liu, P. Boudry, C. Bohn and P. Bouloc, BMC Res. Notes, 2020, 13, 1–6 CrossRef PubMed.
- A. Pelz, K.-P. Wieland, K. Putzbach, P. Hentschel, K. Albert and F. Gotz, J. Biol. Chem., 2005, 280, 32493–32498 CrossRef CAS PubMed.
- L. Xue, Y. Y. Chen, Z. Yan, W. Lu, D. Wan and H. Zhu, Infect. Drug Resist., 2019, 2151–2160 CrossRef CAS PubMed.
- A. Clauditz, A. Resch, K.-P. Wieland, A. Peschel and F. Götz, Infect. Immun., 2006, 74, 4950–4953 CrossRef CAS PubMed.
- G. Y. Liu, A. Essex, J. T. Buchanan, V. Datta, H. M. Hoffman, J. F. Bastian, J. Fierer and V. Nizet, J. Exp. Med., 2005, 202, 209–215 CrossRef CAS PubMed.
- F.-Y. Lin, C.-I. Liu, Y.-L. Liu, Y. Zhang, K. Wang, W.-Y. Jeng, T.-P. Ko, R. Cao, A. H.-J. Wang and E. Oldfield, Proc. Natl. Acad. Sci. U. S. A., 2010, 107, 21337–21342 CrossRef CAS PubMed.
- P. Gao, J. Davies and R. Y. T. Kao, mBio, 2017, 8, e01224-01317 Search PubMed.
- P. T. Dong, H. Mohammad, J. Hui, L. G. Leanse, J. Li, L. Liang, T. Dai, M. N. Seleem and J. X. Cheng, Adv. Sci., 2019, 6, 1900030 CrossRef PubMed.
- S. Ni, B. Li, Y. Xu, F. Mao, X. Li, L. Lan, J. Zhu and J. Li, Med. Res. Rev., 2020, 40, 293–338 CrossRef PubMed.
- M. A. Shareef, K. Sirisha, I. B. Sayeed, I. Khan, T. Ganapathi, S. Akbar, C. G. Kumar, A. Kamal and B. N. Babu, Bioorg. Med. Chem. Lett., 2019, 29, 126621 CrossRef CAS PubMed.
- S. Banu, R. Bollu, M. Naseema, P. M. Gomedhika, L. Nagarapu, K. Sirisha, C. G. Kumar and S. K. Gundasw, Bioorg. Med. Chem. Lett., 2018, 28, 1166–1170 CrossRef CAS PubMed.
- J. Dev, Y. Poornachandra, K. R. Reddy, R. N. Kumar, N. Ravikumar, D. K. Swaroop, P. Ranjithreddy, G. S. Kumar, J. B. Nanubolu and C. G. Kumar, Eur. J. Med. Chem., 2017, 130, 223–239 CrossRef PubMed.
- A. Valliammai, A. Selvaraj, P. Muthuramalingam, A. Priya, M. Ramesh and S. K. Pandian, Biomed. Pharmacother., 2021, 141, 111933 CrossRef CAS PubMed.
- Y. Abe, T. Harukawa, H. Ishikawa, T. Miki, M. Sumi and T. Toga, J. Am. Chem. Soc., 1956, 78, 1422–1426 CrossRef CAS.
- A. Escobar, M. Perez, G. Romanelli and G. Blustein, Arabian J. Chem., 2020, 13, 9243–9269 CrossRef CAS.
- Z. Yuan, Y. Dai, P. Ouyang, T. Rehman, S. Hussain, T. Zhang, Z. Yin, H. Fu, J. Lin and C. He, Microorganisms, 2020, 8, 99 CrossRef CAS PubMed.
- A. Singh, J. V. Singh, A. Rana, K. Bhagat, H. K. Gulati, R. Kumar, R. Salwan, K. Bhagat, G. Kaur and N. Singh, ACS Omega, 2019, 4, 11673–11684 CrossRef CAS PubMed.
- K. Bhagat, J. Bhagat, M. K. Gupta, J. V. Singh, H. K. Gulati, A. Singh, K. Kaur, G. Kaur, S. Sharma and A. Rana, ACS Omega, 2019, 4, 8720–8730 CrossRef CAS PubMed.
- A. Singh, S. Heer, K. Kaur, H. K. Gulati, N. Kumar, A. Sharma, J. V. Singh, K. Bhagat, G. Kaur and K. Kaur, Arch. Pharm., 2022, 355, 2200033 CrossRef CAS PubMed.
- X.-M. Zhang, H. Guo, Z.-S. Li, F.-H. Song, W.-M. Wang, H.-Q. Dai, L.-X. Zhang and J.-G. Wang, Eur. J. Med. Chem., 2015, 101, 419–430 CrossRef CAS PubMed.
- Y. Wang, Z. Liang, Y. Zheng, A. S.-L. Leung, S.-C. Yan, P.-K. So, Y.-C. Leung, W.-L. Wong and K.-Y. Wong, RSC Adv., 2021, 11, 18122–18130 RSC.
- E. I. Elmongy, A. A. Ahmed, I. E. T. El Sayed, G. Fathy, H. M. Awad, A. U. Salman and M. A. Hamed, Antibiotics, 2022, 11, 1507 CrossRef PubMed.
- A. Singh, K. Kaur, H. Kaur, P. Mohana, S. Arora, N. Bedi, R. Chadha and P. M. S. Bedi, J. Mol. Struct., 2023, 1274, 134456 CrossRef CAS.
- A. Singh, H. Kaur, S. Arora and P. M. S. Bedi, Arch. Pharm., 2022, 355, 2100368 CrossRef CAS PubMed.
- A. Selvaraj, A. Valliammai, P. Muthuramalingam, A. Priya, M. Suba, M. Ramesh and S. Karutha Pandian, ACS Omega, 2020, 5, 31100–31114 CrossRef CAS PubMed.
- T. Y. Hargrove, L. Friggeri, Z. Wawrzak, A. Qi, W. J. Hoekstra, R. J. Schotzinger, J. D. York, F. P. Guengerich and G. I. Lepesheva, J. Biol. Chem., 2017, 292, 6728–6743 CrossRef CAS PubMed.
- A. Singh, H. Kaur, H. Singh, B. Singh, P. M. S. Bedi and S. Kaur, J. Herbs, Spices Med. Plants, 2022, 1–10 Search PubMed.
- H.-F. Yang, A.-J. Pan, L.-F. Hu, Y.-Y. Liu, J. Cheng, Y. Ye and J.-B. Li, J. Microbiol., Immunol. Infect., 2017, 50, 55–61 CrossRef CAS PubMed.
- A. Singh, S. Sharma, S. Arora, S. Attri, P. Kaur, H. K. Gulati, K. Bhagat, N. Kumar, H. Singh and J. V. Singh, Bioorg. Med. Chem. Lett., 2020, 30, 127477 CrossRef CAS PubMed.
- T. L. Ng'uni, P. M. dos Santos Abrantes, C. McArthur, J. A. Klaasen and B. C. Fielding, J. Herb. Med., 2022, 32, 100503 CrossRef.
- A. M. Asiri and S. A. Khan, J. Heterocyclic Chem., 2012, 49, 1434–1438 CrossRef CAS.
- T. V. K. Reddy, A. Jyotsna, B. P. Devi, R. Prasad, Y. Poornachandra and C. G. Kumar, Eur. J. Med. Chem., 2016, 118, 98–106 CrossRef PubMed.
- M. Shafiei, H. Toreyhi, L. Firoozpour, T. Akbarzadeh, M. Amini, E. Hosseinzadeh, M. Hashemzadeh, L. Peyton, E. Lotfali and A. Foroumadi, ACS Omega, 2021, 6, 24981–25001 CrossRef CAS PubMed.
- L. Zha, Y. Xie, C. Wu, M. Lei, X. Lu, W. Tang and J. Zhang, Eur. J. Med. Chem., 2022, 236, 114333 CrossRef CAS PubMed.
- Y. Xie, L. Wang, Y. Yang, L. Zha, J. Zhang, K. Rong, W. Tang and J. Zhang, Front. Microbiol., 2022, 13, 1071255 CrossRef PubMed.
- Y. Song, C.-I. Liu, F.-Y. Lin, J. H. No, M. Hensler, Y.-L. Liu, W.-Y. Jeng, J. Low, G. Y. Liu and V. Nizet, J. Med. Chem., 2009, 52, 3869–3880 CrossRef CAS PubMed.
-
K. J. Bowers, E. Chow, H. Xu, R. O. Dror, M. P. Eastwood, B. A. Gregersen, J. L. Klepeis, I. Kolossvary, M. A. Moraes and F. D. Sacerdoti, Proc. 2006 ACM/IEEE Conf. Supercomputing, 2006, p. 84 Search PubMed.
- J. Prajapati, P. Rao, L. Poojara, D. Acharya, S. K. Patel, D. Goswami and R. M. Rawal, Curr. Microbiol., 2023, 80, 47 CrossRef CAS PubMed.
- J. Prajapati, D. Goswami, M. Dabhi, D. Acharya and R. M. Rawal, Comput. Biol. Med., 2022, 151, 106237 CrossRef CAS PubMed.
|
This journal is © The Royal Society of Chemistry 2024 |