DOI:
10.1039/D3MA00498H
(Review Article)
Mater. Adv., 2024,
5, 453-474
Porous nanomaterials for biosensing and related biological application in in vitro/vivo usability
Received
3rd August 2023
, Accepted 18th December 2023
First published on 19th December 2023
Abstract
Porous nanomaterials (PNMs) refer to materials that have a porous structure on the nanoscale, characterized by a network of interconnected pores or voids. These materials exhibit unique physical and chemical properties due to their nanoscale size and the presence of pores. With the continuous development in nanotechnology and materials science, significant advancements have been realized in the design and approaches for various applications. In this case, PNMs have attracted significant attention due to their excellent biocompatibility, high loading capacity, controllable designability, and advantages in optical imaging. Therefore, it is necessary to investigate the ongoing developments in porous structures, particularly their applications in biosensing and in vivo scenarios, to demonstrate the advancements and latest research trends in this field. In this review, we present the diverse applications of PNMs, including porous silicon structures with facile approaches and expandable physical or optical properties, resulting in an excellent bioimaging performance; as useful substrates with superior loading capacity and easy intracellularization for drug delivery; and their good biocompatibility, degradability, and flexibility, making them suitable for in vivo applications, which can be achieved through a self-assembly path. Furthermore, the constituents of PNMs are highlighted and the influence of different factors on their in vivo and in vitro (biocompatibility and cytotoxicity) characteristics demonstrated. All these characteristics are appropriate for in vivo applications, outlining the significant potential of PNMs for biological applications and biosensing. The results show that PNMs are viable alternatives in current research and clinical applications. In this review, each section discusses and summarizes representative examples and analyzes the use of PNMs in biomedicine. Finally, the future of PNMs in biomedicine is explored, which can help researchers in understanding their prospective development.
1. Introduction
In recent decades, encouraging progress has been realized in the biological applications of porous nanomaterials (PNMs).1,2 PNMs are materials with tunable pore sizes and specific pore structures. Nanoporous silica, nanometallic organic frameworks (MOFs), nanoporous polymeric membranes, and nanoporous metals are typical porous nanomaterials.3–6 PNMs exhibit high design flexibility, enabling their properties to be tuned through surface modification or alterations in pore structure. Their biocompatibility stems from the properties of the metal or substrate, rather than relying solely on their porous structure. PNMs have the advantage of being resistant to degradation by living organisms. Thus, they are widely applied in drug delivery, bioimaging, and biosensing, where the interplay between their structure and function significantly influences the modulation of biological tissue and cellular functions. Herein, we review the reported research on the use of porous nanoparticles for various physiological mechanisms, such as drug delivery,7 bioimaging,8,9 and biosensing,4 and their various applications in living organisms.
PNMs have the outstanding advantages of high loading capacity and tunable porosity, allowing different ligand modifications to create materials with specific targeting functions that can not only change shape or size but also have high sensitivity, high spatial resolution, and low toxicity for drug delivery and bioimaging. The inability of conventional oral drug delivery agents to deliver drugs at controlled release rates has generated interest and research in novel drug delivery methods. The developed drug delivery systems include polymer-based systems, MOF-based systems and mesoporous silicones. These different drug delivery routes are roughly classified into organic and inorganic systems. Organic systems such as polymer-based systems benefit from extensive biocompatibility and have the ability to absorb many drugs but lack controlled release mechanisms. Alternatively, inorganic delivery materials such as MOFs can deliver adsorbed drugs at a controlled rate due to their ordered porous network but have a reduced loading capacity. Most inorganic delivery materials have a mesoporous structure for optimal drug uptake and delivery (microporous materials usually lack a pore size large enough for useful drug delivery).10 As drug delivery systems, PNMs can deliver drugs, antigens and stimulatory molecules to target cells/tissues for cancer therapy through multiple pathways that modulate immune dysfunction in the tumor microenvironment.11,12 Cancer immunotherapy using PNMs can avoid multi-drug resistance, reduce genetic mutations in tumor cells, and enhance synergistic therapeutic effects with other treatments such as chemotherapy, radiotherapy, photodynamic therapy (PDT), and photothermal therapy (PTT).13 Furthermore, PNMs can be combined with other treatments such as PDT and PTT or used as radiosensitizers14 to achieve better anti-cancer effects.15 PNMs have inherent advantages such as high biomolecule loading capacity, adjustable structure, abundant surface modification and controlled release behavior of loading molecules such as immunomodulators.13,16,17 They can enhance cancer immunotherapy by delivering antigen and stimulatory molecules to target cells/tissues, modulating immune dysfunction in the tumor microenvironment and promoting ACT therapeutic effects in various ways.11,18
The development of biomedical imaging technologies has greatly facilitated the diagnosis of various diseases. PNMs as imaging agents can generate signals or enhance the signal contrast of the target tissue for better diagnosis. Nanoparticle imaging agents for cancer diagnosis preferentially accumulate in tumors through EPR effects for passive targeting or through tumor-specific receptor binding for active targeting19 and imaged using techniques such as positron emission tomography (PET), computed tomography (CT), ultrasound (US), and photoacoustic imaging (PAI). The combination of fluorophores with PNMs also enables optical sensing and imaging of physiologically significant species. Usually, fluorescence imaging is influenced by the intensity of tissue autofluorescence in in vivo bioimaging. Various tissue components for example, elastin and collagen, and intracellular molecules for example, amino acids such as flavin, tyrosine and phenylalanine are known to act as endogenous fluorophores in vivo. These biological chromophores strongly absorb visible light and limit the excitation and emission of light in the visible spectral range based on the penetration depth. Other biological components such as water and lipids also strongly absorb light in the visible to infrared ragion.20 The long-lived PL emission lifetime of PSiNP (typically in the order of a few microseconds) allows gated luminescence imaging to completely eliminate short-lived tissue autofluorescence, and thus gated luminescence imaging21 can be effectively used for bioimaging. Other PNMs can be loaded with substances such as lanthanides to enable nanospatial resolution thermography, time-resolved fluorescence immunoassay, etc. Magnetic substances such as iron oxide, magnesium oxide porous nanoclusters and paramagnetic/superparamagnetic metal ions as ligands can be used as contrast agents for magnetic resonance imaging (MRI),22 which have good biocompatibility and targeting properties, and also allow real-time monitoring of the contrast agents due to their magnetic properties, demonstrating good prospects in bioimaging. In conclusion, PNMs show potential to overcome current barriers to cancer immunotherapy and enhance anticancer efficacy, and also are highly attractive for bioimaging.
A sensor is a device that converts quantitative or qualitative chemical or biochemical information into an analytically useful signal as a result of a chemical interaction or process between the analyte and sensor device. The use of specific and sensitive sensors to detect and monitor changes (electrical, electrochemical, optical and thermal signals) in biological elements (enzymes, antibodies, tissues or living cells) when they come in contact with sensitive materials can help diagnose diseases and reveal underlying biological problems.19 These changes can be in the form of electrical (conductance, resistance and capacitance), electrochemical (conductance, amperage, impedance and potential), optical (reflection, photoluminescence and fluorescence) and thermal (temperature change) signals. Biosensors have a wide range of applications, including glucose monitoring, pathogen detection, quantitative measurement of toxicity and bioactivity assessment of new compounds.23 Porous nanomaterials often have porous structures to allow the entry of multiple active substances (such as enzymes, proteins and molecules), and thus they can be applied in many different types of biosensors, allowing the analysis of many different types of biomolecules (such as enzymes) and their binding to specific targets,24 such as label-free sensing substrates based on silicon nanowires for the detection of bilirubin25 and the use of label-free electrochemical adaptive sensor with bimetallic hollow MOF for effective detection of adenosine.26
Therefore, it is of great significance to study the latest progress in biosensing and in vivo applications of porous nanoparticles and introduce their latest technologies. This article reviews the latest advances in various PNMs in medical applications such as drug delivery, bioimaging and biosensing, emphasizing the biocompatibility, degradability and flexibility of porous nanomaterials, making them suitable for in vivo applications through self-assembly pathways. This property shows great potential in the biomedical field, providing researchers with new possibilities. In the future, the application of porous nanomaterials is expected to achieve greater breakthroughs through more precise regulation of the size and shape of porous structures, and modification of their functional groups. Herein, we also emphasize the interdisciplinary nature of porous nanomaterials research, encouraging collaboration and interdisciplinary research to accelerate their development and innovation of their applications. These studies show that PNMs have great potential as an alternative to current clinical applications. Each section includes an in-depth discussion and summary of representative examples to highlight the wide range of applications of PNMs in the biomedical field. By analyzing the latest research trends, we also provide researchers with deep insight into the future direction of PNMs in the biomedical field. This not only provides scientists with a comprehensive understanding of the current status of PNMs in biomedicine, but also provides strong guidelines for future research and innovation. Overall, porous nanomaterials show broad prospects for in vivo/in vitro applications and biosensing, providing strong support for the development of the medical field.
2. Silicon-based assembly materials
Porous silicon (PS) is a nanostructured material that offers a wealth of advantages to the current materials community. As its name implies, porous silicon is similar to quantum sponge, containing a meshwork of nanocrystals and pores.27 Also, because silicon is readily available and inexpensive, it is an attractive and useful material for diverse applications. Porous silicon nanoparticles (PSiNPs) have outstanding properties including biocompatibility coupled with flexible pore morphology, structural properties, biodegradability, and unique optical and luminescent properties, making them preferable for drug delivery, bioimmunochemistry, bioimaging and biosensing applications (Fig. 1a).28 It was shown that PSiNPs do not cause inflammatory responses in animal models and show good biocompatibility, gradually degrading to non-toxic silicic acid in aqueous media.29 PSiNPs as drug delivery systems (DDS) have the advantages of high loading, good biocompatibility, and ease of functionalization (Fig. 1b), where their unique properties and advantages as nanomedicines can be summarized as follows: (1) they can be used as multifunctional platforms or therapeutic agents for diagnosis and treatment by integrating multiple imaging techniques; (2) their large specific surface area and internal volume allow for simple immersion or chemical adjuvant loading to introduce large amounts of cargo; (3) ligand modifications can be used as physicochemical optimization of targeted drugs and nanoparticles, allowing simultaneous drug delivery and imaging of targeted disease sites; and (4) fine-tuned control of PSiNPs to increase circulation time in the blood by reducing the conditioning effect and uptake of the mononuclear phagocytic system (MPS).30 To date, poor water solubility remains a major factor limiting the efficacy of drugs; accordingly, by incorporating drug molecules in PSiNPs, premature drug degradation can be effectively prevented while maintaining complete control of the drug release.31 PSi nanodrug delivery has abundant flexibility to achieve drug delivery by oral, intravenous or subcutaneous administration, and accordingly, it is predicted that lower doses of conventional anticancer agents can be used to achieve better therapeutic results.32 Furthermore, PSiNPs can be used as subcutaneous implants to deliver trace minerals such as iodine, selenium, chromium, manganese and molybdenum as dietary supplements and other therapeutic agents such as lithium for depression, gold and silver with antimicrobial properties or platinum and palladium for oncologic diseases.33
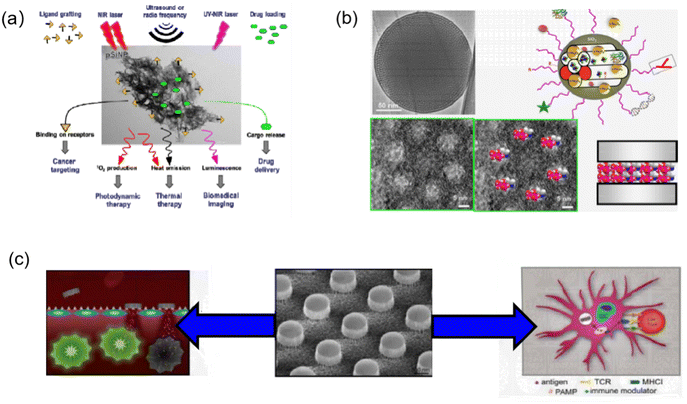 |
| Fig. 1 (a) Multifunctionalities of PSiNPs for cancer theranostics. (b) Schematic illustration of drug loading in the mesopores of mesoporous silica nanoparticles (MSNs). Top left image: Transmission electron micrograph of MSN. Top right illustration: Schematic depiction of an MSN showing its role as a versatile multifunctional nanoplatform. Bottom left image: Enlargement of the above-mentioned image, where the ordered hexagonal arrangement of the pores to be loaded is clearly seen. Bottom center: Depiction of drug molecules loaded in mesoporous channels. Bottom right: Schematic representation of a horizontal perspective of silica channel loaded with drug molecules. (c) Schematic overview presenting porous silicon microparticle fabrication and applications in drug delivery and immunotherapy the central image is a scanning electron micrograph showing a patterned pSi wafer prior to sonication-based release of the patterned particles. The multistage vector concept of drug delivery is shown on the left, with intravascular administration of pSi particles resulting in tumor-associated vascular accumulation based on geometric properties, charge or targeting ligands. Images reprinted with permission from Bimbo et al. (2010, ACS), Kulyavtsev et al. (2017, Pharm. Pat. Anal.) and Serda et al. (2015, US8926994), respectively. | |
The Jeong research group utilized the condensation chemistry of calcium silicate to load the rheumatoid arthritis drug methotrexate (MTX) in PSiNPs. Systemic administration of the constructs formed by PSiNPs loaded with MTX, known as pCaSiNPs, resulted in its accumulation in inflamed joints, leading to improvements in the progression of rheumatoid arthritis in both early and established stages. The biological degradation products of the pCaSiNP drug carrier exhibit immunomodulatory and anti-absorptive effects, emphasizing their crucial role in the biocompatibility of implantable devices.34 Studies on the different pathways of absorption, distribution and excretion in mice have shown that PSiNPs have good tissue biocompatibility for oral and intravenous administration.35 PSiNPs have been shown to deliver large biomolecules that can be used for immunotherapy (Fig. 1c). Zhang et al. from Wuhan University co-loaded silica nanoparticles with a hypoxia-activated prodrug (HAP) and a vascular disruptor, and then coated the particles with platelet membranes. Once in the tumor, this nanotherapy could disrupt local blood vessels, and thus inhibit the tumor.36 Ferrari et al. of the Methodist Hospital Research Institute developed PSiNPs functionalized with toll-like receptor ligands as adjuvants for anticancer immunity. It was shown that mesoporous PSiNPs conjugated with lipopolysaccharide (LPS) or monophospholipids could be readily internalized by dendritic cells.37 The Sailor group used the optical properties of PSiNPs to design immune protocols by loading antigens in luminescent PSiNPs (LPSiNPs). The antigens were loaded in PSiNPs with antibodies that are designed to selectively target and induce T cell-mediated immune responses.38 Kim et al. proposed that nanoparticles can target activated macrophages and loaded them with siRNAs targeting the Irf5 gene. This formulation could induce >80% gene silencing in activated macrophages in vivo and inhibit excessive inflammatory responses, producing significantly improved therapeutic outcomes in a mouse model of bacterial infection.39
The unique advantages of PSiNPs, such as good compatibility, inherent photoluminescence (PL), high surface area and ease of surface functionalization, make PSiNPs-based contrast agents (CAs) clinically suitable for biomedical imaging.40 The high surface area of PSiNPs allows the loading of different CAs for different imaging modalities. The potential bioimaging methods using PSiNPs include optical imaging, MRI, positron emission tomography (PET), and single-photon emission computed tomography (SPECT) imaging. Cunin et al. successfully constructed multifunctional PSiNPs to deliver Ru(II) complex photosensitizers for near-infrared imaging and photodynamic therapy (PDT).41 Powerful NIR imaging capabilities and effective inhibition of cell growth were observed in vitro under NIR irradiation. Zabotnov et al. used picosecond laser ablation of porous silicon films and nanowires to synthesize small silicon nanoparticles (14–65 nm) with fluorescence emission in the NIR range (600–1000 nm), which created a new prospect for use as optical imaging contrast agents.42 Xia et al. fabricated PSiNPs@Fe3O4 nanocomposites for dual-mode imaging by covalently bonding superparamagnetic Fe3O4 NPs with photoluminescent PSiNPs.43 In this nanocomposite, PSiNPs were used as the NIR imaging agents and Fe3O4 NPs the CAs for MRI. This nanocomposite exhibited good biocompatibility and performed well in fluorescence/MR bimodal imaging of cells in vitro and tumors in vivo. Microsecond photoluminescence is a unique feature of PSiNPs for bioimaging. Kim et al. reported the fabrication of iRGD-PEG-modified PSiNPs for two-photon imaging of living animals. By limiting the size of PSiNPs to 60 nm and increasing the two-photon absorption cross section, the photoluminescence intensity of NPs and the signal-to-noise ratio of two-photon imaging significantly improved.2 In addition, the tumor-targeting effect of 60 nm PSiNPs-iRGD was confirmed by experiments in a mouse orthotopic HeLa tumor model. Park et al. used dextran-coated LPSiNPs (D-LPSiNPs) for tumor imaging, demonstrating luminescent porous silica nanoparticles (LPSiNPs) that can carry drug payloads with inherent near-infrared photoluminescence capable of monitoring accumulation and degradation in vivo (Fig. 2) with low toxicity degradation pathways suitable for in vivo applications.44 Overall, PSiNP-based nanosystems have great potential for bioimaging, given that their long-lived photoluminescence can be utilized to eliminate the interference of tissue autofluorescence. This enhances the differentiation of CA from endogenous fluorophores of living tissues.
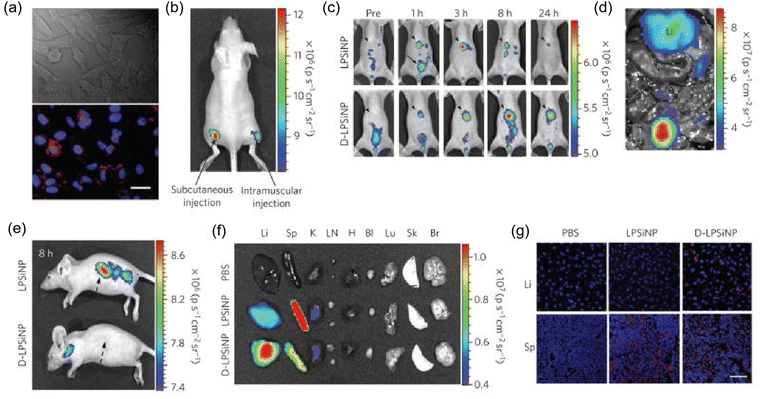 |
| Fig. 2 (a) In vitro cellular imaging with LPSiNPs. HeLa cells were treated with LPSiNPs for 2 h, and then imaged. Red and blue indicate LPSiNPs and cell nuclei, respectively. The scale bar is 20 μm. (b) In vivo fluorescence image of LPSiNPs (20 μL of 0.1 mg mL−1) injected subcutaneously and intramuscularly on each flank of a mouse. (c) In vivo images of LPSiNPs and D-LPSiNPs. The mice were imaged at multiple time points after intravenous injection of LPSiNPs and D-LPSiNPs (20 mg kg−1). Arrowheads and arrows with solid lines indicate liver and bladder, respectively. (d) In vivo image showing the clearance of a portion of the injected dose of LPSiNPs in the bladder, 1 h post-injection. Li and Bl indicate liver and bladder, respectively. (e) Lateral image of the same mice shown in (c), 8 h after LPSiNP or D-LPSiNP injection. Arrows with dashed lines indicate spleen. (f) Fluorescence images showing the ex vivo biodistribution of LPSiNPs and D-LPSiNPs in a mouse. Organs were collected from the animals shown in c, 24 h after injection. Li, Sp, K, LN, H, Bl, Lu, Sk and Br indicate liver, spleen, kidney, lymph nodes, heart, bladder, lung, skin and brain, respectively. (g) Fluorescence histology images of liver and spleen from the mice shown in (c) and (f), 24 h after injection. Red and blue indicate (D-)LPSiNPs and cell nuclei, respectively. The scale bar is 50 μm for all images. Images reprinted with permission from Park et al. (2009, Nat. Mater.). | |
PSiNPs have captivating and tunable features including easy fabrication, special optico-physico properties, tailored morphological structure and versatile surface chemistry enhancing their prospects as a transducer for the fabrication of biosensors. Furthermore, PSiNPs can be used for other biomedically relevant assays such as glucose, DNA, bacteria, viruses and proteins. Syshchyk et al. fabricated biosensors for the determination of glucose and urea based on the fact that the photoluminescence of porous silicon varies with the pH of the medium.45 Experiments showed that the photoluminescence intensity of porous silicon increased by 1.7-fold when the glucose concentration increased from 0 to 3.0 mM, whereas it decreased by 1.45-fold when the urea concentration was increased to the same level. Also, the presence of heavy metal ions (Cu2+, Pb2+ and Cd2+) in the solution inhibited the enzymatic reactions catalyzed by glucose oxidase and urease, thus restoring the photoluminescence quantum yield of porous silicon, and thus this biosensor could also be applied for the inhibition analysis of heavy metal ions (Fig. 3a). Zhang et al. proposed a non-spectral porous silicon optical biosensor technique based on dual-signal light detection of the concentration of DNA molecules.46 In the dual-signal light detection method, the first detection signal light is the detection light reflected from the surface of a porous silicon Bragg mirror. The second detection signal light is fluorescence, and the Bragg mirror structure further enhances the fluorescence signal. After receiving digital images of the two signal lights superimposed on the porous silicon surface simultaneously using a digital microscope, a corresponding algorithm is used to calculate the change in the average gray value before and after the hybridization reaction to calculate the concentration of DNA molecules (Fig. 3b). This method allows the detection of target DNA not only by hybridization, but also by immunoreactivity or parallel biochips with porous silicon biosensors to detect antigens. Vercauteren et al. used PSi membranes (PSiMs) for the optical detection of Bacillus cereus lysates (Fig. 3c). Prior to detection, the bacteria were selectively lysed by PlyB221, an endolysin encoded by Deep-Blue, which is a phage targeting Bacillus cereus. This detection platform not only enabled the rapid detection of bacteria, but the same technique could be extended to other bacteria by selective lysis, such as the detection of Staphylococcus epidermidis and selective lysozyme lysis.47 Layouni et al. combined peptides with a multifunctional PSi optical biosensor platform for the detection of the chikungunya virus E2 protein. Peptide functionalization and selective E2 protein capture were confirmed by contact angle measurements, attenuated total reflection-Fourier transform infrared spectroscopy and optical reflectance measurements, and this biosensor could be exposed to high-temperature environments.48
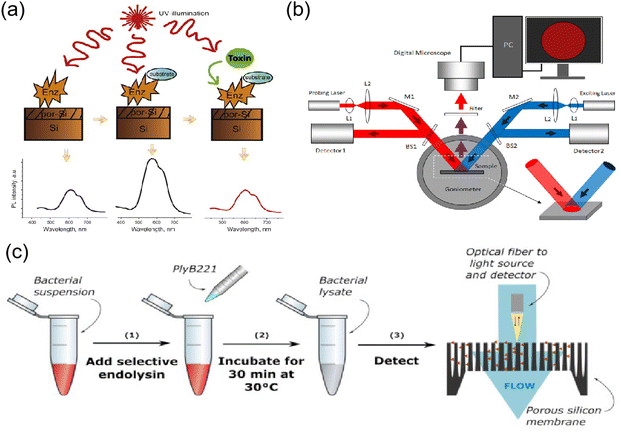 |
| Fig. 3 (a) Schematic of luminescent biosensors based on GOD or urease for the detection of glucose, urea and heavy metals. (b) PS optical biosensor with different concentrations of DNA by the double-signal optical detection mechanism. (c) Protocol for the detection of bacteria on a PSi membrane. Images reprinted with permission from Syshchyk et al. (2015, Biosens. Bioelectron.), Zhang et al. (2022, Sensors (Basel, Switzerland)) and Vercauteren et al. (2021, Biosensors), respectively. | |
3. Porous assembly structures based on metal organic frameworks (MOFs)
Since the pioneering work by Yaghi's in 1995,49 an increasing number of researchers have been focused on the design and application of MOFs. Metal–organic frameworks (MOFs) are constructed based on organic and inorganic building blocks, with vacant spaces (pores) arising between individual structural units. As a new class of porous organic–inorganic crystalline hybrid materials, MOFs are dominated by the self-assembly of metal atoms and organic backbones, and some functional species including small biomolecules, metal ions, and magnetic groups can be loaded in the pores of the framework to confer functions. In addition, some MOFs containing lanthanides or luminescent organic ligands can generate fluorescence or phosphorescence under UV light.1 Due to these superior properties of MOFs, they have been widely used in different types of applications such as sensing,50 drug delivery,51 bioimaging52–54 and other bio-related applications.55
Metal organic backbones (MOFs) with luminescent properties and size- or shape-selective adsorption properties can be used as sensor devices. nMOF-based sensors can satisfy the requirements of high sensitivity, resolution and accuracy for cellular biosensing. The pH dysregulation of intracellular fluids is associated with tumorigenesis and drug resistance, and real-time sensing and monitoring of intracellular pH changes in living cells is important for exploring disease mechanisms and designing pH-responsive intracellular drug delivery systems. Lin and colleagues reported the first nMOFs for real-time intracellular pH sensing.56 A fluorescein moiety was attached to Zr-based UiO nMOFs via thiourea bonds, with two excitation wavelengths (435 vs. 488 nm) associated with pH-dependent relative fluorescence intensity. They were imaged by live cell confocal microscopy to illustrate the acidification process during endosome maturation with high spatial resolution and fast temporal response. Various diseases, such as cancer and vascular diseases, can lead to tissue hypoxia and reduced oxygen levels. Therefore, intracellular oxygen sensing is important for the diagnosis and treatment of cancer. Lin et al. designed the first phosphorescent/fluorescent dual-emission nMOF for intracellular oxygen quantification.57 A hybrid ligand M-nMOF containing the oxygen-responsive phosphorescent ligand Pt-5,15-bis(p-benzoate) porphyrin and the amino-functionalized ligand amino-quaternary ammonium phenyl dicarboxylic acid was first synthesized. Subsequently, rhodamine B was covalently attached to the amino group via a thiourea bond as an oxygen-independent reference to provide R-nMOF. These two chromophores share the same excitation energy, while their phosphorescent/fluorescent emission does not interfere with each other. Subsequently, a ratiometric photoluminescence method for quantifying oxygen was established, allowing the confocal microscopic imaging of living cells by R-nMOF to determine the oxygen levels in hypoxic, normoxic, and aerated cells (Fig. 4a). Several metal ions play a key role in cellular activity, but their presence at high concentrations can be toxic and lethal. Accordingly, the selective detection of metal ions in cells is required for diagnostic purposes. Because energy/charge transfer between ligands and metal ions leads to fluorescence burst, MOFs can carry the corresponding ligands for their detection. Lu et al. developed nMOF-253 with 2,2′-bipyridine-5,5′-dicarboxylic acid (bpydc) ligands for the detection of Fe2+.58 The fluorescence of the ligand was selectively burst by Fe2+, which is probably due to light-induced electron transfer, allowing nMOF to be used for intracellular Fe2+ sensing in HeLa cells. NMOF-based systems have also been developed for the detection of the metal ion Cu2+ based on the luminescence burst of Tb3+ ions.59
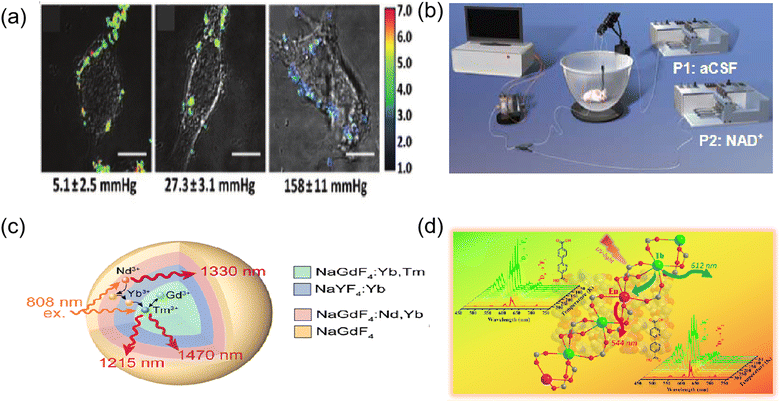 |
| Fig. 4 (a) Ratiometric imaging of CT26 cells incubated with biosensor based on R-UiO under hypoxia (left), normoxia (middle), and aerated (right) conditions. (b) Schematic illustration of the online electrochemical detecting system for in vivo monitoring of glucose in cerebral system. (c) Schematic of the NaGdF4:Yb, Tm@NaYF4:Yb@NaGdF4:Yb, Nd@NaGdF4 nanocomposite as a dual ratiometric luminescence nanothermometer (DRLNT). (d) Characterization of Ln3+-based metal–organic frameworks (LnMOFs) compounds by single-crystal X-ray diffraction (XRD), powder XRD, and thermogravimetric analysis. Images reprinted with permission from Xu et al. (2016, J. Am. Chem. Soc.), Ma et al. (2013, Anal. Chem.), Hu et al. (2022, Nanoscale Horiz.) and Zhao et al. (2019, Inorg. Chem.), respectively. | |
Ma et al. prepared an integrated dehydrogenase electrochemical biosensor (Fig. 4b) based on zeolitic imidazolate frameworks (ZIFs) as a substrate for the construction of an integrated dehydrogenase electrochemical biosensor with high sensitivity to glucose for in vivo measurement of neurochemicals.60 It was demonstrated that ZIFs are capable of acting as substrates for immobilizing electrocatalysts (i.e., methylene green, MG) and dehydrogenases (i.e., glucose dehydrogenase, GDH) on the electrode surface, exhibit excellent adsorption capacity for MG and GDH, and are easy to form integrated electrochemical biosensors, and thus were used as substrates for our glucose biosensor. The ZIF-based biosensor was shown to be more selective for glucose than other endogenous electroactive substances in the brain system and could selectively monitor dialysate glucose collected from guinea pig brains in a near real-time mode.
In the case of Ln-based nMOFs, as thermal probes, they were evaluated as multifunctional luminescent thermometers, which are luminescent mixed lanthanide MOF thermometers capable of directly visualizing temperature changes with an excellent linear correlation between temperature and luminescence intensity ratios in the range of 50–200 K.61 This was first investigated on the microscale, and more recently on the nanoscale. Zhao et al. prepared two types of isostructural Ln3+-based metal–organic skeletons under solvent heating conditions, in which two structurally similar pyridine-containing dicarboxylic acid ligands, i.e., 6-(4-carboxyphenyl)nicotinic acid and [2,2′-bipyridyl]-5,5′-propanedioic acid, were used as organic connectors. Two hybrid LnMOFs, namely, Tb0.95 Eu0.05 cpna and Tb0.95 Eu0.05 bpydc, were obtained by the lanthanide co-doping method and evaluated for their application as proportional luminescence thermometers.62 It was experimentally confirmed that both materials exhibited a good S-shaped response in the temperature range of 25–300 K with good relative sensitivity and temperature uncertainty (Fig. 4d). In addition, their color changes from green at 25 K to red at 300 K make them suitable as colorimetric luminescence probes. Hu et al. used a rare earth-doped nanocomposite of Tm3+ and Nd3+ ions as emitters to construct a dual-scale LNT.63 A strategy for enhanced Tm3+ NIR radiation for bioimaging-based in vivo temperature detection was developed using cross-relaxation processes between lanthanide ions (Fig. 4c). The dual-ratio probe components in the nanocomposites have the potential to monitor temperature differences and heat transfer at the nanoscale, which will help regulate heating operations more precisely in thermotherapy and other biomedical applications. This work not only provides a powerful tool for in vivo temperature sensing, but also presents a method for building highly efficient NIR-II/III rare earth luminescent nanomaterials for a broader range of biological applications. These pioneering studies highlight the possibility of using nano MOFs for monitoring physiological temperature in medical applications, which can be further explored with nanoscale hybrid rare earth MOFs. These novel luminescent thermometers can be further applied in the near future as nanothermometers for intracellular sensing and thermal imaging with nanospatial resolution.
Inspired by early studies on the development of polymer-based drug delivery systems, several researchers have attempted to develop MOF-based nanoparticles as drug carriers for targeted cancer therapy.64–66 MOFs have unique advantages as potential drug carriers, as follows: (1) highly tunable properties and porosity, (2) high drug loading capacity, and (3) controlled multifunctionality. MOF-based drug delivery systems such as pH-responsive delivery systems, magnetically guided delivery systems, folate-targeting delivery systems, and temperature-responsive delivery systems have been developed. Various structures of MOFs are available as carriers for different drugs, some of which have a loading capacity of up to 81.6% ± 0.6%.67 Choudhuri et al.68 designed a pH-responsive DDS drug delivery system that can be used to construct multifunctional nanoparticles in a simple and rapid way, which combines upconversion nanoparticles (UCNPs) and MOFs. The outstanding advantages of encapsulated targeting (Fig. 5a) make it suitable for releasing drug molecules in the tumor region. We adsorbed 5-FU as a model drug in UCNP@ZIF-8/FA nanocomposites at 37 °C and pH 5.5 and 7.4. Finally, we observed that the 5-FU release process has a significant pH response, as shown in Fig. 5b. This showed that the release behavior of 5-FU changed significantly under different pH environments, highlighting the controllability of the nanocomposite in drug release. Ke et al. reported the synthesis of a core–shell nanomaterial (Fe3O4@MOF-5) consisting of Fe3O4 nanorods (magnetite) and nanocrystals of MOF-5.69 Nimesulide was selected as the model drug and loaded in the pores of Fe3O4@MOF-5. The experimental results showed that a high drug loading rate was achieved (20 wt%), while the incorporated drug exhibited a rapid response to a magnetic field. Magnetic drug delivery (MDD) is one of the most promising targeting drug delivery systems. With the help of external magnetic conditions, magnetic drug agents can be precisely targeted to a specific location, while magnetic properties also allow the real-time monitoring of magnetic agents, which can be used as contrast agents for bioimaging.70 The folate receptor (FR) is overexpressed in some tumor tissues, and when MOFs are encapsulated by folic acid, they will be carried into the cell and take up folic acid more readily than in normal tissues. For example, Li et al. reported the synthesis of a nanoscale metal–organic framework, DOX@UiO-68-FA, which was decorated with UiO-68 and folic acid.70 They performed cell biocompatibility and membrane permeability experiments to demonstrate that DOX@UiO-68-FA had higher cellular uptake in Hep G2 cells compared to DOX@Mi-UiO-68 and free DOX. Furthermore, the in vivo antitumor assays showed that among free DOX, DOX@Mi-UiO-68 and DOX@UiO-68-FA, DOX@UiO-68-FA exhibited the highest antitumor effect in PBS. Tavakolizadeh et al. designed a novel UiO-66 MOF and MXene nanocomposite modified with rosemary (RO) leaf extract to co-deliver doxorubicin (DOX)/clustered regularly spaced short palindromic repeats (CRISPR). For the first time, the synthesis of nanomaterials was completed with the aid of a super-gravity system and a rotating packed bed device. The modeling results confirmed the successful synthesis of the nanomaterials. Based on the drug release and loading results, the DOX payload efficiency of around 46% was recorded and sustained drug release at acidic pH (the same as cancer cells) was observed.71 Wang and colleagues constructed a nanoscale metal–organic framework, CaZol, using zoledronic acid and calcium, and then targeted by drug-free Fol liposomes to incorporate folic acid as a targeting ligand in CaZol.72 The cellular uptake experiments showed that Fol-targeting CaZol showed a higher uptake efficiency than non-targeting CaZol in H460 and PC3 cells. Also, the biodistribution experiments in thymus-free nude mice showed that Fol-targeting CaZol accumulated in tumors at higher amounts than non-targeting CaZol under the same conditions. The temperature in vivo usually does not vary significantly, and in this case, temperature-responsive drug carriers can be manufactured based on an external thermal field. For example, Lin et al. synthesized two MOFs, ZJU-64 and ZJU64-CH3, using zinc nitrate, adenine and carboxylate ligands. Methotrexate (MTX) was added to these MOFs as a model drug according to the impregnation method.73 In the drug release assay, methotrexate was released from the framework and exhibited temperature-responsive phenomena. When the release progress was measured at 60 °C, ZJU-64 and ZJU-64-CH3 released MTX from their frameworks in approximately 1.5 and 6 h, respectively, which is equal to the amount released at 37 °C for 72 h. At present, only a few studies have been reported on the thermo-responsive release of MOF-based drug carriers, which may be due to the fact that the in vivo temperature is basically stable at 37 °C and it is difficult to realize significant changes to trigger drug release.
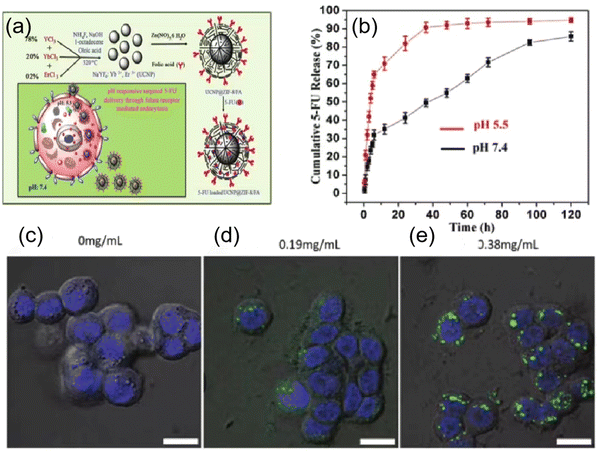 |
| Fig. 5 (a) Schematic representation of encapsulated process of upconversion NMOFs modified with folic acid and pH responsive drug delivery (left). (b) In vitro 5-FU release profiles from 5-FU@UCNP@ZIF-8/FA measured at pH 5.5 and 7.4 at 37 °C (right). (c) and (d) Confocal fluorescence images of the DRAQ5 channel (blue, nuclear stain) and the BDC-NH-BODIPY channel (green) of HT-29 cells incubated with no particles 0.19 mg mL−1 and 0.38 mg mL−1 particles, respectively. (e) Confocal microscopy images of H460 cells incubated with various nanoparticles. Images reprinted with permission from Xiao et al. (2011, J. Mater. Chem.), Taylor-Pashow et al. (2020, JCIM) and Xu et al. (2009, J. Am. Chem. Soc.), respectively. | |
MOFs have attracted great interest in the field of bioimaging due to their easy intracellularization, good dispersion stability and good bioavailability. Also, the surface of MOFs can be decorated with silica, organic polymers and lipid bilayers to improve their stability and confer biocompatibility.74 Thus, new hybrid materials can be used as contrast agents for magnetic resonance imaging (MRI) by incorporating a large number of paramagnetic/superparamagnetic metal ions. For example, Wilasinee and colleagues reported the synthesis of a Gd-based NMOF as a contrast agent for MRI.75 A simple route to control nanoparticles such as sodium salicylate (NaSal), salicylic acid and their derivatives, and other hydrophobic compounds were used in the synthesis process, as shown in this paper. Most NMR studies demonstrated that Gd-based MOF nanoparticles show higher T relaxation than conventional magnetic materials. Some MOFs with luminescent building blocks (ligands or metal ions) or bound fluorescein can be used for optical imaging (OI). Lin et al. reported the preparation of an Fe(III)-based MOF with 2-aminoterephthalic acid, whose structure is very similar to MIL-101.76 An optical contrast agent (BODIPY dye) and an ethoxysuccinic acid-cisplatin anticancer prodrug were successfully integrated in the NMOFs by post-synthetic modification. To evaluate the in vitro effect of this nanoparticle as an optical contrast agent, a laser scanning confocal microscopy study was performed on HT-29 human colon adenocarcinoma cells. The results showed that the fluorescence of BODIPY appeared in the cells incubated with the nanoparticles, while no fluorescence was observed in the control group (Fig. 5c–e). These results suggest that nano-sized MIL-101 (iron) particles provide an effective platform for delivering optical contrast agents in vitro. MOF optical imaging probes are limited by their poor penetration and difficulty reaching the interior of cells. However, the rational combination of an imaging modality and contrast agent can provide advantages in intraoperative imaging and image-guided cancer surgery. Iodinated ligands or iodide loads can be used for X-ray computed tomography. Zhang et al. found that MOF nanocrystals containing iodine-boron diphenyl ether (BODIPY), which are known as UiO-PDT, exhibited strong X-ray attenuation in in vitro CT imaging and preferentially aggregated at tumor sites. More importantly, no significant side effects were observed for this nanocrystal at all the tested injection doses.77
4. Porous polymer membranes
Porous polymer membranes show great potential for biological and biomedical applications such as tissue engineering, bioseparation and biosensing due to their structural flexibility, multiple surface chemistry and biocompatibility.78 Furthermore, due to their unique properties, such as tunable pore size and shape, density, and large surface-to-volume ratio, they can bind a large number of functional groups in their pores and have a wide range of applications, including separation, such as water filtration and hemodialysis, support scaffolds, such as tissue engineering, and diagnostic tools, such as sensing platforms.79,80Fig. 6a presents a summary of the latest technologies and future prospects for the biomedical applications of polymeric membranes. The biocompatibility and biodegradability of membranes are crucial factors for their application.81 Surface properties determine the degree of biocompatibility, and thus the membrane surface must often be modified to enhance its interactions with biological samples or inhibit immune responses.82
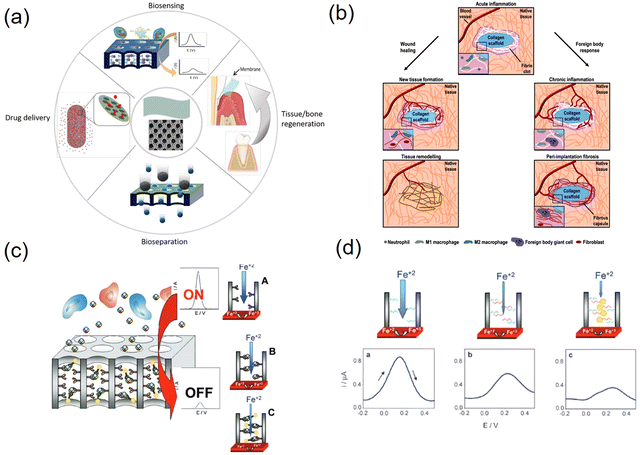 |
| Fig. 6 (a) Overview of biomedical/biological applications of porous polymeric membranes. (b) Mechanism of the two possible pathways of acute inflammation. In acute inflammation neutrophils, monocytes, and macrophages are observed. The left scheme corresponds to a wound healing process and the right scheme corresponds to a foreign body response. In wound healing, tissue remodelling occurs at the end of the process. In foreign body response, acute inflammation remains for a long period. (c) Mechanism of nanochannel blockage electrochemical sensing. (d) Differential pulse voltammograms (DPVs) of (a) only bioreceptor is attached on the channel walls and (b) after target analytes are captured by the bioreceptor and block the pores. Images reprinted with permission from Shiohara et al. (2020, J. Mater. Chem. B), Delgado et al. (2015, Tissue Eng., Part B), de et al. (2011, Small) and de et al. (2010, Chem. Commun.), respectively. | |
One of these drug delivery systems (DDS) has been extensively studied, given that controlled drug delivery to the target site is currently in great demand to reduce the serious side effects that occur when drugs come into direct contact with normal cells. The current drug delivery methods are mainly intravenous and oral. However, these methods have important drawbacks such as poor drug solubility and biodistribution, rapid drug breakdown, and extravasation of tissue damage. DDS aims to reduce these drawbacks by maximizing the therapeutic efficacy, drug release time, and drug stability in the body, increasing the bioavailability and reducing the adverse effects of drugs.83 Porous polymer membranes are used in DDS to control the diffusion of drug molecules by regulating their permeation and drug release. Yang et al. prepared cylindrical nanochannels via the self-assembly of PS-b-PMMA block copolymers to demonstrate the biotherapeutic, controlled release of human growth hormone (hGH) in vitro and in vivo.84 As shown in Fig. 6b, the porous polymer membranes induced fibroblast chemotaxis and hemostasis for wound healing with a lower immune response compared to many other synthetic polymers (e.g., polystyrene and polyethylene).85–88 For use in tissue engineering, biopolymer membranes have several advantages compared to synthetic membranes in that they offer good biocompatibility, biodegradability, inherent bioactivity, ability to attract cells, and susceptibility to cell-triggered protein degradation and natural remodeling.
Polymers are ideal materials for the fabrication of disposable biosensors and are beneficial for biomedical applications. Porous polymer membranes can be used in biosensor strategies to limit the diffusion of interfering high-molecular-weight biological agents (e.g., large proteins), while still allowing the diffusion of low-molecular-weight solutes (e.g., metabolites) to the surface of the sensor for their detection.89 They can also selectively capture target analytes by interacting with bioreceptors immobilized on the surface of the nanopore/nanochannel.90,91 Porous polymer membranes can be used to modify the surface of working electrodes to improve their sensitivity, for example, by introducing nanoscale pores that not only increase the surface area but also can be used as nanopores where biometric events can be confined, maximizing binding compared to the use of conventional non-nanostructured electrodes. These pores can also be used as filters to minimize interference.92,93Fig. 6c and d depict an example of a sensing platform that utilizes nanopore plugging caused upon analyte binding as the sensing mechanism, as well as the expected electrochemical response. Bioreceptors are immobilized on nanopore structures ready to bind to the target analyte, resulting in changes in the measured electrochemical signal due to partial nanopore blockage.94,95 Sadeghi et al. reported a unique method for the electrostatic separation of organic molecules of similar size based on their creation of nanoporous membranes with filled arrays of polymer micelles in alcohol.96 Overall, the combination of porous polymer membranes with other technologies to dope other materials or explore new polymeric materials with special properties such as high conductivity and high electrocatalytic activity adds new opportunities and may also help overcome the drawbacks of each technology and the disadvantages of porous polymer membranes such as poor stability at high temperatures or in organic solvents, poor electrical conductivity and low electrocatalytic activity to advance the development of biosensors.
5. Metal-based porous structures
5.1 Monometallic-based porous structures
Porous metal-based materials have long been known as good biomaterials and drug carriers97,98 and have good prospects for application in biological applications and biosensing. In early studies, metallic materials were one of the candidates for metal implants in the human body due to their sufficient strength and suitable mechanical properties,99 and other properties, as follows: (1) porous metal has good biocompatibility, stiffness and porosity and (2) porous metal provides open space for the inward growth of bone tissue, thus accelerating the osseointegration process.100–102 The unique bone-like properties of porous tantalum allow it to be used as a material for large implants, not just as a coating like other porous metals. In addition, porous tantalum also promotes the growth of soft tissues,103 including the formation of blood vessels, which are found assembled on the surface of porous tantalum and inside the structure.104 Porous tantalum has a trabecular geometry that can be used to simulate bone tissue, as well as open cell structures (Fig. 7a). The mechanical properties of porous materials significantly depend on their geometric design, including their porosity and unit cell type. The porosity of all types of human bones shows a gradual change from a dense outer cortex to a spongy inner cancellous tissue. Therefore, porous biomaterials must mimic the natural progressive structure of the human skeleton. Li et al. demonstrated that porous Fe and Zn with a precisely controlled geometry can be successfully prepared using SLM and possess mechanical properties comparable to that of human bone trabeculae.105,106 However, although porous nanoparticles significantly increase the available surface area, there is a lack of theoretical understanding and scarce experimental information on how their porosity is controlled or it dominates their stability. As shown in Fig. 7b, Felipe et al. used classical molecular dynamics simulations to elucidate the specific characteristics and properties of gold porous hollow nanoparticles and how they differ from nonporous nanoparticles,107 demonstrating how porosity introduces surface stresses and causes minor transitions in various scenarios with an increase in temperature, improving the stability of porous materials and providing new opportunities for biological applications. In addition, porous metal-based nanomaterials have a wide range of development prospects in drug delivery systems due to their good loading rates and biocompatibility.
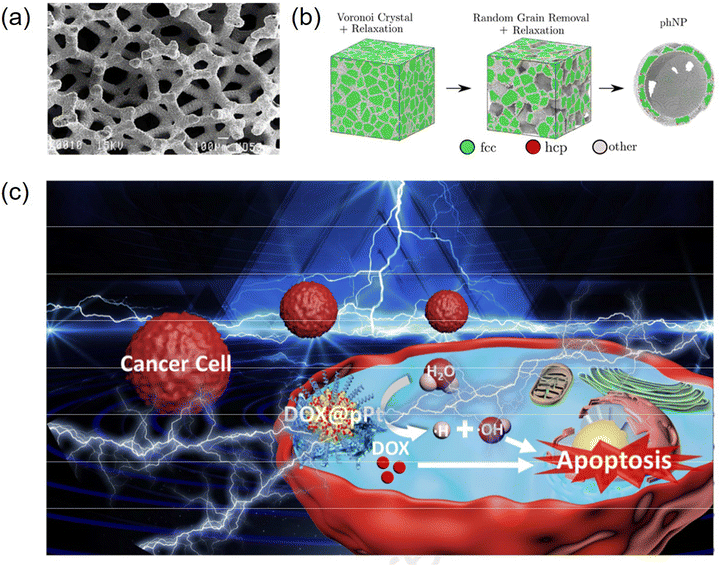 |
| Fig. 7 (a) Structure of porous tantalum. SEM of porous tantalum with small pores and high interconnectivity and porosity. (b) Schematic illustration of thermal stability of hollow porous gold nanoparticles: a molecular dynamics study. (c) Schematic illustration of pPt-based drug delivery system for synergistic electrodynamic/chemotherapy of cancer. Images reprinted with permission from Hacking et al. (2000, J. Biomed. Mater. Res.), Felipe et al. (2020, JCIM) and Xu et al. (2020, Biomaterials), respectively. | |
Noble metal nanoparticles possess catalytic properties for scavenging reactive oxygen species (ROS) and good biocompatibility, and thus can be used as promising drug delivery platforms for mitigating oxidative stress damage to normal tissues by certain chemotherapeutic drugs. Precious metal materials have inert reactivity in vivo and in vitro, are biocompatible and have low cytotoxicity.108 Chen et al. used platinum nanoparticles (pPt NPs) with porous structures as anticancer drug delivery carriers to achieve synergistic chemotherapy (Fig. 7c).109 Using polyethylene glycol (PEG) as a capping layer and the porous structure of pPt NPs, the obtained pPt-PEG NPs were loaded with the anticancer drug adriamycin (DOX), and this study proposed a new concept of cancer combination therapy with better therapeutic effects. Metal nanoparticles are designed and prepared as a multifunctional platform for the combined treatment of multiple cancers, especially chemophotothermal therapy, due to their excellent optical properties and photothermal conversion.110,111 Nanocarriers with porous structures have demonstrated potential and possibilities for drug delivery and photothermal therapy.112 Yang et al. constructed porous Au@Pt nanoparticles and explored their performance in mitigating oxidative stress damage and tumor growth inhibition by combined chemophotothermal therapy (Fig. 8a and b), showing that porous Au@Pt nanoparticles are a promising platform for photoacoustic imaging-guided DOX delivery and combined chemophotothermal therapy to reduce oxidative stress.113 He et al. found that porous heterogeneously assembled structures of Au–Ag nanoclusters (NCs) are good drug carriers with high loading performance and biocompatibility for cancer therapy, as shown in Fig. 8c, where the porous structures with high loading performance were formed by the assembly of different metal clusters.114 The composite structure of different metallic materials generates new chemical and physical functions that have a wide range of applications in the biological field.115 In general, porous metal-based structures have good biocompatibility and offer good prospects for biological applications. The Ahmed research team successfully developed an innovative nanomaterial known as the nanostructured mesoporous gold electrode (NMGE). This electrode exhibited exceptionally high sensitivity for the detection of protein phosphorylation through electrochemical signal amplification. By directly adsorbing the BRAF protein, which was purified through immunoprecipitation, on the mesoporous gold electrode, this team successfully bypassed the intricate steps of sensor surface functionalization. This not only streamlined the process but also resulted in a 2.5-fold improvement in sensitivity. Furthermore, there was a significant tenfold enhancement in the detection limit (LOD) compared to previous levels, marking a substantial advancement in this field.116 Masud and team designed an innovative electrode known as the mesoporous polystyrene-block-polyethylene oxide-gold electrode (MPGE), aimed at creating an ultra-sensitive miRNA detection platform. This electrode was crafted through the utilization of block copolymer micelles (polystyrene-block-polyethylene oxide; PS-b-PEO), resulting in a uniform porous structure and an augmented functional surface area. Consequently, this approach significantly enhanced the electrocatalytic activity and facilitated the efficient adsorption of a substantial quantity of miRNA.117 Park and colleagues employed an innovative technique, showcasing its application in microRNA sensors, through the electrochemical assembly of mesoporous gold (Au)–silver (Ag) alloy films. In an electrolyte solution containing spherical polymer micelles, mesoporous gold–silver alloy films with varying molar fractions were electrochemically prepared. Through the adjustment of the composition ratio of Au and Ag ions, precise control of the electrochemically active regions and catalytic activity of the mesoporous bimetallic films was achieved, resulting in outstanding sensitivity.118 In general, porous metal-based structures exhibit excellent biocompatibility, offering promising prospects for biological applications. These advancements underscore the potential of these nanomaterials in biomedical applications, paving the way for enhanced diagnostic and therapeutic strategies.
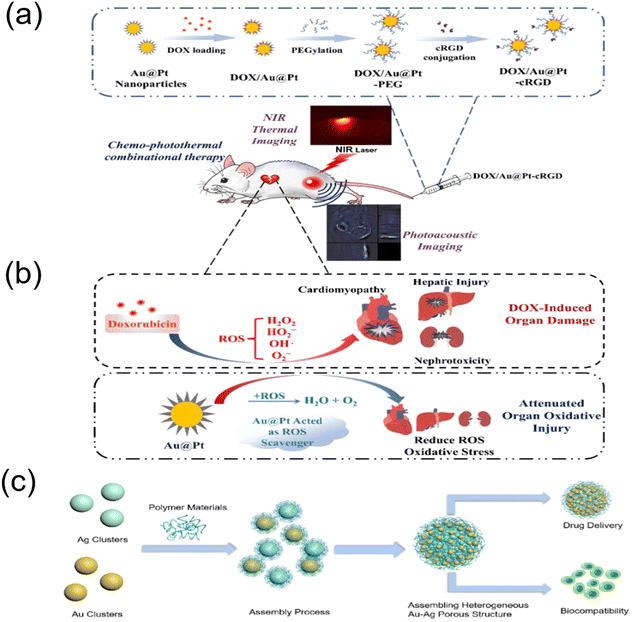 |
| Fig. 8 Schematic illustration of chemo-photothermal combinational therapy for tumors by multifunctional Au@Pt nanoparticles. (a) Construction of DOX/Au@Pt-cRGD and (b) ROS scavenging property by the catalysis of platinum shell enables the nanostructure to attenuate the DOX-induced organ oxidative injury, especially cardiomyopathy during chemotherapy. The gold core-based nanostructure maintains photoacoustic imaging and high photothermal conversion property, while the surface porous platinum shell not only provides the capability for drug loading but also alleviates the adverse effects of chemodrugs. (c) Schematic diagram of the formation and synthesis of heterogeneous assembly structures of precious metal NCs (Au, Ag). Images reprinted with permission from Yang et al. (2018, ACS) and He et al. (2022, IJMS) respectively. | |
5.2 Metal oxide porous structures
Porous metal oxides, including iron oxide, copper oxide, zinc oxide, titanium oxide, zirconium oxide, magnesium oxide, and aluminum oxide, have a wide range of biological and biosensing applications. In recent years, an increasing number of anti-cancer drugs have shown good efficacy in clinical treatment, such as cisplatin, adriamycin (DOX) and paclitaxel, which are widely used in tumor treatment.119–121 However, there are certain disadvantages associated with the use of these drugs, such as short blood half-lives due to rapid metabolism and elimination by the immune system and non-specific targeting, which can cause damage to normal tissues and cells.122 In this case, a promising approach to increase the circulation time of anticancer drugs and reduce side effects is the use of suitable drug carriers, which has led to great interest in nanoparticles as drug delivery systems.123 In addition, porous metal oxides are widely utilized in the field of biosensors because of their good biocompatibility, short response time, high sensitivity and low cost.
5.2.1 Porous structure of iron oxide.
Iron oxide porous nanoparticles (IOPNP) are promising for biological applications, with the main advantages of simple synthetic route, good biocompatibility, easy modification, maximum signal-active surface, and good dispersion in liquid media.124,125 Yu et al. used polyethylene glycol bisamine (NH2–PEG–NH2) as a spacer to encapsulate folic acid (FA) on the surface of iron oxide porous nanorods (IOPNR) to produce effective targeting agents.123 As shown in Fig. 9a, the results indicate that iron oxide porous nanorods loaded with DOX could reduce the cardiotoxicity of DOX compared to pure DOX. Wu et al. found that porous iron oxide-based nanorods coated with polyelectrolyte polymers126 formed nanocapsules, and depending on the denseness of the polyelectrolyte shell layer on the nanorod surface, the permeability of the synthesized nanocapsules could be varied by ionic strength and exhibit stimulus-responsive controlled behavior or slow-release behavior, which could be used as stimulus-responsive controlled carriers or slow-release carriers, respectively. In addition, Gong et al. reported a simple method for the preparation of novel HA cross-linked Fe3O4 nanoparticles (Fe3O4-DOX + HA) for DOX delivery (Fig. 9b). The nanoparticles combined the tumor-targeting function of chemotherapy with the tumor-associated macrophage (TAM)-targeting function of M1 polarization.127 Masud's group constructed gold-supported nanoporous iron oxide nanocubes (Au–NPFe2O3NC) for the detection of horseradish peroxidase (HRP)-like activity. This inherently autoantibody sensor exhibited excellent detection sensitivity (LOD = 0.08 U mL−1) and repeatability (% RSD = <5% for n = 3), and the readings of p53-specific autoantibodies could be analyzed using electrochemistry and colorimetry (naked-eye).128 Islam et al. developed an ultra-sensitive and specific electrochemical sensor using the electrocatalytic activity of a novel superparamagnetic nanoparticle graphene oxide-supported iron oxide (GO/IO hybrid material) and the signal enhancement capability of the [Ru(NH3)6]3+/[Fe(CN)6]3− electrocatalytic cycle. It was used to detect cancer-associated microRNAs (miRNAs). Clinical utility in ovarian cancer cell lines with high sensitivity (10 cells) and good reproducibility (% RSD = <5% for n = 3).129 Bhattacharjee et al. reported the synthesis and application of the peroxidase-mimetic activity of mesoporous iron oxide (MIO) for the detection of global DNA methylation in colorectal cancer cell lines. The target DNA was extracted and denatured to obtain ssDNA, followed by its direct adsorption on the surface of a bare screen-printed gold electrode (SPGE). Subsequently, a 5-methylcytosine antibody (5mC)-functionalized nanomaterial (MIO-5mC) was used to recognise the methylcytosine groups present on the SPGE.130 Boriachek et al. described a simple method for the direct isolation and subsequent detection of specific populations of exosomes using an engineered superparamagnetic material with multifunctional properties, namely, gold-loaded iron oxide nanocubes (Au–NPFe2O3NC). The PLAP-specific exosomes present in placental cell-conditioned media could be visually observed, as well as recognized by ultraviolet-visible and electrochemical detection. This highly sensitive, rapid, and inexpensive assay can be used to quantify specific groups of exosomes for a variety of clinical applications, especially pregnancy complications.131 The porous structure formed by iron oxide combined with different nanomaterials can be used more effectively as a drug carrier for cancer therapy with good biocompatibility and targeting.
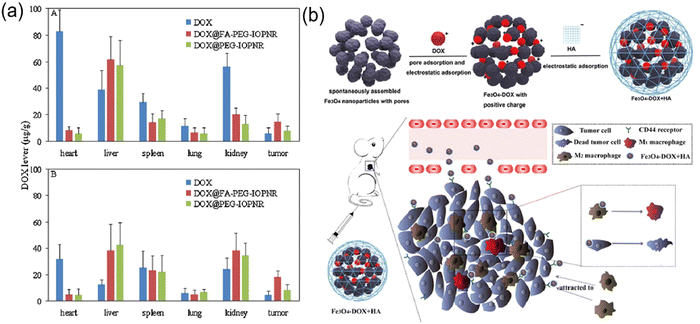 |
| Fig. 9 (a) Biodistribution of free DOX and DOX@modified IOPNR after (A) 4 h and (B) 12 h. (b) Schematic illustration of the preparation and antitumor mechanism. Images reprinted with permission from Yu et al. (2014, ELSEVIER) and Gong et al. (2019, ELSEVIER), respectively. | |
5.2.2 Porous copper oxides structures.
Copper oxide (CuO) nanoparticles have a controllable particle size of less than 5 nm.132,133 When self-assembled into nanoclusters, the porous structure leads to a large specific surface area with high Cu content, which increases the active reaction sites and improves the efficiency of Fenton-like reactions.134,135 As shown in Fig. 10a, CuO nanoclusters (CuO NCs) obtained after the addition of sodium dodecyl sulfate (SDS) surfactant were encapsulated by SiO2 shells and etched with NaOH solution. Subsequently, they were modified with folic acid (FA) to target cancer cells, and the obtained CuO NCs-FA were delivered to HeLa cells to verify the feasibility of intracellular Fenton-like reactions.136 In addition, polydopamine-modified CuO combined with natural glucose oxidase (GOD), in which CuO maximizes the cascade catalytic efficiency with a high loading rate (47.1%) due to the elaborate hollow mesoporous structure (Fig. 10b), was combined with NIR-II-induced thermal effects for combined O2-promoted starvation and photothermal-chemical kinetic treatment.137 In addition, copper oxide (Cu2O), as a low-toxic, environmentally friendly p-type semiconductor, has received widespread attention for its application in the fields of photocatalysis, catalysts, adsorption, sensors, etc.138–140
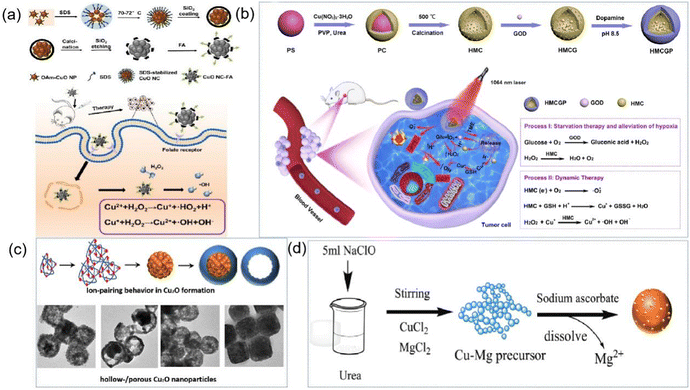 |
| Fig. 10 (a) Schematic illustration for the synthesis of CuO NCs and CuO NCs-FA, cellular uptake of CuO NCs-FA and intracellular Fenton-like reaction. (b) Schematic illustration of the synthesis of HMCGP and the theranostic mechanism of HMCGP for starvation therapy-promoted alleviation of tumor hypoxia and combined photothermal-catalytic therapy by a cascade enzymatic reaction. (c) Schematic illustration of the synthesis of hollow Cu2O nanostructure. (d) Synthetic route of Cu2O NPs. Images reprinted with permission from Jin et al. (2022, ACES), Wang et al. (2022, ACS Appl.), Song et al. (2020, ACS) and Zhu et al. (2021, J. Mater. Chem. B), respectively. | |
Among the developed nanostructures, porous structures are highly desirable because of their high specific surface area, abundant active sites and surface accessibility. Thus far, several synthetic strategies have been successfully developed to prepare porous Cu2O crystals, including templating, surfactant-assisted synthesis, electrochemical deposition, and dealloying.141 For example, as shown in Fig. 10c, Song et al. synthesized porous Cu2O nanostructures by adjusting the concentration of PVP and the growth solution environment.142 Yu et al. synthesized carbon layer-coated Cu2O mesoporous nanorods via a facile method of chemical oxidation and subsequent carbonization at 500 °C under N2.143 However, these methods are relatively complex, and thus Zhu et al. developed a rapid, simple and economical method for the synthesis of porous nanomaterials by oxidizing urea to CO2 as a carbon source using sodium hypochlorite as a green oxidant to prepare fine-grained cross-linked Cu precursors (Fig. 10d). These precursors were further reduced by sodium ascorbate to pure Cu2O nanospheres (NPs) with a porous morphology at room temperature and found to exhibit high sensitivity and excellent selectivity in the determination of glucose and L-cysteine, making them suitable for the detection of serum samples with reliable results.144
5.2.3 Porous structures of titanium oxide.
The ideal anticancer drug delivery system should possess the combination of cancer cell-targeting and stimulator-triggered drug release. Porous titanium dioxide (TiO2) has the potential to satisfy this requirement because of its interesting properties, such as high photocatalytic activity and functional surface.145 Titanium dioxide is a typical n-type semiconductor material with a wide range of applications including photocatalysis,146 energy storage and conversion,147 sun protection148 and sensor research.149 Especially in the medical field, it is used as a matrix for anti-cancer agents, implants and stem cell expansion due to its low cost, chemical stability,150 non-toxicity151 and good biocompatibility.152
Wang et al. developed novel polyethyleneimine (PEI)-modified multifunctional porous titanium dioxide nanoparticles.153 To further achieve targeted delivery, folic acid, as a tumor-targeting agent that effectively promotes specific uptake by tumor cells through receptor-mediated endocytosis, was chemically coupled to the surface of the functionalized porous titanium dioxide nanoparticles by the free amine groups of PEI. Malignancies remain a major health burden worldwide and there is an urgent need for effective treatment strategies. Herein, Yin et al. reported the synthesis of upconversion nanoparticles with a mesoporous titanium dioxide (mTiO2) shell layer for near-infrared (NIR)-triggered drug delivery and synergistic targeting of cancer therapy,154 which provides another drug-carrying function for anticancer drugs due to the large specific surface area and porous structure of titanium dioxide. The surface-modified hyaluronic acid not only ensures controlled release of the drug, but also endows the system with the ability to target cancer cells. TiO2 has good biocompatibility, relatively high electrical conductivity, environmentally friendly properties, and chemical and thermal stability, and is also widely used in protein and immobilized biosensors.155 The electrochemical properties of titanium dioxide depend not only on its crystal structure but also on its lattice properties (e.g., size, geometry, porosity, and surface area).156 Thus, to develop high-performance biosensors, efforts have been made to synthesize titanium dioxide with a controllable morphology and pore structure. Guo et al. successfully prepared a freestanding titanium dioxide hollow fiber via a simple, scalable electrospun nanofiber membrane-template-assisted sol–gel method and applied it for the immobilization of glucose oxidase (GOD) and biosensing.157
5.2.4 Other metal oxides.
Other metal oxides (alumina, magnesium oxide and zinc oxide) have shown some promise in biological applications and biosensing. Zinc oxide (ZnO) is one of the most representative chemically resistant n-type oxide semiconductor materials. Kim et al. prepared porous nanorods and two hierarchical structures of ZnO nanostructures consisting of porous nanosheets or crystalline nanorods by reacting oleic acid dissolved ethanol solution and a water-soluble zinc precursor solution in the presence of sodium hydroxide.158Fig. 11a shows the pore size, volume distribution and specific surface area of the three ZnO nanostructures, all enabling the sensitive and selective detection of C2H5OH. Nanoporous anodic aluminum oxide (NAA) is suitable for optical biosensing because of its transparency in the visible range, photoluminescence159 and high and tunable surface area/volume ratio. In addition, when produced under appropriate anodic oxidation conditions, its self-ordered structure gives it a photonic resistance band similar to that of photonic crystals. Recently, nano-engineered NAA has also been applied in label-free biosensing applications.160 Magnesium oxide (MgO) nanoparticles, as important metal oxide nanoparticles, have received significant attention for biomedical applications due to their good biocompatibility and biodegradability.161 In addition to their significant bactericidal and tumor suppressive effects,162,163 MgO nanoparticles have been used as potential drug candidates,164 magnetic resonance imaging agents and thermal therapy systems.162 Considering the different sensitivities of cancer cells and normal cells to lysosomes, Qu et al. designed pH-sensitive, biodegradable and imaging-ready porous magnesium oxide nanoparticles (MgO/Eu) that can support Eu atoms,165 as shown in Fig. 11b, which were highly sensitive to H+-rich environments, especially in the lysosomes of cancer cells.
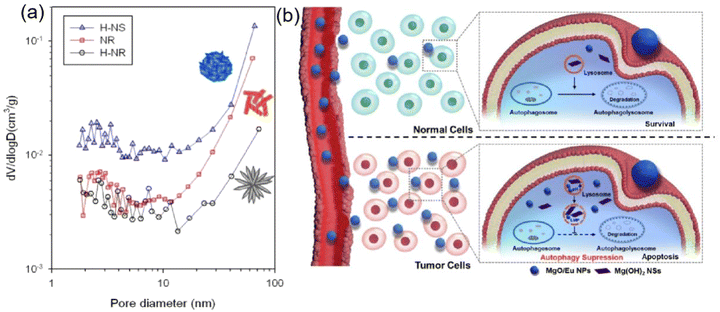 |
| Fig. 11 (a) Pore size distribution of heat-treated H-NS, NR, and H-NR ZnO nanostructures determined from nitrogen adsorption–desorption isotherms. (b) Illustration of the role of MgO/Eu NPs as a selective lysosome-autophagy system inhibitor. Images reprinted with permission from Kim et al. (2011, Sensors) and Qu et al. (2021, ELSEVIER), respectively. | |
6. Conclusions
In conclusion, porous nanomaterials demonstrate extensive prospects in the fields of biosensing and in vivo/in vitro applications. To date, substantial progress has been achieved in the design and synthesis of porous nanomaterials, involving diverse preparation methods combined with various ligands and materials to attain functional porous structures. However, it is still a challenge to effectively utilize the design of these porous nanomaterials. Through the modification of functional groups in porous nanomaterials, a high loading rate, elevated specific surface area, and excellent biocompatibility have been achieved, enhancing their capabilities in cancer treatment and biosensing applications. These materials hold potential to overcome current obstacles in cancer immunotherapy, thereby improving the anticancer efficacy. Their unique porous structure enables the loading of a substantial amount of immunotherapeutically relevant biomolecules for targeted delivery, modulation of the tumor microenvironment, and regulation of immune cell functions. Their high porosity allows efficient drug loading, guiding them to tumor cells through binding to the targeted fraction on their surface, and ultimately, binding to the cleavable shell. The distinctive structure of porous nanomaterials facilitates highly targeted drug delivery, laying the foundation for personalized cancer treatment. The adjustment of the size and shape of porous structures enables precise treatment of different types of tumors, minimizing damage to healthy tissues. Beyond their application in cancer treatment, the porous structure of nanomaterials can also be employed to regulate immune responses, holding potential value in treating autoimmune diseases or enhancing vaccine effectiveness. These porous nanomaterials exhibit promising results in both in vitro and in vivo models. The key to their successful application is their biocompatibility, and further in-depth research is needed to explore the response of these materials in different biological systems, ensuring their long-term safety and stability. Additionally, porous nanomaterials have attracted attention in the field of biosensing due to their large specific surface area, high porosity, abundant functional groups, and ease of modification. Coupled with their excellent conductivity, these materials facilitate charge transfer and substance exchange between electrodes and solutions. Biosensors based on porous materials excel in detecting heavy metal ions, small molecules, proteins, and nucleic acids. In summary, porous nanomaterials hold immense potential not only for in vivo/in vitro applications and biosensing but also for substance detection in various fields. The interdisciplinary nature of porous nanomaterial research, spanning materials science, biology, chemistry, and more, encourages collaboration and cross-disciplinary research, promising to accelerate the development and innovation in the application of porous nanomaterials. Thus, in the future, through different ligand modifications and the assembly of diverse materials, the application of porous nanomaterials is anticipated to achieve significant breakthroughs.
Conflicts of interest
The authors declare no competing financial interest.
Acknowledgements
This work was supported by the Chongqing Municipal Natural Science Foundation: CSTB2022NSCQ-MSX0113; Scientific Foundation Project of Chongqing Education Commission: KJQN201900436; National Natural Science Foundation of China: 81127901, 12074051.
References
- Z. Luo, S. Fan, C. Gu, W. Liu, J. Chen, B. Li and J. Liu, Metal–Organic Framework (MOF)-based Nanomaterials for Biomedical Applications, Curr. Med. Chem., 2019, 26, 3341–3369 CrossRef CAS PubMed.
- D. Karaman, M. P. Sarparanta, J. M. Rosenholm and A. J. Airaksinen, Multimodality Imaging of Silica and Silicon Materials In Vivo, Adv. Mater., 2018, 30, e1703651 CrossRef PubMed.
- H. A. Santos, E. Mäkilä, A. J. Airaksinen, L. M. Bimbo and J. Hirvonen, Porous silicon nanoparticles for nanomedicine: preparation and biomedical applications, Nanomedicine, 2014, 9, 535–554 CrossRef CAS PubMed.
- J. Zhou, Y. Li, W. Wang, X. Tan, Z. Lu and H. Han, Metal–organic frameworks-based sensitive electrochemiluminescence biosensing, Biosens. Bioelectron., 2020, 164, 112332 CrossRef CAS PubMed.
- P. Kumar, K.-H. Kim, K. Vellingiri, P. Samaddar, P. Kumar, A. Deep and N. Kumar, Hybrid porous thin films: Opportunities and challenges for sensing applications, Biosens. Bioelectron., 2018, 104, 120–137 CrossRef CAS PubMed.
- J. Ferre-Borrull, J. Pallares, G. Macias and L. F. Marsal, Nanostructural Engineering of Nanoporous Anodic Alumina for Biosensing Applications, Materials, 2014, 7, 5225–5253 CrossRef PubMed.
- V. Stojanovic, F. Cunin, J. O. Durand, M. Garcia and M. Gary-Bobo, Potential of porous silicon nanoparticles as an emerging platform for cancer theranostics, J. Mater. Chem. B, 2016, 4, 7050–7059 RSC.
- Y. Park, J. Yoo, M. H. Kang, W. Kwon and J. Joo, Photoluminescent and biodegradable porous silicon nanoparticles for biomedical imaging, J. Mater. Chem. B, 2019, 7, 6271–6292 RSC.
- G. Hong, A. L. Antaris and H. Dai, Near-infrared fluorophores for biomedical imaging, Nat. Biomed. Eng., 2017, 1, 0010–0031 CrossRef CAS.
- H. C. Zhou, J. R. Long and O. M. Yaghi, Introduction to metal–organic frameworks, Chem. Rev., 2012, 112, 673–674 CrossRef CAS PubMed.
- A. S. Cheung and D. J. Mooney, Engineered Materials for Cancer Immunotherapy, Nano Today, 2015, 10, 511–531 CrossRef CAS PubMed.
- C. T. t Hagan, Y. B. Medik and A. Z. Wang, Nanotechnology Approaches to Improving Cancer Immunotherapy, Adv. Cancer Res., 2018, 139, 35–56 CrossRef PubMed.
- G. Chong, J. Zang, Y. Han, R. Su, N. Weeranoppanant, H. Dong and Y. Li, Bioengineering of nano metal–organic frameworks for cancer immunotherapy, Nano Res., 2021, 14, 1244–1259 CrossRef CAS PubMed.
- F. Boateng and W. Ngwa, Delivery of Nanoparticle-Based Radiosensitizers for Radiotherapy Applications, Int. J. Mol. Sci., 2020, 21, 273–294 CrossRef CAS PubMed.
- N. Thakur, S. Thakur, S. Chatterjee, J. Das and P. C. Sil, Nanoparticles as Smart Carriers for Enhanced Cancer Immunotherapy, Front. Chem., 2020, 8, 597806 CrossRef CAS PubMed.
- C. Xu, C. Lei and C. Yu, Mesoporous Silica Nanoparticles for Protein Protection and Delivery, Front. Chem., 2019, 7, 290 CrossRef CAS PubMed.
- C. Xu, C. Lei, Y. Wang and C. Yu, Dendritic Mesoporous Nanoparticles: Structure, Synthesis and Properties, Angew. Chem., Int. Ed., 2022, 61, e202112752 CrossRef CAS PubMed.
- I. Mikelez-Alonso, A. Aires and A. L. Cortajarena, Cancer Nano-Immunotherapy from the Injection to the Target: The Role of Protein Corona, Int. J. Mol. Sci., 2020, 21, 519–535 CrossRef CAS PubMed.
- K. Lu, T. Aung, N. Guo, R. Weichselbaum and W. Lin, Nanoscale Metal–Organic Frameworks for Therapeutic,
Imaging, and Sensing Applications, Adv. Mater., 2018, 30, e1707634 CrossRef PubMed.
- A. Cao, Z. Ye, Y. Yang, Y. Liu, H. Wang and Y. Liu, Fluorescent protein-based multi-functional nanoparticles for bioimaging and drug delivery, Nanomedicine, 2016, 12, 455 CrossRef.
- J. Joo, X. Liu, V. R. Kotamraju, E. Ruoslahti, Y. Nam and M. J. Sailor, Gated Luminescence Imaging of Silicon Nanoparticles, ACS Nano, 2015, 9, 6233–6241 CrossRef CAS PubMed.
- C. Martinez-Boubeta, L. Balcells, R. Cristòfol, C. Sanfeliu, E. Rodríguez, R. Weissleder, S. Lope-Piedrafita, K. Simeonidis, M. Angelakeris, F. Sandiumenge, A. Calleja, L. Casas, C. Monty and B. Martínez, Self-assembled multifunctional Fe/MgO nanospheres for magnetic resonance imaging and hyperthermia, Nanomedicine, 2010, 6, 362–370 CrossRef CAS PubMed.
- C. A. Marquette and L. J. Blum, State of the art and recent advances in immunoanalytical systems, Biosens. Bioelectron., 2006, 21, 1424–1433 CrossRef CAS PubMed.
- S. Dhanekar and S. Jain, Porous silicon biosensor: Current status, Biosens. Bioelectron., 2013, 41, 54–64 CrossRef CAS PubMed.
- A. D. Kartashova, K. A. Gonchar, D. A. Chermoshentsev, E. A. Alekseeva, M. B. Gongalsky, I. V. Bozhev, A. A. Eliseev, S. A. Dyakov, J. V. Samsonova and L. A. Osminkina, Surface-Enhanced Raman Scattering-Active Gold-Decorated Silicon Nanowire Substrates for Label-Free Detection of Bilirubin, ACS Biomater. Sci. Eng., 2022, 8, 4175–4184 CrossRef CAS PubMed.
- K. Tian, Y. S. Ma, Y. K. Liu, M. H. Wang and M. Du, Hierarchically structured hollow bimetallic ZnNi MOF microspheres as a sensing platform for adenosine detection, Sens. Actuators, B, 2019, 303, 127199 CrossRef.
-
D. F. Thomas, Porous silicon, Handbook of Nanostructured Materials and Nanotechnology, 2000 Search PubMed.
- L. M. Bimbo, M. Sarparanta, H. A. Santos, A. J. Airaksinen, E. Makila, T. Laaksonen, L. Peltonen, V. P. Lehto, J. Hirvonen and J. Salonen, Biocompatibility of thermally hydrocarbonized porous silicon nanoparticles and their biodistribution in rats, ACS Nano, 2010, 4, 3023–3032 CrossRef CAS PubMed.
- D. X. Zhang, L. Esser, R. B. Vasani, H. Thissen and N. H. Voelcker, Porous silicon nanomaterials: recent advances in surface engineering for controlled drug-delivery applications, Nanomedicine, 2019, 14, 3213–3230 CrossRef CAS PubMed.
- H. A. Santos, E. Makila, A. J. Airaksinen, L. M. Bimbo and J. Hirvonen, Porous silicon nanoparticles for nanomedicine: preparation and biomedical applications, Nanomedicine, 2014, 9, 535–554 CrossRef CAS PubMed.
- J. Salonen, L. Laitinen, A. M. Kaukonen, J. Tuura, M. Bjorkqvist, T. Heikkila, K. Vaha-Heikkila, J. Hirvonen and V. P. Lehto, Mesoporous silicon microparticles for oral drug delivery: loading and release of five model drugs, J. Controlled Release, 2005, 108, 362–374 CrossRef CAS PubMed.
- E. Blanco, A. Hsiao, G. U. Ruiz-Esparza, M. G. Landry, F. Meric-Bernstam and M. Ferrari, Molecular-targeted nanotherapies in cancer: enabling treatment specificity, Mol. Oncol., 2011, 5, 492–503 CrossRef CAS PubMed.
- P. A. Kulyavtsev and R. P. Spencer, Drug delivery via porous silicon: a focused patent review, Pharm. Pat. Anal., 2017, 6, 77–85 CrossRef CAS PubMed.
- M. Jeong, Y. Jung, J. Yoon, J. Kang, S. H. Lee, W. Back, H. Kim, M. J. Sailor, D. Kim and J. H. Park, Porous Silicon-Based Nanomedicine for Simultaneous
Management of Joint Inflammation and Bone Erosion in Rheumatoid Arthritis, ACS Nano, 2022, 16, 16118–16132 CrossRef CAS PubMed.
- K. Trzeciak, A. Chotera-Ouda, S. Bak, II and M. J. Potrzebowski, Mesoporous Silica Particles as Drug Delivery Systems-The State of the Art in Loading Methods and the Recent Progress in Analytical Techniques for Monitoring These Processes, Pharmaceutics, 2021, 13, 14230–14240 CrossRef PubMed.
- M. Zhang, J.-J. Ye, Y. Xia, Z.-Y. Wang, C.-X. Li, X.-S. Wang, W. Yu, W. Song, J. Feng and X.-Z. Zhang, Platelet-Mimicking Biotaxis Targeting Vasculature-Disrupted Tumors for Cascade Amplification of Hypoxia-Sensitive Therapy, ACS Nano, 2019, 13, 14230–14240 CrossRef CAS PubMed.
-
Rita Elena Serda, US8926994, 2015.
-
Michael J. Sailor, US9394369, 2016.
- B. Kim, Q. Yang, L. W. Chan, S. N. Bhatia, E. Ruoslahti and M. J. Sailor, Fusogenic porous silicon nanoparticles as a broad-spectrum immunotherapy against bacterial infections, Nanoscale Horiz., 2021, 6, 330–340 RSC.
- J. Pellico, P. J. Gawne and R. T. M. de Rosales, Radiolabelling of nanomaterials for medical imaging and therapy, Chem. Soc. Rev., 2021, 50, 3355–3423 RSC.
- C. Yao, P. Wang, X. Li, X. Hu, J. Hou, L. Wang and F. Zhang, Near-Infrared-Triggered Azobenzene-Liposome/Upconversion Nanoparticle Hybrid Vesicles for Remotely Controlled Drug Delivery to Overcome Cancer Multidrug Resistance, Adv. Mater., 2016, 28, 9341–9348 CrossRef CAS PubMed.
- T. van der Geest, P. Laverman, J. M. Metselaar, G. Storm and O. C. Boerman, Radionuclide imaging of liposomal drug delivery, Expert Opin. Drug Delivery, 2016, 13, 1231–1242 CrossRef CAS PubMed.
- T. Nakamura, F. Sugihara, H. Matsushita, Y. Yoshioka, S. Mizukami and K. Kikuchi, Mesoporous silica nanoparticles for (19)F magnetic resonance imaging, fluorescence imaging, and drug delivery, Chem. Sci., 2015, 6, 1986–1990 RSC.
- J. H. Park, L. Gu, G. von Maltzahn, E. Ruoslahti, S. N. Bhatia and M. J. Sailor, Biodegradable luminescent porous silicon nanoparticles for in vivo applications, Nat. Mater., 2009, 8, 331–336 CrossRef CAS PubMed.
- O. Syshchyk, V. A. Skryshevsky, O. O. Soldatkin and A. P. Soldatkin, Enzyme biosensor systems based on porous silicon photoluminescence for detection of glucose, urea and heavy metals, Biosens. Bioelectron., 2015, 66, 89–94 CrossRef CAS PubMed.
- S. Zhang, M. Sun, X. Wang, J. Wang, Z. Jia, X. Lv and X. Huang, Spectral-Free Double Light Detection of DNA Based on a Porous Silicon Bragg Mirror, Sensors, 2021, 11, 27–41 Search PubMed.
- R. Vercauteren, A. Leprince, J. Mahillon and L. A. Francis, Porous Silicon Biosensor for the Detection of Bacteria through Their Lysate, Biosensors, 2021, 21, 8248–8257 Search PubMed.
- R. Layouni, T. Cao, M. B. Coppock, P. E. Laibinis and S. M. Weiss, Peptide-Based Capture of Chikungunya Virus E2 Protein Using Porous Silicon Biosensor, Nature, 2021, 21, 8248 CAS.
- O. M. Yaghi, G. Li and H. Li, Selective binding and removal of guests in a microporous metal–organic framework, Nature, 1995, 378, 703–706 CrossRef CAS.
- W. P. Lustig, S. Mukherjee, N. D. Rudd, A. V. Desai, J. Li and S. K. Ghosh, Metal–organic frameworks: functional luminescent and photonic materials for sensing applications, Chem. Soc. Rev., 2017, 46, 3242–3285 RSC.
- P. Horcajada, C. Serre, M. Vallet-Regí, M. Sebban, F. Taulelle and G. Férey, Metal–organic frameworks as efficient materials for drug delivery, Angew. Chem., Int. Ed., 2006, 45, 5974–5978 CrossRef CAS PubMed.
- J. W. Zhang, H. T. Zhang, Z. Y. Du, X. Wang, S. H. Yu and H. L. Jiang, Water-stable metal–organic frameworks with intrinsic peroxidase-like catalytic activity as a colorimetric biosensing platform, Chem. Commun., 2014, 50, 1092–1094 RSC.
- L. Ai, L. Li, C. Zhang, J. Fu and J. Jiang, MIL-53(Fe): a metal–organic framework with intrinsic peroxidase-like catalytic activity for colorimetric biosensing, Chemistry, 2013, 19, 15105–15108 CrossRef CAS PubMed.
- G. H. Qiu, W. Z. Lu, P. P. Hu, Z. H. Jiang, L. P. Bai, T. R. Wang, M. M. Li and J. X. Chen, A metal–organic framework based PCR-free biosensor for the detection of gastric cancer associated microRNAs, J. Inorg. Biochem., 2017, 177, 138–142 CrossRef CAS PubMed.
- P. Horcajada, R. Gref, T. Baati, P. K. Allan, G. Maurin, P. Couvreur, G. Férey, R. E. Morris and C. Serre, Metal–organic frameworks in biomedicine, Chem. Rev., 2012, 112, 1232–1268 CrossRef CAS PubMed.
- C. He, K. Lu and W. Lin, Nanoscale metal–organic frameworks for real-time intracellular pH sensing in live cells, J. Am. Chem. Soc., 2014, 136, 12253–12256 CrossRef CAS PubMed.
- R. Xu, Y. Wang, X. Duan, K. Lu, D. Micheroni, A. Hu and W. Lin, Nanoscale Metal–Organic Frameworks for Ratiometric Oxygen Sensing in Live Cells, J. Am. Chem. Soc., 2016, 138, 2158–2161 CrossRef CAS PubMed.
- Y. Lu, B. Yan and J. L. Liu, Nanoscale metal–organic frameworks as highly sensitive luminescent sensors for Fe2+ in aqueous solution and living cells, Chem. Commun., 2014, 50, 9969–9972 RSC.
- J. Zhao, Y. N. Wang, W. W. Dong, Y. P. Wu, D. S. Li and Q. C. Zhang, A Robust Luminescent Tb(III)-MOF with Lewis Basic Pyridyl Sites for the Highly Sensitive Detection of Metal Ions and Small Molecules, Inorg. Chem., 2016, 55, 3265–3271 CrossRef CAS PubMed.
- W. Ma, Q. Jiang, P. Yu, L. Yang and L. Mao, Zeolitic imidazolate framework-based electrochemical biosensor for in vivo electrochemical measurements, Anal. Chem., 2013, 85, 7550–7557 CrossRef CAS PubMed.
- Y. Cui, H. Xu, Y. Yue, Z. Guo, J. Yu, Z. Chen, J. Gao, Y. Yang, G. Qian and B. Chen, A luminescent mixed-lanthanide metal–organic framework thermometer, J. Am. Chem. Soc., 2012, 134, 3979–3982 CrossRef CAS PubMed.
- D. Zhao, D. Yue, K. Jiang, L. Zhang, C. Li and G. Qian, Isostructural Tb(3+)/Eu(3+) Co-Doped Metal–Organic Framework Based on Pyridine-Containing Dicarboxylate Ligands for Ratiometric Luminescence Temperature Sensing, Inorg. Chem., 2019, 58, 2637–2644 CrossRef CAS PubMed.
- Q. Hu, N. Kong, Y. Chai, Z. Xing, Y. Wu, J. Zhang, F. Li and X. Zhu, A lanthanide nanocomposite with cross-relaxation enhanced near-infrared emissions as a ratiometric nanothermometer, Nanoscale Horiz., 2022, 7, 1177–1185 RSC.
- S. Keskin and S. Kızılel, Biomedical Applications of Metal Organic Frameworks, Ind. Eng. Chem. Res., 2011, 50, 1799–1812 CrossRef CAS.
- D. Yang, G. Yang, S. Gai, F. He, G. An, Y. Dai, R. Lv and P. Yang, Au25 cluster functionalized metal–organic nanostructures for magnetically targeted photodynamic/photothermal therapy triggered by single wavelength 808 nm near-infrared light, Nanoscale, 2015, 7, 19568–19578 RSC.
- W. Wang, L. Wang, Z. Li and Z. Xie, BODIPY-containing nanoscale metal–organic frameworks for photodynamic therapy, Chem. Commun., 2016, 52, 5402–5405 RSC.
- C. He, K. Lu, D. Liu and W. Lin, Nanoscale metal–organic frameworks for the co-delivery of cisplatin and pooled siRNAs to enhance therapeutic efficacy in drug-resistant ovarian cancer cells, J. Am. Chem. Soc., 2014, 136, 5181–5184 CrossRef CAS PubMed.
- A. R. Chowdhuri, D. Laha, S. Pal, P. Karmakar and S. K. Sahu, One-pot synthesis of folic acid encapsulated upconversion nanoscale metal organic frameworks for targeting, imaging and pH responsive drug release, Dalton Trans., 2016, 45, 18120–18132 RSC.
- Q. Xiao, Y. Liu, Y. Zhong and W. Zhu, A citrate sol–gel method to synthesize Li2ZrO3 nanocrystals with improved CO2 capture properties, J. Mater. Chem., 2011, 21, 3838–3842 RSC.
- Y. A. Li, X. D. Zhao, H. P. Yin, G. J. Chen, S. Yang and Y. B. Dong, A drug-loaded nanoscale metal–organic framework with a tumor targeting agent for highly effective hepatoma therapy, Chem. Commun., 2016, 52, 14113–14116 RSC.
- M. Tavakolizadeh, M. Atarod, S. J. Seyyed Tabaei, S. Sojdeh, E. Nazarzadeh Zare, M. Rabiee and N. Rabiee, Green modified-UiO-66/MXene sandwich composites for gene-chemotherapy synergistic cancer suppression: Co-delivery of doxorubicin and pCRISPR, Alexandria Eng. J., 2023, 80, 144–154 CrossRef.
- K. M. Au, A. Satterlee, Y. Min, X. Tian, Y. S. Kim, J. M. Caster, L. Zhang, T. Zhang, L. Huang and A. Z. Wang, Folate-targeted pH-responsive calcium zoledronate nanoscale metal–organic frameworks: Turning a bone antiresorptive agent into an anticancer therapeutic, Biomaterials, 2016, 82, 178–193 CrossRef CAS PubMed.
- W. Lin, Q. Hu, J. Yu, K. Jiang, Y. Yang, S. Xiang, Y. Cui, Y. Yang, Z. Wang and G. Qian, Low Cytotoxic Metal–Organic Frameworks as Temperature-Responsive Drug Carriers, ChemPlusChem, 2016, 81, 804–810 CrossRef CAS PubMed.
- J. Della Rocca, D. Liu and W. Lin, Nanoscale Metal–Organic Frameworks for Biomedical Imaging and Drug Delivery, Acc. Chem. Res., 2011, 44, 957–968 CrossRef CAS PubMed.
- W. Hatakeyama, T. J. Sanchez, M. D. Rowe, N. J. Serkova, M. W. Liberatore and S. G. Boyes, Synthesis of gadolinium nanoscale metal–organic framework with hydrotropes: manipulation of particle size and magnetic resonance imaging capability, ACS Appl. Mater. Interfaces, 2011, 3, 1502–1510 CrossRef CAS PubMed.
- K. M. Taylor-Pashow, J. Della Rocca, Z. Xie, S. Tran and W. Lin, Postsynthetic modifications of iron-carboxylate nanoscale metal–organic frameworks for imaging and drug delivery, J. Am. Chem. Soc., 2009, 131, 14261–14263 CrossRef CAS PubMed.
- T. Zhang, L. Wang, C. Ma, W. Wang, J. Ding, S. Liu, X. Zhang and Z. Xie, BODIPY-containing nanoscale metal–organic frameworks as contrast agents for computed tomography, J. Mater. Chem. B, 2017, 5, 2330–2336 RSC.
- J. Wu, F. Xu, S. Li, P. Ma, X. Zhang, Q. Liu, R. Fu and D. Wu, Porous Polymers as Multifunctional Material Platforms toward Task-Specific Applications, Adv. Mater., 2019, 31, e1802922 CrossRef PubMed.
- P. Stroeve and N. Ileri, Biotechnical and other applications of nanoporous membranes, Trends Biotechnol., 2011, 29, 259–266 CrossRef CAS PubMed.
- P. Guo, J. Huang, Y. Zhao, C. R. Martin, R. N. Zare and M. A. Moses, Nanomaterial Preparation by Extrusion through Nanoporous Membranes, Small, 2018, 14, e1703493 CrossRef PubMed.
- A. Shiohara, B. Prieto-Simon and N. H. Voelcker, Porous polymeric membranes: fabrication techniques and biomedical applications, J. Mater. Chem. B, 2021, 9, 2129–2154 RSC.
- D. Wu, F. Xu, B. Sun, R. Fu, H. He and K. Matyjaszewski, Design and preparation of porous polymers, Chem. Rev., 2012, 112, 3959–4015 CrossRef CAS PubMed.
- N. Oku, Innovations in Liposomal DDS Technology and Its Application for the Treatment of Various Diseases, Biol. Pharm. Bull., 2017, 40, 119–127 CrossRef CAS PubMed.
- S. Y. Yang, J.-A. Yang, E.-S. Kim, G. Jeon, E. J. Oh, K. Y. Choi, S. K. Hahn and J. K. Kim, Single-file diffusion of protein drugs through cylindrical nanochannels, ACS Nano, 2010, 4, 3817–3822 CrossRef CAS PubMed.
- G. I. Benic and C. H. F. Hämmerle, Horizontal bone augmentation by means of guided bone regeneration, Periodontology 2000, 2014, 66, 13–40 CrossRef PubMed.
- D. Rothamel, F. Schwarz, M. Sager, M. Herten, A. Sculean and J. Becker, Biodegradation of differently cross-linked collagen membranes: an experimental study in the rat, Clin. Oral Implants Res., 2005, 16, 369–378 CrossRef PubMed.
- A. Klinger, R. Asad, L. Shapira and Y. Zubery,
In vivo degradation of collagen barrier membranes exposed to the oral cavity, Clin. Oral Implants Res., 2010, 21, 873–876 CrossRef PubMed.
- L. M. Delgado, Y. Bayon, A. Pandit and D. I. Zeugolis, To cross-link or not to cross-link? Cross-linking associated foreign body response of collagen-based devices, Tissue Eng., Part B, 2015, 21, 298–313 CrossRef CAS PubMed.
-
S. M. Reddy, Materials for Chemical Sensing, 2017, ch. 4, pp. 75–103 DOI:10.1007/978-3-319-47835-7_4.
- L. Movileanu, Watching single proteins using engineered nanopores, Protein Pept. Lett., 2014, 21, 235–246 CrossRef CAS PubMed.
- J. Mota, N. Yu, S. G. Caridade, G. M. Luz, M. E. Gomes, R. L. Reis, J. A. Jansen, X. F. Walboomers and J. F. Mano, Chitosan/bioactive glass nanoparticle composite membranes for periodontal regeneration, Acta Biomater., 2012, 8, 4173–4180 CrossRef CAS PubMed.
- M. Herten, R. E. Jung, D. Ferrari, D. Rothamel, V. Golubovic, A. Molenberg, C. H. F. Hämmerle, J. Becker and F. Schwarz, Biodegradation of different synthetic hydrogels made of polyethylene glycol hydrogel/RGD-peptide modifications: an immunohistochemical study in rats, Clin. Oral Implants Res., 2009, 20, 116–125 CrossRef PubMed.
- S. H. Park, D. S. Park, J. W. Shin, Y. G. Kang, H. K. Kim, T. R. Yoon and J.-W. Shin, Scaffolds for bone tissue engineering fabricated from two different materials by the rapid prototyping technique: PCL versus PLGA, J. Mater. Sci.: Mater. Med., 2012, 23, 2671–2678 CrossRef CAS PubMed.
- A. de la Escosura-Muñiz and A. Merkoçi, A nanochannel/nanoparticle-based filtering and sensing platform for direct detection of a cancer biomarker in blood, Small, 2011, 7, 675–682 CrossRef PubMed.
- A. de la Escosura-Muñiz and A. Mekoçi, Nanoparticle based enhancement of electrochemical DNA hybridization signal using nanoporous electrodes, Chem. Commun., 2010, 46, 9007–9009 RSC.
- I. Sadeghi, J. Kronenberg and A. Asatekin, Selective Transport through Membranes with Charged Nanochannels Formed by Scalable Self-Assembly of Random Copolymer Micelles, ACS Nano, 2022, 20, 277–295 Search PubMed.
- S. R. Li, F. Y. Huo, H. Q. Wang, J. Wang, C. Xu, B. Liu and L. L. Bu, Recent advances in porous nanomaterials-based drug delivery systems for cancer immunotherapy, J. Nanobiotechnol., 2022, 20, 277 CrossRef PubMed.
- A. Baeza, D. Ruiz-Molina and M. Vallet-Regi, Recent advances in porous nanoparticles for drug delivery in antitumoral applications: inorganic nanoparticles and nanoscale metal–organic frameworks, Expert Opin. Drug Delivery, 2017, 14, 783–796 CrossRef CAS PubMed.
- Y. Li, H. Jahr, J. Zhou and A. A. Zadpoor, Additively manufactured biodegradable porous metals, Acta Biomater., 2020, 115, 29–50 CrossRef PubMed.
- M. Chimutengwende-Gordon, R. Dowling, C. Pendegrass and G. Blunn, Determining the porous structure for optimal soft-tissue ingrowth: An in vivo histological study, PLoS One, 2018, 13, e0206228 CrossRef PubMed.
- Y. Qin, A. Liu, H. Guo, Y. Shen, P. Wen, H. Lin, D. Xia, M. Voshage, Y. Tian and Y. Zheng, Additive manufacturing of Zn-Mg alloy porous scaffolds with enhanced osseointegration: In vitro and in vivo studies, Acta Biomater., 2022, 145, 403–415 CrossRef CAS PubMed.
- C. Zhang, L. Zhang, L. Liu, L. Lv, L. Gao, N. Liu, X. Wang and J. Ye, Mechanical behavior of a titanium alloy scaffold mimicking trabecular structure, J. Orthop. Surg. Res., 2020, 15, 40 CrossRef PubMed.
- G. Mohandas, N. Oskolkov, M. T. McMahon, P. Walczak and M. Janowski, Porous tantalum and tantalum oxide nanoparticles for regenerative medicine, Acta Neurobiol. Exp., 2014, 74, 188–196 CrossRef PubMed.
- V. K. Balla, S. Bodhak, S. Bose and A. Bandyopadhyay, Porous tantalum structures for bone implants: fabrication, mechanical and in vitro biological properties, Acta Biomater., 2010, 6, 3349–3359 CrossRef CAS PubMed.
- Y. Li, H. Jahr, P. Pavanram, F. S. L. Bobbert, U. Paggi, X. Y. Zhang, B. Pouran, M. A. Leeflang, H. Weinans, J. Zhou and A. A. Zadpoor, Additively manufactured functionally graded biodegradable porous iron, Acta Biomater., 2019, 96, 646–661 CrossRef CAS PubMed.
- Y. Li, P. Pavanram, J. Zhou, K. Lietaert, F. S. L. Bobbert, Y. Kubo, M. A. Leeflang, H. Jahr and A. A. Zadpoor, Additively manufactured functionally graded biodegradable porous zinc, Biomater. Sci., 2020, 8, 2404–2419 RSC.
- F. J. Valencia, M. Ramirez, A. Varas, J. Rogan and M. Kiwi, Thermal Stability of Hollow Porous Gold Nanoparticles: A Molecular Dynamics Study, J. Chem. Inf. Model., 2020, 60, 6204–6210 CrossRef CAS PubMed.
- P. V. Asharani, Y. Lianwu, Z. Gong and S. Valiyaveettil, Comparison of the toxicity of silver, gold and platinum nanoparticles in developing zebrafish embryos, Nanotoxicology, 2011, 5, 43–54 CrossRef CAS PubMed.
- T. Chen, T. Gu, L. Cheng, X. Li, G. Han and Z. Liu, Porous Pt nanoparticles loaded with doxorubicin to enable synergistic Chemo-/Electrodynamic Therapy, Biomaterials, 2020, 255, 120202 CrossRef CAS PubMed.
- N. Zhang, D. Zhu, F. Li, H. Hua, X. Tian and Y. Zhao, Low Density Lipoprotein Peptide-Conjugated Gold Nanorods for Combating Gastric Cancer, J. Biomed. Nanotechnol., 2017, 13, 134–143 CrossRef CAS PubMed.
- Y. Liu, J. R. Ashton, E. J. Moding, H. Yuan, J. K. Register, A. M. Fales, J. Choi, M. J. Whitley, X. Zhao, Y. Qi, Y. Ma, G. Vaidyanathan, M. R. Zalutsky, D. G. Kirsch, C. T. Badea and T. Vo-Dinh, A Plasmonic Gold Nanostar Theranostic Probe for In Vivo Tumor Imaging and Photothermal Therapy, Theranostics, 2015, 5, 946–960 CrossRef CAS PubMed.
- F. Tang, L. Li and D. Chen, Mesoporous silica nanoparticles: synthesis, biocompatibility and drug delivery, Adv. Mater., 2012, 24, 1504–1534 CrossRef CAS PubMed.
- Q. Yang, J. Peng, Y. Xiao, W. Li, L. Tan, X. Xu and Z. Qian, Porous Au@Pt Nanoparticles: Therapeutic Platform for Tumor Chemo-Photothermal Co-Therapy and Alleviating Doxorubicin-Induced Oxidative Damage, ACS Appl. Mater. Interfaces, 2018, 10, 150–164 CrossRef CAS PubMed.
- X. He, X. Ma, Y. Yang, X. Hu, T. Wang, S. Chen and X. Mao, Metal Cluster Triggered-Assembling Heterogeneous Au–Ag Nanoclusters with Highly Loading Performance and Biocompatible Capability, Int. J. Mol. Sci., 2022, 23, 11197–11212 CrossRef CAS PubMed.
- S. A. Khan, R. Kanchanapally, Z. Fan, L. Beqa, A. K. Singh, D. Senapati and P. C. Ray, A gold nanocage-CNT hybrid for targeted imaging and photothermal destruction of cancer cells, Chem. Commun., 2012, 48, 6711–6713 RSC.
- E. Ahmed, M. K. Masud, M. S. A. Hossain, J. Na, A. A. Sina, Y. Yamauchi and M. Trau, Nanostructured mesoporous gold electrodes detect protein phosphorylation in cancer with electrochemical signal amplification, Analyst, 2020, 145, 6639–6648 RSC.
- M. K. Masud, J. Na, T. E. Lin, V. Malgras, A. Preet, A. A. Ibn Sina, K. Wood, M. Billah, J. Kim, J. You, K. Kani, A. E. Whitten, C. Salomon, N. T. Nguyen, M. J. A. Shiddiky, M. Trau, M. S. A. Hossain and Y. Yamauchi, Nanostructured mesoporous gold biosensor for microRNA detection at attomolar level, Biosens. Bioelectron., 2020, 168, 112429 CrossRef CAS PubMed.
- H. Park, M. K. Masud, J. Na, H. Lim, H. P. Phan, Y. V. Kaneti, A. A. Alothman, C. Salomon, N. T. Nguyen, M. S. A. Hossain and Y. Yamauchi, Mesoporous gold–silver alloy films towards amplification-free ultra-sensitive microRNA detection, J. Mater. Chem. B, 2020, 8, 9512–9523 RSC.
- M. Xie, H. Shi, Z. Li, H. Shen, K. Ma, B. Li, S. Shen and Y. Jin, A multifunctional mesoporous silica nanocomposite for targeted delivery, controlled release of doxorubicin and bioimaging, Colloids Surf., B, 2013, 110, 138–147 CrossRef CAS PubMed.
- Z.-S. Chen, R. W. Robey, M. G. Belinsky, I. Shchaveleva, X.-Q. Ren, Y. Sugimoto, D. D. Ross, S. E. Bates and G. D. Kruh, Transport of methotrexate, methotrexate polyglutamates, and 17beta-estradiol 17-(beta-D-glucuronide) by ABCG2: effects of acquired mutations at R482 on methotrexate transport, Cancer Res., 2003, 63, 4048–4054 CAS.
- F. P. Seib and D. L. Kaplan, Doxorubicin-loaded silk films: drug-silk interactions and in vivo performance in human orthotopic breast cancer, Biomaterials, 2012, 33, 8442–8450 CrossRef CAS PubMed.
- Y. Wu, E. K. Shih, A. Ramanathan, S. Vasudevan and T. Weil, Nano-sized albumin-copolymer micelles for efficient doxorubicin delivery, Biointerphases, 2012, 7, 5 CrossRef CAS PubMed.
- L. Sandiford, A. Phinikaridou, A. Protti, L. K. Meszaros, X. Cui, Y. Yan, G. Frodsham, P. A. Williamson, N. Gaddum, R. M. Botnar, P. J. Blower, M. A. Green and R. T. M. de Rosales, Bisphosphonate-anchored PEGylation and radiolabeling of superparamagnetic iron oxide: long-circulating nanoparticles for in vivo multimodal (T1 MRI-SPECT) imaging, ACS Nano, 2013, 7, 500–512 CrossRef CAS PubMed.
- M. J. Jeon, A. C. Gordon, A. C. Larson, J. W. Chung, Y. I. Kim and D. H. Kim, Transcatheter intra-arterial infusion of doxorubicin loaded porous magnetic nano-clusters with iodinated oil for the treatment of liver cancer, Biomaterials, 2016, 88, 25–33 CrossRef CAS PubMed.
- W. Zhao, Z. Huang, L. Liu, W. Wang, J. Leng and Y. Liu, Porous bone tissue scaffold concept based on shape memory PLA/Fe3O4, Compos. Sci. Technol., 2021, 203, 108563–108604 CrossRef CAS.
- P. C. Wu, W. S. Wang, Y. T. Huang, H. S. Sheu, Y. W. Lo, T. L. Tsai, D. B. Shieh and C. S. Yeh, Porous iron oxide based nanorods developed as delivery nanocapsules, Chemistry, 2007, 13, 3878–3885 CrossRef CAS PubMed.
- T. Gong, X. Song, L. Yang, T. Chen, T. Zhao, T. Zheng, X. Sun, T. Gong and Z. Zhang, Spontaneously formed porous structure and M(1) polarization effect of Fe(3)O(4) nanoparticles for enhanced antitumor therapy, Int. J. Pharm., 2019, 559, 329–340 CrossRef CAS PubMed.
- M. K. Masud, S. Yadav, M. N. Islam, N. T. Nguyen, C. Salomon, R. Kline, H. R. Alamri, Z. A. Alothman, Y. Yamauchi, M. S. A. Hossain and M. J. A. Shiddiky, Gold-Loaded Nanoporous Ferric Oxide Nanocubes with Peroxidase-Mimicking Activity for Electrocatalytic and Colorimetric Detection of Autoantibody, Anal. Chem., 2017, 89, 11005–11013 CrossRef CAS PubMed.
- S. Balaban Hanoglu, D. Harmanci, N. Ucar, S. Evran and S. Timur, Recent Approaches in Magnetic Nanoparticle-Based Biosensors of miRNA Detection, Magnetochemistry, 2023, 9, 23 CrossRef CAS.
- R. Bhattacharjee, S. Tanaka, S. Moriam, M. K. Masud, J. Lin, S. M. Alshehri, T. Ahamad, R. R. Salunkhe, N. T. Nguyen, Y. Yamauchi, M. S. A. Hossain and M. J. A. Shiddiky, Porous nanozymes: the peroxidase-mimetic activity of mesoporous iron oxide for the colorimetric and electrochemical detection of global DNA methylation, J. Mater. Chem. B, 2018, 6, 4783–4791 RSC.
- K. Boriachek, M. K. Masud, C. Palma, H. P. Phan, Y. Yamauchi, M. S. A. Hossain, N. T. Nguyen, C. Salomon and M. J. A. Shiddiky, Avoiding Pre-Isolation Step in Exosome Analysis: Direct Isolation and Sensitive Detection of Exosomes Using Gold-Loaded Nanoporous Ferric Oxide Nanozymes, Anal. Chem., 2019, 91, 3827–3834 CrossRef CAS PubMed.
- F. Bai, D. Wang, Z. Huo, W. Chen, L. Liu, X. Liang, C. Chen, X. Wang, Q. Peng and Y. Li, A versatile bottom-up assembly approach to colloidal spheres from nanocrystals, Angew. Chem., Int. Ed., 2007, 46, 6650–6653 CrossRef CAS PubMed.
- Z. Lu, M. Ye, N. Li, W. Zhong and Y. Yin, Self-assembled TiO2 nanocrystal clusters for selective enrichment of intact phosphorylated proteins, Angew. Chem., Int. Ed., 2010, 49, 1862–1866 CrossRef CAS PubMed.
- Y. Gao, F. Yang, Q. Yu, R. Fan, M. Yang, S. Rao, Q. Lan, Z. Yang and Z. Yang, Three-dimensional porous Cu@Cu(2)O aerogels for direct voltammetric sensing of glucose, Mikrochim. Acta, 2019, 186, 192 CrossRef PubMed.
- Y.-Y. Li, P. Kang, S.-Q. Wang, Z.-G. Liu, Y.-X. Li and Z. Guo, Ag nanoparticles anchored onto porous CuO nanobelts for the ultrasensitive electrochemical detection of dopamine in human serum, Sens. Actuators, B, 2021, 327, 12887–12896 Search PubMed.
- X. Jin, H. Zhao, Z. Chao, X. Wang, Q. Zhang, H. Ju and Y. Liu, Self-assembled Cupric Oxide Nanoclusters for Highly efficient chemodynamic therapy, Chem. – Asian J., 2022, 17, e202200296 CrossRef CAS PubMed.
- J. Wang, J. Ye, W. Lv, S. Liu, Z. Zhang, J. Xu, M. Xu, C. Zhao, P. Yang and Y. Fu, Biomimetic Nanoarchitectonics of Hollow Mesoporous Copper Oxide-Based Nanozymes with Cascade Catalytic Reaction for Near Infrared-II Reinforced Photothermal-Catalytic Therapy, ACS Appl. Mater. Interfaces, 2022, 14, 40645–40658 CrossRef CAS PubMed.
- P. Ling, Q. Zhang, T. Cao and F. Gao, Versatile Three-Dimensional Porous Cu@Cu(2) O Aerogel Networks as Electrocatalysts and Mimicking Peroxidases, Angew. Chem., Int. Ed., 2018, 57, 6819–6824 CrossRef CAS PubMed.
- L. Feng, C. Zhang, G. Gao and D. Cui, Facile synthesis of hollow Cu2O octahedral and spherical nanocrystals and their morphology-dependent photocatalytic properties, Nanoscale Res. Lett., 2012, 7, 276 CrossRef PubMed.
- S. Sun and Z. Yang, Cu2O-templated strategy for synthesis of definable hollow architectures, Chem. Commun., 2014, 50, 7403–7415 RSC.
- Z. Qi and J. Weissmüller, Hierarchical nested-network nanostructure by dealloying, ACS Nano, 2013, 7, 5948–5954 CrossRef CAS PubMed.
- X. Song, W. Xu, D. Su, J. Tang and X. Liu, The Synthesis of Hollow/Porous CuO Nanoparticles by Ion-Pairing Behavior Control, ACS Omega, 2020, 5, 1879–1886 CrossRef CAS PubMed.
- L. Yu, G. Li, X. Zhang, X. Ba, G. Shi, Y. Li, P. K. Wong, J. C. Yu and Y. Yu, Enhanced Activity and Stability of Carbon-Decorated Cuprous Oxide Mesoporous Nanorods for CO2 Reduction in Artificial Photosynthesis, ACS Catal., 2016, 6, 6444–6454 CrossRef CAS.
- Y. Zhu, Z. Zhang, X. Song and Y. Bu, A facile strategy for synthesis of porous Cu(2)O nanospheres and application as nanozymes in colorimetric biosensing, J. Mater. Chem. B, 2021, 9, 3533–3543 RSC.
- T.-Y. Tsai, S.-J. Chang, T.-J. Hsueh, H.-T. Hsueh, W.-Y. Weng, C.-L. Hsu and B.-T. Dai, p-Cu2O-shell/n-TiO2-nanowire-core heterostucture photodiodes, Nanoscale Res. Lett., 2011, 6, 575 CrossRef PubMed.
- E. Martínez-Ferrero, Y. Sakatani, C. Boissière, D. Grosso, A. Fuertes, J. Fraxedas and C. Sanchez, Nanostructured Titanium Oxynitride Porous Thin Films as Efficient Visible-Active Photocatalysts, Adv. Funct. Mater., 2007, 17, 3348–3354 CrossRef.
- Y.-G. Guo, J.-S. Hu and L.-J. Wan, Nanostructured Materials for Electrochemical Energy Conversion and Storage Devices, Adv. Mater., 2008, 20, 2878–2887 CrossRef CAS.
- A. Jaroenworaluck, W. Sunsaneeyametha, N. Kosachan and R. Stevens, Characteristics of silica-coated TiO2 and its UV absorption for sunscreen cosmetic applications, Surf. Interface Anal., 2006, 38, 473–477 CrossRef CAS.
- Q. Zheng, B. Zhou, J. Bai, L. Li, Z. Jin, J. Zhang, J. Li, Y. Liu, W. Cai and X. Zhu, Self-Organized TiO2 Nanotube Array Sensor for the Determination of Chemical Oxygen Demand, Adv. Mater., 2008, 20, 1044–1049 CrossRef CAS.
- J. Xu, Y. Sun, J. Huang, C. Chen, G. Liu, Y. Jiang, Y. Zhao and Z. Jiang, Photokilling cancer cells using highly cell-specific antibody-TiO(2) bioconjugates and electroporation, Bioelectrochemistry, 2007, 71, 217–222 CrossRef CAS PubMed.
- M. Geetha, A. K. Singh, R. Asokamani and A. K. Gogia, Ti based biomaterials, the ultimate choice for orthopaedic implants – A review, Prog. Mater. Sci., 2009, 54, 397–425 CrossRef CAS.
- M. Belicchi, S. Erratico, P. Razini, M. Meregalli, A. Cattaneo, E. Jacchetti, A. Farini, C. Villa, N. Bresolin, L. Porretti, C. Lenardi, P. Milani and Y. Torrente,
Ex vivo expansion of human circulating myogenic progenitors on cluster-assembled nanostructured TiO2, Biomaterials, 2010, 31, 5385–5396 CrossRef CAS PubMed.
- T. Wang, H. Jiang, L. Wan, Q. Zhao, T. Jiang, B. Wang and S. Wang, Potential application of functional porous TiO2 nanoparticles in light-controlled drug release and targeted drug delivery, Acta Biomater., 2015, 13, 354–363 CrossRef CAS PubMed.
- M. Yin, E. Ju, Z. Chen, Z. Li, J. Ren and X. Qu, Upconverting nanoparticles with a mesoporous TiO(2) shell for near-infrared-triggered drug delivery and synergistic targeted cancer therapy, Chemistry, 2014, 20, 14012–14017 CrossRef CAS PubMed.
- P. Si, S. Ding, J. Yuan, X. W. D. Lou and D.-H. Kim, Hierarchically structured one-dimensional TiO2 for protein immobilization, direct electrochemistry, and mediator-free glucose sensing, ACS Nano, 2011, 5, 7617–7626 CrossRef CAS PubMed.
- G. K. Mor, K. Shankar, M. Paulose, O. K. Varghese and C. A. Grimes, Enhanced photocleavage of water using titania nanotube arrays, Nano Lett., 2005, 5, 191–195 CrossRef CAS PubMed.
- Q. Guo, L. Liu, M. Zhang, H. Hou, Y. Song, H. Wang, B. Zhong and L. Wang, Hierarchically mesostructured porous TiO(2) hollow nanofibers for high performance glucose biosensing, Biosens. Bioelectron., 2017, 92, 654–660 CrossRef CAS PubMed.
- K. M. Kim, H. R. Kim, K. I. Choi, H. J. Kim and J. H. Lee, Design of highly sensitive C2H5OH sensors using self-assembled ZnO nanostructures, Sensors, 2011, 11, 9685–9699 CrossRef CAS PubMed.
- A. Santos, V. S. Balderrama, M. Alba, P. Formentín, J. Ferré-Borrull, J. Pallarès and L. F. Marsal, Tunable Fabry–Pérot interferometer based on nanoporous anodic alumina for optical biosensing purposes, Nanoscale Res. Lett., 2012, 7, 370 CrossRef PubMed.
- A. Santos, T. Kumeria and D. Losic, Optically optimized photoluminescent and interferometric biosensors based on nanoporous anodic alumina: a comparison, Anal. Chem., 2013, 85, 7904–7911 CrossRef CAS PubMed.
- B. Mangalampalli, N. Dumala and P. Grover, Acute oral toxicity study of magnesium oxide nanoparticles and microparticles in female albino Wistar rats, Regul. Toxicol. Pharmacol., 2017, 90, 170–184 CrossRef CAS PubMed.
- C. Martinez-Boubeta, L. Balcells, R. Cristòfol, C. Sanfeliu, E. Rodríguez, R. Weissleder, S. Lope-Piedrafita, K. Simeonidis, M. Angelakeris, F. Sandiumenge, A. Calleja, L. Casas, C. Monty and B. Martínez, Self-assembled multifunctional Fe/MgO nanospheres for magnetic resonance imaging and hyperthermia, Nanomedicine, 2010, 6, 362–370 CrossRef CAS PubMed.
- D.-R. Di, Z.-Z. He, Z.-Q. Sun and J. Liu, A new nano-cryosurgical modality for tumor treatment using biodegradable MgO nanoparticles, Nanomedicine, 2012, 8, 1233–1241 CrossRef CAS PubMed.
- S. Shen, P. S. Chow, F. Chen and R. B. H. Tan, Submicron particles of SBA-15 modified with MgO as carriers for controlled drug delivery, Chem. Pharm. Bull., 2007, 55, 985–991 CrossRef CAS PubMed.
- X. Qu, Z. Liu, N. Li, B. Ma, H. Zhao, Y. Li, B. Lei and Y. Du, Biodegradable biocompatible MgO/Eu nanodrug with Acid–Base conversion capacity for targeted lung cancer therapy, Chem. Eng. J., 2022, 446, 136323–136333 CrossRef CAS.
|
This journal is © The Royal Society of Chemistry 2024 |