Tetrahydrocannabinol (THC)-modified screen-printed carbon electrodes (SPCEs): insights into stability†
Received
1st April 2024
, Accepted 21st June 2024
First published on 3rd July 2024
Abstract
THC (Δ9-tetrahydrocannabinol) is the major psychoactive constituent of cannabis, accountable for instigating euphoric effects in individuals. With the imperative need for a roadside testing device for the detection of THC, this study aims to overcome the challenges associated with the stability of a biomolecule-free sensor capable of detecting ultra-low concentrations of THC. Since THC is highly susceptible to oxidation, this study explores different avenues to create optimal storage conditions to minimize the oxidation of THC prior to detection. Hence, all experiments were conducted by controlling the principal factors contributing to the oxidation of THC: temperature, humidity, airflow and light, to extend the shelf-life of the manufactured electrodes and engender stable electrochemical signals. It is concluded that frozen storage conditions and a second acidic pH modification were ideal for improving the stability of the modified electrodes from day one up to a period of six months.
1. Introduction
Δ9-Tetrahydrocannabinol (THC) is a common natural component of the plant Cannabis sativa.1 It is the chief psychoactive product of marijuana (cannabis) and is responsible for exhibiting euphoric effects in individuals. The legalization of medical and recreational marijuana in Canada has increased the number of drug-impaired drivers and the associated number of accidents.2–5 Also, epidemiologic data shows that the danger of being involved in a motor vehicle accident (MVA) increases 2-fold after cannabis consumption.4 Hence, regulatory authorities require the development of roadside testing devices for the detection of THC to reduce cannabis-impaired driving. In order to be viable for the roadside testing of THC, the sensing device must be easy to use, portable, economical, and operable under ambient environmental conditions, and it must administer results preferably in less than 5 minutes. However, the current sensing devices available are either enzyme or biomolecule-based6 and, therefore, are obligated to undergo complex procedures for the adsorption of the analyte7 resulting in the whole process being extremely time-consuming. The primary challenges confronting these sensors are nonspecific interactions and interference from compounds and species within the matrix. Additionally, there is a significant challenge in the specialized storage requirements for sensors modified with antibodies or proteins.8
Several devices have been reported for the effective and rapid roadside detection of cannabis-impaired drivers. The study9 demonstrates the voltammetric detection of phenol, 4-phenoxyphenol, methylphenol (para and meta), nitrophenol, and notably, tetrahydrocannabinol (THC), highlighting its potential for roadside testing of cannabis in drivers. Organic electrochemical transistors (OECTs) have been employed for various biosensing applications such as glucose, pH, and ion detection. Majak and colleagues10 showcase the use of unfunctionalized OECTs for detecting Δ9-THC at concentrations as low as 0.1 nM in deionized water and 1 nM in synthetic saliva buffer. Goodwin and associates11 reported a detection protocol involves the electrochemical formation of quinoneimine, which specifically reacts with Δ9-THC, leading to its depletion that can be monitored via voltammetry. Another biosensor reported by Stevenson et al.,12 demonstrates its functionality when integrated with low-power electronics and a portable saliva swab, serving as a handheld roadside DUI testing platform for rapid THC detection in human saliva samples.
Ortega and co-workers13 introduced a biomolecule-free electrochemical sensing approach for the detection of ultra-low concentrations of THC in phosphate buffer (PBS), real saliva, and simulated saliva. Their sensing device uses the approach of depositing THC molecules (THCi) onto the working electrode (WE) of the screen-printed carbon electrode (SPCE) prior to detection (Fig. 1). In this case, the THCi on the WE improves the interaction with the THC analytes by covalent binding and physical interactions. The final electrochemical detection is associated with the irreversible oxidation of the phenol group of THC. The proposed design is also successful in meeting the desired criteria listed above, for the device is easily operable, portable, cost-effective, and has a testing time of only 38 seconds.
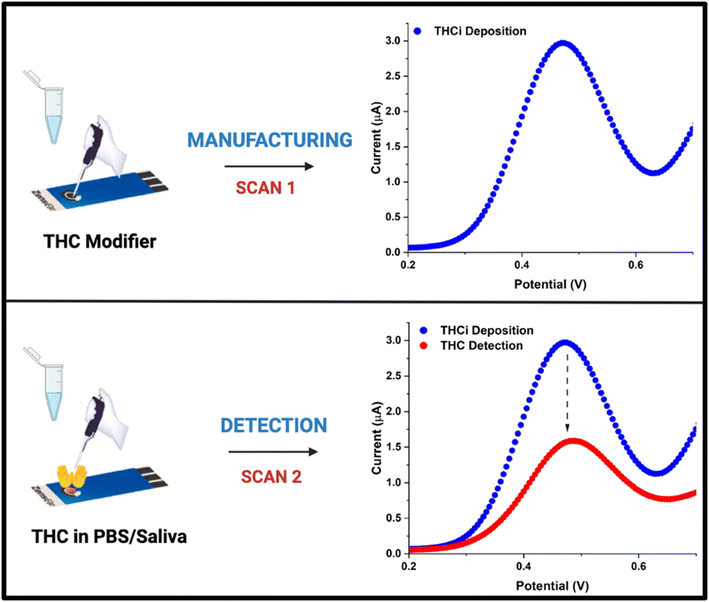 |
| Fig. 1 Schematic representation of the manufacturing and detection process proposed by Ortega et al.13 | |
Although this approach13 has created an extremely efficient testing system for the detection of THC, it presents a major challenge. After the working electrode of the sensor is manufactured with an initial THCi electrodeposition, the intensity of the current is observed to be proportional to the amount of THC deposited when subjected to the electrochemical method of square wave voltammetry (SWV). Also, the decrease in the intensity of the signals from the manufacturing (SCAN 1) to the detection scan (SCAN 2) is a result of the partial oxidation of THCi plus the contribution of the THC analyte (Fig. 1). However, with THCi being highly susceptible to oxidation, the intensity of the current decreases significantly with a lack of reproducibility when testing the modified electrode for sample detection via SWV. The ability of THC to oxidize so easily makes the pre-treatment process very difficult, impacting the test process and the corresponding test results negatively. Thus, this research paper stems from the study above13 and aims to explore different avenues to create an ambient environment that can potentially minimize THC oxidation prior to detection. Characteristics pertaining to the shelf-life of biosensors are highly crucial to their overall performance and yet are often not reported in literature studies.
The stability of the biosensor strongly dictates its commercial viability. All biological products, including biosensors, are prone to aging, directly influencing the functionality of the sensor. The ageing of a biosensor can be defined as a decrease in its sensitivity over the course of time. A study conducted by Panjan and colleagues14 provides insight into the ageing of biosensors to be linearly dependent on temperature conditions. The study concludes that irrespective of their application, all electrochemical biosensors degraded faster at higher temperatures. Additionally, the study proposes a novel method to examine the characteristics of rapid aging by using Arrhenius and linear models. Consequently, temperature, humidity, airflow & light are the four principal factors which contribute to the oxidation of THC. Controlling these factors can result in extending the shelf-life of the manufactured electrodes and provide stable intensity signals during the detection of THC. Therefore, the main objective of this research is to explore different storage conditions suitable for preserving THC by minimizing the oxidation of THC during manufacturing. Finally, the sensor performance will be evaluated after applying the optimized storage conditions.
2. Materials and methods
2.1 Materials
TE-100 SPCEs with carbon-based working (geometric dimensions 3 mm/7.1 mm2 and electroactive surface area of 6 mm2) and counter electrodes (10.6 mm2), including a silver ink-printed pseudo-reference electrode (1 mm2) purchased from Zensor R&D were used for the purposes of this research. In addition, the working electrode presented a geometric area of 0.07 cm2 with an effective electroactive area of 85% (0.06 cm2) by the traditional determination by cyclic voltammetry interrogation at different scan rates in a ferricyanide/ferrocyanide solution.13,15 The deposition of the analyte onto the working electrode (WE) of the Zensor electrodes was performed using BioDot, a machine-driven dispensing technology (AD15200107). The Glove Box 2700 Series and a multifunctional food vacuum sealer were purchased from Cleatech LLC and Hogance, respectively, to store the electrodes in a low-pressure and an inert environment. Metallic mylar Ziploc bags lined with aluminum foil were used to impede the exposure of the THC-modified electrodes to light. Additionally, oxygen scavenger sachets were used to reduce the oxygen levels to less than or equal to 0.1%. The electrochemical detection was carried out using a PalmSens4 potentiostat driven by the PSTrace 5-Palm-Sens software. Lastly, Δ9-tetrahydrocannabinol solution (1.0 mg mL−1) in methanol was purchased from Sigma-Aldrich.
2.2 Methods
2.2.1 Electrode cleaning.
The TE-100 Zensor pristine electrodes were washed using milli Q water and dried using hot airflow in the fume hood.
2.2.2 Electrode modification.
The washed electrodes were modified with 130 ng of the Δ9-THC solution (THCi) prepared onto the WE using BioDot and then dried in the fume hood using warm airflow. The dried electrodes were then inserted into the multi-potentiostat and scanned (SCAN 1) with PBS (pH 7.4) using the following conditions for SWV: a precondition potential of 0.05 V for 0 s, an equilibration time of 3 s, a voltammetric potential scan from 0 to 0.8 V, a frequency of 15 Hz, an amplitude of 0.025 V and a step potential of 0.005 V.
2.2.3 Additional modifiers.
The THCi-modified electrodes were also used in conjunction with a second modification of an additional modifier to minimize further oxidation. Hence, this modification was performed upon the completion of section 2.2.2. The additional modifiers include PBS (pH 4), 13 ng of ascorbic acid (AA), 13 ng of citric acid (CA), and 5.2 ng of butylated hydroxytoluene (BHT) in conjunction with diethylene glycol monoethyl ether (Transcutol).
2.2.4 Alternative modifiers.
Modifiers with similar electrochemical behaviour to that of THC were pre-deposited onto the WE as an alternative to THCi deposition. In this case, the modification stated in section 2.2.2 was performed using the following modifiers instead of THC: 130 ng of tetrahydrocannabinolic acid (THCA), 130 ng of Δ9-tetrahydrocannabinol acetate (THC-OAc), 100 ng of dopamine (DA), and 100 ng and 130 ng of cannabidiol (CBD).
2.2.5 Electrode storage.
Electrodes prepared in section 2.2.2, 2.2.3, and 2.2.4 were vacuum sealed using oxygen absorber sachets in mylar Ziploc bags and then stored at different temperature conditions.
2.2.5.1 Optimizing temperature.
It has been reported that THC is highly unstable at room temperature16 and exhibits favourable stability conditions at lower temperatures.17–19 Hence, the electrochemical sensors were stored at room temperature (RT), 4 °C, and −20 °C for the purposes of shelf-life studies.
2.2.5.2 Controlling atmospheric oxidation.
Oxygen absorbers function via the occurrence of a chemical reaction; they are comprised of powdered iron, which reacts with the oxygen in the atmosphere to form iron oxide, resulting in the rusting of the iron powder. Upon the oxidization of the iron powder, the O2 absorbers are loaded, and hence, the absorbing mechanism ends. Subsequently, they are known to help reduce oxygen levels to less than or equal to 0.1%. Air oxidation plays a crucial role in contributing to the oxidation of THC.20,21
2.2.5.3 Eliminating exposure to light.
Mylar is a clear material made from polyester resin, which possesses numerous desirable properties for long-term storage, such as gas and moisture resistance, chemical stability, and high tensile strength. Mylar bags offer an extremely low oxygen transmission rate (OTR) due to their thick foil laminate layer and are impermeable to gases. These bags help in providing a thick layer of protection from moisture, light, and odour. The oxidative degradation of THC to CBN is known to have been accelerated when exposed to light.22,23
2.2.5.4 Minimizing airflow.
Lastly, to minimize airflow, two different vacuum systems were implemented. In the first system, the vacuum pump was used in conjunction with a glove box purged with nitrogen (N2) gas. The electrodes and desiccants were vacuum sealed for the second vacuum system using a simple food vacuum sealer. Vacuum packing diminishes the amount of oxygen exposure and airflow protects the contents from dust and moisture, and shields the electrodes from any physical damage. Literature has previously recommended storing THC under nitrogen gas while in the dark.24–26
2.2.6 Schematic procedural overview.
Fig. 2 shows a schematic representation of the preparation of the THCi-modified electrode and the different storage conditions used during the manufacturing process.
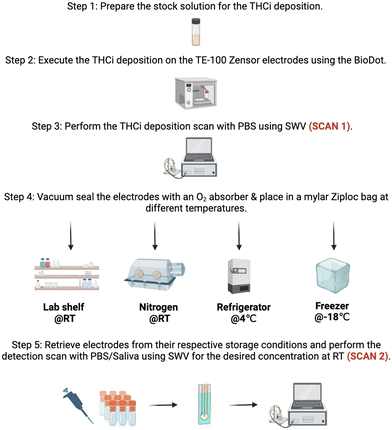 |
| Fig. 2 Schematic representation of the procedural overview of the storage conditions during the manufacturing. | |
2.3 Detection
The electrodes were retrieved from their respective storage conditions after the specified time (Table 1) and subjected to SWV for the final reproducibility scan (SCAN 2) post-storage. All of the electrodes were tested at RT using PBS using the following SWV conditions: a precondition potential of 0.05 V for 30 s, an equilibration time of 3 s, a voltammetric potential scan from 0 to 0.8 V, a frequency of 15 Hz, an amplitude of 0.025 V and a step potential of 0.005 V.
Table 1 A summary of all the stability experiments conducted using different temperature conditions
2.4 Sensor performance after storage conditions optimization
The TE-100 Zensor electrodes were modified with 100 ng of THCi deposited onto the WE using the BioDot, vacuum sealed with oxygen absorbers and then stored in the freezer at −18 °C for one week. The electrodes were then retrieved from their respective storage conditions after days 5, 6 and 7 and then tested (after reaching RT upon thawing) with THC samples in PBS (pH 7.4) with concentrations 0, 5, 10, 25, 50, 100, 300 and 1000 ng mL−1. The electrodes were tested using the following SWV conditions: a precondition potential of 0.05 V for 30 s, an equilibration time of 3 s, a voltammetric potential scan from 0 to 0.8 V, a frequency of 15 Hz, an amplitude of 0.025 V and a step potential of 0.005 V.
2.5 Characterization
X-ray photoelectron spectroscopy (XPS) was performed for the purposes of examining the best temperature condition for the storage of the THCi-modified electrodes. The THCi-modified electrodes were stored at RT, 4 °C, and −18 °C for a period of one week for XPS analysis. The XPS analyses were performed with a PHI Quantera II Scanning XPS Microprobe spectrometer. Survey scan analyses were collected with 300 × 700 μm for analysis area and 224 eV of pass energy. High-resolution studies were recorded with 20 eV of pass energy. Spectra have been charged and corrected to the main line of the carbon 1s spectrum (organic carbon) set to 285 eV. Spectra were analyzed using CasaXPS software (version 2.3.14).
3. Results and discussion
3.1 Electrode degradation
The THCi-modified electrodes vacuum sealed with O2 absorbers and stored at RT for a period of one week (Fig. 3) is detrimental in showcasing the instability of the modified electrodes as a result of the oxidation of THC. Additionally, as portrayed in Fig. 1, the decrease in the peak values of the THCi-modified electrodes from the manufacturing (SCAN 1) to the detection scan (SCAN 2), also performed at RT, further validates the instability of the electrodes.
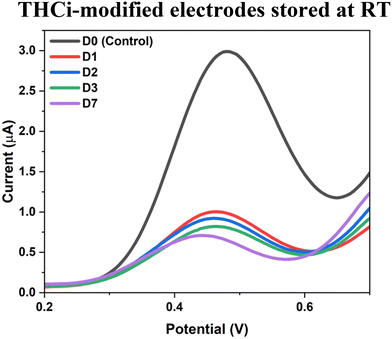 |
| Fig. 3 THCi-modified electrodes (130 ng) vacuum sealed with oxygen absorbers at RT for a period of one week, where D = day. | |
It has been well established in the literature that cannabis-based products tend to lose their potency during storage due to a loss in the amount of Δ9-tetrahydrocannabinol (Δ9-THC).27 Also, the stability of different cannabinoids is directly dependent on their storage conditions.28 Before extraction, THC and CBD exist in their acidic forms, i.e., as THCA and CBDA in the corporeal plant. The neutral cannabinoids, THC and CBD, are known to be stable for a period of two weeks at room temperature when stored in darkness. However, exposure to light can result in the deterioration of these compounds. Opposingly, the acidic cannabinoids are capable of decarboxylating into their neutral forms in the daylight, as well as in the dark. Besides, oxidation is known to be a process that is highly reliant on temperature.21,22
In addition, THC is a highly unstable compound which is easily oxidized to its nonenzymatic oxidation by-product cannabinol (CBN) under improper and prolonged storage conditions (Fig. 4). CBN consists of 1/4th the potency of THC, and hence, it is considered to be the less psychoactive compound. CBN is also the final product of the oxidation process, which seems to be dependent predominantly on heat and light.27 Studies17–19 validate the effect of heat and light on cannabinoid stability by presenting that the Δ9-THC content was found to be higher in samples stored at 4 °C and −18 °C in the dark, as opposed to samples stored at room temperature with exposure to light.
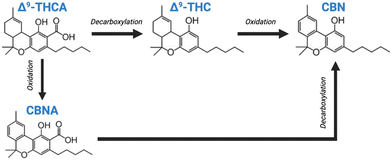 |
| Fig. 4 The decarboxylation and oxidation of Δ9-THC. | |
Both cannabinoids, THC and CBD, are highly electroactive species due to the presence of their respective hydroxyl groups.29 Subsequently, the hydroxyl groups become deprotonated during oxidation and result in the formation of active phenoxy radicals.30 This can lead to the prospective irreversible dimerization of both cannabinoids at low potentials, resulting in the generation of an insulating layer on the surface of the WE after the first run.31 Jaidee and colleagues17 conducted a study to examine the kinetics of Δ9-THC degradation in cannabis resin at different temperatures and pH conditions. Their study exhibits first-order and pseudo-first-order degradation kinetics for CBD and THC, respectively. Additionally, minimal transformation of THC was achieved with low temperature and moderately acidic pH values in conjunction with shorter processing times. Another study20 showed that THC samples stored at lower humidity levels (0% and 13% relative humidity) were deemed more stable with respect to degradation, as opposed to those stored at higher humidity levels. Additionally, it was found that air oxidation is another major factor contributing to the loss of Δ9-THC during cannabis storage.21
3.2 Electrode stability
3.2.1 Electrode storage.
The THCi-modified TE-100 Zensor electrodes vacuum sealed with O2 absorbers and stored on the lab bench at RT, under N2 gas in the glove box at RT, in the refrigerator at 4 °C and, in the freezer at −18 °C for a span of one week are illustrated below in Fig. 5. The corresponding electrochemical signals obtained via SWV indicate that the set of electrodes stored at RT, irrespective of the storage condition, demonstrate instability as a result of the decrease in the peak intensity values of the electrodes over time. The electrochemical signals for electrodes stored at 4 °C present a decrease in the intensity after day one, after which the electrodes maintained their stability. Irrevocably, the electrodes stored at −18 °C presented a consistent stability over the entire span of one week, and hence, this condition was further used to examine the long-term stability of the electrodes.
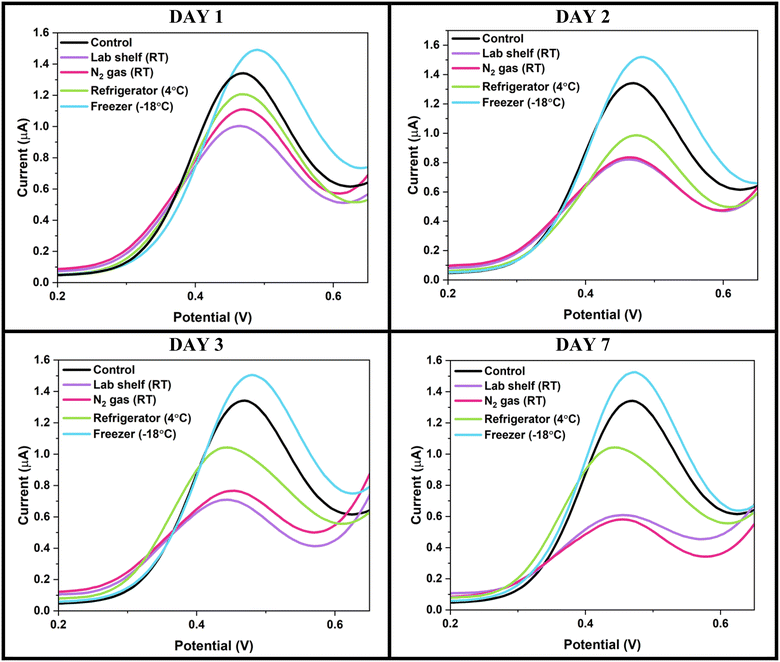 |
| Fig. 5 THCi-modified electrodes (130 ng) vacuum sealed with oxygen absorbers at different temperature conditions. | |
The THCi-modified TE-100 Zensor electrodes vacuum sealed with O2 absorbers and stored in the freezer at −18 °C for a period of six months, is depicted in Fig. 6. The electrodes were tested after 15–20 minutes (i.e., the time consumed to reach RT upon thawing) with PBS (pH 7.4) using SWV. The electrodes are stable over the entire span of the 6-month storage period at −18 °C, supported by the low variability presented over time in the corresponding column graph.
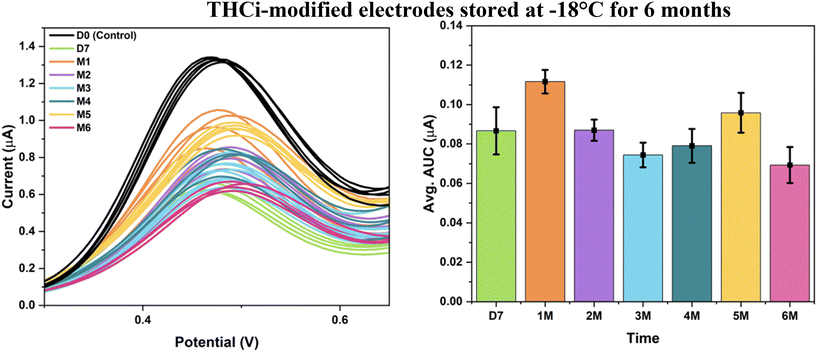 |
| Fig. 6 THCi-modified electrodes (130 ng) at −18 °C over the span of 6 months (tested at RT). | |
The practical significance of using these storage conditions for the long-term stability of the modified electrodes was further validated using statistical analyses (see ESI,† section S1). Specifically, a one-way analysis of variance (ANOVA) test was conducted to provide statistical evidence associated with the best storage conditions presented in conjunction with Levene's test for determining the homogeneity of variance and the Tukey–Kramer test to compare multiple groups' means for each of the time periods. The corresponding coefficient of variation (CV) obtained for Fig. 6 (S1,† section a) of 4.28% indicates a relatively low level of variability in the dataset when compared with the mean. The results from the Tukey test showcased that the group means for the pairs M2–D7 and M5–M4 comprised insignificant differences statistically. With these time periods crucial in serving as the beginning and the end of the shelf-life study, the practical significance of storing the THCi-modified electrodes at −18 °C for a period of 6 months is validated. Additionally, the data also implies that although the error bars present higher variability amongst the electrodes during the first month of storage, this variability begins to decrease from the first month till the sixth.
The results are in compliance with literature studies, which state that the oxidation of THC is arrested at temperatures as low as −18 °C. Repka et al.32 fabricated an HPC polymeric film comprised of THC to examine THC content and the extent of its degradation to CBN at fixed temperatures and time intervals. Their study concludes that the degradation of THC was insignificant for up to 15 months when stored at −18 °C, with nearly 70% of the THC content still preserved. The study also reports that the percentage of CBN formation was at its maximum at 25 °C, as opposed to lower temperatures such as 4 °C and −18 °C. Another study33 articulates that the concentrations of THC-glucuronide in urine samples were stable for a period of one year at −20 °C, after which the occurrence of adsorption, protein binding, or molecular degradation is probable. Additionally, the decarboxylation of THC has been proven to be occurring moderately when stored in the dark at −25 °C.34
3.2.1.1 XPS characterization.
The XPS characterization of the modified electrodes before and after being stored at different conditions (room temperature (RT), fridge at 4 °C (4CT), and freezer at −18 °C (−18CT)) was carried out to understand the effect of each condition on the working electrode modification better. Carbon, oxygen, nitrogen, chlorine, silicon, and iron are the principal elements present in the working electrode of the screen-printed electrode employed in this study. After the electrode modification, an increase in the atomic percent of the carbon and oxygen elements is evidenced on the surface of the working electrode due to the incorporation of THC molecules (ESI,† Table S2a).
The C 1s high-resolution signal for the pristine screen-printed electrode (P–Z) was deconvoluted into four peaks at 284.4, 285.0, 286.5, and 289.0 eV attributed to C
C (aromatic), C–C/C–CH, C–OH/C–O–C/C–Cl, and O–C
O, respectively.35 After the electrode modification, an increase in the atomic percent of the second and third contributions and a decrease in the second peak are verified, which corroborates the incorporation of THC on the surface of the working electrode (Fig. 7, Table S2b in ESI†). In the case of O 1s high-resolution signal for P–Z electrodes, two contributions were employed for this signal fit, which were related to C
O (532.5 eV) and O–(C
O)–C/C–O (aromatic) (533.5 eV).35 Once the THCi-modified electrodes are prepared, a decrease and an increase in the atomic percent in the first (68.3% to 51.5%) and second contributions (31.7% to 48.5), respectively, is observed (Fig. 7, Table S2c in ESI†). These results are attributed to the incorporation of the THC molecules on the working electrode surface and the oxidation of the quinones groups of THC structure, which appear at 532.2 eV (C
O, first contribution).
 |
| Fig. 7 C 1s and O 1s high resolution for THCi-modified electrodes after working electrode modification, a and e) control, and after being stored at different conditions, b and f) room temperature (RT), c and g) fridge at 4 °C (4CT), and d and h) freezer −18 °C (−18CT). | |
After the preparation of THCi-modified electrodes, different conditions for the modified electrode storage were studied to find the best requirements that allow the extension of the modified electrodes' usability for long periods. Fig. 7 shows the results of C 1s and O 1s high-resolution signals before and after storing the modified electrodes for one week at room temperature (RT), fridge at 4 °C (4CT), and freezer at −18 °C (−18CT) (Fig. 7b, c, e, and f). As evidenced in the figure, the signals for both regions (C 1s and O1s) are wider when the electrodes are kept at room temperature (Fig. 7b and f) and 4 °C (fridge) (Fig. 7c and g). However, when the electrodes are stored in the freezer (−18 °C), the signals are less noisy and narrow (Fig. 7d and h). The principal changes in the C 1s high-resolution signals are observed in the three first contributions for RT and 4CT storage conditions when they are compared to the control signal (electrode analyzed after its preparation) (Fig. 7b and c). These three peaks are assigned to C
C (aromatic), C–C/C–CH, and C–OH/C–O–C/C–Cl; for these storage conditions, there is no proper control of the OH functional groups oxidation in the THC molecules and the following formation of quinone, adducts or more complex structures.13 In the case of the −18 °C storage condition, the main changes are observed in the C
C (aromatic), C–OH/C–O–C/C–Cl, and O–C
O (Fig. 7d), where an increase in the atomic percent is evidenced for the last contribution related to the quinone formation, this result suggests that under this temperature there is better control of the THC molecules oxidation and stability of the electrodes during the storage process.
On the other hand, more evident changes are observed in the O 1s peaks after storing the electrodes at the three different conditions. In the case of RT and 4CT storage conditions, the main changes in the atomic percent observed were a decrease in the first contribution (C
O, 532.5 eV) and an increase in the second contribution (O–(C
O)–C/C–O (aromatic), 533.5 eV) (Fig. 7f and g). This result suggests that other processes are occurring in the THC molecules simultaneously as the OH oxidation, such as adducts or more complex structures. In the case of the −18CT condition, an increase in the first contribution (C
O, from 51.5% to 60.0%) and a decrease in the second contribution (O–(C
O)–C/C–O, from 48.5% to 39.4%) (Fig. 7h) can be observed when these results are compared to the control (Fig. 7e). This is related to the possibility that during the application of a potential to create C
O due to the OH groups oxidation in the THC molecules, the formation of quinones is more suitable than other adducts or more complex molecules when the modified electrodes are stored at this temperature, which give the THCi-modified electrode the possibility to be storage for more extended periods of time under this condition. Overall, it was evidenced that oxygen plays the primary role during the working electrode modification and that the best condition for the storage of the THCi-modified electrodes is −18 °C.
3.2.2 Additional modifiers.
The THCi 130 ng modified electrodes were used in conjunction with a second modification of PBS (pH 4), 13 ng AA, 13 ng CA, and 5.2 ng BHT. The results demonstrate that the THCi-modified TE-100 Zensor electrodes with a second modification of PBS (pH 4) vacuum sealed with O2 absorbers and stored at −18 °C were found to be stable for six months when tested at RT with PBS (pH 7.4) (Fig. 8) (see statistical analysis in ESI,† section b). Comparing these results with those depicted in Fig. 7, the electrodes with a second modification of PBS (pH 4) increased the intensity of the electrochemical peak values of the electrodes due to the increase in conductivity caused by the acidic pH value.
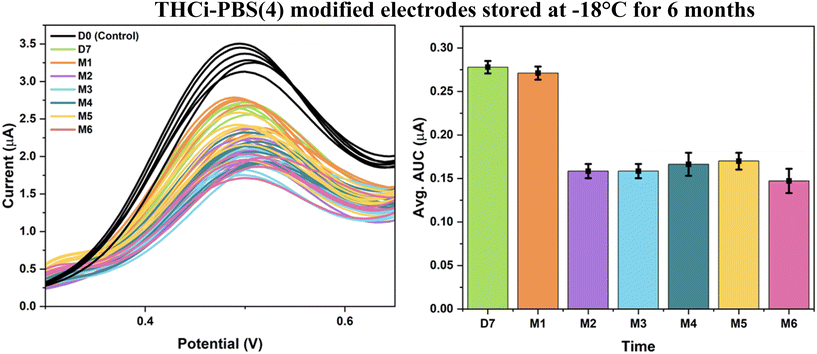 |
| Fig. 8 THCi-modified electrodes (130 ng) with a second modification of PBS (pH 4) at −18 °C over the span of 6 months (tested at RT). | |
When the THCi-modified electrodes with a second modification of PBS (pH 4) were tested using the same conditions at 4 °C, it was evidenced that these electrodes were stable for a period of three months (Fig. 9). Upon the completion of three months in storage, the THC is perceived to be fully oxidized, as depicted by the flat electrochemical signal observed from 4–6 months. The associated Levene's test for absolute deviations concluded that with 0.05 > α for both Fig. 8 and 9, the variances in the AUC values of the electrodes were found to be statistically insignificant over time (ESI,† sections b and c). Interpreting Tukey's test, multiple group means exhibited insignificant differences in the AUC values for the modified electrodes in Fig. 8 and 9 at the 0.05 significance level, crucial for quality control and the stability of the electrode.
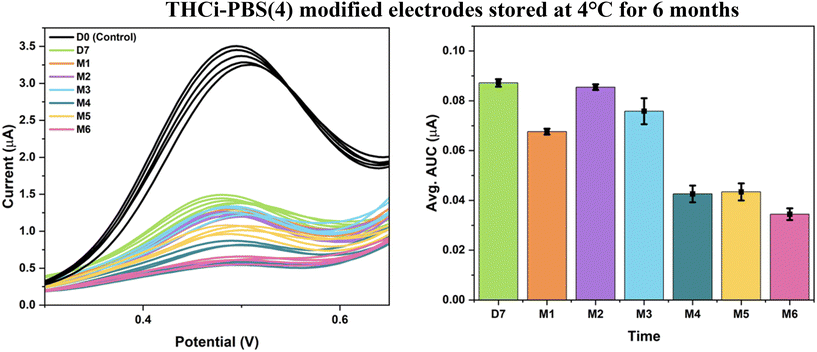 |
| Fig. 9 THCi-modified electrodes (130 ng) with a second modification of PBS (pH 4) at 4 °C over the span of 6 months (tested at RT). | |
As observed by Zanfrognini and colleagues,31 all cases of a modified electrode resulted in increasing the intensity of the peak current as a result of the local conductivity increment by coating the electrode with PBS salt. The surface acidic pH undermines the phenol oxidation of THC and results in improving electrode stabilization. The second modification of PBS was adjusted to pH 4 in order to facilitate restoring the ideal surface pH of the electrode to 7.4 for the purposes of THC detection. As previously stated, Repka's study32 reports the difference between THC degradation occurring at −18 °C versus 4 °C. The study demonstrates that although THC degradation was insignificant at −18 °C with 70% THC content still preserved after 15 months, the degradation continued to occur at 4 °C but at a significantly slower rate, i.e., with 45% THC content preserved for the same time. Therefore, the electrodes stored at −18 °C were able to resist oxidation for a period of 6 months (Fig. 8), as opposed to the electrodes stored at 4 °C, which demonstrated stability for only 3 months (Fig. 9).
Other additional modifiers, including ascorbic acid (AA) and citric acid (CA), were tested as oxygen scavengers (ESI,† section S3). AA-modified and CA-modified electrodes continued to cause a shift in the potential of the peak over time and provided low reproducibility of the scans when stored at 4 °C (Fig. S3a in ESI†). Conjointly, electrodes with a second modification of BHT and Transcutol only remained stable for a period of 48 hours at RT, after which a flat signal was observed for the modified electrode (Fig. S3b in ESI†).
3.2.3 Alternative modifiers.
In this provision, derivates of THC or molecules with similar chemistry and electrochemical performance were used to mimic the THCi function and to obtain better stability. In doing so, electrodes were modified with tetrahydrocannabinolic acid (THCA), Δ9-tetrahydrocannabinol acetate (THC-OAc), dopamine (DA), and cannabidiol (CBD) molecules by using the same electrodeposition protocol (see ESI,† section S3). Electrodes modified with THCA displayed an additional second peak during detection (SCAN 2), implying partial decomposition of the compound into THC (Fig. S4a in ESI†). Opposingly, the DA-modified electrodes displayed an extremely broad electrochemical signal and were unable to detect the oxidation of THC (Fig. S4b in ESI†). With the limit of detection being as low as 2 ng mL−1 for THC, the oxidation of phenolic compounds to quinones was expected to cause interferences in the electrochemical signal. The electrochemical signals obtained for electrodes modified with 100 ng and 130 ng of CBD were similar to that of THC, i.e., illustrated by an electrochemical oxidation peak observed at 0.48 V (Fig. S4c in ESI†). Both THC and CBD are known to undergo the course of oxidation at the same potential values due to their analogous redox active moieties.31 Nevertheless, for both species, the products of the electrochemical reaction present fouling on the surface of the electrode, which causes a drastic decay in the subsequent scans. Lastly, an acetate-protected THC (THC-OAc), a synthetic cannabinoid with a higher stability than THC to oxidation, was also tested as an alternative modifier. This cannabinoid resisted unaltered electrochemical oxidation under the established SWV condition, serving as a perfect candidate to improve electrode stability (Fig. S4d in ESI†). However, aggressive chemical conditions are necessary to hydrolyze the acetate group to obtain the oxidation signal that mimics the THCi function during the THC detection. Therefore, THC-OAc is impractical for our sensor approach.
3.2.4 Experimental summary.
An overview of the stability experiments conducted using different temperature conditions is presented below in Table 1. The temperature conditions that ministered the best stability for the electrodes included storage at 4 °C and −18 °C. Although storing the electrodes at 4 °C rendered short-term stability using a majority of the modifiers tested, storing the electrodes at −18 °C provided superlative long-term stability for the THCi-modified electrodes. Conjointly, the THCi-modified electrodes with a second modification of PBS (pH 4) stored at −18 °C also successfully extended the long-term stability of the electrode to a period of 6 months.
3.3 Sensor performance using the best storage conditions for the modified electrodes
The calibration curves associated with THCi 100 ng modified electrodes vacuum sealed with oxygen absorbers and stored in the freezer at −18 °C for one week, tested using 0, 5, 10, 25, 50, 100, 300, and 1000 ng mL−1 of THC in PBS (pH 7.4) are illustrated in Fig. 10. The electrodes were tested using different concentrations of THC on days 5, 6 and 7 to examine the stability of modified electrodes during detection. With the limit of detection (LOD) and sensitivity proven to be dependent on the temperature of the substrate,36 storing the THCi-modified electrodes at −18 °C was able to influence these parameters positively. The corresponding results demonstrate better linearity, an improved LOD, and maintaining sensitivity over time. Nevertheless, for day 7 the LOD was 0.85 ng mL−1, improving our previous report of 1.1 ng mL−1 in PBS (pH 7.4).13 This suggests that the signal stability of the modified electrodes enhanced with increasing time, implying that the electrodes required a span of 7 days after storage before attaining stability.
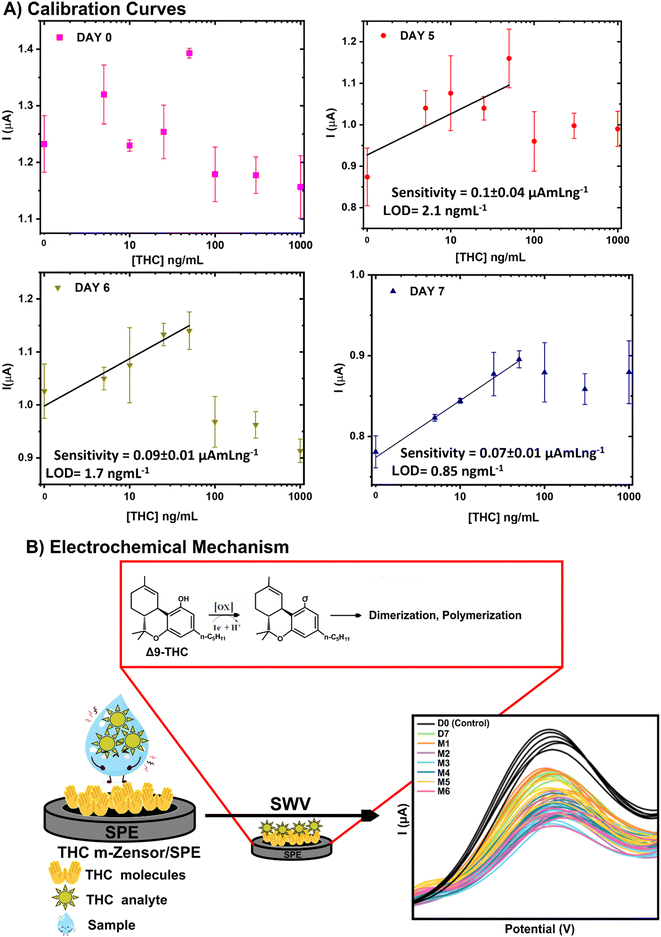 |
| Fig. 10 A) Calibration curves for THCi-modified electrodes (100 ng) stored at −18 °C for a span of one week with THC samples in PBS (pH 7.4). B) Possible electrochemical mechanism. | |
A biosensor must be stored at its reference conditions to examine the time period at which saturation occurs (exponential progression). The time period cannot be defined until saturation transpires, with the process being directly dependent on the application of the biosensor.37,38 The long-term shelf life of a thermally accelerated aging biosensor is known to be established typically after 4 days.14 In this case, the 7-day time period allowed for the electrodes to acclimatize to the temperature and the physical storage environment before suspending the natural phenomena of aging. With biosensor aging defined as a decrease in the electrochemical signal over time, the 7-day time period was adequate in stabilizing the electrochemical signals of the sensor prior to detection. Electrodes often require a period of stabilization for the purposes of conditioning, removal of contaminants, equilibration, minimizing drift, and optimizing performance associated with consistency and reproducibility.39
In addition, the possible electrochemical mechanism for THC-based sensor during the detection of Δ9-tetrahydrocannabinol (THC) via square wave voltammetry (SWV) is presented in Fig. 10B.13,40,41 THC deposition on the working electrode improved the affinity of THC molecules to be detected in the samples through physical or chemical interactions amplifying the final electrochemical signal. In addition, at the molecular level, these interactions facilitate the transfer of electrons or protons between the THC molecules and the electrode surface. This electron/proton exchange process increases the concentration of THC near the electrode surface, enhancing the overall electrochemical activity. As a result, when SWV is applied, the resulting stronger and more distinct signal is due to the increased electron/proton transfer. This enhances the sensitivity and accuracy of THC detection, leveraging the principle that molecules with similar properties recognize and bind to each other more effectively, thus improving the overall performance and reliability of the biosensor. Also, the THC-based electrochemical sensor is supported by the oxidation of the hydroxyl group of the THC under an applied potential to form C
O moieties followed by the formation of adducts or more complex structures.13,41
4. Conclusions
The results obtained during this study substantiate that the frozen storage of the THCi-modified electrodes provided optimal THC stability. Although the results illustrate that the degradation of THC impedes at 4 °C, the oxidation of THC was found to be arrested at −18 °C. While the storage of the THCi-modified electrodes vacuum sealed with oxygen absorbers in the absence of light at 4 °C demonstrated stability for about 3 months, the same electrodes stored at −18 °C exhibited an extended stability for about 6 months. Hence, temperatures as low as −18 °C, and even lower possess the ability to arrest THC degradation and are ideal for the long-term stability of the sensor. Additional conditions implemented including the use of different oxygen scavenger additives, THC derivatives, and pH control deemed unsuccessful in improving the stability of the electrode. Finally, modified electrodes stored under optimal conditions were interrogated with different THC samples, delivering improved calibration curves after an aging period of one week. Although further studies must be conducted to apply this lab-scale discovery to real-life roadside testing applications, the optimal storage conditions identified in this study can help regulation authorities detect the toxicity of THC rapidly, accurately, and effortlessly via the use of stable biosensors.
Data availability
The data supporting this article have been included as part of the ESI† in an Excel file.
Conflicts of interest
The authors declare that they have no known competing financial interests or personal relationships that could have appeared to influence the work reported in this article.
Acknowledgements
The authors would like to acknowledge the support of Collaborative Research and Development Grants from the Natural Sciences and Engineering Research Council of Canada, Mitacs, and eye3Concepts Inc. for funding this research.
References
- L. Cristino, T. Bisogno and V. Di Marzo, Nat. Rev. Neurol., 2019, 16, 9–29 CrossRef PubMed.
- G. D. Pearlson, M. C. Stevens and D. C. D'Souza, Front. Psychiatry, 2021, 12, 689444 CrossRef PubMed.
- R. L. Hartman, T. L. Brown, G. Milavetz, A. Spurgin, R. S. Pierce, D. A. Gorelick, G. Gaffney and M. A. Huestis, Drug Alcohol Depend., 2015, 154, 25–37 CrossRef CAS PubMed.
- R. L. Hartman and M. A. Huestis, Clin. Chem., 2013, 59, 478–492 CrossRef CAS PubMed.
- M. G. Lenné, P. M. Dietze, T. J. Triggs, S. Walmsley, B. Murphy and J. R. Redman, Accid. Anal. Prev., 2010, 42, 859–866 CrossRef PubMed.
- Ç. Kip, K. Ö. Hamaloğlu and A. Tuncel, Nanozymes, 2021, 97–113 Search PubMed.
- R. Chand, N. Mittal, S. Srinivasan and A. R. Rajabzadeh, Analyst, 2021, 146, 574–580 RSC.
- K. Amini, A. Sepehrifard, A. Valinasabpouri, J. Safruk, D. Angelone and T. de Campos Lourenco, J. Cannabis Res., 2022, 4, 1–7 CrossRef PubMed.
- E. R. Lowe, C. E. Banks and R. G. Compton, Anal. Bioanal. Chem., 2005, 383, 523–531 CrossRef CAS PubMed.
- D. Majak, J. Fan, S. Kang and M. Gupta, J. Mater. Chem. B, 2021, 9, 2107–2117 RSC.
- A. Goodwin, C. E. Banks and R. G. Compton, Electroanalysis, 2006, 18, 1063–1067 CrossRef CAS.
- H. Stevenson, A. Bacon, K. M. Joseph, W. R. W. Gwandaru, A. Bhide, D. Sankhala, V. N. Dhamu and S. Prasad, Sci. Rep., 2019, 9, 1–11 CrossRef CAS PubMed.
- G. A. Ortega, S. R. Ahmed, S. K. Tuteja, S. Srinivasan and A. R. Rajabzadeh, Talanta, 2022, 236, 122863 CrossRef CAS PubMed.
- P. Panjan, V. Virtanen and A. M. Sesay, Talanta, 2017, 170, 331–336 CrossRef CAS PubMed.
- A. G. M. Ferrari, C. W. Foster, P. J. Kelly, D. A. C. Brownson and C. E. Banks, Biosensors, 2018, 8, 53 CrossRef CAS PubMed.
- D. J. Harvey, J. Ethnopharmacol., 1990, 28, 117–128 CrossRef CAS PubMed.
- W. Jaidee, I. Siridechakorn, S. Nessopa, V. Wisuitiprot, N. Chaiwangrach, K. Ingkaninan and N. Waranuch, Cannabis Cannabinoid Res., 2022, 7, 537–547 CrossRef CAS PubMed.
- G. Dabija and F. Laurentiu, Rev. Chim., 2012, 63, 422–427 Search PubMed.
- J. Meija, G. McRae, C. O. Miles and J. E. Melanson, Anal. Bioanal. Chem., 2022, 414, 377–384 CrossRef CAS PubMed.
- S. Thumma, S. Majumdar, M. A. ElSohly, W. Gul and M. A. Repka, AAPS PharmSciTech, 2008, 9, 982–990 CrossRef CAS PubMed.
- J. W. Fairbairn, J. A. Liebmann and M. G. Rowan, J. Pharm. Pharmacol., 2011, 28, 1–7 CrossRef PubMed.
- R. N. Smith and C. G. Vaughan, J. Pharm. Pharmacol., 2011, 29, 286–290 CrossRef PubMed.
-
S. Ross and M. Elsohly, Bulletin on Narcotics XIX and L, 1997, pp. 139–147 Search PubMed.
- E. R. Garrett and J. Tsau, J. Pharm. Sci., 1974, 63, 1563–1574 CrossRef CAS PubMed.
- R. F. Turk, J. E. Manno, N. C. Jain and R. B. Forney, J. Pharm. Pharmacol., 2011, 23, 190–195 CrossRef PubMed.
- R. Mechoulam, Science, 1970, 168, 1159–1166 CrossRef CAS PubMed.
- M. Carbone, F. Castelluccio, A. Daniele, A. Sutton, A. Ligresti, V. Di Marzo and M. Gavagnin, Tetrahedron, 2010, 66, 9497–9501 CrossRef CAS.
- C. Lindholst, Aust. J. Forensic Sci., 2010, 42, 181–190 CrossRef.
- B. Zanfrognini, L. Pigani and C. Zanardi, J. Solid State Electrochem., 2020, 24, 2603–2616 CrossRef CAS.
- R. Tanasescu and C. S. Constantinescu, Expert Opin. Drug Metab. Toxicol., 2013, 9, 1219–1228 CrossRef CAS PubMed.
- B. Zanfrognini, A. Monari, G. Foca, A. Ulrici, L. Pigani and C. Zanardi, Microchem. J., 2022, 183, 108108 CrossRef CAS.
- M. A. Repka, M. A. Elsohly, M. Munjal and S. A. Ross, Drug Dev. Ind. Pharm., 2006, 32, 21–32 CrossRef CAS PubMed.
- N. A. Desrosiers, D. Lee, K. B. Scheidweiler, M. Concheiro-Guisan, D. A. Gorelick and M. A. Huestis, Anal. Bioanal. Chem., 2014, 406, 785–792 CrossRef CAS PubMed.
- M. Taschwer and M. G. Schmid, Forensic Sci. Int., 2015, 254, 167–171 CrossRef CAS PubMed.
- H. Viltres, O. F. Odio, M. C. Biesinger, G. Montiel, R. Borja and E. Reguera, ChemistrySelect, 2020, 5, 4875–4884 CrossRef CAS.
- D. Zhang, A. Chen, Y. Chen, Q. Wang, S. Li, Y. Jiang and M. Jin, J. Anal. At. Spectrom., 2021, 36, 1280–1286 RSC.
- G. Rocchitta, A. Spanu, S. Babudieri, G. Latte, G. Madeddu, G. Galleri, S. Nuvoli, P. Bagella, M. I. Demartis, V. Fiore, R. Manetti and P. A. Serra, Sensors, 2016, 16, 780 CrossRef PubMed.
- P. Bhalla and N. Singh, Eur. Phys. J. B, 2016, 89, 1–8 CrossRef CAS.
- N. Maganzini, I. Thompson, B. Wilson and H. T. Soh, Nat. Commun., 2022, 13, 1–15 Search PubMed.
- M. Renaud-Young, R. M. Mayall, V. Salehi, M. Goledzinowski, F. J. E. Comeau, J. L. MacCallum and V. I. Birss, Electrochim. Acta, 2019, 307, 351–359 CrossRef CAS.
- A. G. Cardoso, H. Viltres, G. A. Ortega, V. Phung, R. Grewal, H. Mozaffari, S. R. Ahmed, A. R. Rajabzadeh and S. Srinivasan, TrAC, Trends Anal. Chem., 2023, 160, 116965 CrossRef CAS.
|
This journal is © The Royal Society of Chemistry 2024 |
Click here to see how this site uses Cookies. View our privacy policy here.