DOI:
10.1039/D4LC00203B
(Paper)
Lab Chip, 2024,
24, 3226-3232
Surface acoustic wave digital microfluidics with surface wettability gradient
Received
5th March 2024
, Accepted 16th May 2024
First published on 17th May 2024
Abstract
This paper reports a digital microfluidic technology that combines surface wettability gradient and surface acoustic waves. The technology enables selection of the driven object, facilitating reactions among multiple droplets and improving the precision of droplet control. Octagonal patterns with a wetting gradient and orthogonally distributed interdigital transducers were created on the surface of a LiNbO3 wafer by photolithography. Leveraging the propagation characteristics of surface acoustic waves on different wetting models, the latter serve as a switch for microfluidic motion, successfully achieving selection of the driven object and demonstrating sequential reactions among multiple droplets. Also, under the excitation of standing surface acoustic waves, droplets on the wetting gradient surface move slightly in the direction of wetting gradient descent, significantly enhancing the positional accuracy of the droplets to the micrometer level. With the advantages of surface acoustic wave digital microfluidics, this technology addresses the challenges of multi-droplet digital manipulation and improved droplet positional accuracy.
Introduction
Over the past 20 years, microfluidic technology has found a myriad of applications in fields such as materials synthesis, environmental detection, and biomedical research.1–4 Unlike controlling continuous flow in pipelines, manipulating discrete droplets on surfaces offers advantages such as easy bubble removal, error correction, and seamless integration, thereby attracting widespread attention particularly for biological reactions and medical diagnostics.5,6 Also known as digital microfluidics (DMF), the manipulation of discrete droplets allows droplet size control ranging from nanoliters to microliters, and DMF has multiple benefits including minimal reagent consumption, high-throughput analysis, and compact devices.7–11 However, as applications diversify, droplet reactions involve more droplets and intermediate products, thereby necessitating more-intricate reaction sequences and so posing challenges for DMF technology.
A popular DMF technique is electrowetting-on-dielectric (EWOD), in which droplets are moved by changing their contact angles via an electric field, and this has allowed complex reactions among droplets to be achieved.12,13 However, EWOD is limited by high electric potentials that can have irreversible effects on proteins, DNA, microorganisms, and other entities.14 Currently, researchers are mitigating this by optimizing device structures15,16 and developing dielectric materials17,18 to reduce electric potentials, but despite recent reports of reducing potentials to a few volts, widespread adoption is hindered by high cost and technical immaturity. Moreover, complex reactions among droplets often result in significant volume changes, thereby posing challenges in the selection of electrode size parameters. Among various other driving methods, surface acoustic wave (SAW) DMF technology is highly efficient, recognized for its rapid response, contactless operation, low power consumption, and seamless integration capabilities.19,20 DMF technology based on SAWs has achieved the generation, transfer, splitting, and distribution of droplets.21,22 Because SAWs are elastic waves, droplets in the acoustic field are subjected to the action of acoustic streaming forces. Therefore, on solid surfaces, SAW DMF technology is used to manipulate single droplets.23,24 Moreover, constructing an array of interdigital transducers (IDTs) in a liquid medium creates a fluidic trap by exciting the transducers, successfully achieving the manipulation of multiple droplets.25 However, despite the benefits of reducing biological contamination and isolating heat in a liquid medium, this method has drawbacks such as high integration costs, complex power supply circuits, and significant power consumption.
Presented herein is a DMF technology that combines SAWs and graduated surface wettability, thereby enabling complex reactions among multiple droplets on an open surface. Two sets of orthogonally arranged IDTs and a surface with a wettability gradient were fabricated on a LiNbO3 chip using photolithography. The surface with a wettability gradient is located on the IDT delay lines and comprises an array of “rooms” with octagonal patterns, each composed of nested octagonal micro-ridges. Adjusting the microstructure parameters modifies the wetting model of a droplet on the surface and induces a wettability gradient within the rooms. By using the wetting model as a driving switch, selection of the driven object is achieved. Under the excitation of standing SAWs (SSAWs), droplets move slightly in the direction of lower wettability, thereby controlling droplet positions more precisely, and because droplets are driven on an open surface, the droplet size is flexible. This driving method offers advantages such as low integration cost, simple control, and low power consumption, and it provides a new design approach for achieving complex reactions among droplets.
Materials and methods
Working principle
To investigate the wetting states of droplets on different surfaces, we use wetting models including Young's equation, the Wenzel model, the Cassie–Baxter (CB) model, and the hybrid model. Young's equation is used to describe the wetting state of droplets on ideal surfaces. The Wenzel model describes the wetting state of droplets on rough but chemically uniform surfaces; in the Wenzel model, there are no enclosed cavities between the liquid and solid surface. The CB model describes the wetting state of droplets on smooth but chemically non-uniform surfaces; in the CB model, the liquid is suspended above cavities. The hybrid model is intermediate to the Wenzel and CB models, with partial cavities filled completely with liquid or part of each cavity filled with liquid.26
Next, we model how SAWs propagate on the wetting models.27 Being elastic waves, SAWs require the support of a medium for propagation, and we fully leverage this characteristic, combining SAWs with the wetting models. The wetting model serves as a switch for microfluidic motion, and we use a valve to describe this process. In the Wenzel model, the SAWs fully enter the droplet by propagating through the liquid within the cavities, and thus the Wenzel model represents a fully open valve where fluid can flow freely [Fig. 1(a)]. In the hybrid model, the SAWs partially enter the droplet by propagating through the liquid within some of the cavities, and thus the hybrid model represents a partially open valve that limits the fluid flow [Fig. 1(b)]. In the CB model, the SAWs propagate along the interface without entering the droplet, and thus the CB model represents a fully closed valve that isolates the fluid [Fig. 1(c)]. Therefore, by constructing different wetting models along the SAWs propagation path, selection of the driven object is achieved.
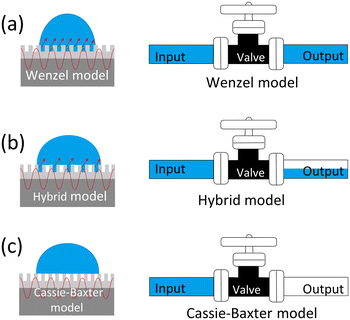 |
| Fig. 1 Wetting model as a switch for microfluidic motion: (a) Wenzel model representing a fully open valve; (b) hybrid model representing a partially open valve; (c) Cassie–Baxter (CB) model representing a fully closed valve isolating the fluid. | |
Different wetting models on the surface can be constructed by controlling the microstructure spacing. Based on the relationship that the contact angle in the Wenzel model is negatively correlated with the microstructure spacing, we construct a wetting gradient surface (WGS) by varying the spacing of grating microstructures.28 On the WGS, droplets tend to move in the direction of wetting descent. The wetting gradient (wg) is given by
|  | (1) |
where
θ is the contact angle and
s is the displacement.
To investigate the driving force of droplets on a WGS, we construct a wetting-band model; the wetting bands have lengths that are significantly greater than the size of the droplets, and the wetting angle at each position inside a band is uniform. Taking two wetting bands as an example, with the droplet's three-phase contact line forming a circular shape on the contact surface and the droplet distributed symmetrically on wetting bands 1 and 2, the wetting gradient force satisfies
| Fc = Liγ(cos θ2 − cos θ1), | (2) |
where
θ1 and
θ2 are the wetting angles of wetting bands 1 and 2,
γ is the surface tension, and
Li is the length of the intersection line between the droplet and the wetting bands [
Fig. 2(a)]. In the model, the diameter of the circular pattern enclosed by the three-phase contact lines should be greater than the width of the wetting bands.
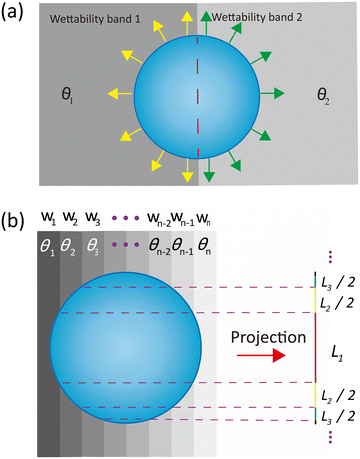 |
| Fig. 2 Wetting-band model: (a) force on a droplet in the model with two wetting bands; (b) intersection line between droplet and projection of wetting bands. | |
Next, we extend the model to n wetting bands [Fig. 2(b)]. When n is an even number, the wetting gradient force satisfies
| 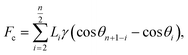 | (3) |
when
n is an odd number, the wetting gradient force satisfies
| 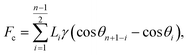 | (4) |
where
θn is the wetting angle of wetting band
n. The narrower the wetting bands, the more that a droplet can span for the same volume, resulting in a larger wetting gradient force acting on the droplet. At the same time, the volume driving the droplet becomes more flexible. However, if the wetting bands are too densely packed, it will inevitably lead to a small wetting gradient between adjacent wetting bands, preventing small droplets from obtaining a sufficiently large wetting gradient force. Therefore, when designing the width of the wetting bands, it is necessary to consider a balanced approach that accounts for the volume of the driven droplet and the driving distance.
Device design
The design of two sets of orthogonal IDTs is shown in Fig. 3(a) and (b), where the IDT width (W) is 20 mm. The IDT structure is based on the delay-line configuration, with the linewidth and gap between adjacent fingers being λ/4 = 50 μm. The SAW wavelength (λ) is 200 μm, the number of excitation electrodes (N) is 80, and the delay-line length (L1) is 22.4 mm. Because of their design, the IDTs have two excitation modes: single-ended SAWs and SSAWs.
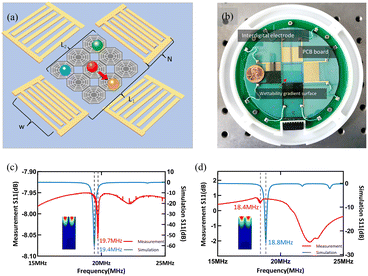 |
| Fig. 3 Surface acoustic wave (SAW) driver device design with surface gradient wettability and frequency measurements. (a) Device structure: the wetting gradient surface (WGS) is located on the delay lines of two sets of orthogonal interdigital transducers (IDTs) and is an extended third-order array of nested octagonal microstructures. (b) Top view of the device after encapsulation. (c) S11 measurement and simulation results parallel to 128° YX cut direction. The scanning frequency starts from 15 MHz and ends at 25 MHz. The insets show the simulated SAW mode at the resonant frequency of 19.4 MHz, and the operating frequency parallel to the 128° YX cut direction is 19.7 MHz. (d) S11 measurement and simulation results perpendicular to 128° YX cut direction. The scanning frequency starts from 15 MHz and ends at 25 MHz. The insets show the simulated SAW mode at the resonant frequency of 18.8 MHz, and the operating frequency perpendicular to the 128° YX cut direction is 18.4 MHz. | |
To estimate the SAW mode, two-dimensional finite-element simulations of the IDT vibration modes were established using COMSOL Multiphysics. The two-dimensional periodic model consists of a lithium niobate (LiNbO3) substrate with periodic structural boundary conditions on both sides of the simulation cell and fixed constraints on the bottom edge. The bottom edge of the simulation cell is set as a low-reflection boundary to reduce substrate reflection. The IDT electrodes are subjected to 1 W power and ground, respectively. Fig. 3(c) and (d) show the simulation results for the SAW mode at resonant frequency. The S11 parameter is measured using a vector network analyzer (E5063A KEYSIGHT) to determine the operating frequency of the device. The S11 simulation result of resonant frequency matched well with the S11 measurement result of operating frequency.
To fabricate the IDTs, we used a 4-inch single-side polished 128° YX-cut LiNbO3 wafer with a thickness of 0.5 mm. First, IDTs were patterned on the polished side by photolithography. Next, Au and Cr thin films were deposited on the surface via electron beam evaporation. Finally, the substrate was soaked in acetone for photoresist lift-off; the photoresist was dissolved, resulting in the interdigitated electrode structure consistent with the pattern.
The WGS is located on the delay lines of two sets of orthogonal IDTs [Fig. 3(a)]. The WGS is an extended third-order array of nested octagonal microstructures [Fig. 4(a)], with each side of the array measuring 18.6 mm. We define the nested octagonal microstructures as “rooms”: each room is composed of seven octagonal microstructures, with increasing spacing between the nested octagons from outer to inner layers [Fig. 4(b)]. The outer layer of the room conforms to the CB model, while the inner layer conforms to the Wenzel model [Fig. 4(c)]. To achieve complex reactions among droplets, we design the driving path, connecting different rooms using a fusiform microstructure based on the Wenzel model [Fig. 4(d)].
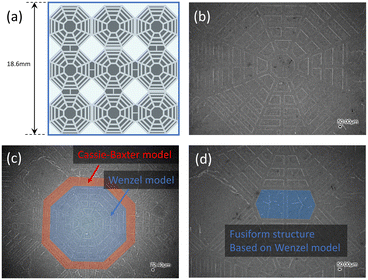 |
| Fig. 4 Wetting gradient surface (WGS): (a) third-order array of nested octagonal microstructures; (b) nested octagonal microstructures; (c) distribution of wetting models in “room” (the outer layer conforms to the CB model, and the inner layer conforms to the Wenzel model); (d) fusiform structure connecting different rooms. | |
To fabricate the WGS, we spin-coat a layer of photoresist on the polished side of LiNbO3. Next, we perform photolithography on the photoresist using a mask consistent with the desired pattern. Finally, the unexposed photoresist is removed using development technology. To enhance the hydrophobic properties of the surface, we use gas molecular modification technology to deposit a hydrophobic layer of SiO2 on the surface of the microstructures.
Droplet manipulation test setup
The signal generator (DG4202, RIGOL) outputs sine-wave signals at frequencies of 18.4 MHz and 19.7 MHz, which are amplified by a power amplifier (UWBPA20M1G-100 W, ULTRA WIDEBAND TECHNOLOGIES). The signals are then distributed to the target IDT by a self-developed control module, causing excitation of the target IDT. For enhanced visibility, dye is added to deionized water, and the water is transferred to the WGS using a pipette (N3-2-20, NOKE). The movement of the droplets is recorded using a smartphone with a macro lens.
Results and discussion
Selection of driven objects
Using a pipette, red and green 5 μL deionized water droplets are transferred to position 1 on the WGS [Fig. 5(a) and (b)]. The IDT is excited, generating SAWs propagating to the left, whereupon the red droplet moves continuously to the left while the green droplet hardly moves [Fig. 5(c)–(e)]. When the IDT excitation is stopped, both the red and green droplets are at position 2 [Fig. 5(f)]. Hindered by the CB wetting model, the green droplet does not move out of the octagonal room. By using the wetting model as a driving switch, selection of the driven object is achieved.
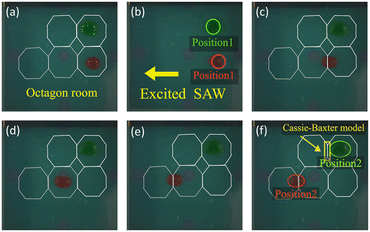 |
| Fig. 5 Selective droplet manipulation by wetting model: (a) octagonal room on the surface; (b) droplet initial position; (c) red droplet moves out of the first room; (d) red droplet moves into the second room; (e) red droplet moves out of the second room; (f) droplet end position and CB model in the direction of droplet advancement. The CB model prevents the green droplet from moving. | |
Intricate reaction among multiple droplets
Next, we demonstrate a sequential reaction among three droplets. Using a pipette, red, blue, and green droplets are transferred to the WGS. When the left IDT is excited, the blue droplet moves continuously to the right, while the red and green droplets move to the right for a short distance before stopping in the room [Fig. 6(a)–(c)]. Exciting the bottom IDT, the green and blue droplets move upward, with the green droplet moving faster than the red droplet [Fig. 6(d)]. Consequently, the green droplet catches up with the red droplet, leading to droplet mixing [Fig. 6(e) and (f)]. The blue droplet moves to the edge of the octagonal room and stops moving upward. By exciting the top IDT, the red and blue droplets move downward into the middle octagonal room [Fig. 6(g)]. Exciting the left IDT again, the mixed droplet moves toward the blue droplet until both droplets mix [Fig. 6(h) and (i)].
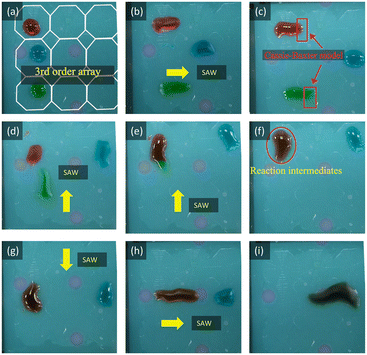 |
| Fig. 6 Intricate reaction among three droplets: (a) distribution of droplets in room; (b) excitation of SAWs to drive droplets to move to right; (c) CB model prevents red and green droplets from moving; (d) excitation of SAWs to drive droplets to move upward; (e) mixing of red and green droplets; (f) generation of reaction intermediates; (g) adjustment of droplet positions to ensure that path between mixed droplet and blue droplet is Wenzel model; (h) excitation of SAWs to drive the mixed droplet to move to the blue droplet; (i) droplet mixing to produce final reaction product. | |
A microfluidic chip of third order can effectively manipulate four droplets concurrently and generate intermediate products for two reactions. To achieve more intricate droplet reactions, we can design arrays with higher order. By using the wetting model as a driving switch, the limitations of elastic wave-driven microfluidics are overcome, thereby allowing for intricate reactions among multiple droplets.
Next, we investigate the principles of driving droplets on a WGS. Because of the rigid coupling between the WGS and the LiNbO3 surface, SAWs propagate in the form of Stoneley waves at the interface. In the Wenzel model when the SAWs propagate to the droplet–surface contact point, they enter the droplet as leaky waves by propagating through the liquid within the cavities [Fig. 7(a)]. The driving force on the droplet is the acoustic streaming force,29 which satisfies
|  | (5) |
where
A is the wave amplitude,
ω is the angular velocity, and
kI (
kL =
kR +
jkI) represents the SAW energy dissipation in the liquid medium, where
kL is the leaky SAW wavenumber, which is a complex number whose real part
kR is the SAW wavenumber and whose imaginary part is
kI. Furthermore,
ρ is the fluid density, and
α =
jα1, where
α (
α2 = 1−[
vL/
vW]
2) is the attenuation constant with
vL the leaky SAW velocity and
vW the sound velocity in the liquid.
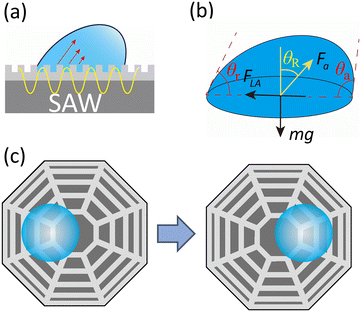 |
| Fig. 7 Schematics of principles behind using SAWs to drive a droplet: (a) SAWs enter droplet in the form of leaky waves on WGS; (b) force diagram of a droplet under single-ended SAW excitation; (c) acoustic streaming force drives droplet movement on WGS. | |
The direction of the acoustic streaming force follows the Rayleigh angle θR, which satisfies
|  | (6) |
where
vR is the sound velocity in the flexible substrate.
The droplet undergoes lateral adhesion on the solid surface because of surface tension and hysteresis of the contact angle, and the force required to overcome this is the pinning force. If the footprint of the droplet is assumed to be circular, then the pinning force satisfies30
| FLA = γD(cos θr − cos θa), | (7) |
where
D is the diameter of the three-phase contact line,
θa is the advancing contact angle, and
θr is the receding contact angle. As the SAW streaming force increases, the droplet deforms to generate a greater pinning force until it reaches a critical value, and the minimum acoustic streaming force driving droplet motion satisfies
| Fa sin θR − FLA = 0. | (8) |
Once the droplet starts moving under SAW driving, the viscous resistance force becomes significant, and this satisfies
| 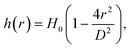 | (9) |
| 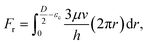 | (10) |
where
εc is the cutoff length to prevent singularity at the contact line,
μ is the liquid viscosity,
v is the droplet velocity,
h(
r) is the height of the droplet at a certain position dependent on the radial coordinate
r,
H0 is the characteristic central height, and
D is the characteristic base diameter.
In the horizontal direction, the force balance equation is [Fig. 7(b)]
| 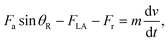 | (11) |
where
m is the droplet mass. Different wetting models are distributed along the direction of SAW propagation. In the Wenzel model, SAWs drive droplet motion by propagating through the liquid within the cavities, and the direction of droplet motion is controlled by exciting different orientations of IDTs. When the droplet is on the CB model or SAW excitation is stopped, the droplet stops moving under the influence of the viscous resistance force [
Fig. 7(c)].
Movement of droplets under SSAW excitation
Despite having constructed the WGS, the wetting gradient force is typically too small to drive droplets autonomously. Therefore, to solve this problem, we propose a method in which droplets are kept in an unstable state via SSAW excitation.
The ball-in-valley model is chosen to understand the instability of droplets [Fig. 8], which consists of a valley, a peak, and a ball at each equilibrium position. When the ball is at the bottom of the valley as is in position A Fig. 8(a), a slight disturbance moves it to position B, whereupon the ball rolls back to position A, showing resistance to minor disturbances and indicating a stable state. However, when the ball is at the top of the peak as is in position Fig. 8(b), a slight disturbance moves it to position B, whereupon the ball continues to roll downhill, indicating an unstable state.
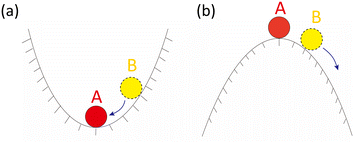 |
| Fig. 8 Ball-and-valley model: (a) the ball in the valley resembles a stable state; (b) the ball at the peak resembles an unstable state. | |
We use a set of delay-line IDTs for SSAW coupling. The droplet is in an unstable state under SSAW excitation [Fig. 9(a)]. At this point, the wetting gradient force acts as a small disturbance, and the droplet on the WGS moves in the direction of wetting gradient descent. In the horizontal direction, the force balance equation is [Fig. 9(b)]
|  | (12) |
The droplet is positioned in an octagonal room, where the red arrow represents the wetting gradient force acting on the droplet [
Fig. 9(c)]. SAWs are excited on both sides of the droplet, coupling to form SSAWs on the interface. The droplet vibrates up and down and the three-phase contact line shrinks periodically under SSAW excitation [
Fig. 9(a)]. Guided by the wetting gradient force, the droplet moves gradually toward the center of the octagonal room [
Fig. 9(c)]. Combining SAWs with surface gradient wettability has enabled the precise movement of droplets toward the center of the octagonal room in an open DMF device, and this innovative approach shows the potential for precise control of droplets.
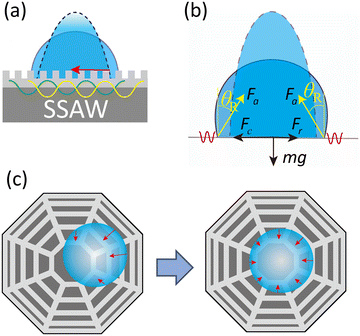 |
| Fig. 9 Schematics of principles behind using SSAWs to drive a droplet: (a) droplet vibrates up and down under SSAW excitation; (b) force diagram of a droplet under SSAW excitation; (c) wetting gradient forces drive droplet movement on WGS. | |
Using a pipette, the red droplet is transferred to an octagonal room on the WGS [Fig. 10(a) and (b)], and IDT excitation is used to couple SAWs into SSAWs [Fig. 10(c)]. The droplet's three-phase contact line shrinks and expands periodically, and the droplet moves slightly in the direction of wetting gradient descent [Fig. 10(c)–(f)]. When the IDT excitation is stopped, the red droplet's displacement (d) is ca. 1 mm [Fig. 10(f)].
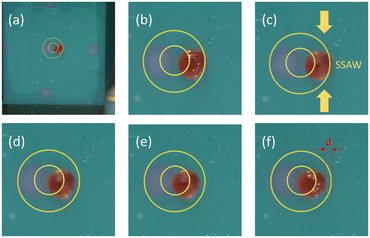 |
| Fig. 10 Movement of a droplet on WGS under SSAW excitation: (a) droplet on WGS; (b) magnified view of a droplet on WGS; (c) SAWs coupled to SSAWs; (d) and (e) droplet moves in direction of wetting gradient descent; (f) droplet on WGS has moved slightly. | |
Under SSAW excitation, the droplet on the surface moves slightly along the wetting gradient, enhancing the precision of the droplet's position. Also, the driving voltage threshold for SSAW excitation is typically lower than that for single-ended SAW excitation, which is advantageous for reducing the power consumption of the device.
Both the wetting gradient force and the pinning force are essentially influenced by surface tension, with the distinction being that the wetting gradient force serves as the driving force for droplet movement while the pinning force acts as resistance to droplet motion. The flexible application of surface tension is a key factor in achieving droplet movement under different SAW excitation modes.
Conclusions
Proposed herein was combining a WGS and SAWs to achieve digital manipulation of multiple droplets. Leveraging the propagation characteristics of SAWs on wetting models, we used the latter as a switch for microfluidic motion, enabling the selection of the driven object. We successfully demonstrated sequential reactions among three droplets. Also, we established a force model for the droplet under single-ended SAW excitation and analyzed the principles of driving droplets on the WGS. We used a ball-and-valley model to analyze the unstable state of the droplet. By using delay-line transducers coupled to generate SSAWs, droplets were placed in an unstable state. Under SSAW excitation, droplets on the WGS moved slightly along the wetting gradient, achieving precise control over droplet positions.
Conflicts of interest
There are no conflicts to declare.
Acknowledgements
This work was partially supported by the Priority Academic Program Development of Jiangsu Higher Education Institutions.
References
- J. Li and C. C. Kim, Lab Chip, 2020, 20, 1705–1712 RSC.
- H. Cheng, H. Liu, W. Li and M. Li, Electrophoresis, 2021, 42, 2329–2346 CrossRef CAS PubMed.
- M. J. Jebrail and A. R. Wheeler, Curr. Opin. Chem. Biol., 2010, 14, 574–581 CrossRef CAS PubMed.
- A. B. Theberge, F. Courtois, Y. Schaerli, M. Fischlechner, C. Abell, F. Hollfelder and W. T. S. Huck, Angew. Chem., Int. Ed., 2010, 49, 5846–5868 CrossRef CAS PubMed.
- H. Song, D. L. Chen and R. F. Ismagilov, Angew. Chem., Int. Ed., 2006, 45, 7336–7356 CrossRef CAS PubMed.
- L. Qi, Y. Niu, C. Ruck and Y. Zhao, Lab Chip, 2019, 19, 223–232 RSC.
- S. Y. Teh, R. Lin, L. H. Hung and A. P. Lee, Lab Chip, 2008, 8, 198–220 RSC.
- H. Yang, V. N. Luk, M. Abelgawad, I. Barbulovic-Nad and A. R. Wheeler, Anal. Chem., 2009, 81, 1061–1067 CrossRef CAS PubMed.
- X. C. I. Solvas and A. DeMello, Chem. Commun., 2011, 47, 1936–1942 RSC.
- H. Wang, L. Chen and L. Sun, Front. Mech. Eng., 2017, 12, 510–525 CrossRef.
- E. Samiei, M. Tabrizian and M. Hoorfar, Lab Chip, 2016, 16, 2376–2396 RSC.
- S. K. Cho, H. Moon and C. J. Kim, J. Microelectromech. Syst., 2003, 12, 70–80 CrossRef.
- Y. Zhang and Y. Liu, Sens. Diagn., 2022, 1, 648–672 RSC.
- X. Min and W. S. Kim, Microfluid. Nanofluid., 2019, 23, 127 CrossRef.
- Y. Li, R. J. Baker and D. Raad, Sens. Actuators, B, 2016, 229, 63–74 CrossRef CAS.
- P. Datta, A. Dutta, R. Majumder, A. Chakraborty, D. Dhal and R. K. Pal, Procedia Comput. Sci., 2016, 93, 183–190 CrossRef.
- M. Mibus and G. Zangari, ACS Appl. Mater. Interfaces, 2017, 9, 42278–42286 CrossRef CAS PubMed.
- M. Samad, A. Z. Kouzani, M. F. Hossain, M. Mohammed and M. Alam, Microsyst. Technol., 2017, 23, 3005–3013 CrossRef CAS.
- M. Sesen, C. Devendran, S. Malikides, T. Alan and A. Neild, Lab Chip, 2017, 17, 438–447 RSC.
- Y. Zhang and N. T. Nguyen, Lab Chip, 2017, 17, 994–1008 RSC.
- C. J. Strobl, Z. von Guttenberg and A. Wixforth, IEEE Trans. Sonics Ultrason., 2004, 51, 1432–1436 Search PubMed.
- A. L. Zhang, Z. Q. Wu and X. H. Xia, Talanta, 2011, 84, 293–297 CrossRef CAS PubMed.
- A. Renaudin, J.-P. Sozanski, B. Verbeke, V. Zhang, P. Tabourier and C. Druon, Sens. Actuators, B, 2009, 138, 374–382 CrossRef CAS.
- R. Tao, G. McHale, J. Reboud, J. M. Cooper, H. Torun, J. Luo, J. Luo, X. Yang, J. Zhou, P. Canyelles-Pericas, Q. Wu and Y. Fu, Nano Lett., 2020, 20, 3263–3270 CrossRef CAS PubMed.
- S. P. Zhang, J. Lata, C. Chen, J. Mai, F. Guo, Z. Tian, L. Ren, Z. Mao, P. H. Huang, P. Li, S. Yang and T. J. Huang, Nat. Commun., 2018, 9, 2928 CrossRef PubMed.
- S. Min, S. Li, Z. Zhu, W. Li, X. Tang, C. Liang, L. Wang, X. Cheng and W. D. Li, Microsyst. Nanoeng., 2020, 6, 106 CrossRef CAS PubMed.
- M. H. Biroun, L. Haworth, P. Agrawal, B. Orme, G. McHale, H. Torun, M. Rahmati and Y. Fu, ACS Appl. Mater. Interfaces, 2021, 13, 46076–46087 CrossRef CAS PubMed.
- C. Sun, X. W. Zhao, Y. H. Han and Z. Z. Gu, Thin Solid Films, 2008, 516, 4059–4063 CrossRef CAS.
- M. Alghane, B. Chen, Y. Q. Fu, Y. Li, J. Luo and A. Walton, J. Micromech. Microeng., 2010, 21, 015005 CrossRef.
- G. Ahmed, M. Sellier, M. Jermy and M. Taylor, Eur. J. Mech. B Fluids, 2014, 48, 218–230 CrossRef.
|
This journal is © The Royal Society of Chemistry 2024 |