DOI:
10.1039/D4GC00486H
(Paper)
Green Chem., 2024,
26, 5879-5889
A solvent-free mechanochemical electrophilic C–H thiocyanation of indoles and imidazo[1,2-a]pyridines using a cost-effective combination of N-chlorosuccinimide-NaSCN and tandem C–C and C–S bond formation†
Received
27th January 2024
, Accepted 27th March 2024
First published on 3rd April 2024
Abstract
Herein, we report a solvent-free and sustainable methodology for the electrophilic C–H thiocyanation of indoles and imidazo[1,2-a]pyridines in a mixer-mill. A combination of commercially available and cheaper precursors N-chlorosuccinimide and NaSCN was used under milling conditions for the in situ generation of N-thiocyanatosuccinimide (NTS) which facilitates the C-3 selective thiocyanation reaction on indoles and imidazo[1,2-a]pyridines. A series of thiocyanated products containing electron-rich and electron-deficient rings were synthesized in silica gel as the solid reaction media and good to excellent yields were obtained. The scalability of the reaction was validated with selected substrates at the gram scale. Moreover, we explored mechanochemical tandem C–C and C–S bond formation reactions by double C–H activation, leading to easy access to C-2 aryl and C-3 thiocyanato compounds from unsubstituted indoles. In addition, mechanochemical conversion of the –SCN precursors into –SCF3 and 5-substituted sulfenyl tetrazole was also demonstrated in the current methodology.
Introduction
Organothiocyanates represent an intriguing class of compounds within the realm of organic chemistry and have garnered significant attention in both academic research and industrial applications due to their distinctive chemical properties and profound bioactivities.1,2 Furthermore, thiocyanates serve as versatile synthetic precursors for generating a diverse range of sulfur-containing compounds such as thiocarbamates, thioethers, thiols, thioesters, disulfides, etc. (Fig. 1A).3–7 From their roles in drug discovery and medicinal chemistry to their utility in materials science8 and organocatalysis,9 thiocyanated heterocyclic scaffolds exemplify the intersection of heterocyclic chemistry and sulfur-containing functional groups, making them an interesting and valuable subject for synthetic organic chemists. Notably, nitrogen-containing heterocycles with –SCN or other sulfur functionalities are known to show anti-cancer, anti-HIV, and anti-fungal activities, etc. (Fig. 1B).10–15 Interestingly, indoles16,17 and imidazo[1,2-a]pyridines18,19 are important N-containing heterocyclic compounds and are ubiquitous among many natural products, pharmaceuticals, and biologically active molecules. In the past, the synthesis of organic thiocyanates has relied on thiocyanate metal salts,20,21 and a number of thiocyanation reactions primarily involved nucleophilic22–24 or radical pathways.25–28 Nevertheless, there have been significant interest and fast progress in electrophilic thiocyanation reagents in recent years due to their higher reactivity.29–32 Emerging as an attractive and alternative category of reagents, electrophilic thiocyanating agents like N-thiocyanatosuccinimide (NTS),29N-thiocyanatophthalimide (NTP),30N-thiocyanatosaccharin (NTSc),31 and N-thiocyanatodibenzenesulfonimide (NTBS)32 have significantly advanced the field of direct thiocyanation reactions. The design of NTS drew inspiration from commercially available reagents, and the method was first performed by Still's research group.33 Later Chen's group successfully synthesized NTSc31 and NTBS32 which emerged as versatile thiocyanating agents for several chemical transformations. Notably, visible-light mediated green protocols for thiocyanation are available.25–28 On the other hand, a number of metal-catalyzed thiocyanation reactions have also been reported.34–36 For example, Wu and co-workers reported Cu-catalyzed oxidative thiocyanation reactions on aromatics and heterocycles.34 Palladium-catalyzed synthesis of 3-thiocyanatoindoles assisted by NBS was reported by Hu et al.35
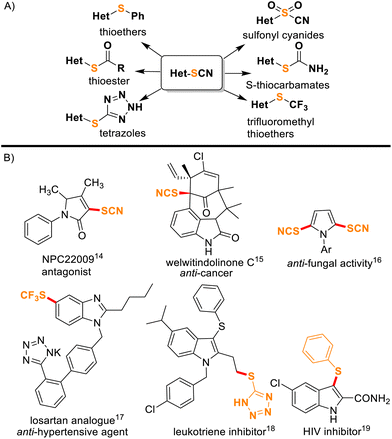 |
| Fig. 1 (A) Thiocyanates as synthetic precursors of different functionalities. (B) Some typical examples of thiocyanate or other sulfur functionality containing bioactive compounds. | |
The concept of metal-free C–H functionalization stands at the forefront of organic chemistry, introducing a paradigm shift in the manipulation and derivatization of organic molecules.37 This approach facilitates the direct activation and modification of carbon–hydrogen (C–H) bonds, without relying on the traditional use of transition metal catalysts, which have long been the primary driving force behind C–H activation. The advent of metal-free C–H functionalization has ushered in a new era in synthetic chemistry, presenting environment-friendly, sustainable, and economically feasible routes for crafting intricate organic compounds.38 The functionalization of the C–H bond introduces a sulfur atom into the heterocyclic framework, opening up new avenues for organic reactions and enhancing their potential applications. However, only limited literature is available on the metal-free thiocyanation of indoles and imidazo[1,2-a]pyridines (Scheme 1).25,26,31
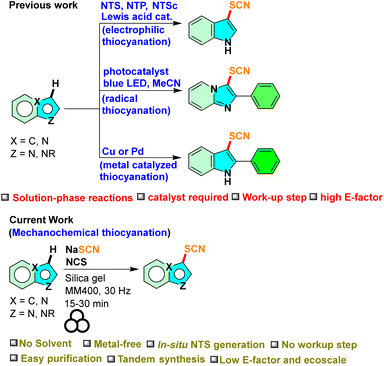 |
| Scheme 1 Available synthetic routes to thiocynation of indoles and imidazo[1,2-a]pyridines. | |
The demand for cleaner, safer, and cost-effective chemical transformations in the pharmaceutical and industrial sectors is what primarily motivated the rediscovery of mechanochemistry.39–41 Without the necessity for the use of bulk solvents, mechanochemistry achieves chemical changes through shear frictional force via milling or grinding.40,41 These include various organic transformations,42 inorganic materials,43 pharmaceutical chemistry,44 synthesis of dyes and fluorophores,45etc. Solid-state mechanochemistry offers a scope for tandem reactions.46 With our interest in mechanochemical organosynthesis,46–48 herein, we report an efficient mechanochemical method for the thiocyanation of indoles and imidazo[1,2-a]pyridines via metal-free C–H functionalization through the in situ generation of NTS from NaSCN and N-chlorosuccinimide (NCS). Furthermore, tandem C-2 arylation and C-3 thiocyanation reactions on indoles were carried out under mechanochemical conditions.
Results and discussion
Optimization of the reaction conditions
At the outset, we started our investigation of mechanochemical thiocyanation using 1.5 equiv. N-thiocyanatosuccinimide (NTS) and N-methyl indole (1a) as our model substrate, and silica gel as the grinding matrix in a 5 mL stainless steel (SS) jar with one 10 mm ball at a certain frequency in a RETSCH mixer-mill MM400. The monitoring of the reaction after an interval of 10 min through TLC showed the formation of 2a. The complete conversion took 30 min at a frequency of 25 Hz yielding 87% of 2a (entry 1, Table 1). Next, we tried to synthesize NTS in situ by ball milling carrying out a reaction with NaSCN and N-chlorosuccinimide (NCS) in the silica matrix at 25 Hz for 10 min. The product was obtained by extraction with chloroform and characterized with NMR as NTS. The thiocyanation reaction progresses to completion in 30 min with the in situ generation of NTS when the model substrate was allowed to react (entry 2, Table 1). 13C NMR spectral data showed a characteristic peak near δ 110 ppm, and the disappearance of the C-3 proton peak near δ 6.5 ppm in 1H NMR confirmed the incorporation of the C-3 -SCN group to 1a. In the absence of any milling auxiliary, the model reaction formed a pasty mass and did not proceed much even after 120 min of milling indicating a suitable auxiliary is required for smooth milling of the reaction mixture (entry 3, Table 1). We then pursued our investigations to further optimize the reaction conditions varying the stoichiometry of the –SCN source, and the additive/oxidant used at a frequency of 25 Hz for 30 min. By reducing the stoichiometry of NaSCN and NCS, a gradual improvement in the overall yield was obtained (entries 4–6, Table 1) and it is evident from entry 6 that the reaction yields the maximum product (2a) when 1.1 equiv. of NaSCN and 1 equiv. of NCS is used. When we altered the frequency of the reaction to 30 Hz, the reaction was completed in just 15 min, affording 2a in an excellent yield of 95% (entry 7, Table 1). As an alternative to NCS, reagents like NBS and I2 were attempted (entries 8 and 9, Table 1). A similar yield of 2a (91%) was observed for NBS (entry 8, Table 1), whereas, a low conversion of 2a (31%) was observed in the case of I2 with 56% recovery of the starting material 1a (entry 9, Table 1). In the next attempt, 1a was allowed to react with NaSCN without any oxidant keeping all other conditions the same (entry 10, Table 1). No product formation was observed indicating that nucleophilic thiocyanation does not prevail under these mechanochemical conditions. Later, we used NH4SCN and KSCN as SCN sources to check the feasibility of the model reaction which offered relatively lower yields (entries 11 and 12, Table 1). Next, different milling auxiliaries such as neutral, basic, and acidic alumina were screened (entries 13–15, Table 1) and it was observed that with neutral alumina, the reaction yields better as compared to acidic and basic alumina and 2a was obtained in reasonably good yield (entry 13, Table 1). In the end, a liquid grinding media in the form of PEG-400 was attempted, and a good yield of 82% was obtained (entry 16, Table 1). Subsequently, optimization of the milling parameters was carried out by taking the model substrate, 1a (1.0 equiv.), NCS (1.0 equiv.), NaSCN (1.1 equiv.) and silica gel (60–120 mesh, 300 mg) in 5 mL jars and the material of milling jars, milling frequency, and the size of the milling balls were varied (Table S1, ESI†). It was observed that the milling jar material hardly affected the course of the reaction (entries 2–4, Tables S1, ESI†), however, the SS jar was identified for further studies considering less cost of the material. Notably, a ball size of 10 mm diameter and a frequency of 30 Hz is ideal for faster reaction and higher yields (Table S1, ESI†). The optimization studies allowed to achieve the best thiocyanation conditions and subsequent reactions were carried out using a combination of NCS (1.0 equiv.) and NaSCN (1.1 equiv.) in silica matrix (300 mg per mmol of 1) at 30 Hz frequency. To gain further insight into the mechanochemical thiocyanation reaction, solid-state time-scale IR spectroscopy was conducted on the model substrate (1a). IR data of NaSCN, NCS, and the reaction mixture were plotted (Fig. S1, ESI†). The samples from the reaction mixture were collected after an interval of 5 min of milling, ranging from 0 to 15 min (shown in different colours). The study showed the formation of a new peak at 2148 cm−1 and the disappearance of the peak of NaSCN at 2063 cm−1 just after 5 min of milling (Fig. S1a, ESI†). Thus, the conversion of NCS and NaSCN to NTS occurs in first few minutes of the reaction. As the reaction progressed, the peak of –SCN at 2148 cm−1 from product 2a gradually became stronger. Thus, the time-scale IR spectroscopy indicates the formation of 2a in solid-state by mixer-milling.
Table 1 Optimization of mechanochemical C–H thiocyanation of indolesa
Entry |
–SCN source (equiv.) |
Milling auxiliary |
Additive/oxidant (equiv.) |
Frequency (Hz) |
Time (min) |
Isolated yieldb (%) |
1a recovered (%) |
The reactions were carried out in a RETSCH mixer-mill MM400 instrument with one 10 mm SS ball in a 5 mL SS jar containing 300 mg of silica gel (60–120 mesh) as the milling auxiliary to optimize the reaction conditions. N-Methyl indole (1a) is considered as the model substrate.
All yields reported here are isolated yields. n.r.: no reaction.
|
1. |
NTS (1.5) |
Silica gel |
— |
25 |
30 |
87 |
— |
2. |
NaSCN (2.0) |
Silica gel |
NCS (1.5) |
25 |
30 |
72 |
— |
3. |
NaSCN (2.0) |
Neat |
NCS (1.5) |
25 |
120 |
5 |
85 |
4. |
NaSCN (1.5) |
Silica gel |
NCS (1.2) |
25 |
30 |
78 |
— |
5. |
NaSCN (1.2) |
Silica gel |
NCS (1.0) |
25 |
30 |
83 |
— |
6. |
NaSCN (1.1) |
Silica gel |
NCS (1.0) |
25 |
30 |
89 |
— |
7.
|
NaSCN (1.1)
|
Silica gel |
NCS (1.0)
|
30
|
15
|
95
|
—
|
8. |
NaSCN (1.1) |
Silica gel |
NBS (1.0) |
30 |
15 |
91 |
— |
9. |
NaSCN (1.1) |
Silica gel |
I2 (1.0) |
30 |
15 |
31 |
56 |
10. |
NaSCN (1.1) |
Silica gel |
— |
30 |
30 |
n.r. |
88 |
11. |
NH4SCN (1.1) |
Silica gel |
NCS (1.0) |
30 |
15 |
84 |
— |
12. |
KSCN (1.1) |
Silica gel |
NCS (1.0) |
30 |
15 |
88 |
— |
13. |
NaSCN (1.1) |
Alumina (neutral) |
NCS (1.0) |
30 |
15 |
71 |
— |
14. |
NaSCN (1.1) |
Alumina (basic) |
NCS (1.0) |
30 |
15 |
26 |
62 |
15. |
NaSCN (1.1) |
Alumina (acidic) |
NCS (1.0) |
30 |
15 |
31 |
54 |
16. |
NaSCN (1.1) |
PEG-400 |
NCS (1.0) |
30 |
15 |
82 |
— |
|
Substrate scope for the mechanochemical C–H thiocyanation reaction on indoles (1)
With the optimized conditions in hand, a series of N-protected indoles such as N-Me, N-Bn, and N-Boc indoles and unprotected indoles (N–H) with different substituents in the aromatic carbocycle were studied to explore the scope of the mechanochemical thiocyanation reaction (Table 2). The reactions were carried out in a 5 mL stainless steel (SS) jar with 1 mmol 1, 1.1 mmol NaSCN, 1 mmol NCS, and 300 mg of silica gel at a frequency of 30 Hz. The progress of the reaction was monitored by TLC, and it was found that the reactions were completed in a very short period of time (15 min) in each case indicating effortless thiocyanation in the indole ring. As shown in Fig. S2 of the ESI† with the model substrate 1a, the reaction mixture in the silica matrix forms a powdery slurry after milling for a few minutes and the reaction proceeded smoothly in the solid media. After completion of the reaction, the solid slurry was directly charged to the column for purification, and isolated yields were reported (Table 2). All the synthesized compounds were characterized by 1H, 13C, HRMS, and IR analysis which were in good agreement with the reported C-3 thiocyanoindoles.26,29,31 Therefore, non-directed, exclusive C-3 thiocyanation occurred under mechanochemical conditions as well.26 Notably, no significant electronic effect was observed in terms of yields of 2 by varying the protecting groups at the N-atom of indole as substrates having electron-donating (such as N-Me and N-Bn) and electron-withdrawing (such as N-Boc) groups afforded the -SCN derivatives in excellent yields (entries 2a, 2e, 2f and 2l, Table 2).
Table 2 Substrate scope for the mechanochemical thiocyanation reactions of indolesa,b
In each case, 1 mmol of 1, 1.1 mmol NaSCN, 1 mmol NCS, and 300 mg of silica gel were milled together in a 5 mL SS jar with one 10 mm SS ball in a RETSCH mixer-mill MM400 instrument at 30 Hz frequency for 15 min.
All yields reported here are isolated yields of products 2 after column chromatography.
|
|
Furthermore, several entries with 5-substituted indoles having both electron-withdrawing and electron-donating groups in the fused benzene ring revealed a negligible substituent effect in terms of reaction kinetics and yields, as in several cases high to excellent yields were obtained within 15 min (entries 2b–d and 2g–2j, Table 2). However, the yield of the thiocyanated product marginally dropped in the case of a strong EWG (–NO2) in the ring (entry 2k, Table 2). In our next attempt, we tried a series of reactions with 2-aryl indoles having variable substituents at different positions of the aromatic ring (entries 2n–2x, Table 2). It was observed that N-methyl and N-benzyl indoles with bulky aryl substituents at the C-2 position, neither affected the reaction kinetics nor the yield under identical mechanochemical conditions, and high to excellent yields ranging from 80–91% were obtained (entries 2n–2x, Table 2); once again hardly any substituent effect was observed from the para-position of the C2-aryl group (entries 2n–2s and 2v–2x, Table 2). A couple of entries with a representative methyl substituent at ortho- and meta-positions successfully afforded the desired thiocyanated products in over 80% yields suggesting that the variation of position of the substituents in the ring hardly affects the course of the reactions (entries 2t and 2u, Table 2). In one attempt, we tried the thiocyanation reaction on an indole substrate having the electron-withdrawing –COOH group at the C-2 position (entry 2aa, Table 2). A good yield of the corresponding product (76%) indicated the versatility of the mechanochemical protocol (entry 2aa, Table 2). The methodology worked even when the C-3 position of indole is blocked and in that case, the C-2 thiocyanated product was obtained in an excellent yield (entry 2ab, Table 2). In the next few attempts, the positions of substitution at the fused benzene ring of indole were varied with groups such as 4-CN, 6-Br, 6-COOMe, and 7-Me and the reactions afforded high yields of products again indicating a nominal substituent effect on the C-3 thiocyanation of indoles (entries 2ac–2af, Table 2). Interestingly, a couple of attempts of thiocyanation on other heterocycles such as unsubstituted 1H-pyrrolo[2,3-b]pyridine or carbazole seamlessly afforded the desired products in high yields indicating the versatility of the method (entries 2ag and 2ah, Table 2). Thus, a broad substrate scope was observed for the present protocol.
Substrate scope for the mechanochemical C–H thiocyanation reaction on imidazo[1,2-a]pyridines (3)
To further broaden the scope of this mechanochemical thiocyanation, we conducted thiocyanation reactions on several 2-aryl substituted imidazo[1,2-a]pyridines under identical mechanochemical conditions. Thus, the reactions were carried out in a 5 mL stainless steel jar with 0.5 mmol 3, 0.55 mmol NaSCN, 0.5 mmol NCS, and 200 mg of silica gel at a frequency of 30 Hz. The reactions were monitored by TLC and were found to be completed within 30 min. To our delight, the reaction afforded good to high yields of 3-thiocyanated imidazo[1,2-a]pyridines, tolerating a large variety of functional groups in the ring (Table 3). The spectral characterization of the thiocyanato imidazo[1,2-a]pyridine was conducted by 1H, 13C, IR, and HRMS analysis. The 1H and 13C spectra were in accordance with the reported data.21,25 Again, the 13C NMR spectrum showed a peak near δ 91–106 ppm confirming the incorporation of -SCN into the imidazo[1,2-a]pyridine scaffold. We checked the scope of thiocyanation reactions on 2-substituted phenyl derivatives having the EDG as well as the EWG attached to the 4-position of the phenyl ring (entries 4a–4g, Table 3). On changing the electronic character in the 2-substituted aryl group, no prominent substituent effect was observed on the yields of 4. Notably, in this case, even strong electron-withdrawing substituents (such as CN and F) showed higher yields ranging from 89–91% (entry 4e–4h, Table 3). Next, a meta-OMe group on the 2-aryl ring afforded the product in a comparable yield of 83% indicating that both electronic and steric crowd play an insignificant role during mechanochemical thiocyanation (entry 4h, Table 3). A few entries with substituent variation in the fused pyridine-ring of imidazo[1,2-a]pyridine were carried out and in each case high to excellent yields of thiocyanated products were obtained (entries 4i–4m, Table 3). Notably, the substitution of the pyridine-ring with an EWG and the 2-aryl ring by an EDG does not affect the yield of the reaction and the reaction afforded an yield of 91% (entry 4l, Table 3). We also tried reactions with furan and thiophene at the 2 position of imidazo[1,2-a]pyridine, and observed that thiocyanation regioselectivity occurs in the vacant C-3 position of the imidazole ring of imidazo[1,2-a]pyridine and no thiocyanation was observed in the other five-membered heterocycles (entries 4n and 4o, Table 3). Therefore, mechanochemical C-3 thiocynation of imidazo[1,2-a]pyridine has been validated as a highly efficient protocol.
Table 3 Substrate scope on the mechanochemical thiocyanation reactions of imidazo[1,2-a]pyridinesa,b
In each case, 0.5 mmol 3, 0.55 mmol NaSCN, 0.5 mmol NCS, and 200 mg of silica gel were milled together in a 5 mL SS jar with one 10 mm SS ball in a RETSCH mixer-mill MM400 instrument at 30 Hz frequency for 30 min.
All yields reported here are isolated yields of products 4 after column chromatography.
|
|
Mechanochemical tandem synthesis: C-2 arylation and C-3 thiocyanation of indoles via double C–H activation
The scope of mechanochemistry is mostly confined to single-step transformations and tandem processes are being scarcely exemplified.46,49 We envisaged that the tandem mechanochemical C-2 arylation48 and C-3 thiocyanation by double C–H activation in a single-pot is possible on indole derivatives (1). The first step of the mechanochemical tandem reaction was the synthesis of 2-aryl indoles using Pd(II) as the catalyst via our reported procedure,48 followed by thiocyanation on the intermediate as shown in Table 4. At first, N-methylindole (1a) (1 mmol), iodobenzene (1.1 mmol), Pd(OAc)2 (5 mol%), AgOAc (1.2 mmol), AcOH (0.5 mmol), and 300 mg of silica gel were allowed to react at a frequency of 30 Hz for 1.5 h. Assessing the complete conversion to 1n through TLC monitoring, NaSCN (1.1 mmol), and NCS (1 mmol) were further added to the reaction mixture for the thiocyanation step of 2-aryl indole for another 20 min for complete C-3 thiocyanation to afford 2n in 74% isolated yield. Notably, the reported yield of N-methyl-2-phenyl-1H-indole (1n) is 91% and considering thiocyanation of 1n to 2n afforded 84% yield, the expected overall yield is 76% which is very similar to the isolated yield (74%) of the tandem process (entry 1, Table 4). All the tandem reactions were carried out under similar conditions. The time requirement for the C-2 arylation step was several hours and varied as per the electronic character of the starting indole48 but the second thiocyanation step was faster and completed within 20 min for each case (Table 4). The reaction mixture was then directly charged to the column for purification avoiding the work-up steps. Like the previous case, the thiocyanation step was hardly affected by the presence of both the EDG and EWG at the para-position of the C-2 aryl group of indoles. The yields are generally in the range of 70–80%, which matches closely with the expected overall yields.48 Therefore, the mechanochemical tandem C-2 and C-3 functionalization by double C–H activation of indole derivatives seems a very efficient and cost-effective protocol.
Table 4 Tandem mechanochemistry for double C–H activation of indolesa,b
Entry |
Ar |
Product |
Yield (%) |
Time (h) |
Indoles (1) (1 mmol), iodobenzene (1.1 mmol), Pd(OAc)2 (5 mol%), AgOAc (1.2 mmol), AcOH (0.5 mmol), and 300 mg of silica gel were allowed to react in a 5 mL SS jar with one SS ball at a frequency of 30 Hz in a RETSCH mixer-mill MM400 instrument. Subsequently, NaSCN (1.1 mmol) and NCS (1 mmol) were added and the milling was continued.
All yields reported here are isolated yields of the products (2) after column chromatography.
|
1 |
Ph |
|
74 |
2 |
2 |
4-Ph-Me |
|
73 |
3 |
3 |
4-Ph-OMe |
|
72 |
3 |
4 |
4-Ph-Br |
|
76 |
2 |
5 |
4-Ph-CN |
|
71 |
2 |
6 |
4-Ph-CF3 |
|
73 |
3 |
7 |
Ph |
|
79 |
2 |
8 |
4-Ph-Me |
|
80 |
3 |
9 |
4-Ph-Br |
|
74 |
3 |
10 |
Ph |
|
63 |
5 |
|
Gram scale synthesis
To validate the potential of the mechanochemical method from the laboratory scale to the pilot scale, the scalability of the reaction was investigated by performing gram scale reactions taking 1a, 1f, and 3a as the representative substrates. 10 mmol each of the substrates was allowed to react in a 25 mL SS jar with one 15 mm ball of the same material. The reagents and silica gel (3 g) were scaled up as well. The complete conversion of the gram-scale reaction took a little extra time than small-scale reactions, however, the reaction yields were comparable to milligram-scale reactions (Table 5). This indicates that the scaling up of the reaction with a choice of adequate size of milling jars and balls is possible without hampering the reaction yields giving a provision for bulk scale thiocyanation.
Table 5 Gram scale synthesis
Substrate |
Product |
Timea (min) |
Yielda,b (%) |
Within parenthesis, the corresponding time required for the completion of the reaction and yield on a 1 mmol scale is mentioned.
All yields reported here are isolated yields.
|
1a (R = Me) |
2a
|
20 (15) |
93 (95) |
1f (R = H) |
2f
|
20 (15) |
90 (91) |
3a
|
4a
|
45 (30) |
87 (88) |
|
trans-Functionalization of –SCN functionality
Thiocyanates are well-known synthetic precursors for trans-fuctionalization to a diverse range of sulfur-containing compounds and we envisaged to demonstrate mechanochemical trans-fuctionalization of –SCN with representative examples. Thus, further functionalization of a thiocyanato-indole (2a) and thiocyanato-carbazole (2ad) to trifluoromethyl thioethers and 5-substituted sulfenyl tetrazoles were attempted in a mixer-mill (Scheme 2). We were pleased to observe the successful conversion into corresponding trifluoromethyl thioethers by milling at 30 Hz for 3 h affording 5a and 5c in very high yields (94% and 91%, respectively). Similarly, 5-substituted sulfenyl tetrazole (5b and 5d) were obtained in over 90% yields from the –SCN precursors (2a and 2ad) this time by milling at 30 Hz for 2 h (Scheme 2). Although limited substrates were attempted, the study indicates the potential of mechanochemical milling for trans-fuctionalization of –SCN to other sulfur-containing functionalities.
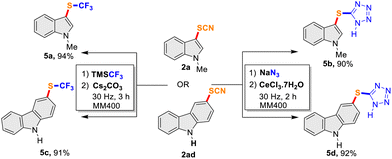 |
| Scheme 2
trans-Functionalization reactions of –SCN precursors. | |
Plausible mechanism
A plausible mechanism is proposed based on the experimental studies (Scheme 3). Firstly, the in situ formation of NTS from NCS and NaSCN during the milling process was established from NMR and IR studies. However, there is still a possibility of electrophilic, nucleophilic, or a radical pathway. A control experiment only with NaSCN did not afford any thiocyanated product (2a) ruling out the possibility of nucleophilic thiocyanaion. Whereas, when the reaction was conducted with NCS, NaSCN, and TEMPO (1 mmol) as the radical scavenger, the formation of 2a in a similar proportion (yield, 78%) suggested no involvement of a radical pathway indicating the expected electrophilic substitution reaction. As C-3 is more susceptible to electrophilic substitution, presumably, indole attacks the polarized N–S bond of NTS through C-3 and a temporary intermediate is formed, which quickly loses a proton to fall back to the product (2). A similar pathway is expected for imidazo[1,2-a]pyridines as well leading to the formation of 4.
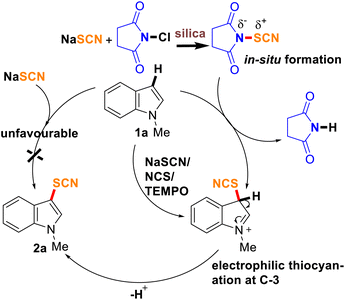 |
| Scheme 3 Plausible mechanism of mechanochemical thiocyanation. | |
Sustainability matrices
Finally, a comparative study of the present method was performed with available conventional solution phase thiocyanation protocols and other similar methods (Table S2 ESI†). The sustainability of this mechanochemical thiocyanation protocol was assessed from the calculations of key green metrics like the E-factor and Ecoscale score with other available thiocyanation protocols (Table S2, ESI†). For high-yielding reactions, for example, in the formation of 2a, the Ecoscale score is 86 and the E-factor is just 3 (entry 8, Table S2†). In contrast, most of the available solution-phase protocols show an Ecoscale score below 60 and the E-factors are several folds higher (up to 48) than the present method (Table S2, ESI†). Moreover, metal catalysts or additives are required for most cases, whereas, the present method is metal-free, catalyst-free, and devoid of workup steps which makes it a cost-effective and sustainable process. A quick cost calculation revealed that the cost for the manufacturing of 2a per gram in terms of the cost of raw materials only is USD 4.75 for our method, whereas, it costed USD 36.5 for Hu's method35 as they used a costly and toxic palladium catalyst for the cross-coupling reactions (Table S2, ESI†). From the comparative study, it may easily be stated that the mechanochemical method is much superior to other methods.
Experimental
General experimental details
The chemicals were obtained from Sigma-Aldrich, Alfa Aesar, or TCI India and used directly without further purification. Common reagents and solvents of AR grade were obtained from local suppliers. The mechanochemical reactions at the milligram scale were carried out in 5 mL jars made of stainless steel with a single 10 mm ball of the same material in the RETSCH mixer-mill MM400. The reactions were monitored by thin layer chromatography (TLC) carried out on 0.25 mm silica gel aluminum plates (60F-254) using UV light (254 or 365 nm) for monitoring the progress of the reactions. 1H NMR, 19F NMR, and 13C NMR spectra were recorded on a Bruker Avance (500 MHz) NMR instrument with CDCl3 (or DMSO-d6) as the solvent. Tetramethylsilane (TMS) was used as an internal standard for 1H and 13C NMR spectroscopy while trifluoroacetic acid (δ −76.80 ppm) was used as an internal standard for 19F NMR. Isolation and purification of all the compounds were conducted by conventional column chromatography (silica gel, 60–120 mesh) using variable proportions of ethyl acetate (EA)–petroleum ether (PE) as the eluents. Chemical shifts are reported in parts per million (δ) units. Coupling constants are reported in hertz (Hz), and standard abbreviations are used for representing the multiplicity of NMR peaks such as s (singlet), bs (broad singlet), d (doublet), dd (doublet of doublets), t (triplet), q (quartet), and m (multiplet). IR affinity-1 FTIR spectrophotometer (Shimadzu) was used to record IR spectra. Mass spectra were recorded on a quadrupole time-of-flight liquid crystal mass spectrometer (6545 Q-TOF LC-MS, Agilent) using electrospray ionization (ESI) as an ion source.
Synthetic procedures
General procedure for mechanochemical thiocyanation of indoles (1) and imidazo[1,2-a]pyridines (3).
A mixture of 1 (1 mmol) or 3 (0.5 mmol), NaSCN (1.1 equiv.), NCS (1 equiv.), and silica gel (60–120 mesh, 300 mg for 1 and 200 mg for 3) was added to a 5 mL SS jar along with a 10 mm ball of the same material. The reaction was set to a frequency of 30 Hz in a RETSCH mixer-mill MM400 instrument and it was milled for 15 min (or 30 min for 3). The slurry after the completion of the reaction was immediately charged to a column for purification and was eluted with ethyl acetate–petroleum ether to afford the thiocyanated products 2 (or 4).
General procedure for tandem mechanochemical C-2, C-3 functionalization of indoles (1).
A mixture of the indole substrate (1 mmol), iodoarene (1.1 mmol), Pd(OAc)2 (0.05 mmol), AgOAc (1.2 mmol), and CH3COOH (0.5 mmol) was taken in a 5 mL stainless steel milling jar containing 300 mg of silica gel and a ball of 10 mm diameter of the same material was used. The jar was capped properly and the reaction mixture was subjected to milling in a RETSCH MM400 instrument at a frequency of 30 Hz. After completion of the C-2 arylation step as suggested by TLC, the reaction mixture was further milled with NaSCN (1.1 mmol) and NCS (1 mmol) for 20 min. The slurry formed was charged to the column for purification to obtain the C-2, C-3 functionalized indoles (2).
Conclusions
In summary, we developed a simple and cost-effective route for solid-phase electrophilic C–H thiocyanation of indoles (1) and imidazo[1,2-a]pyridines (3) using a mixer-mill. The reaction was initiated by the in situ generation of N-thiocyanatosuccinimide from commercially available cheaper precursors, N-chlorosuccinimide and NaSCN, in silica gel as the milling media. Subsequently, the C-3 selective thiocyanation reaction on indoles and imidazo[1,2-a]pyridines proceeded under catalyst-free and solvent-free conditions by milling at 30 Hz to afford the products within 15–30 min in high to excellent yields. The products were obtained by charging the solid reaction slurry directly to the column chromatography system and thus, a work-up step could be avoided. A diverse array of substrates with different electronic characters both in indole and imidazo[1,2-a]pyridine scaffolds took part in the reactions with similar ease, highlighting the versatility and efficiency of this mechanochemical protocol. The gram scale reactions afforded products in comparable yield and reactivity, indicating the scalability of this method in terms of potential industrial applications. Notably, mechanochemical tandem C–C and C–S bond formation reactions on indoles by double C–H activation were achieved in high overall yields, leading to easy access to C-2 aryl and C-3 thiocyanato compounds from unsubstituted indoles. Moreover, selected examples demonstrated the feasibility of mechanochemical trans-functionalization of the –SCN precursors to –SCF3 and 5-substituted sulfenyl tetrazole. In terms of sustainability metrics, with a low E-factor of 3 and an eco-scale score above 80, the present method is several fold greener than other literature-known methods.
Author contributions
The manuscript was written through the contributions of all authors. SS carried out optimization studies, thiocyanation of indoles, and spectral data interpretation; ABP carried out thiocyanation reactions of imidazo[1,2-a]pyridines; ZTB was engaged in the synthesis of the starting materials of imidazo[1,2-a]pyridines; AC contributed with supervision and revision of the manuscript; MB was engaged in the design of the project, investigation, and overviewing of the entire project, supervision, manuscript writing, and reviewing. All authors have given approval for the final version of the manuscript.
Conflicts of interest
The authors declare no personal, financial, or organizational conflict of interest.
Acknowledgements
M. B. is grateful to the DBT BUILDER-BITS Pilani KK Birla Goa Campus Interdisciplinary Life Science program (Grant number: BT/INF/22/SP42543/2021) for financial support. S. S. is indebted to BITS Pilani, KK Birla Goa campus for SRFship. A. B. P. is indebted to BITS Pilani, KK Birla Goa campus for JRFship. We acknowledge the central sophisticated instrumentation facilities (CSIFs) of BITS Pilani, KK Birla Goa campus, for NMR analysis, and BITS Pilani, Pilani and Hyderabad campus for HRMS facilities.
References
- T. Castanheiro, J. Suffert, M. Donnard and M. Gulea, Recent advances in the chemistry of organic thiocyanates, Chem. Soc. Rev., 2016, 45, 494–505, 10.1039/c5cs00532a.
- Z. Israfilova, P. Taslimi, İ. Gülçin, Y. Abdullayev, V. Farzaliyev, M. Karaman, A. Sujayev and S. H. Alwasel, Some thiocyanate containing heterocyclic compounds: Synthesis, bioactivity and molecular docking study, ChemistrySelect, 2023, 8, e20220365, DOI:10.1002/slct.202203653.
- R. Riemschneider, Thiocarbamates and related compounds. A new reaction of thiocyanates, J. Am. Chem. Soc., 1956, 78, 844–847, DOI:10.1021/ja01585a038.
- B. Bayarmagnai, C. Matheis, K. Jouvin and L. J. Goossen, Synthesis of difluoromethyl thioethers from difluoromethyl trimethylsilane and organothiocyanates generated in situ, Angew. Chem., 2015, 127, 5845–5848, DOI:10.1002/ange.201500899.
- C. K. Maurya, A. Mazumder and P. K. Gupta, Phosphorus pentasulfide mediated conversion of organic thiocyanates to thiols, Beilstein J. Org. Chem., 2017, 13, 1184–1188, DOI:10.3762/bjoc.13.117.
- P. A. Grieco, Y. Yokoyama and E. Williams, Aryl selenocyanates and aryl thiocyanates: Reagents for the preparation of activated esters, J. Org. Chem., 1978, 43, 1283–1285, DOI:10.1021/jo00400a070.
- K. R. Prabhu, A. R. Ramesha and S. Chandrasekaran, Reductive dimerization of organic thiocyanates to disulfides mediated by tetrathiomolybdate, J. Org. Chem., 1995, 60, 7142–7143, DOI:10.1021/jo00127a017.
- H. Gao, Q. Zhang and J. M. Shreeve, Fused heterocycle-based energetic materials (2012–2019), J. Mater. Chem. A, 2020, 8, 4193–4216, 10.1039/c9ta12704f.
- J. Yu, Y. Zhou, D.-F. Chen and L.-Z. Gong, Organocatalytic asymmetric synthesis of chiral nitrogenous heterocycles and natural products, Pure Appl. Chem., 2014, 86, 1217–1226, DOI:10.1515/pac-2013-1208.
- R. S. Gross, Z. Guo, B. Dyck, T. Coon, C. Q. Huang, R. F. Lowe, D. Marinkovic, M. Moorjani, J. Nelson, S. Zamani-Kord, D. E. Grigoriadis, S. R. Hoare, P. D. Crowe, J. H. Bu, M. Haddach, J. McCarthy, J. Saunders, R. Sullivan, T. Chen and J. P. Williams, Design and synthesis of tricyclic corticotropin-releasing factor-1 antagonists, J. Med. Chem., 2005, 48, 5780–5793, DOI:10.1021/jm049085v.
- M. L. Hillwig, H. A. Fuhrman, K. Ittiamornkul, T. J. Sevco, D. H. Kwak and X. Liu, Identification and characterization of a welwitindolinone alkaloid biosynthetic gene cluster in the stigonematalean cyanobacterium hapalosiphon welwitschii, ChemBioChem, 2014, 15, 665–669, DOI:10.1002/cbic.201300794.
- V. A. Kokorekin, A. O. Terent'ev, G. V. Ramenskaya, N. Grammatikova, G. M. Rodionova and A. I. Ilovaiskii, Synthesis and antifungal activity of arylthiocyanates, Pharm. Chem. J., 2013, 47, 422–425, DOI:10.1007/s11094-013-0973-7.
- D. V. Fedyuk, I. I. Maletina and L. M. Yagupol'skii, Fluorine-containing derivatives of imidazole and benzimidazole exhibiting hypotensive properties, Ukr. Khim. Zh., 1999, 65, 81–86 CAS.
-
J. W. Gillard, H. E. Morton, R. Fortin and Y. Guindon, 3-Hetero-substituted-N-benzyl-indoles and prevention of leucotriene synthesis therewith, EP Pat, 0275667, 1987 Search PubMed.
- R. Ragno, A. Coluccia, G. La Regina, G. De Martino, F. Piscitelli, A. Lavecchia, E. Novellino, A. Bergamini, C. Ciaprini, A. Sinistro, G. Maga, E. Crespan, M. Artico and R. Silvestri, Design, molecular modeling, synthesis, and anti-HIV-1 activity of new indolyl aryl sulfones. Novel derivatives of the indole-2-carboxamide, J. Med. Chem., 2006, 49, 3172–3184, DOI:10.1021/jm0512490.
- J. Vaca, F. Salazar, A. Ortiz and E. Sansinenea, Indole alkaloid derivatives as building blocks of natural products from bacillus thuringiensis and bacillus velezensis and their antibacterial and antifungal activity study, J. Antibiot., 2020, 73, 798–802, DOI:10.1038/s41429-020-0333-2.
- J. Dhuguru and R. Skouta, Role of indole scaffolds as pharmacophores in the development of anti-lung cancer agents, Molecules, 2020, 25, 1615, DOI:10.3390/molecules25071615.
- B. O. Sucu, Biological evaluation of imidazopyridine derivatives as potential anticancer agents against breast cancer cells, Med. Chem. Res., 2022, 31, 2231–2242, DOI:10.1007/s00044-022-02984-x.
- N. Devi, D. Singh, R. K. Rawal, J. Bariwal and V. Singh, Medicinal attributes of imidazo[1,2-a]pyridine derivatives: An update, Curr. Top. Med. Chem., 2016, 16, 2963–2994, DOI:10.2174/1568026616666160506145539.
- A. Dey and A. Hajra, Potassium persulfate-mediated thiocyanation of 2H-indazole under iron-catalysis, Adv. Synth. Catal., 2018, 361, 842–849, DOI:10.1002/adsc.201801232.
- D. Yang, K. Yan, W. Wei, G. Li, S. Lu, C. Zhao, L. Tian and H. Wang, Catalyst-free regioselective C-3 thiocyanation of Imidazopyridines, J. Org. Chem., 2015, 80, 11073–11079, DOI:10.1021/acs.joc.5b01637.
- F. Wang, X. Yu, Z. Qi and X. Li, Rhodium–catalyzed C-S and C-N functionalization of Arenes: Combination of C-H activation and hypervalent iodine chemistry, Chem. – Eur. J., 2015, 22, 511–516, DOI:10.1002/chem.201504179.
- N. Iranpoor, H. Firouzabadi and H. R. Shaterian, Efficient conversion of thiols to thiocyanates by in situ generated Ph3P(SCN)2, Tetrahedron Lett., 2002, 43, 3439–3441, DOI:10.1016/s0040-4039(02)00421-5.
- Y. Ju, D. Kumar and R. S. Varma, Revisiting nucleophilic substitution reactions: Microwave-assisted synthesis of azides, thiocyanates, and sulfones in an aqueous medium, J. Org. Chem., 2006, 71, 6697–6700, DOI:10.1021/jo061114h.
- S. Mitra, M. Ghosh, S. Mishra and A. Hajra, Metal-free thiocyanation of imidazoheterocycles through visible light photoredox catalysis, J. Org. Chem., 2015, 80, 8275–8281, DOI:10.1021/acs.joc.5b01369.
- W. Fan, Q. Yang, F. Xu and P. Li, A visible-light-promoted aerobic metal-free C-3 thiocyanation of Indoles, J. Org. Chem., 2014, 79, 10588–10592, DOI:10.1021/jo5015799.
- F.-L. Zeng, H.-L. Zhu, X.-L. Chen, L.-B. Qu and B. Yu, Visible light-induced recyclable g,-C3N4 catalyzed thiocyanation of C(sp2)–H bonds in sustainable solvents, Green Chem., 2021, 23, 3677–3682, 10.1039/d1gc00938a.
- G. Li, Q. Yan, X. Gong, X. Dou and D. Yang, Photocatalyst-free regioselective C–H thiocyanation of 4-anilinocoumarins under visible light, ACS Sustainable Chem. Eng., 2019, 7, 14009–14015, DOI:10.1021/acssuschemeng.9b02511.
- K. Jouvin, C. Matheis and L. J. Goossen, Synthesis of aryl tri– and difluoromethyl thioethers via a C-H thiocyanation/fluoroalkylation cascade, Chem. – Eur. J., 2015, 21, 14324–14327, DOI:10.1002/chem.201502914.
- J. Qiu, D. Wu, P. G. Karmaker, H. Yin and F.-X. Chen, Enantioselective organocatalyzed direct α-thiocyanation of cyclic β-ketoesters by N-thiocyanatophthalimide, Org. Lett., 2018, 20, 1600–1603, DOI:10.1021/acs.orglett.8b00342.
- D. Wu, J. Qiu, P. G. Karmaker, H. Yin and F.-X. Chen, N-Thiocyanatosaccharin: A “sweet” electrophilic thiocyanation reagent and the synthetic applications, J. Org. Chem., 2018, 83, 1576–1583, DOI:10.1021/acs.joc.7b02850.
- C. Li, P. Long, H. Wu, H. Yin and F.-X. Chen, N-Thiocyanato-dibenzenesulfonimide: A new electrophilic thiocyanating reagent with enhanced reactivity, Org. Biomol. Chem., 2019, 17, 7131–7134, 10.1039/c9ob01340g.
- F. D. Toste, V. D. Stefano and I. W. Still, A versatile procedure for the preparation of aryl thiocyanates using N-thiocyanatosuccinimide (NTS), Synth. Commun., 1995, 25, 1277–1286, DOI:10.1080/00397919508012691.
- H. Jiang, W. Yu, X. Tang, J. Li and W. Wu, Copper-catalyzed aerobic oxidative regioselective thiocyanation of aromatics and heteroaromatics, J. Org. Chem., 2017, 82, 9312–9320, DOI:10.1021/acs.joc.7b01122.
- G. Hu, P. Li, Z. Zhou, F. Yang, S. Xu, H. Fan, X. Zhao and X. Zhang, NBS-assisted palladium-catalyzed bromination/cross-coupling reaction of 2-alkynyl arylazides with KSCN: An efficient method to synthesize 3-thiocyanindoles, New J. Chem., 2021, 45, 3828–3832, 10.1039/d0nj05894g.
- M. Gao, M. Chen, X. Pannecoucke, P. Jubault and T. Besset, Pd–catalyzed directed thiocyanation reaction by C−H bond activation, Chem. – Eur. J., 2020, 26, 15497–15500, DOI:10.1002/chem.202003521.
- I. Habib, K. Singha and M. Hossain, Transition metal-free C–H activation of heteroarenes: An overview (2018–2022), Synth. Commun., 2022, 53, 1–22, DOI:10.1080/00397911.2022.2155837.
- J. Grover, G. Prakash, N. Goswami and D. Maiti, Traditional and sustainable approaches for the construction of C–C bonds by harnessing C–H arylation, Nat. Commun., 2022, 13, 1085, DOI:10.1038/s41467-022-28707-9.
- K. J. Ardila-Fierro and J. G. Hernández, Sustainability assessment of mechanochemistry by using the twelve principles of green chemistry, ChemSusChem, 2021, 14, 2145–2162, DOI:10.1002/cssc.202100478.
- T. Friščić, C. Mottillo and H. M. Titi, Mechanochemistry for Synthesis. Angew. Chem., Int. Ed., 2019, 59, 1018–1029, DOI:10.1002/anie.201906755.
- V. Martinez, T. Stolar, B. Karadeniz, I. Brekalo and K. Užarević, Advancing mechanochemical synthesis by combining milling with different energy sources, Nat. Rev. Chem., 2022, 7, 51–65, DOI:10.1038/s41570-022-00442-1.
- D. Tan and T. Friščić, Mechanochemistry for organic chemists: An update, Eur. J. Org. Chem., 2017, 18–33, DOI:10.1002/ejoc.201700961.
- T. Auvray and T. Friščić, Shaking things from the ground-up: A systematic overview of the mechanochemistry of hard and high-melting inorganic materials, Molecules, 2023, 28, 897, DOI:10.3390/molecules28020897.
- D. Tan, L. Loots and T. Friščić, Towards medicinal mechanochemistry: Evolution of milling from pharmaceutical solid form screening to the synthesis of active pharmaceutical ingredients (APIs), Chem. Commun., 2016, 52, 7760–7781, 10.1039/c6cc02015a.
- M. Banerjee, A. A. Bhosle, A. Chatterjee and S. Saha, Mechanochemical synthesis of organic dyes and fluorophores, J. Org. Chem., 2021, 86, 13911–13923, DOI:10.1021/acs.joc.1c01540.
- S. Saha, A. A. Bhosle, A. Chatterjee and M. Banerjee, Mechanochemical Duff reaction in solid phase for easy access to mono- and di-formyl electron-rich arenes, J. Org. Chem., 2023, 88, 10002–10013, DOI:10.1021/acs.joc.3c00789.
- D. Das, A. A. Bhosle, P. C. Panjikar, A. Chatterjee and M. Banerjee, Mn(I)-catalyzed mechanochemical C–H bond activation: C-2 selective alkenylation of Indoles, ACS Sustainable Chem. Eng., 2020, 8, 19105–19116, DOI:10.1021/acssuschemeng.0c07465.
- D. Das, Z. T. Bhutia, A. Chatterjee and M. Banerjee, Mechanochemical Pd(II)-catalyzed direct and C-2-selective arylation of Indoles, J. Org. Chem., 2019, 84, 10764–10774, DOI:10.1021/acs.joc.9b01280.
- M. Ferguson, N. Giri, X. Huang, D. Apperley and S. L. James, One-pot two-step mechanochemical synthesis: Ligand and complex preparation without isolating intermediates, Green Chem., 2014, 16, 1374–1382, 10.1039/c3gc42141d.
Footnote |
† Electronic supplementary information (ESI) available: Experimental procedures, optimization of milling parameters, comparative table, spectral data and copies of spectra. See DOI: https://doi.org/10.1039/d4gc00486h |
|
This journal is © The Royal Society of Chemistry 2024 |
Click here to see how this site uses Cookies. View our privacy policy here.