DOI:
10.1039/D3GC04273A
(Communication)
Green Chem., 2024,
26, 300-305
Photocatalytic synthesis of 2,3-diamines from anilines and DIPEA via C–N bond cleavage and C–C bond formation†
Received
5th November 2023
, Accepted 4th December 2023
First published on 5th December 2023
Abstract
2,3-Diamines are skeleton structures widely found in natural products and drug molecules and can also be used as ligands in transition metal catalysis. A novel visible light-driven synthesis method for 2,3-diamines using anilines and diisopropylethylamine as starting materials and aldehydes as proton acceptors under the action of the organic photosensitiser 4CzIPN has been developed. The method has mild conditions, avoids the use of metal reagents and the reaction mechanism does not involve the imine intermediate. Under optimal conditions, the yield of 2,3-diamines is up to 90%. In addition, the aldehydes give rise to 2,3-diols after the reaction, which means that the method can synthesise diamines and diols in one pot.
Introduction
Vicinal diamines are widely found in natural products and drug molecules1 and are widely used as ligands for transition metal catalysis in organic chemistry.2 For the synthesis of 2,3-diamines, many methods have been developed (Fig. 1); diamination of olefins is an obvious approach, but alternatives are the nitro-Mannich reaction,3 reductive coupling of imines,4 oxidative coupling of amines,5 ring opening of aziridines,6 nucleophilic addition to diimines and substitution of diols.7 Concerning the reductive coupling of imines, a variety of metal reductants and auxiliaries have been developed.8 However, these methods have major limitations such as the use of stoichiometric metal reagents and the need to prepare the imine in advance.
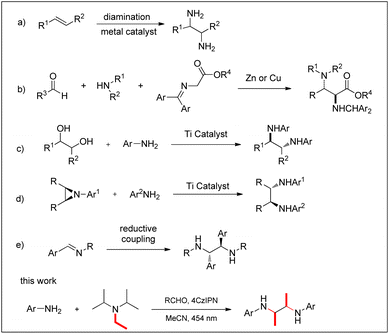 |
| Fig. 1 Synthesis routes of vicinal diamines (a–e). | |
Over the last few years, organic photochemical synthesis has become a powerful tool to avoid or reduce the use of environmentally harmful metal reagents in conventional synthesis.9 For example, Sudo et al. reported the photocatalytic reductive coupling of imines using perylene as the photosensitizer and trialkylamine as the reducing agent.10 Rueping's group used iridium photosensitizers to achieve visible light-mediated photoinduced catalytic reductive coupling of imines and direct coupling of imine–aniline and aldehyde–aniline to access a wide range of substrates under mild reaction conditions.11 Cozzi used coumarin dyes for photoredox catalysis and NEt3 as a reducing agent to accomplish imine reduction.12
Aniline is a low-cost, commercial product, and coupling of aniline with imines or aldehydes to synthesize 2,3-diamines is an efficient process. Zhang used BiCl3 as a catalyst to synthesize diamines by in situ reductive coupling of aniline and aldehydes.13 Although this avoided the need to prepare the imine beforehand, it still required an excess of metal reducing agent. Therefore, an environmentally more benign system for the efficient catalytic synthesis of 2,3-diamines would be a desirable development.
Trialkylamines such as triethylamine and diisopropylethylamine are widely used as electron transfer reagents or reducing agents in organic synthesis.14 In addition, trialkylamines can be used as carbon sources to participate in the reaction and provide ethyl or methyl.15 However, studies on trialkylamines as carbon sources have mostly focused on the construction of nitrogen-containing heterocycles. The use of trialkylamines to provide carbon chains in the synthesis of 2,3-diamines has not been reported yet.
Herein, we report a new method for the direct synthesis of 2,3-diamines from aniline with visible light catalysis and the organic photosensitizer 4CzIPN as the photocatalyst. Diisopropylethylamine not only acts as a reagent to provide an ethyl group, but also acts as a reducing agent. Benzaldehyde is used as a proton transfer reagent, producing 2,3-diols as a by-product.
Results and discussion
In preliminary experiments to optimize the reaction conditions, phenylamine 1a and (i-Pr)2NEt 2 were selected as model substrates, Table 1. Using fac-Ir(ppy)3 as a photocatalyst and benzaldehyde as a hydrogen atom transfer (HAT) reagent under 20 W blue LED irradiation, the desired 2,3-diamine 3a was obtained in 53% yield (Table 1, entry 1). When 4CzIPN was used as a photocatalyst, 3a was obtained in 69% yield (entry 2). Other carbazole photocatalysts such as 4CzIPN, 4CzPN, 4CzTPN, 4t-BuCzIPN and 4DPAIPN (entry 3) did not give the best results. The reaction failed with other photocatalysts such as Ru(bpy)3Cl2, benzophenone, eosin Y, DDQ, riboflavin, xanthone and 1,4-dicyanobenzene (entry 4). The solvent strongly influenced the reaction, which did not occur in toluene, DMSO, EtOH and HFIP (entry 8). THF, acetone, DMF and 1,4-dioxane gave poor to moderate yields of 3a (entries 5–7, 9), although DCE gave 65% yield (entry 10). Subsequently, in a series of control experiments, reactions in the dark (entry 11) at 70 °C (entry 12) and under an air atmosphere (entry 13) gave no, or only a trace amount of, detectable product, suggesting a free radical mechanism facilitated by light. On the other hand, a higher catalyst loading (10 mol% instead of 2 mol% 4CzIPN, entry 14) significantly improved the yield of 3a to 82%. We speculate that during 2,3-diamine formation, the conversion of benzaldehyde into the diol also needs a photosensitizer, so a higher photocatalyst loading is beneficial. Finally, the optimal reaction conditions developed require 10 mol% 4CzIPN as a photocatalyst, benzaldehyde as a proton transfer reagent, and CH3CN as a solvent at room temperature under irradiation.
Table 1 Preliminary development of reductive coupling conditionsa

|
Entry |
Photocatalyst |
Solvent |
Yield 3a b (%) |
dr |
Reaction conditions: 1a (0.3 mmol), (iPr)2NEt (0.6 mmol), PhCHO (0.3 mmol) and photocatalyst (2 mol%) in solvent (2 mL) at room temperature under argon and 20 W blue LED (454 nm) irradiation, unless otherwise stated.
Isolated yields, dr = diastereomeric ratio.
The reaction was carried out in the dark.
The reaction was carried out at 70 °C.
The reaction was carried out under an air atmosphere.
10 mol% 4CzIPN.
|
1 |
fac-Ir(ppy)3 |
CH3CN |
53 |
1 : 1 |
2 |
4CzIPN |
CH3CN |
69 |
2 : 3 |
3 |
4CzPN, 4CzTPN, 4t-BuCzIPN, 4DPAIPN |
CH3CN |
42, 55, 61, 56 |
1 : 1 |
4 |
Ru(bpy)3Cl2, benzophenone, eosin Y, DDQ, rhodamine B, riboflavin, xanthone, 1,4-dicyanobenzene |
CH3CN |
Trace or n.d. |
— |
5 |
4CzIPN |
THF |
34 |
2 : 1 |
6 |
4CzIPN |
Acetone |
58 |
1 : 1 |
7 |
4CzIPN |
DMF |
52 |
2 : 3 |
8 |
4CzIPN |
Toluene; DMSO; EtOH; HFIP |
Trace |
— |
9 |
4CzIPN |
1,4-Dioxane |
50 |
1 : 1 |
10 |
4CzIPN` |
DCE |
65 |
1 : 2 |
11c |
4CzIPN |
CH3CN |
n.d. |
— |
12d |
4CzIPN |
CH3CN |
n.d. |
— |
13e |
4CzIPN |
CH3CN |
Trace |
— |
14f |
4CzIPN |
CH3CN |
82 |
2 : 1 |
|
To probe the substrate scope, a variety of substituted phenylamines 1a–z were applied (Table 2). For aniline substrates bearing substituents at the para position of the benzene ring, both electron-donating and -withdrawing groups are tolerated to give the corresponding product 3 in moderate to excellent yields. para-substitution with –Me 1b, –Et 1c, –iPr 1d and sterically-hindered tBu 1e gave 77, 80, 76 and 66% yields, respectively. Halogen-substituted substrates 1f–h gave 60–72% yields. The aniline containing the strong electron-withdrawing trifluoromethoxy group can react smoothly to give the desired product in 56% yield. The substituent pattern also affects the yield and dr. meta-substitution with –Et 1l, –iPr 1m and –tBu 1n or halides 1o–q gave better yields than the ortho- or para-substituted analogues. The strong electron-withdrawing –CF3 substitution group 1r gave 60% yield. ortho-substituted anilines 1s–u had a negative effect on the yield and dr. Disubstitution is well tolerated. For example, 3,4-dimethylaniline and 3,5-dimethylaniline gave 57% and 67% yields of 3v and 3w, respectively. Aniline-d5 1y and 5-aminoindan 1z also reacted smoothly.
Table 2 Phenylamine substrate scope
Reaction conditions: 1a–z (0.3 mmol), (iPr)2NEt (0.6 mmol), PhCHO (0.3 mmol) and 4CzIPN (10 mol%) in CH3CN (2 mL) at room temperature under argon and 20 W blue LED (454 nm) irradiation, unless otherwise stated. Isolated yields of 3a–z are provided. |
|
We then tested this photocatalytic system in the reaction of aldehydes to diol products. The substrates contain electron-donating and electron-withdrawing groups which can react easily to give the corresponding diol products, as shown in Table 3. When the para-position of the benzaldehyde has electron-donating groups such as –Me (5b), –OMe (5c), –Ph (5f) and –OBn (5g), moderate to good yields can be obtained. Halogen-substituted benzaldehyde substrates are also suitable for photocatalytic systems and target products can be obtained in moderate to good yields (5h and 5i). The steric hindrance effect has a great influence on the reaction, for example, the yields of 2-OMe (5e), 3-OMe (5d) and 4-OMe (5c) substituted benzaldehydes increased with the increase in steric hindrance and were 63%, 73% and 82%, respectively. The reason for this may be that as the steric hindrance increases, the phenylethanol radical becomes more stable, which favours its formation. The heterocyclic thiophene-formaldehyde (5m) and furan-formaldehyde (5n) can also yield the target diols. In addition, adamantane containing benzaldehyde can also be reacted to obtain 56% yield (5o).
Table 3 Benzaldehyde substrate scope
Reaction conditions: ArCHO (0.3 mmol), (iPr)2NEt (0.6 mmol), PhNH2 (0.3 mmol) and 4CzIPN (10 mol%) in CH3CN (2 mL) at room temperature under argon and 20 W blue LED (454 nm) irradiation, unless otherwise stated. Isolated yields of 5a–o are provided. |
|
Next, the proton transfer reagent was investigated, replacing benzaldehyde with other alkyl aldehydes. Acetaldehyde increases the yield of diamine 3a to 85% (Scheme 1a). When propanal is used, not only the symmetric diamine 3a but also symmetric 6 and asymmetric 7 are formed, resulting from the incorporation of C2 and C3 fragments from diisopropylethylamine and propanal, respectively (Scheme 1b). In validation of this observation, butanal behaved similarly to provide a C4 fragment and a mixture of 3a, symmetric 8 and asymmetric 9 (Scheme 1c). When the aldehyde is replaced by benzophenone, the reaction proceeds smoothly to give only a single product 3a, indicating that benzophenone acts only as an oxidant, accepting hydrogen in the reaction (Scheme 1d).
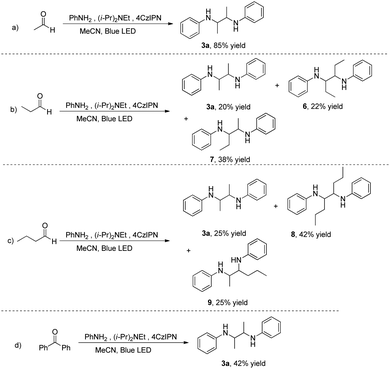 |
| Scheme 1 Alkyl aldehyde reagent scope (a–d). | |
To investigate the scalability of the silane alkynylation protocol, we carried out a reaction using 1.1 g of phenylamine 1a, which gave 3a in 71% yield and 5a in 46% yield (Scheme 2a). We then used the diamine product to synthesize cyclic molecules and found that the cyclization reaction of diamine with SOCl2 or POCl3 can proceed smoothly to form nitrogen-substituted thiosulfinyl compounds (Scheme 2b, 9 and 10).
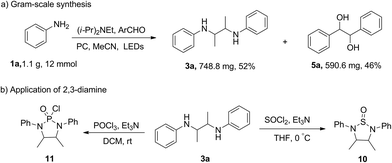 |
| Scheme 2 Synthetic applications of the reaction. | |
To investigate the mechanism of this reaction, control experiments were carried out. First, the imine formed between benzaldehyde and aniline was added to the reaction under standard conditions, resulting in no detectable 3a (Scheme 3a). The imine formed between aniline and acetaldehyde also failed to react (Scheme 3b), indicating that the reaction did not proceed through imine formation. Addition of the radical scavenger 1,1-stilbene gave the addition product of the N-ethylaniline radical and stilbene in 60% yield (Scheme 3c).
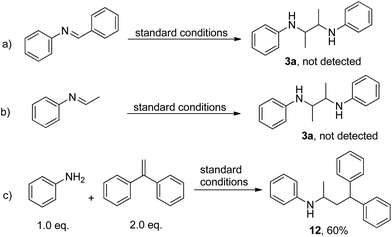 |
| Scheme 3 Control experiments (a–c). | |
Based on the above observations, a tentative mechanism for this coupling reaction is shown in Fig. 2. First, the photosensitizer (PC) is excited under visible light irradiation, diisopropylethylamine undergoes oxidative single electron transfer to form the radical cation A, and the photosensitizer is reduced. Then, the radical cation A forms iminium intermediate B, which transfers protons and electrons to benzaldehyde via proton-coupled electron transfer (PCET). The resulting benzylalcohol radical dimerizes to form diol 4. Iminium B then reacts with aniline to give aminal cation tautomers C and D, which eliminates a molecule of diisopropylamine to give N-ethylidenebenzenaminium E. Finally, E is reduced by the photosensitizer to give the N-ethylaniline radical F, which dimerizes to give the final 2,3-diamine 3a.
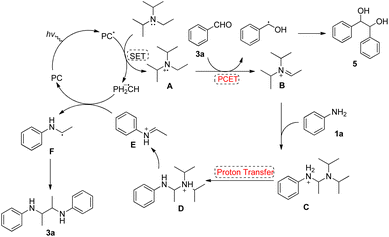 |
| Fig. 2 Proposed mechanism. | |
Conclusions
In conclusion, we have developed a direct photocatalytic method for the synthesis of 2,3-diamines from anilines, diisopropylethylamine and alkyl aldehydes, demonstrating the use of trialkylamines to provide a key carbon fragment. This mild method has a good aniline substrate scope and avoids the use of stoichiometric metal reagents. Control experiments show that the reaction mechanism does not proceed through the imine intermediate.
Conflicts of interest
There are no conflicts to declare.
Acknowledgements
We are grateful to the National Natural Science Foundation of China (21961045) and the Applied Basic Research Project of Yunnan Province (202301AT070013).
References
-
(a) A. Viso, R. F. D. Pradilla, A. Garcia and A. Flores, Chem. Rev., 2005, 105, 3167–3196 CrossRef CAS PubMed;
(b) S. R. S. S. Kotti, C. Timmons and G. Li, Chem. Biol. Drug Des., 2006, 67, 101–114 CrossRef CAS;
(c) F. Cardona and A. Goti, Nat. Chem., 2009, 1, 269–275 CrossRef CAS;
(d) D. C. Blakemore, L. Castro, I. Churcher, D. C. Rees, A. W. Thomas, D. M. Wilson and A. Wood, Nat. Chem., 2018, 10, 383–394 CrossRef CAS.
-
(a) W. Zhang, J. L. Loebach, S. R. Wilson and E. N. Jacobsen, J. Am. Chem. Soc., 1990, 112, 2801–2803 CrossRef CAS;
(b) M. Dai, B. Liang, C. Wang, J. Chen and Z. Yang, Org. Lett., 2004, 6, 221–224 CrossRef CAS PubMed;
(c) K. Nakayama and K. Maruoka, J. Am. Chem. Soc., 2008, 30, 17666–17667 CrossRef PubMed;
(d) H. Liao, L. Zhong, Z. Xiao, T. Zheng, H. Gao and Q. Wu, Chem. – Eur. J., 2016, 22, 14048–14055 CrossRef CAS PubMed.
-
(a) L. Bernardi, A. S. Gothelf, R. G. Hazell and K. A. Jørgensen, J. Org. Chem., 2003, 68, 2583–2591 CrossRef CAS PubMed;
(b) T. Ooi, M. Kameda, J.-I. Fujii and K. Maruoka, Org. Lett., 2004, 6, 2397–2399 CrossRef CAS PubMed;
(c) A. Okada, T. Shibuguchi, T. Ohshima, H. Masu, K. Yamaguchi and M. Shibasaki, Angew. Chem., Int. Ed., 2005, 44, 4564–4567 CrossRef CAS PubMed;
(d) M. M. Salter, J. Kobayashi, Y. Shimizu and S. Kobayashi, Org. Lett., 2006, 8, 3533–3536 CrossRef CAS PubMed;
(e) X. X. Yan, Q. Peng, Q. Li, K. Zhang, J. Yao and X. L. Hou, J. Am. Chem. Soc., 2008, 130, 14362–14363 CrossRef CAS PubMed;
(f) J. Hernández-Toribio, R. G. Arrayás and J. C. Carretero, J. Am. Chem. Soc., 2008, 130, 16150–16151 CrossRef PubMed;
(g) D. Shang, Y. Liu, X. Zhou, X. Liu and X. Feng, Chem. – Eur. J., 2009, 15, 3678–3681 CrossRef CAS PubMed;
(h) H. Toribio, J. Gómez, R. Arrayás and J. C. Carretero, Chem. – Eur. J., 2010, 16, 1153–1157 CrossRef PubMed;
(i) H. Zhang, S. Syed and C. F. Barbas, Org. Lett., 2010, 12, 708–711 CrossRef CAS PubMed;
(j) J. Jiang, H. D. Xu, J. B. Xi, B. Y. Ren, F. P. Lv, X. Guo, L. Q. Jiang, Z. Y. Zhang and W. H. Hu, J. Am. Chem. Soc., 2011, 133, 8428–8431 CrossRef CAS PubMed;
(k) T. Kano, R. Sakamoto, M. Akakura and K. Maruoka, J. Am. Chem. Soc., 2012, 134, 7516–7520 CrossRef CAS PubMed.
-
(a) E. Vellemäe, O. Tšubrik, S. Mäeorg and U. Mäeorg, J. Org. Chem., 2006, 5, 149–150 Search PubMed;
(b) K. Okamoto, H. Kojiyama, H. Tsujioka and A. Sudo, Chem. Commun., 2016, 52, 11339–11342 RSC;
(c) M. Zhou, K. Li, D. Chen, R. Xu, G. Xu and W. Tang, J. Am. Chem. Soc., 2020, 142, 10337–10342 CrossRef CAS PubMed;
(d) T. R. Swaroop, Z.-Q. Wang, Q.-Y. Li and H. S. Wang, J. Electrochem. Soc., 2020, 167, 46504–46513 CrossRef CAS;
(e) H. Wang, J. P. Qu and Y. B. Kang, Org. Lett., 2021, 23, 2900–2903 CrossRef CAS PubMed;
(f) K. N. R. Dumoleijn, E. Van Den Broeck, J. Stavinoha, V. Van Speybroeck, K. Moonen and C. V. Stevens, J. Catal., 2021, 400, 103–113 CrossRef CAS;
(g) J. Cao, D. Lv, F. Yu, M. F. Chiou, Y. Li and H. Bao, Org. Lett., 2021, 23, 3184–3189 CrossRef CAS PubMed;
(h) L. Cardinale, M. W. S. Schmotz, M. O. Konev and A. J. Wangelin, Org. Lett., 2022, 24, 506–510 CrossRef CAS PubMed.
-
(a) K. Selvakumar and J. F. Harrod, Angew. Chem., Int. Ed., 2001, 40, 2129–2131 CrossRef CAS;
(b) M. J. MacDonald, D. J. Schipper, P. J. Ng, J. Moran and A. M. Beauchemin, J. Am. Chem. Soc., 2011, 133, 20100–20103 CrossRef CAS PubMed;
(c) Q. Qin, Y. Y. Han, Y. Y. Jiao, Y. He and S. Yu, Org. Lett., 2017, 19, 2909–2912 CrossRef CAS PubMed;
(d) K. Muniz, L. Barreiro, R. M. Romero and C. Martinez, J. Am. Chem. Soc., 2017, 139, 4354–4357 CrossRef CAS PubMed;
(e) N. K. Fu, G. S. Sauer, A. Saha, A. Loo and S. Lin, Science, 2017, 357, 575–579 CrossRef CAS PubMed;
(f) F. Liu, G. Zhao, W. Cai, D. Xu and B. Zhao, Org. Biomol. Chem., 2018, 16, 7498–7502 RSC;
(g) L. Yu and P. Somfai, Angew. Chem., 2019, 131, 8639–8643 CrossRef;
(h) S. Minakata, H. Miwa, K. Yamamoto, A. Hirayama and S. Okumura, J. Am. Chem. Soc., 2021, 143, 4112–4118 CrossRef CAS PubMed;
(i) G. Tan, M. Das, R. Kleinmans, F. Katzenburg, C. Daniliuc and F. Glorius, Nat. Catal., 2022, 5, 1120–1130 CrossRef CAS;
(j) Y. Zheng, Z. J. Wang, Z. P. Ye, K. Tang, Z. Z. Xie, J. A. Xiao, H. Y. Xiang, K. Chen, X.-Q. Chen and H. Yang, Angew. Chem., Int. Ed., 2022, 61, e202212292 CrossRef CAS PubMed;
(k) C. Schneider, S. Peruncheralathan and M. Henze, Synlett, 2007, 2289–2291 CrossRef.
-
(a) P. Saravanan, T. Henrik and S. Christoph, Angew. Chem., Int. Ed., 2009, 48, 4849–4852 CrossRef PubMed;
(b) C. Schneider, A. Marti and L. Richter, Synlett, 2011, 2513–2516 CrossRef;
(c) S. Peruncheralathan, S. Aurich, H. Teller and C. Schneider, Org. Biomol. Chem., 2013, 11, 2787–2803 RSC;
(d) Z. Chai, P. J. Yang, H. Zhang, S. Wang and G. S. Yang, Angew. Chem., Int. Ed., 2016, 56, 650–654 CrossRef PubMed.
-
(a) X. Zhu and H. Du, Org. Lett., 2015, 17, 3106–3109 CrossRef CAS PubMed;
(b) E. Papadaki and V. Magrioti, Tetrahedron Lett., 2019, 61, 151419–151426 CrossRef;
(c) H. J. Pan, Y. Lin, T. Gao, K. K. Lau, W. Feng, B. Yang and Y. Zhao, Angew. Chem., Int. Ed., 2021, 60, 18599–18604 CrossRef CAS PubMed.
-
(a) S. Talukdar and A. Banerji, J. Org. Chem., 1998, 63, 3468–3470 CrossRef CAS;
(b) H. C. Aspinall, N. Greeves and S. L. F. Hin, Tetrahedron Lett., 2010, 51, 1558–1561 CrossRef CAS;
(c) K. Kitajima, R. Nagatomo and T. Fujimoto, Tetrahedron, 2015, 71, 977–981 CrossRef CAS;
(d) H. Wang, G. Lu, G. J. Sormunen, H. A. Malik, P. Liu and J. Montgomery, J. Am. Chem. Soc., 2017, 139, 9317–9324 CrossRef CAS PubMed;
(e) B. V. Rokade and P. J. Guiry, ACS Catal., 2017, 7, 2334–2338 CrossRef CAS.
-
(a) B. Lu, Y. Cheng, L. Y. Chen, J. R. Cheng and W. J. Xiao, ACS Catal., 2019, 9, 8159–8164 CrossRef CAS;
(b) H. P. Lv, R. D. Laishram, J. Yang, J. Y. Li, Y. Y. Zhou, D. D. Xu, S. More, Y. Z. Da and B. M. Fan, Green Chem., 2019, 21, 4055–4061 RSC;
(c) J. B. Xu, N. Liu, H. P. Lv, C. X. He, Z. N. Liu, X. F. Shen, F. X. Cheng and B. M. Fan, Green Chem., 2020, 22, 2739–2743 RSC;
(d) X. Zhao, X. L. Feng, F. Chen, S. Q. Zhu, F. L. Qing and L. L. Chu, Angew. Chem., Int. Ed., 2021, 60, 26511–26517 CrossRef CAS PubMed;
(e) W. Wu, F. Q. Zhang, N. Liu, Z. X. Wei, J. B. Xu, Z. X. He, Y. F. Guo and B. M. Fan, Asian J. Org. Chem., 2022, 11, 2022–00336 Search PubMed;
(f) P. J. Pedersen, D. C. Blakemore, G. M. Chinigo, T. Knauber and D. W. C. MacMillan, J. Am. Chem. Soc., 2023, 145, 21189–21196 CrossRef CAS PubMed;
(g) Y. Guo, X. Wang, C. Li, J. Su, J. Xu and Q. Song, Nat. Commun., 2023, 14, 5693 CrossRef CAS;
(h) C. X. Pan, Y. Y. Meng, Y. Deng, B. J. Zhou, J. C. Chen, Z. X. He, W. Q. Sun, R. Khan and B. M. Fan, Chin. J. Chem., 2022, 40, 2040–2046 CrossRef CAS;
(i) H. M. Wang, W. Y. Shi, Y. L. Li, M. M. Yu, Y. Gao and A. W. Lei, CCS Chem., 2022, 3, 1710–1717 CrossRef.
- S. Okamoto, R. Ariki, H. Tsujioka and A. Sudo, J. Org. Chem., 2017, 82, 9731–9736 CrossRef CAS PubMed.
- E. Fava, A. Millet, M. Nakajima, S. Loescher and M. Rueping, Angew. Chem., Int. Ed., 2016, 55, 6776–6779 CrossRef CAS PubMed.
- A. Gualandi, G. Rodeghiero, E. D. Rocca, F. Bertoni, M. Marchini, R. Perciaccante, T. P. Jansen, P. Ceroni and P. G. Cozzi, Chem. Commun., 2018, 54, 10044–10047 RSC.
- C.-C. Wang, Y. Tang, Y.-H. Pan, J. Yang and Y.-M. Zhang, Tetrahedron Lett., 2015, 56, 2863–2866 CrossRef CAS.
-
(a) L. C. Portis, V. V. Bhat and C. K. Mann, J. Org. Chem., 1970, 35, 2175–2178 CrossRef CAS;
(b) Y. J. Lu, X. Zhang, S. Malakar, K. Krogh-Jespersen, F. Hasanayn and A. S. Goldman, J. Org. Chem., 2020, 85, 3020–3028 CrossRef CAS PubMed;
(c) H. Zhao and D. Leonori, Angew. Chem., Int. Ed., 2021, 60, 7669–7674 CrossRef CAS PubMed;
(d) N. E. S. Tay, D. Lehnherr and T. Rovis, Chem. Rev., 2022, 122, 2487–2649 CrossRef CAS PubMed;
(e) J. C. Bawden, P. S. Francis, S. DiLuzio, D. J. Hayne, E. H. Doeven, J. Truong, R. Alexander, L. C. Henderson, D. E. Gomez, M. Massi, B. I. Armstrong, F. A. Draper, S. Bernhard and T. U. Connell, J. Am. Chem. Soc., 2022, 144, 11189–11202 CrossRef CAS.
-
(a) S. I. Murahashi, T. Naota and K. Yonemura, J. Am. Chem. Soc., 1988, 110, 8256–8258 CrossRef CAS;
(b) R. J. Carroll, H. Leisch, E. Scocchera, T. Hudlicky and D. P. Cox, Adv. Synth. Catal., 2008, 350, 2984–2992 CrossRef CAS;
(c) O. I. Afanasyev, E. Kuchuk, D. L. Usanov and D. Chusov, Chem. Rev., 2019, 119, 11857–11911 CrossRef CAS PubMed;
(d) S. Firoozi and M. Hosseini-Sarvari, J. Org. Chem., 2021, 86, 2117–2134 CrossRef CAS PubMed;
(e) Y. Shen and T. Rovis, J. Am. Chem. Soc., 2021, 143, 16364–16369 CrossRef CAS PubMed;
(f) A. A. Najmi, R. Bischoff and H. P. Permentier, Molecules, 2022, 27, 3293–3326 CrossRef CAS PubMed;
(g) Q. Gao, Y. Guo, Z. Sun, X. He, Y. Gao, G. Fan, P. Cao, L. Fang, S. Bai and Y. Jia, Org. Lett., 2023, 25, 109–114 CrossRef CAS PubMed.
|
This journal is © The Royal Society of Chemistry 2024 |