DOI:
10.1039/D3GC04272C
(Communication)
Green Chem., 2024,
26, 1302-1305
Beyond acceptable limits: intrinsic contamination in commercial 15N2 impedes reliable N2 reduction experiments†
Received
4th November 2023
, Accepted 4th January 2024
First published on 8th January 2024
Abstract
The validity of N2 reduction experiments relies on control experiments with isotopically labeled 15N2. Here we discovered significant 15NH3 contamination (73 ppm) in commercial 15N2 and also observed the prescence of oxidized 15N in amounts comparable to the typical reported ammonia yields.
Introduction
The Haber–Bosch (HB) process, with its high carbon intensity (4.21 tCO2/tNH3), accounts for about 1.3% of the world's CO2 emissions through the production of ammonia.1,2 Over the past few years, various alternative synthesis routes for ammonia have been proposed. Amongst them is the direct nitrogen reduction reaction (NRR) in aqueous conditions (eqn (3)). This approach omits the use of a carbon-based hydrogen source and can operate at pressures and temperatures close to ambient. However, irrefutable experimental evidence for actual (photo)electrochemical ammonia formation at the cathode is typically elusive, this is mainly due to the extremely low reported ammonia production rates (10−12–10−9 mol s−1). Moreover, the omnipresence of reactive nitrogen species, which are more easily converted to ammonia than the extremely stable dinitrogen, has led to concerns about false positives in many studies. In response, validation strategies as well as rigorous experimental protocols have been implemented. While most studies stress the use of high-purity reagents, blank tests, controls, etc., the cornerstone of NRR validation lies in the use of isotopically labelled nitrogen gas (15N2). Detection of 15NH3 post reaction is typically taken as an indicator of NRR occurrence. However, until now, there has been a lack of comprehensive information regarding the purity of commercial 15N2 gas, and the impact of activated, 15N labelled impurities on the measured ammonia yield. Furthermore, there is a notable absence of precise, quantitative comparison between NRR experiments conducted under 14N2 and 15N2.
False positives and contaminants in the 14N2 feed gas
In a typical electrochemical NRR experiment, nitrogen gas is bubbled through the cathode compartments’ aqueous electrolyte solution, where it serves as nitrogen source to generate ammonia. Depending on the pH, either gaseous NH3 or dissolved NH+4 is formed (eqn (1.1) and (1.2)). The required protons originate from the oxidation of water, conducted in a separate anode compartment (eqn (2.1) and (2.2)). The cathode's production rate and selectivity are typically determined by quantifying the ammonia yield over the course of a few hours.
Cathode:
pH = 0
| N2 (g) + 6e− + 8H+ (aq) → 2NH+4 (aq) E° = +0.275 V | (1.1) |
pH = 14
| N2 (g) + 6e− + 6H2O (l) → 2NH3 (g) + 6OH− (aq) E° = −0.772 V | (1.2) |
Anode:
pH = 0
| 2H2O (l) → O2 (g) + 4e− + 4H+(aq) E° = −1.229 V | (2.1) |
pH = 14
| 4OH− (aq) → O2 (g) + 4e− + 2H2O (l) E° = −0.401 V | (2.2) |
Overall:
| 2N2 (g) + 6 H2O (l) → 4 NH3 + 3 O2 E°cell = −1.17 V | (3) |
Considering that the reported ammonia production rates are typically small (10−12–10−9 mol s−1 cm−2 of cathode), the challenge lies in verifying that the measured ammonia indeed originates from N2 reduction and not from more easily reducible, activated nitrogen species such as NO−3, NO−2, NO, NO2, NH2OH, etc. These latter species are often found as contaminants in the reaction system, for instance, being inherently present in the electrolytes, catalyst materials and/or the feed gasses.3,4 While the concentration of these activated nitrogen species in the reaction system can be reduced, they cannot be entirely eliminated. Notably, even under optimal conditions, their amounts match typical ammonia yields.
A key distinction exists between oxidized nitrogen species like NO−3 or NOx and NH3/NH+4. While background levels of NH3 (g) and NH+4 (aq.) in the experimental setup can be factored in via blank tests and careful analysis of the reagents and electrodes, identifying contributions from oxidized nitrogen species poses a more significant challenge. Moreover, the ubiquitous and heavily fluctuating presence of ammonia in the atmosphere and on labware, complicate the accurate quantification of a background level.5–7
One contamination source of oxidized nitrogen species is the feed gas. Our mass spectrometry results (ESI Fig. 2a†) reveal that a commercial, high-purity (N6) 14N2 gas cylinder contains approximately 52 ppb of 14NH3, 254 ppb of 14NO, and 22 ppb of 14NO2 (Table 1). The 14NOx and 14NH3 contamination levels found in a lower purity (N3) 14N2 gas was found to be comparable and in the same order of magnitude as the 220 ppb of 14N2O reported previously by Choi et al. in N5 purity 14N2.5 In these feed gasses, the background level of ammonia is approximately 5–6 times lower than the total amount of oxidized nitrogen species. For typical NRR experiments with 14N2 feed gas flowing through the cell at 100 ml min−1, the total amount of oxidized nitrogen species introduced from high purity 14N2 gas (N6) can be expected in the order of 10−11mol cm−2 s−1 (for a cathode of 1 cm2). As this molar flux of contaminants matches the ammonia production rate of many reported electrocatalysts, it is important to consider that reduction of contaminants in the feed gas alone could potentially account for the entire ammonia output, overshadowing the reduction of N2.
Table 1 Absolute concentrations of NH3, NO and NO2 in commercially obtained high purity (N6) and low purity (N3) 14N2 gas as well as 15N2 (≥98 at%), determined by SIFT-MS
|
Background NH3 |
Oxidized nitrogen species |
14NH3 (ppb) |
14NO (ppb) |
14NO2 (ppb) |
14N2O (ppb) |
14N2 (N6 purity) |
52 |
254 |
22 |
220a |
14N2 (N3 purity) |
76 |
220 |
34 |
NA |
|
15NH3 (ppb) |
15NO (ppb) |
15NO2 (ppb) |
15N2O (ppb) |
N2O concentration reported by Choi et al. for commercially obtained 14N2 (N5) and 15N2 (N3).
|
15N2 (98 at%) |
73 293 |
659 |
20 |
230a |
The credibility of a given NRR study is primarily based on the ammonia production rate of the cathode. Consequently, thresholds to determine the plausibility of electrochemical NRR have been suggested by Choi et al.5 Given typical contamination levels, electrodes exhibiting production rates lower than 10−10 mol cm−2 s−1 are not considered promising, while promising NRR experiments should present ammonia production rate exceeding 10−8 mol cm−2 s−1. Taking this threshold and the above mentioned feed-gas contaminant quantification into account; at modest flow rates, the risk of measuring false positives due to contaminated feed gas is rather limited if high purity (N6) 14N2 gas is used. Obviously, this needs to be assessed for each individual case and researchers should always establish background contamination levels prior to performing NRR experiments.
Contamination levels of commercial 15N2 gas
While the contamination level in high-purity (N6) 14N2 gas is relatively minor compared to the literature-reported ammonia production thresholds, contamination in the form of NH3/NH+4 and oxidized nitrogen (14N) species such as NO−3, NO−2, NH2OH, etc., present in the used electrolytes, chemicals, and labware is significantly higher – orders of magnitude above the threshold for plausible NRR. As an example, Li et al. measured a NO−3 concentration of 11 ppm in an aqueous 0.5 M Li2SO4 electrolyte solution.7 Distinctly separating the reduction of these contaminants to ammonia from the NRR proves even more challenging than eliminating the contribution of background NH3/NH+4 in the system. As a consequence, adopting isotopically labelled N2 gas (15N2) and subsequent quantification of the produced 15NH3 has been proposed as a valid strategy to confirm ammonia production truly originates from N2.5,8 By switching to 15N2 feed gas, the interference of previously mentioned contaminants decreases substantially, as the natural abundance of the 15N isotope is only 0.33–0.42%.9
Previous research by Choi and Dabundo pointed out the existence of contaminants such as 15NOx in commercial 15N2 gas. However, these studies were limited in their capability to provide precise quantifications of 15N labelled contaminants.10 Dabundo's approach relied on indirect means of measurement, identifying the presence but not the exact amounts of reactive 15N species in the gas.10 In contrast, our study employs mass spectrometry to explicitly quantify both oxidized 15N species (15NO and 15NO2) and 15NH3 in the gas phase. Alarmingly, our findings show that commercial 15N2 is considerably contaminated with 15NH3 (Table 1, ESI Fig. 3†) (approx. 73 ppm), consistent with dissolved 15NH+4 concentrations reported by Dabundo (34–1900 ppm). Herein lies a profound risk of measuring false positives: using as-obtained 15N2 gas bubbled at a 100 ml min−1 flow rate either during the pre-experimental electrolyte saturation step or consistently throughout the entire experiment introduces sufficient 15NH3 to account for an apparent ammonia yield of approximately 1 × 10−8 mol s−1. This exceeds most reported ammonia production rates by factors of 100–1000. It is important to note that despite the evident risks, many recent NRR studies continue to use unpurified 15N2, even though pre-experimental gas purification has been suggested.5,8,11–13
NRR experiments using 15N2 and 14N2
To effectively demonstrate the impact of 15N labelled impurities on the reported ammonia yield, we conducted NRR experiments at a constant potential (−0.91 V vs. RHE), using bismuth, reportedly a highly active element for N2 reduction, as cathode.14 We performed two sets of experiments under 14N2 and 15N2 saturated conditions (Fig. 1). Regularly sampling the catholyte revealed a measurable increase in ammonium content in the 15N2 experiment. Specifically, after four hours, the 15N2 experiment showed 8.6 μmol of NH+4, compared to only 0.68 μmol NH+4 in the 14N2 experiment (Fig. 2). This result clearly demonstrates that when using unpurified 15N2 even at low flow rates (20 mL min−1), a considerable amount of ammonia will be detected, primarily originating from the 15N labelled impurities rather than from the direct reduction of 15N2. If the 15N2 contamination were overlooked, an apparent ammonia yield of 6 × 10−10 mol s−1 would be calculated for the 15N2 experiment. This figure is over ten times higher than the ammonia yield (5 × 10−11 mol s−1) of the same experiment under 14N2.
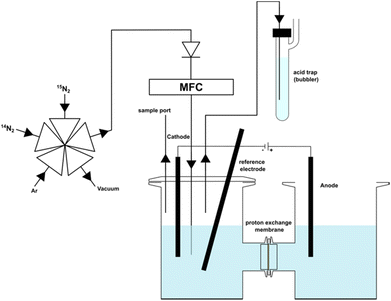 |
| Fig. 1 Schematic representation of the setup used for studying the NRR under 14N2 or 15N2 atmospheres. NRR experiments were conducted in an H-cell configuration. Feed gases (14N2 and 15N2) were continuously bubbled into the cathode compartment at a predefined flow rate of 20 mL min−1. An acid trap was employed at the exit to completely isolate the cathode compartment from the surrounding atmosphere and to trap any gaseous ammonia. | |
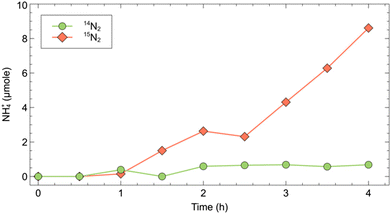 |
| Fig. 2 Cumulative ammonium production (15NH+4 or 14NH+4) in a four hour NRR experiment with a Bismuth cathode. | |
Mitigation strategies for the 15N contaminants
Our precise quantification reveals that the level of oxidized 15N species in this gas is nearly three times higher than in both high (N6) and low purity (N3) 14N2 gases, exacerbating the risk of false positives in control experiments. Purifying commercial 15N2 gas, which comes in extremely limited volumes of 500–1000 cc at 1 to 5 atm, presents significant technical and economic challenges for NRR research. Traditional methods for purification, such as bulky, home-made scrubbers or acid traps, are neither practical nor effective in removing all 15N contaminants, and even more advanced solutions like small, commercial gas filters require complex, leak-tight setups for recirculating the limited 15N2 supply. Moreover, to ensure data reliability, continuous monitoring of activated 15Nx species using sensitive techniques like mass spectrometry is necessary, as is the tracking of 15NH+4 levels in solution over time, rather than relying on a single post-reaction measurement, as is often performed nowadays.
Given these constraints, the risk of false positives in isotopic control experiments for NRR studies is significantly larger when using 15N2 gas than 14N2, regardless of purity level. This casts serious doubt on the reliability and cost-effectiveness of using 15N2 gas as it currently stands and highlights the urgent need for alternative validation methods in NRR research that can reliably account for these challenges.
Conclusion
In recent years, the predominant strategy to exclude false positives in NRR experiments has centered on using isotopically labelled 15N2 gas as a feed. While Choi et al. warned researchers about potential interferences from isotopically labelled reactive nitrogen species in these gasses, a comprehensive understanding of the precise contamination levels of these gaseous, 15N labelled contaminants remained elusive. In this work, we quantify the exact levels of 15Nx contaminants in commercially obtained 15N2, typically used for performing NRR control experiments. Alarmingly, the contamination levels in these gasses make them unsuitable, increasing the chances of obtaining false positives. Thus, given the current gas purity, validation experiments with 15N2 do not enhance the credibility of the NRR study when the production rate is low (e.g. <10−8 mol cm−2 s−1), neither do they confirm NRR is occurring. Moreover, the 15N labelling experiments might even mislead researchers into wrongly attributing the measured 15NH3 to 15N2 reduction at the cathode; as we clearly demonstrated in our comparative NRR experiments. Indeed, the ammonia production rates originating from 15N labelled impurities in the feed gas is in the order 10−9–10−10 mol s−1, which is comparable to most reported NRR studies in aqueous conditions. Therefore, the most convincing proof for successful NRR is consistently measuring high ammonia yields (e.g. >10−8 mol s−1), particularly when using purer 14N2, which is more realistic to purify, over the scarce 15N2.
Author contributions
M. D. R wrote the paper and assisted in performing the SIFT-MS measurements. M. B. J. R. participated in the writing and editing of the manuscript. L. H., J. A. M. and J. H. participated in the revision of the manuscript. T. L., B. M. N. and M. L. A. T. M. H contributed to scientific discussions and setting up the methodology.
Conflicts of interest
There are no conflicts to declare.
Acknowledgements
We thank Tessa Vanempten for her assistance with the SIFT-MS measurements and subsequent quantification. M. D. R. acknowledges the Research Foundation Flanders (FWO) for his personal grant (1SA3321N). J. H. and M. B. J. R gratefully acknowledge the financial support from the Research Foundation-Flanders (FWO, Grant No. G0983.19N, G0F2322N, VS06523N), Interne Fondsen KU Leuven through project (C3/20/067 and CELSA/21/016). J. H. acknowledges the Flemish Government Through Long-Term Structural Funding Methusalem (CASAS2, Meth/15/04) and the Moonshot cSBO project P2C (HBC.2019.0108), and the MPI as MPI fellow.
References
- R. Nayak-Luke, R. Bañares-Alcántara and I. Wilkinson, Ind. Eng. Chem. Res., 2018, 57, 14607–14616 CrossRef CAS.
-
P. Gilbert and P. Thornley, Energy and carbon balance of ammonia production from biomass gasification, ed. A. V. Bridgwater, Proceedings of the Bioten Conference on Biomass, Bioenergy and Biofuels, Host publication, 2010 Search PubMed.
- J. Kibsgaard, J. K. Nørskov and I. Chorkendorff, ACS Energy Lett., 2019, 4, 2986–2988 CrossRef CAS.
- L. F. Greenlee, J. N. Renner and S. L. Foster, ACS Catal., 2018, 8, 7820–7827 CrossRef CAS.
- J. Choi, B. H. R. Suryanto, D. Wang, H.-L. Du, R. Y. Hodgetts, Federico M. Ferrero Vallana, D. R. MacFarlane and A. N. Simonov, Nat. Commun., 2020, 11, 5546 CrossRef CAS PubMed.
- B. Hu, M. Hu, L. Seefeldt and T. L. Liu, ACS Energy Lett., 2019, 4, 1053–1054 CrossRef CAS.
- L. Li, C. Tang, D. Yao, Y. Zheng and S.-Z. Qiao, ACS Energy Lett., 2019, 4, 2111–2116 CrossRef CAS.
- S. Z. Andersen, V. Čolić, S. Yang, J. A. Schwalbe, A. C. Nielander, J. M. McEnaney, K. Enemark-Rasmussen, J. G. Baker, A. R. Singh, B. A. Rohr, M. J. Statt, S. J. Blair, S. Mezzavilla, J. Kibsgaard, P. C. K. Vesborg, M. Cargnello, S. F. Bent, T. F. Jaramillo, I. E. L. Stephens, J. K. Nørskov and I. Chorkendorff, Nature, 2019, 570, 504–508 CrossRef CAS PubMed.
- J. R. de Laeter, J. K. Böhlke, P. De Bièvre, H. Hidaka, H. S. Peiser, K. J. R. Rosman and P. D. P. Taylor, Pure Appl. Chem., 2009, 81, 1535–1536 CrossRef CAS.
- R. Dabundo, M. F. Lehmann, L. Treibergs, C. R. Tobias, M. A. Altabet, P. H. Moisander and J. Granger, PLoS One, 2014, 9, e110335 CrossRef PubMed.
- Y. Ashida, Y. Onozuka, K. Arashiba, A. Konomi, H. Tanaka, S. Kuriyama, Y. Yamazaki, K. Yoshizawa and Y. Nishibayashi, Nat. Commun., 2022, 13, 7263 CrossRef CAS PubMed.
- P. L. Arnold, T. Ochiai, F. Y. T. Lam, R. P. Kelly, M. L. Seymour and L. Maron, Nat. Chem., 2020, 12, 654–659 CrossRef CAS PubMed.
- Q. Wang, J. Pan, J. Guo, H. A. Hansen, H. Xie, L. Jiang, L. Hua, H. Li, Y. Guan, P. Wang, W. Gao, L. Liu, H. Cao, Z. Xiong, T. Vegge and P. Chen, Nat. Catal., 2021, 4, 959–967 CrossRef CAS.
- Y.-C. Hao, Y. Guo, L.-W. Chen, M. Shu, X.-Y. Wang, T.-A. Bu, W.-Y. Gao, N. Zhang, X. Su, X. Feng, J.-W. Zhou, B. Wang, C.-W. Hu, A.-X. Yin, R. Si, Y.-W. Zhang and C.-H. Yan, Nat. Catal., 2019, 2, 448–456 CrossRef CAS.
Footnote |
† Electronic supplementary information (ESI) available: Materials and methods, technical drawing of the custom setup and obtained SIFT-MS data. See DOI: https://doi.org/10.1039/d3gc04272c |
|
This journal is © The Royal Society of Chemistry 2024 |