DOI:
10.1039/D3GC02897F
(Paper)
Green Chem., 2024,
26, 323-329
Visible-light-driven graphitic carbon nitride-catalyzed ATRA of alkynes: highly regio- and stereoselective synthesis of (E)-β-functionalized vinylsulfones†
Received
4th August 2023
, Accepted 12th October 2023
First published on 17th October 2023
Abstract
Heterogeneous photocatalysis has emerged as a powerful and sustainable technique in organic synthesis; however, the application of graphitic carbon nitride (g-C3N4) as a heterogeneous photocatalyst for organic transformations is still in its infancy. The development of novel organic transformations catalyzed by g-C3N4 is actively being pursued by chemists but it remains challenging. Herein, we describe a visible-light-driven g-C3N4-catalyzed atom transfer radical addition (ATRA) of alkynes to synthesize valuable (E)-β-thio/seleno vinylsulfones. This approach features heterogeneous photocatalysis, excellent regio- and stereoselectivities, 100% atom economy, a metal- and additive-free nature, and broad substrate scope covering (hetero)aryl and alkyl alkynes, especially the industrial feedstock propyne. Furthermore, this method can be applied to the late-stage functionalization of complex molecules. Notably, g-C3N4 can be recovered and reused in five runs without loss of catalytic activity. Mechanistic studies demonstrate that the reaction occurs via an energy transfer process rather than single electron transfer.
Introduction
Photoredox catalysis has attracted great interest from chemists not only because of the current global energy and environmental crisis, but also because it is a powerful method for the development of new and valuable organic transformations.1 The most common photoredox approaches employ homogeneous photocatalysts such as transition-metal complexes based on [Ir] or [Ru]2 and organic dyes.3 However, these homogeneous photocatalysts are difficult to recycle and reuse, which may cause high costs and environmental issues. Furthermore, they also suffer from the limitations of incompatibility with reactive radical intermediates, strong nucleophiles and electrophiles, or strong acidic and basic reaction media.4 Therefore, growing interest has been focused on using metal-free, recyclable, and stable materials as photocatalysts in visible light-induced reactions.
Polymeric graphitic carbon nitride (g-C3N4) as a heterogeneous photocatalyst has opened up new vistas in organic synthesis because of its unique properties as described here (Scheme 1). (1) g-C3N4 is easy to prepare from feedstock chemicals and can be recycled multiple times as a photocatalyst.5 (2) g-C3N4 has excellent stability in the presence of high temperatures (up to 600 °C), reactive radicals, and strong nucleophiles and electrophiles.6 (3) g-C3N4 is a transition-metal-free organic semiconductor, which can avoid the introduction of transition metals into late-stage functionalization reactions.7 (4) g-C3N4 possesses a suitable band gap (2.7 eV, λEx ≈ 460 nm) and favorable valence and conduction band positions for the controlled oxidation and reduction of substrates.8 (5) g-C3N4 is a two-dimensional material with rich surface characteristics and more importantly, the properties of g-C3N4 can be readily tuned by synthetic modifications.7 Recently, g-C3N4 has often been utilized in carbon dioxide reduction,9 organic pollutant degradation,10 and water splitting.11 The application of g-C3N4 as a heterogeneous photocatalyst for organic transformations is still in its infancy.12 Hence, the development of novel organic transformations catalyzed by g-C3N4 is highly desired.
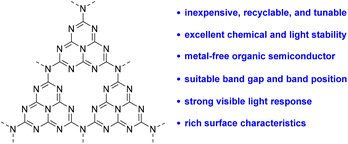 |
| Scheme 1 Properties of graphitic carbon nitride. | |
Vinylsulfones are ubiquitous in pharmaceuticals and bioactive molecules,13 and are also important organic building blocks14 (Scheme 2a). The conventional approaches for the synthesis of vinylsulfones involve addition, oxidation, and elimination reactions15 (Scheme 2b), which require tedious operations and complex purification processes, and also suffer from poor atom economy. Atom transfer radical addition (ATRA) of alkynes is a straightforward and atom economical way to construct various functionalized vinylsulfones.16 Nevertheless, the regio- and stereoselectivity control of vinylsulfones via ATRA reaction is difficult and challenging due to the involvement of highly reactive vinyl radical intermediates (Scheme 2c).17 In order to synthesize the specific configuration of (E)-β-thio/seleno vinylsulfones, Xu developed a gold and photoredox combined ATRA approach.18 Subsequently, Ji achieved Co- or Cu-catalyzed selenosulfonylation of alkynes to regioselectively synthesize β-seleno vinylsulfones, but with poor stereoselectivity.19 In the presence of eosin Y and a strong base KOH, Jia altered the regioselectivity of ATRA of alkynes to afford (E)-β-arylsulfonylvinyl sulfides.20 Although some progress in the regio- and stereoselectivity control of vinylsulfones via ATRA reaction has been made, these methods employ complex catalytic systems and extra additives, and also have limited alkyne scope mainly covering aromatic alkynes. Furthermore, the homogeneous nature of catalysts used in the process makes their separation from the product and recycling difficult. The aforementioned aspects would significantly hinder their wide practical application. In light of the unique properties of g-C3N4, we wondered if recyclable g-C3N4 could be utilized as the sole catalyst for realizing the highly regio- and stereoselective synthesis of valuable thio/seleno vinylsulfones with broader substrate scope via visible light-induced ATRA of alkynes without using metals and additives (Scheme 2d).
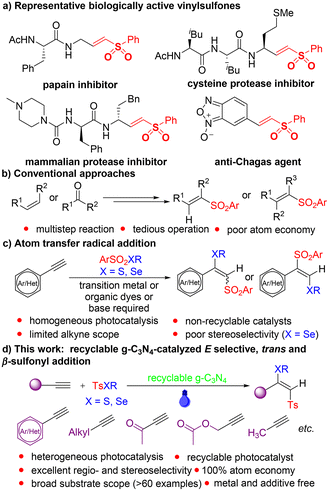 |
| Scheme 2 Synthetic strategies for functionalized vinylsulfones. | |
Results and discussion
Based on the above-mentioned proposal, we began to investigate g-C3N4-catalyzed ATRA of phenylacetylene 1a with TsSPh 2a under blue LED irradiation at room temperature. Initially, the reaction was attempted in toluene; however, no product was detected (Table 1, entry 1). Excitingly, when 1,2-dimethoxyethane (DME) was used as the solvent, the reaction exclusively gave (E)-β-sulfonyl addition product 3, although with poor yield (entry 2). The possible competitive products 3′ and 3′′ were not observed. Inspired by the above results, we further screened various solvents and found that DMSO was superior (entries 3–8). It is noteworthy that reducing the amount of g-C3N4 or 2a had a remarkable influence on the yield (entries 9 and 10). For comparison, heterogeneous photocatalysts such as BiVO4 and TiO2 and homogeneous photocatalysts such as Ru(bpy)3Cl2 and Ir(ppy)3 were also tested. The results showed that 3 was obtained with lower yields (entries 11–14). Control experiments indicated that g-C3N4 and visible light were essential to the success of this catalytic transformation (entries 15 and 16, for more optimization details, see Tables S1–S4 in the ESI†).
Table 1 Optimization of the reaction conditionsa
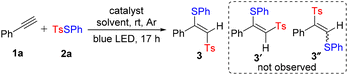
|
Entry |
Catalyst |
Solvent |
Isolated yield (%) |
Reaction conditions: 1a (0.1 mmol), 2a (0.2 mmol), heterogeneous PC (5 mg) or homogeneous PC (1 mol%) in solvent (1 mL) at room temperature under blue LED (7 W) irradiation for 17 h under argon.
g-C3N4 (3 mg).
2a (0.12 mmol).
Without light.
|
1 |
g-C3N4 |
Toluene |
NR |
2 |
g-C3N4 |
DME |
45 |
3 |
g-C3N4 |
THF |
67 |
4 |
g-C3N4 |
CH3CN |
Trace |
5 |
g-C3N4 |
CH2Cl2 |
73 |
6 |
g-C3N4 |
DMF |
88 |
7 |
g-C3N4 |
DMSO |
89 |
8 |
g-C3N4 |
Acetone |
86 |
9b |
g-C3N4 |
DMSO |
33 |
10c |
g-C3N4 |
DMSO |
51 |
11 |
BiVO4 |
DMSO |
9 |
12 |
TiO2 |
DMSO |
7 |
13 |
Ru(bpy)3Cl2 |
DMSO |
77 |
14 |
Ir(ppy)3 |
DMSO |
50 |
15 |
— |
DMSO |
11 |
16d |
g-C3N4 |
DMSO |
NR |
With the optimal reaction conditions established, we explored the generality of the method by testing various substrates 1 and 2 (Scheme 3). Notably, compared with Co- or Cu-catalyzed selenosulfonylation of alkynes with poor stereoselectivity,19 the newly developed approach could exclusively generate (E)-β-seleno vinylsulfones with a modified procedure. We first investigated different aryl alkynes and found that both electron-donating and electron-withdrawing groups at different positions (ortho, meta, or para) of phenyl rings and a naphthalene ring were well tolerated, giving the desired thio/seleno vinylsulfones 3–24 in good yields with excellent regio- and stereoselectivities. Many potentially reactive groups, such as formyl (18 and 19), nitrile (21) and ester (22), were left untouched under the standard conditions. The structure of 19 was further confirmed by X-ray crystallography. In addition, mono- and double selenosulfonylation of 1,4-diethynylbenzene (24 and 25) were realized by tuning the amount of 2. The reaction with heteroaryl alkynes including medicinally relevant heterocycles, such as pyridine (26), thiophene (27–29) and quinoline (30 and 31), also gave outstanding outcomes. Direct synthesis of complex synthetic intermediates or medicinally relevant compounds from industrial feedstock is a central goal of organic synthesis. As such, the simple commodity chemical propyne was subjected to the standard conditions, providing the corresponding products in good yields with excellent regio- and stereoselectivities (32 and 33). Besides, other acyclic and cyclic aliphatic alkynes with different functional groups such as chloro (44 and 45), acetate (46 and 47), and keto (48 and 49) were also well tolerated (34–49). The configuration of 36 was also confirmed by NOESY spectra. It is worth noting that for 1-ethynylcyclohex-1-ene, the alkyne reacted chemoselectively over the alkene (50 and 51). Subsequently, various thiosulfonates were examined and both aromatic and aliphatic thio reagents were suitable to deliver the thio vinylsulfones (52–56). More significantly, to further demonstrate the value of this protocol, we evaluated the late-stage functionalization of a series of complex alkynes derived from biologically active molecules such as estrone (57 and 58), L-phenylalanine (59), L(−)-borneol (60 and 61), atomoxetine (62 and 63), and diacetonefructose (64). The desired (E)-β-thio/seleno vinylsulfones were exclusively formed in moderate to excellent yields.
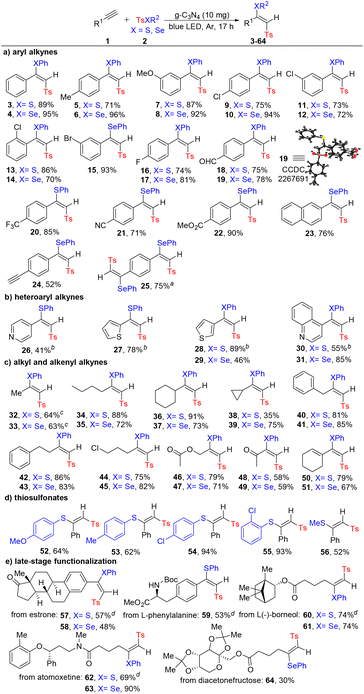 |
| Scheme 3 Substrate scope. Reaction conditions: 1 (0.2 mmol), 2 (0.4 mmol) and g-C3N4 (10 mg) in DMSO (2 mL) at rt for thiosulfonates and DCM (2 mL) at −20 °C for selenosulfonates. Isolated yields are reported. a2 (4 equiv.). b2 (5 equiv.). cPropyne (5 equiv.). dReaction time is 72 h. | |
The potential of this method was further demonstrated by large-scale synthesis of 3 with an 81% yield (Scheme 4a). Furthermore, the resulting vinylsulfones are versatile synthetic building blocks and their synthetic transformations were also explored (Scheme 4b). Upon treatment of 3 with Ni(acac)2 and MeMgBr, the coupling product 65 was obtained in 73% yield. 3 could be reduced by LiAlH4 to generate 66 in 65% yield. Noteworthily, the selective oxidation of 3 was realized under different oxidative conditions (67 and 68).
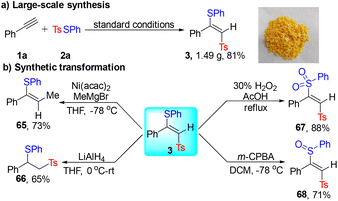 |
| Scheme 4 Synthetic applications. | |
We next studied the stability of g-C3N4 by carrying out a recycling experiment and g-C3N4 was recycled five times without losing its catalytic activity, highlighting its high potential for sustainable photocatalysis (Fig. 1a). Furthermore, X-ray powder diffraction (XRD) of recycled g-C3N4 displayed a characteristic peak at 27.6 which was consistent with that of fresh g-C3N4 (Fig. 1b). Scanning electron microscopy (SEM) showed that there was no obvious difference in the morphology of fresh g-C3N4 and recycled g-C3N4 (Fig. 1c). The characteristic peaks at 809 cm−1 of triazine and in the range of 1200–1650 cm−1 of recycled g-C3N4 were retained when analysed by Fourier-transform infrared spectroscopy (FT-IR) (Fig. 1d). These experiments demonstrated the high sustainability and photostability of g-C3N4.
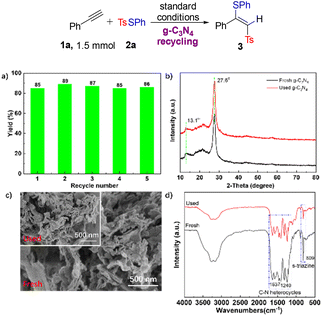 |
| Fig. 1 Evaluation of g-C3N4 recycling. aRecycling experiment. bXRD patterns. cSEM images. dFT-IR spectra. | |
To gain insight into the reaction mechanism, control experiments were conducted. When the radical scavenger TEMPO or ethene-1,1-diyldibenzene was added, the reaction was completely inhibited and the corresponding adducts were detected by HRMS, indicating the radical nature of the reaction (Scheme 5a). Furthermore, 69 underwent radical cyclization with 2a to deliver pyrrolidine 70 in 75% yield, which suggested that sulfonyl and sulfenyl radicals were involved in the reaction (Scheme 5b). To further verify the production mechanism of the sulfonyl and sulfenyl radicals, a cross-over experiment with 2b and 2c was performed and scrambled thiosulfonates 2d and 2e were obtained in 19% yield, showing that the sulfonyl and sulfenyl radicals were generated via the homolytic cleavage of the SO2–S bond rather than single electron transfer (SET) in the presence of g-C3N4 (Scheme 5c). Furthermore, the quenching of electrons and valence band holes had almost no influence on the reaction (Scheme 5a). When the standard chemical one-electron reductants (SmI2 and Zn) or an oxidant (ceric ammonium nitrate) were used instead of g-C3N4, 3 was hardly generated. On the other hand, the use of benzil as a triplet sensitizer led to the formation of 3 (Scheme 5d).21 These observations further supported that the reaction was initiated via an energy transfer pathway. In order to check if the acidic proton on the alkyne played a role in the reaction, deuterated alkyne [D]-1b was subjected to the standard conditions and 5′ was obtained with no observation of H/D exchange. Furthermore, no H/D-exchange products were detected in the NMR spectrum with D2O isotopic labeling. These experimental results indicated that the acidic proton did not participate in the reaction, which was in consistent with a mechanism involving radical addition to the triple bond (Scheme 5e). The quantum yield of the model reaction was determined to be 3.67, showing that a free radical chain mechanism might be involved in the reaction. The Hammett plot of our reaction system was constructed and a negative slope (ρ = −0.58) was observed (see Fig. S3 in the ESI†), which indicated that (1) the electron-deficient alpha-alkenyl radical was formed and that (2) this was a stepwise reaction and the radical addition to the triple bond was the rate-determining step.22
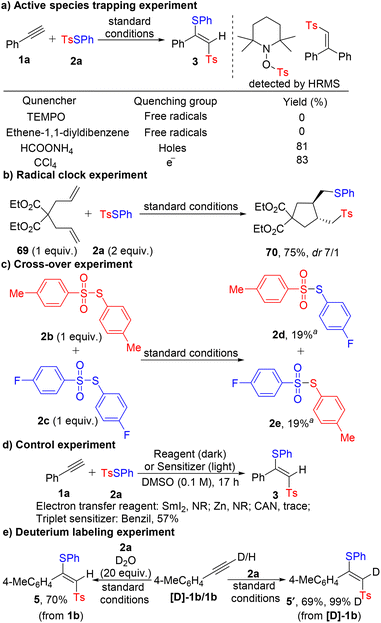 |
| Scheme 5 Mechanistic studies. a19F NMR yield with hexafluorobenzene as the internal standard. | |
Based on the experimental results and literature precedents, a plausible reaction mechanism was proposed (Scheme 6). Initially, an energy transfer to 2a from excited-state g-C3N4* occurred to yield excited 2a* and regenerate ground-state g-C3N4 under visible-light irradiation. Then, sulfenyl radical A and sulfonyl radical B were generated via the homolytic cleavage of the SO2–S bond in 2a*. The sulfonyl radical B was intercepted by phenylacetylene 1a to give α-alkenyl carbon radical C. Finally, the target product 3 was formed via the addition of A or 2a to vinyl radical C.
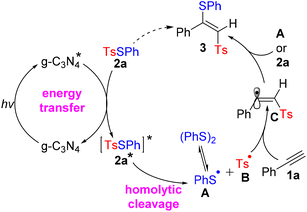 |
| Scheme 6 Proposed reaction mechanism. | |
Conclusions
In summary, we have developed a novel strategy for ATRA of alkynes to construct (E)-β-thio/seleno vinylsulfones utilizing a heterogeneous photocatalyst g-C3N4 under visible-light irradiation. Key features of this method include heterogeneous photocatalysis, excellent regio- and stereoselectivities, 100% atom economy, a metal- and additive-free nature, and broad substrate scope covering (hetero)aryl and alkyl alkynes, especially the industrial feedstock propyne. In addition, the potential of this method is further demonstrated by the late-stage modification of complex molecules and large-scale synthesis. The sustainability and photostability of g-C3N4 is also evaluated by multiple recycle experiments. Preliminary mechanistic studies indicate the involvement of an energy transfer process in the reaction. g-C3N4-catalyzed valuable organic transformations are currently being explored further in our laboratory.
Author contributions
S.-L. Xie, J.-Z. Yan, M.-J. Xie, X. Li, F. Zhou, M.-Q. Zheng, X.-L. Wang, J. Feng, Y. Zhang and Y.-N. Duan performed the experiments. Y.-D. Niu, D. Li, and H.-D. Xia wrote and revised the paper. All authors discussed the results and commented on the manuscript.
Conflicts of interest
The authors declare no competing financial interest.
Acknowledgements
This work was financially supported by the National Natural Science Foundation of China (22301094), the Guangdong Basic and Applied Basic Research Foundation (2023A1515010728, 2022A1515110829 and 2023A1515010873), the Special Fund for the Sci-Tech Innovation Strategy of Guangdong Province (STKJ202209066, 210730166882026) and the Chemistry and Chemical Engineering Guangdong Laboratory (2111008, 2011006, 2132013 and 2211003).
References
- For general reviews, see:
(a) F.-D. Lu, J. Chen, X. Jiang, J.-R. Chen, L.-Q. Lu and W.-J. Xiao, Recent advances in transition-metal-catalysed asymmetric coupling reactions with light intervention, Chem. Soc. Rev., 2021, 50, 12808–12827 RSC;
(b) Y. Chen, L.-Q. Lu, D.-G. Yu, C.-J. Zhu and W.-J. Xiao, Visible light-driven organic photochemical synthesis in China, Sci. China: Chem., 2019, 62, 24–57 CrossRef CAS;
(c) D. M. Schultz and T. P. Yoon, Solar synthesis: Prospects in visible light photocatalysis, Science, 2014, 343, 1239176 CrossRef.
-
(a) C. K. Prier, D. A. Rankic and D. W. C. MacMillan, Visible light photoredox catalysis with transition metal complexes: Applications in organic synthesis, Chem. Rev., 2013, 113, 5322–5363 CrossRef CAS;
(b) D. A. Nicewicz and D. W. C. MacMillan, Merging photoredox catalysis with organocatalysis: The direct asymmetric alkylation of aldehydes, Science, 2008, 322, 77–80 CrossRef CAS PubMed.
-
(a) N. A. Romero and D. A. Nicewicz, Organic photoredox catalysis, Chem. Rev., 2016, 116, 10075–10166 CrossRef CAS PubMed;
(b) I. Ghosh, T. Ghosh, J. I. Bardagi and B. König, Reduction of aryl halides by consecutive visible light-induced electron transfer processes, Science, 2014, 346, 725–728 CrossRef CAS PubMed.
-
(a) H.-D. Xia, Z.-L. Li, Q.-S. Gu, X.-Y. Dong, J.-H. Fang, X.-Y. Du, L.-L. Wang and X.-Y. Liu, Photoinduced copper-catalyzed asymmetric decarboxylative alkynylation with terminal alkynes, Angew. Chem., Int. Ed., 2020, 59, 16926–16932 (
Angew. Chem.
, 2020
, 132
, 17074–17080
) CrossRef CAS;
(b) J. B. McManus and D. A. Nicewicz, Direct C–H cyanation of arenes via organic photoredox catalysis, J. Am. Chem. Soc., 2017, 139, 2880–2883 CrossRef CAS;
(c) N. A. Romero, K. A. Margrey, N. E. Tay and D. A. Nicewicz, Site-selective arene C–H amination via photoredox catalysis, Science, 2015, 349, 1326–1330 CrossRef CAS PubMed;
(d) M. Majek, F. Filace and A. J. von Wangelin, On the mechanism of photocatalytic reactions with eosin Y., Beilstein J. Org. Chem., 2014, 10, 981–989 CrossRef.
- I. Ghosh, J. Khamrai, A. Savateev, N. Shlapakov, M. Antonietti and B. König, Organic semiconductor photocatalyst can bifunctionalize arenes and heteroarenes, Science, 2019, 365, 360–366 CrossRef CAS PubMed.
- Y. Wang, X. Wang and M. Antonietti, Polymeric graphitic carbon nitride as a heterogeneous organocatalyst: From photochemistry to multipurpose catalysis to sustainable chemistry, Angew. Chem., Int. Ed., 2012, 51, 68–89 (
Angew. Chem.
, 2012
, 124
, 70–92
) CrossRef CAS.
- A. Savateev, I. Ghosh, B. König and M. Antonietti, Photoredox catalytic organic transformations using heterogeneous carbon nitrides, Angew. Chem., Int. Ed., 2018, 57, 15936–15947 (
Angew. Chem.
, 2018
, 130
, 16164–16176
) CrossRef CAS PubMed.
- X. C. Wang, K. Maeda, A. Thomas, K. Takanabe, G. Xin, J. M. Carlsson, K. Domen and M. Antonietti, A metal-free polymeric photocatalyst for hydrogen production from water under visible light, Nat. Mater., 2009, 8, 76–80 CrossRef CAS.
- J. L. Lin, Z. M. Pan and X. C. Wang, Photochemical reduction of CO2 by graphitic carbon nitride polymers, ACS Sustainable Chem. Eng., 2014, 2, 353–358 CrossRef CAS.
- C. Chen, W. Ma and J. Zhao, Semiconductor-mediated photodegradation of pollutants under visible-light irradiation, Chem. Soc. Rev., 2010, 39, 4206–4219 RSC.
- G. G. Zhang, M. W. Zhang, X. X. Ye, X. Q. Qiu, S. Lin and X. C. Wang, Iodine modified carbon nitride semiconductors as visible light photocatalysts for hydrogen evolution, Adv. Mater., 2014, 26, 805–809 CrossRef CAS PubMed.
- For representative examples, see:
(a) A. Galushchinskiy, Y. Zou, J. Odutola, P. Nikačević, J.-W. Shi, N. Tkachenko, N. López, P. Farràs and O. Savateev, Insights into the role of graphitic carbon nitride as a photobase in proton-coupled electron transfer in (sp3)C–H oxygenation of oxazolidinones, Angew. Chem., Int. Ed., 2023, 62, e202301815 (
Angew. Chem.
, 2023
, 135
, e202301
) CrossRef CAS PubMed;
(b) J. Tian, L. Zhao, C. Yang, C. Yang, L. Guo and W. Xia, Four-component synthesis of spiro-imidazolidines enabled by carbon nitride photocatalysis, ACS Catal., 2023, 13, 866–876 CrossRef CAS;
(c) Q. Cao, X. Wu, Y. Zhang, B. Yang, X. Ma, J. Song and J. Zhang, Potassium-doped carbon nitride: Highly efficient photoredox catalyst for selective oxygen reduction and arylboronic acid hydroxylation, J. Catal., 2022, 414, 64–75 CrossRef CAS;
(d) V. C. Gerken and E. M. Carreira, Carbon nitride photoredox catalysis enables the generation of the dioxolanyl radical for conjugate addition reactions, ACS Catal., 2022, 12, 10787–10792 CrossRef CAS;
(e) A. J. Rieth, Y. Qin, B. C. M. Martindale and D. G. Nocera, Long-lived triplet excited state in a heterogeneous modified carbon nitride photocatalyst, J. Am. Chem. Soc., 2021, 143, 4646–4652 CrossRef CAS PubMed;
(f) F.-L. Zeng, H.-L. Zhu, X.-L. Chen, L.-B. Qu and B. Yu, Visible light-induced recyclable g-C3N4 catalyzed thiocyanation of C(sp2)–H bonds in sustainable solvents, Green Chem., 2021, 23, 3677–3682 RSC;
(g) J. Bai, S. Yan, Z. Zhang, Z. Guo and C.-Y. Zhou, Visible-light carbon nitride-catalyzed aerobic cyclization of thiobenzanilides under ambient air conditions, Org. Lett., 2021, 23, 4843–4848 CrossRef CAS;
(h) S. Mazzanti, B. Kurpil, B. Pieber, M. Antonietti and A. Savateev, Dichloromethylation of enones by carbon nitride photocatalysis, Nat. Commun., 2020, 11, 1387 CrossRef CAS PubMed;
(i) B. Ni, B. Zhang, J. Han, B. Peng, Y. Shan and T. Niu, Heterogeneous carbon nitrides photocatalysis multicomponent hydrosulfonylation of alkynes to access β-keto sulfones with the insertion of sulfur dioxide in aerobic aqueous medium, Org. Lett., 2020, 22, 670–674 CrossRef CAS;
(j) J. Guo, Y. Wang, Y. Li, K. Lu, S. Liu, W. Wang and Y. Zhang, Graphitic carbon nitride polymer as a recyclable photoredox catalyst for decarboxylative alkynylation of carboxylic acids, Adv. Synth. Catal., 2020, 362, 3898–3904 CrossRef CAS;
(k) A. Vijeta and E. Reisner, Carbon nitride as a heterogeneous visible-light photocatalyst for the Minisci reaction and coupling to H2 production, Chem. Commun., 2019, 55, 14007–14010 RSC;
(l) Y. F. Cai, Y. R. Tang, L. L. Fan, Q. Lefebvre, H. Hou and M. Rueping, Heterogeneous visible-light photoredox catalysis with graphitic carbon nitride for α-aminoalkyl radical additions, allylations, and heteroarylations, ACS Catal., 2018, 8, 9471–9476 CrossRef CAS;
(m) Y. Zhao and M. Antonietti, Visible-light-irradiated graphitic carbon nitride photocatalyzed Diels–Alder reactions with dioxygen as sustainable mediator for photoinduced electrons, Angew. Chem., Int. Ed., 2017, 56, 9336–9340 (
Angew. Chem.
, 2017
, 129
, 9464–9468
) CrossRef CAS.
-
(a) T. H. Schneider, M. Rieger, K. Ansorg, A. N. Sobolev, T. Schirmeister, B. Engels and S. Grabowsky, Vinyl sulfone building blocks in covalently reversible reactions with thiols, New J. Chem., 2015, 39, 5841–5853 RSC;
(b) B. D. Fennell, J. M. Warren, K. K. Chung, H. L. Main, A. B. Arend, A. Tochowicz and M. G. J. J. Götz, Optimization of peptidyl allyl sulfones as clan CA cysteine protease inhibitors, Enzyme Inhib. Med. Chem., 2013, 28, 468–478 CrossRef CAS;
(c) D. C. Meadows and J. Gervay-Hague, Vinyl sulfones: Synthetic preparations and medicinal chemistry applications, Med. Res. Rev., 2006, 26, 793–814 CrossRef CAS PubMed.
-
(a) B. K. Peters, T. Zhou, J. Rujirawanich, A. Cadu, T. Singh, W. Rabten, S. Kerdphon and P. G. Andersson, An enantioselective approach to the preparation of chiral sulfones by Ir-catalyzed asymmetric hydrogenation, J. Am. Chem. Soc., 2014, 136, 16557–16562 CrossRef CAS PubMed;
(b) D. Sahu, S. Dey, T. Pathak and B. Ganguly, Regioselectivity of vinyl sulfone based 1,3-dipolar cycloaddition reactions with sugar azides by computational and experimental studies, Org. Lett., 2014, 16, 2100–2103 CrossRef CAS PubMed;
(c) M. N. Noshi, A. El-awa, E. Torres and P. L. Fuchs, Conversion of cyclic vinyl sulfones to transposed vinyl phosphonates, J. Am. Chem. Soc., 2007, 129, 11242–11247 CrossRef CAS PubMed.
-
(a) M. He, A. K. Ghosh and B. Zajc, Julia olefination as a general route to phenyl (α-fluoro)vinyl sulfones, Synlett, 2008, 999–1004 CAS;
(b) H. E. Fakih, F. Pautet and H. Fillon, Sonochemical Wittig–Horner synthesis of vinyl sulfones from 4-nitro acetophenone as a model for enolisable ketones, Tetrahedron Lett., 1992, 33, 4909–4910 CrossRef;
(c) N. S. Simpkins, The chemistry of vinyl sulphones, Tetrahedron, 1990, 46, 6951–6984 CrossRef CAS.
-
(a) K. Gadde, P. Mampuys, A. Guidetti, H. Y. V. Ching, W. A. Herrebout, S. V. Doorslaer, K. A. Tehrani and B. U. W. Maes, Thiosulfonylation of unactivated alkenes with visible-light organic photocatalysis, ACS Catal., 2020, 10, 8765–8779 CrossRef CAS;
(b) Y. Fang, Z. Luo and X. Xu, Recent advances in the synthesis of vinyl sulfones, RSC Adv., 2016, 6, 59661–59676 RSC.
-
(a) W. Xie, P. Ma, Y. Zhang, L. Xi, S. Qiu, X. Huang, B. Yang, Y. Gao and J. Zhang, Visible light-induced highly regioselective and stereoselective oxysulfonylation of alkynes for the synthesis of (E)-β-phenoxy vinylsulfones, Org. Lett., 2022, 24, 6099–6104 CrossRef CAS PubMed;
(b) M. Y. Ansari, N. Kumar and A. Kumar, Regioselective intermolecular sulfur-oxygen difunctionalization (phenoxysulfonylation) of alkynes: One-pot construction of (Z)-β-phenoxy vinylsulfones, Org. Lett., 2019, 21, 3931–3936 CrossRef CAS PubMed;
(c) O. Reiser, Shining light on copper: unique opportunities for visible-light catalyzed atom transfer radical addition reactions and related processes, Acc. Chem. Res., 2016, 49, 1990–1996 CrossRef CAS;
(d) U. Wille, Radical cascades initiated by intermolecular radical addition to alkynes and related triple bond systems, Chem. Rev., 2013, 113, 813–853 CrossRef CAS.
-
(a) T. Song, H. Li, F. Wei, C.-H. Tung and Z. Xu, Gold/photoredox-cocatalyzed atom transfer thiosulfonylation of alkynes: Stereoselective synthesis of vinylsulfones, Tetrahedron Lett., 2019, 60, 916–919 CrossRef CAS;
(b) H. Li, Z. Cheng, C.-H. Tung and Z. Xu, Atom transfer radical addition to alkynes and enynes: A versatile gold/photoredox approach to thio-functionalized vinylsulfones, ACS Catal., 2018, 8, 8237–8243 CrossRef CAS.
- R. Zhang, P. Xu, S.-Y. Wang and S.-J. Ji, Visible light-induced Co- or Cu-catalyzed selenosulfonylation of alkynes: Synthesis of β-(seleno)vinyl sulfones, J. Org. Chem., 2019, 84, 12324–12333 CrossRef CAS PubMed.
- Z. Peng, H. Yin, H. Zhang and T. Jia, Regio- and stereoselective photoredox-catalyzed atom transfer radical addition of thiosulfonates to aryl alkynes, Org. Lett., 2020, 22, 5885–5889 CrossRef CAS.
- W. Lee, Y. Koo, H. Jung, S. Chang and S. Hong, Energy-transfer-induced [3+2] cycloadditions of N-N pyridinium ylides, Nat. Chem., 2023, 15, 1091–1099 CrossRef CAS.
-
(a) J. Ren, K. Liu, N. Wang, X. Kong, J. Li and K. Li, Copper-catalyzed 1,2-difunctionalization trifluoromethylamidation of alkynes assisted by a coordinating group, ACS Catal., 2023, 13, 11001–11011 CrossRef CAS;
(b) O. Ito and M. D. C. M. Fleming, Substituent effects on the free-radical addition reactions of arylthiyl radicals with arylacetylenes, J. Chem. Soc., Perkin Trans. 2, 1989, 2, 689–693 RSC.
Footnotes |
† Electronic supplementary information (ESI) available. CCDC 2267691. For ESI and crystallographic data in CIF or other electronic format see DOI: https://doi.org/10.1039/d3gc02897f |
‡ These authors contributed equally to this work. |
|
This journal is © The Royal Society of Chemistry 2024 |